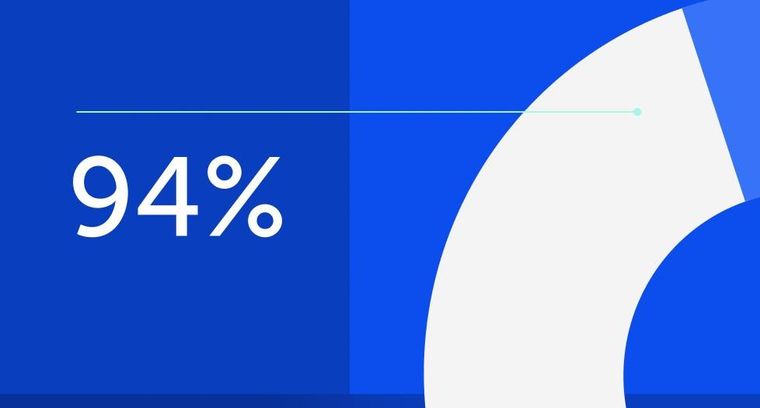
94% of researchers rate our articles as excellent or good
Learn more about the work of our research integrity team to safeguard the quality of each article we publish.
Find out more
ORIGINAL RESEARCH article
Front. Mar. Sci., 08 February 2019
Sec. Marine Ecosystem Ecology
Volume 6 - 2019 | https://doi.org/10.3389/fmars.2019.00038
This article is part of the Research TopicSeaFlower Biosphere Reserve: New Findings and Trends in the Largest Caribbean Marine Protected AreaView all 14 articles
Historical records of growth rates of the key Caribbean reef framework-building coral Orbicella faveolata can be fundamental not only to understand how these organisms respond to environmental changes but also to infer future responses of reef ecosystems in a changing world. While coral growth rates have been widely documented throughout the Caribbean, the drivers of coral growth variability remain poorly understood. Here, we provide a record spanning 53 years (1963–2015) of the coral growth parameters for five O. faveolata core samples collected at Serrana Atoll, inside the Seaflower Biosphere Reserve, Colombian Caribbean. Coral cores were extracted from reefs isolated from direct anthropogenic impacts, and growth estimations (skeletal density, linear extension, and calcification rates) were derived using computerized tomography. Master records of coral growth parameters were evaluated to identify long-term trends and to relate growth responses with sea surface temperature (SST), the Atlantic Multi-decadal Oscillation (AMO), North Atlantic Oscillation (NAO) and Southern Oscillation indexes, aragonite saturation state (Ωarag), and degree heating months (DHM). We found significant negative relationships between density and mean SST, maximum SST, AMO, and DHM. Moreover, density showed significant positive correlations with NAO and Ωarag. Extension rate did not show significant correlations with any environmental variable. However, there were significant negative correlations between calcification and maximum SST, AMO, and DHM. Trends of coral growth indicated a significant reduction in density and calcification over time, which were best explained by changes in Ωarag. Inter-annual declines in calcification and density up to 25% (relative to historical mean) were associated to the impacts of previously recorded mass bleaching events (1998, 2005, and 2010). Our study provides further evidence that AMO and Ωarag are important drivers affecting coral growth rates in the Southwestern Caribbean. Therefore, we suggest upcoming variations of AMO and future trajectories of Ωarag in the Anthropocene could have a substantial influence on future disturbances, ecological process and responses of the Caribbean reefs.
Interpreting coral growth records is critical to identifying responses of coral reefs to environmental conditions over time and to predict future trajectories of these ecosystems (Pandolfi, 2011). For instance, the annual density banding of massive coral skeletons allows retrospective analysis of different coral growth parameters such as skeletal density, linear extension and calcification rates (Lough and Barnes, 1992). Because coral growth responses are determined by changes in environmental conditions (Lough and Cooper, 2011), it is possible to associate the variability in annual growth with previously known environmental and climatic conditions and disturbances (Bessat and Buigues, 2001; Lough, 2008; Carilli et al., 2010). While coral growth is controlled by several environmental factors such as seawater temperature, light, carbonate saturation state, wave energy and turbidity (Lough and Barnes, 2000; Cruz-Piñón et al., 2003; Fabricius, 2005; Marubini et al., 2008; Tanzil et al., 2009; Yan et al., 2011), drivers of recent observed declines in coral growth parameters require further investigation (Lough and Cantin, 2014). Reductions in coral growth rates of massive corals have been linked to bleaching events (Carilli et al., 2009a,b), SST increase (Cantin et al., 2010; Tanzil et al., 2013), attributed to ocean acidification (Crook et al., 2013; D’Olivo et al., 2013), and to the combination of warming and ocean acidification (Cooper et al., 2008; De’ath et al., 2009). Results are, however, inconsistent across broad spatial scales.
Several studies in the Caribbean have documented a wide variability among species and reef habitats in the response of coral growth rates to environmental conditions, thus complicating accurate region-wide predictions of future climatic impacts on coral reefs. Guzman et al. (2008) linked declines in growth rates of the coral Siderastrea siderea to runoff and sedimentation derived from the construction of the Panama Canal. Reefs subject to chronic anthropogenic stressors in the Mesoamerican Reef (MR) displayed suppressed growth rates of massive Orbicella faveolata for several years after the 1998 mass bleaching event (Carilli et al., 2009a). In contrast, for inshore O. faveolata colonies from the Florida Keys, growth rates recovered quickly (∼within 1 year) after thermal stress (Manzello et al., 2015). Also MR coral reefs experiencing thermal variability appear to enhance the tolerance of corals to thermal stress, as skeletal rates of Siderastrea siderea were not affected in back reef and nearshore colonies compared to colonies within more thermally stable fore reef environments, in which a significant decline in skeletal extension was found (Castillo et al., 2011, 2012). Evaluating calcification rates and SST trends from MR, Carricart-Ganivet et al. (2012) found Porites astreoides to be more sensitive to increasing temperature than O. faveolata and O. franksi. Furthermore, Crook et al. (2013) documented a significant decrease in calcification rates of P. astreoides concomitant with a natural gradient in pH and aragonite saturation (Ωarag) on the MR. Considering that declines in coral growth parameters seem to be primarily temperature-related rather than attributable to ocean acidification (Lough and Cantin, 2014), negative impacts on coral growth rates are excepted under future ocean warming. Yet, responses of growth parameters under the combined effects of ocean warming and acidification are not well understood for the Caribbean.
The modulating effect of climatic cycles such as the Atlantic Multi-decadal Oscillation (AMO) on coral records has also been identified as an important driver of coral growth rates in the Caribbean (Hetzinger et al., 2008). However, the identification of primary drivers of coral growth that are modulated by decadal climate variability requires more attention (Lough and Cantin, 2014). Evidence of multidecadal variability in annual coral growth rates has been documented for S. siderea in Belize and the Bahamas (Saenger et al., 2009), and Mexico (Vásquez-Bedoya et al., 2012); and for O. faveolata in Florida (Helmle et al., 2011). Furthermore, reanalyzing previously published coral growth records from Belize (O. faveolata) and Panama (S. siderea), Kwiatkowski et al. (2013) found that variations in growth rates between 1880 and 2000 displayed a decadal variability which was linked to regional climatic drivers (i.e., volcanic and anthropogenic aerosol emissions) rather than global drivers (i.e., climate change or ocean acidification). Thus, coral records from remote reefs, isolated from direct anthropogenic influence (e.g., coastal development and contamination, eutrophication, particulate organic matter, tourism) can be useful to clarify sources of decadal climate variability and support understanding of past and future climatic impacts on the Caribbean coral reefs.
Here, we provide the first decadal-scale record (1963–2015) of coral growth parameters from O. faveolata colonies that grew on patch reefs isolated from direct anthropogenic impacts at Serrana Atoll, Southwestern Caribbean. Growth parameters such as skeletal density, linear extension and calcification rates were evaluated to identify long-term trends and to relate growth responses with SST, AMO, The North Atlantic Oscillation (NAO) and Southern Oscillation (SOI) indexes, aragonite saturation states (Ωarag), and degree heating months (DHM). This analysis provides insight into how corals from an isolated Caribbean reef responded to environmental and climatic conditions over the last 50 years, and how they are likely to respond to a changing ocean.
Five cores from the massive coral Orbicella faveolata were collected on SCUBA at 4–9 m depth in lagoonal patch reefs of Serrana Atoll, inside the Seaflower Biosphere Reserve, Colombian Caribbean (Figure 1), during August 2016. Each coral core was extracted using an underwater pneumatic hand drill supplied with compressed air. Coral colonies were drilled along the maximum vertical growth axis (Helmle and Dodge, 2011). Cores were 6 cm in diameter and 0.6–0.9 m long (Table 1). A polystyrene ball was inserted and fixed with epoxy resin into the core hole to avoid colonization by boring organisms, diseases on exposed surfaces and promote coral tissue recovery.
Figure 1. Location of the Serrana Atoll in the Colombian Caribbean (dashed line). Sampling sites are indicated by white dots with the corresponding core code shown. Satellite image obtained and edited from the Landsat 8 OLI/TIRS C1 L-1 dataset (https://earthexplorer.usgs.gov/), taken on March 4, 2018.
The cores were rinsed with freshwater and then oven dried for 24 h at 50–60°C. A GE LightSpeed VCT 64 (General Electric Company) computerized tomography (CT) scanner was used to image the coral cores along with an aragonite step-wedge standard obtained from the shell of the giant clam Tridacna maxima (see Supplementary Information). The solid aragonite standard with known density was used to estimate coral density. The software RadiAnt DICOM Viewer 4.0 was used to view the DICOM files obtained from the CT scan and generate multi-planar reconstructions of all coral cores. Coronal and sagittal planes were examined to choose one image for each core, respectively. By using this method, the alternate pattern of horizontal density bands (high and low density) in the coral skeleton were visualized from different angles and slices. A density banding pattern is important to follow the vertical growth of massive corals and construct the coral chronology (see Supplementary Information). Skeletal density was calculated following the method by Carricart-Ganivet and Barnes (2007) for X-ray images, adapted to CT scan images (see Supplementary Information). Linear extension was measured between contiguous years or high-to-high density bands. Each coral year is formed by a pair of bands, corresponding to high and low density bands. Calcification rates were estimated as the product of skeletal density and linear extension rate. These three growth characteristics are sensitive to environmental changes and their variations are recorded in the coral skeleton (Barnes and Lough, 1996).
Annual growth values (i.e., density, extension and calcification) of each coral core were derived from the averaged measurements made in one transect, of both the coronal and sagittal planes, along the vertical growth axis of the coral colony. Master chronologies of skeletal density, linear extension and calcification were constructed averaging the annual values of the five cores, therefore the number of cores contributing to a specific year varied through the maser chronology (Table 1). To detect trends in growth parameters over time, linear regressions were applied to the master chronologies. Coefficients of variation (CV) were calculated for each growth parameter to demonstrate the inter-annual variability irrespective of differences in the means (Helmle et al., 2011). Annual density, extension and calcification rates were correlated to examine the relationship between coral growth parameters. Significance level for correlations was α = 0.05. In addition, master chronologies of the standardized anomalies (STDA) were obtained for each growth parameter. STDA consisted of the annual values minus the mean (over the period 1963–2015), divided by the standard deviation (Helmle et al., 2011). The five standardized chronologies were then averaged to create a single standardized record. As a result, the inherent variability of each core was accounted for, and a regional signal was detected (Jones et al., 2009; Grove et al., 2013).
To explore potential drivers of growth variability, annual means, minimum, and maximum of sea surface temperature (SST), DHM, Ωarag, AMO, Southern Oscillation Index (SOI) and NAO, were correlated with annual values of skeletal density, linear extension and calcification rates (Pearson’s correlations). Additionally, growth responses to thermal stress were assessed by plotting growth master chronologies and annual changes of growth parameters against DHM. Annual changes in growth parameters were calculated as the percentage of change relative to the 1963–2015 mean values. Finally, master chronologies of STDA were plotted against SST and AMO to comparatively evaluate long-term trends. A 13-year smoothing was applied to STDA and SST data to reduce inter-annual variability and facilitate the comparison of both time series.
SST was obtained from the 1° × 1° gridded HadISST1 dataset (Rayner et al., 2003) centered on 13.5°–14.5°N and 79.5°–80.5°W. The AMO was calculated from the Kaplan SST dataset (Enfield et al., 2001), which is an index of North Atlantic temperatures. The NAO and SOI indexes were constructed from normalized sea level pressure differences between the Azores–Iceland (Jones et al., 1997) and Darwin–Tahiti (Ropelewski and Jones, 1987), respectively. These indices are typically associated with climatic changes at large scales both in the Pacific and Atlantic oceans (i.e., ENSO events).
Aragonite saturation state (Ωarag) data were extracted from the NOAA Coral Reef Watch (CRW) Experimental Ocean Acidification Product Suite (OAPS) for the period 1988–2011 centered on 15°–16°N and 79°–81°W (Gledhill et al., 2008). The Ωarag data used here was 1°N adjacent to the area where Serrana Atoll is located.
DHM were calculated using the SST monthly data from HadISST on the same grid, in a similar manner to the degree heating weeks (DHW) used by NOAA Coral Reef Watch to predict coral bleaching. Thermal stress is considered to occur when temperatures surpass the bleaching threshold, which is established as 1°C above the mean of the climatological month with the highest temperature (Liu et al., 2006). However, for the HadISST dataset DHM were calculated as the annual sum of the difference between average monthly SSTs that exceeded the long-term maximum monthly mean (Carilli et al., 2010).
To further explore potential drivers of growth variability, a distance-based linear model (DISTLM) using a resemblance matrix of master chronologies values of density, calcification and extension (based on Euclidean distance), and forward selection, was adopted (Legendre and Anderson, 1999; Anderson et al., 2008). Forward selection adds one variable at a time to the model, selecting the variable that produces the greatest improvement in the value of adjusted r2 at each step. We used adjusted r2 as the selection criterion instead of r2 as we aimed to include only variables that significantly explained variation in the model. This method was applied to the time period 1988–2011, including as explanatory variables DHM, AMO, Ωarag, and NAO (data sets available for this period). DISTLM results provide a marginal test, fitting each variable individually (ignoring all other variables), and a sequential test, fitting each variable one at a time, restricted to the variables that were already included in the model (Anderson, 2003; Anderson et al., 2008). In addition, for the period 1963–2015, we applied the DISTML only to evaluate the individual contribution of each variable (marginal test) as our set of explanatory variables for this period were temperature-based (redundant variables). DISTLM analysis were performed in PERMANOVA+ package for PRIMER v6.
Over the period 1963–2015, the coral Orbicella faveolata of Serrana Atoll presented an overall mean ± SD skeletal density of 1.08 ± 0.08 g cm-3, linear extension rate of 0.96 ± 0.11 cm year-1 and calcification rate of 1.02 ± 0.12 g cm-2 year-1. Mean values for individual cores are shown in Table 1. The CV was 7% for density, 12% for extension rate and 12% for calcification rate. Extension rate was positively related to calcification rate (r = 0.82, p < 0.001, n = 53), calcification rate was positively related to density rate (r = 0.29, p = 0.04, n = 53) and density rate was negatively related to extension rate (r = -0.28, p = 0.04, n = 53). Skeletal density and calcification rate increased significantly over time (Figure 2A, r2 = 0.16, p = 0.004, n = 53; r2 = 0.10, p = 0.021, n = 53, respectively), whereas no significant change was observed in linear extension rate (r2 = 0.003, p = 0.698, n = 53).
Figure 2. Coral growth master chronologies during 1963–2015 for Serrana Atoll (black solid lines) (A). Trends (red solid lines) are significant for density (r2= 0.16, p = 0.004, n = 53) and calcification (r2= 0.10, p = 0.021, n = 53), but not for extension (r2 < 0.00, p = 0.698, n = 53). The thermal stress index given by degree heating months (DHM) are represented by gray bars (B). Dashed lines indicate the years (1969, 1998, 2005, 2010, and 2015) with the highest DHM values.
Mean ± SD of environmental conditions in Serrana Atoll were SST of 28.04 ± 0.31°C for the period 1963–2015, and Ωarag of 4.06 ± 0.09 for the period 1988–2011.
Skeletal density was negatively related to the mean SST, maximum SST, AMO, and DHM, based on coral growth master chronologies and environmental records (Table 2). In contrast, density was positively related to the NAO and Ωarag. Extension rate was not related to any of the environmental variables. However, there were significant negative correlations between calcification and maximum SST, AMO, and DHM. The 13-year smoothed anomalies of coral growth master chronologies tended to show opposite variation patterns to SST and AMO (Figure 3).
Table 2. Pearson correlations of coral growth parameters with environmental variables during 1963–2015 (n = 53), and 1988–2011 for Ωarag (n = 24).
Figure 3. Coral growth, SST and AMO correlations. Values of coral growth parameters (black solid lines) and SST (red solid lines) are presented as 13-year smoothed standardized anomalies (STDA). AMO is represented by red dotted lines. Density and calcification are negatively correlated with cyclical temperature trends.
Thermal stress in recent decades has been recurrent and intense (Figure 2B). Peaks of DHM in 1998, 2005, 2010 and 2015, coincident with El Niño events, had a negative effect on coral growth parameters. Reductions up to 25% (2005), 13% (2010), and 15% (2005) in calcification, extension and density, respectively, were detected (Figure 4). However, high DHM values observed before 1997, as in 1969, showed no negative effects on coral growth. During the period 1963–1997, thermal stress events were sporadic, and did not explained important reductions in growth parameters (e.g., 1964, 1976 and 1977).
Figure 4. Percentage of inter-annual variations (relative to historical mean) of growth parameters and their relationship with the annual values of degree heating months (DHM).
DISTLM analysis for the period 1963–2015 did not identify a predominant predictor explaining most of the variation in the master chronologies (see marginal test, Table 3). For the period 1988–2011, the variation in coral density was mainly explain by Ωarag, which accounted for 37% of the total variation (see marginal and sequential tests, Tables 4, 5).
Table 4. Results of the marginal test for the period 1988–2011 performed by DISTLM forward analysis.
Located far away from direct anthropogenic disturbances, corals from Serrana Atoll allow a clear identification of environmental and climatic signatures on growth rates. Here we present a 53-year record of coral growth parameters for O. faveolata for remote Southwestern Caribbean reefs. To the best of our knowledge, this is the first record of its kind for this region of the Caribbean, complementing the geographic coverage of previous studies and providing new insights into the understanding of coral growth responses in a period characterized by human-induced environmental and climatic changes (1963–2015).
Overall, coral growth records from Serrana Atoll indicate that calcification processes for O. faveolata have suffered disparate changes over the last 50 years, mainly associated to thermal stress, and occurring more frequently during recent decades. While the values of growth parameters of O. faveolata are within the ranges found for other Caribbean reefs (Dodge and Brass, 1984; van Veghel and Bosscher, 1995; Gischler and Oschmann, 2005; Helmle et al., 2011; Manzello et al., 2015), the CVs (7–12%) observed suggest important inter-annual variations in growth responses to environmental conditions. Indeed, between 1963 and 1990 growth parameters were characterized by positive anomalies. However, in the last 20 years negative anomalies have dominated the variability of growth parameters (Figure 3). Although values of CV were low in comparison to those reported by Helmle et al. (2011) for O. faveolata in Florida, probably reflecting latitudinal differences as well as lesser variability in environmental conditions in Serrana Atoll during the last decades, it is highly likely that thermal stress caused significant inter-annual variations over the studied period.
Degree heating months showed a negative effect on calcification rates during 1998, 2005 and 2010, coinciding with severe coral bleaching events in the region (Hughes et al., 2017). Signatures of bleaching on coral growth parameters include an increase in skeletal density and a marked reduction in extension and calcification rates (Carilli et al., 2009a). Corals growing in clear and oligotrophic waters are highly vulnerable to changes in temperature and thermal stress, which can result in mass coral bleaching (Hughes et al., 2017), and significant decreases in their growth parameters (Carilli et al., 2009a,b). Whilst we cannot relate every single decline in growth parameters to thermal stress, we highlight that bleaching-induced impacts on growth parameters were unprecedented before 1997, and more frequent in recent years, corresponding with bleaching events linked to global warming (Hughes et al., 2018). Nevertheless, the magnitude of declines in Serrana Atoll were relatively small when compared to those in Belize during the 1998 bleaching, where O. faveolata corals showed pronounced decreases in extension and calcification rates (over four standard deviations outside the mean more chronology) and even interruption of growth (Carilli et al., 2009a). This outcome suggests that O. faveolata corals at Serrana Atoll, isolated from direct chronic human impacts (e.g., pollution, costal development, tourism), seem to have responded better to recent bleaching events than Belizean counterparts. This observation supports the hypothesis that corals with higher local anthropogenic stressors present reduced coral resistance to bleaching or thermal tolerance (Carilli et al., 2009a, 2010).
The significant relationships between growth parameters provide insights into historical growth strategies of O. faveolata at Serrana Atoll. We found a strong positive relationship between extension and calcification, and weaker relationships between extension and density (negative), and density and calcification (positive). These correlations are usually used to infer how corals invest increased availability of resources for calcification in extension or density (Carricart-Ganivet, 2007). While previous studies report that skeletal density tends to be the primary driver of variations in calcification for O. faveolata (Carricart-Ganivet, 2004), here extension was the key driver. This indicates that the growth strategy of the corals is favoring extension rather than the skeletal density at Serrana Atoll, as the extension did not showed a declining trend, but instead was strong related with the skeletal calcification rate. We argue that corals at Serrana Atoll are investing their calcification resources into maintaining extension as a response to a reduction of Ωarag, attributed to ocean acidification in recent years (see below for details), and long-term increases in SST. This growth response seems to correspond to the “stretching” modulation phenomenon (in a temporal context) postulated by Carricart-Ganivet and Merino (2001), in which corals respond to environmental stress by extending their skeletons and maintaining calcification. This was clearly observed during the last decade (Figure 3), where despite the decline in density, both extension and calcification did not decline and remained relatively unchanged.
The most significant responses detected in growth parameters were the long-term decreases in skeletal density and calcification rates, and the absence of a temporal trend in extension rates. The downward trend in density was mainly linked to a decline in Ωarag (Table 2 and Figure 5) and, to a lesser extent, to warmer conditions in recent decades, which in turn could affect calcification responses. For the studied period (1963–2015) moderate to weak relationships were observed between density and the environmental variables considered (mainly SST related). The DISTML analysis indicated that temperature-based explanatory variables explained a modest part of the observed variability in density (<19%), suggesting that there are additional factors implicated in the declining trend. For the period 1998–2011, the DISTLM analysis identified that Ωarag explained most of the variability observed in density. Thus, it is reasonable to suppose that long-term declining trends in density and calcification were modulated by a decrease in Ωarag, the latter attributed to ocean acidification (Gledhill et al., 2008). Other studies have found that ocean acidification has a direct effect on skeletal density but not on linear extension rates (Tambutté et al., 2015; Mollica et al., 2018) as observed in our coral cores. Similarly to Kleypas (1999) and Helmle et al. (2011), we argue that Ωarag has not yet reached a critical threshold needed to negatively impact linear extension. Nevertheless, ocean acidification is an increasing threat for corals as ongoing reduction in carbonate ion concentration will lead to less dense and more fragile coral skeletons (Fantazzini et al., 2015; Tambutté et al., 2015; Mollica et al., 2018), compromising reef ecosystem function within decades (Kleypas and Yates, 2009; Albright et al., 2018). Some models predict an average 12.4% decline in skeletal density for Porites corals globally by the end of the century due to ocean acidification (Mollica et al., 2018). In light of the decreasing trends documented here and global predictions of decreasing coral density, we speculate that ocean acidification will play a major role in coral calcification at Serrana Atoll (and elsewhere within the coralline complexes from the Seaflower Reserve) in the near future.
Figure 5. Aragonite saturation state (Ωarag) in the vicinity of Serrana Atoll, during 1988–2011 (solid line). The dashed line indicates a significant tendency (r2 = 0.94, p < 0.001) described by the equation y = 28.815 – 0.012x.
Our study provides further evidence concerning the multi-decadal variability in coral growth rates in the Caribbean (Hetzinger et al., 2008; Helmle et al., 2011). Particularly we found a significant negative correlation of the skeletal density and calcification with AMO, and no relationship between extension and AMO. The majority of studies only report the relationship between AMO and extension, identifying a positive correlation for locations such as Belize and Panama (Kwiatkowski et al., 2013), and negative correlations in Mexico (Vásquez-Bedoya et al., 2012), Bahamas and Belize (Saenger et al., 2009), and Florida (Helmle et al., 2011). In Florida, Helmle et al. (2011) found a positive relationship between density and AMO, and no relationship with calcification, contrary to our finding. These varying outcomes do not diminish the importance of multi-decadal variability in coral growth rates in the Caribbean but underline the need to improve our understanding of the significant role of the AMO in influencing coral growth rates in the region. As previous AMO analyses were based on coral records up to only 2000 (Helmle et al., 2011; Kwiatkowski et al., 2013), just after the AMO shifted from a cold to a warm phase (Zhang and Delworth, 2006), our master chronologies add crucial data to infer coral growth responses in such warm AMO phases. In our study area, long-term trends of skeletal density and calcification varied inversely to AMO, with positive anomalies during the cold phase of the AMO (1965–1995), and negative anomalies during the last warm phase after 1995 (Zhang and Delworth, 2006).
The master chronologies analyzed provide key details regarding the latest trends of coral growth parameters that can support predictions of future trajectories of coral growth and calcification processes for the Southwestern Caribbean. Since 2010, calcification and extension rates have shown baseline averages, but density values remain below the long-term average (negative anomalies). We attribute this to ocean acidification given that Ωarag apparently contributed to the declining trend observed for density. Thus, although long-term calcification processes at Serrana Atoll have been modulated by SST oscillations at decadal scales (AMO), it appears that in the most recent phase of the AMO decreasing Ωarag dominated the effect of SST. The ocean acidification and temperature oscillations controlled by AMO are therefore important to understand how coral growth parameters may respond to those oscillations in the coming decades. Climatic predictions indicate the oceans will continue to acidify, negatively affecting coral reefs by reducing carbonate ion concentrations which result in corals with more fragile skeletons and more susceptible to bioerosion (Kleypas, 1999; van Woesik et al., 2013; Mollica et al., 2018). Temperature is another key variable that is expected to continue increasing and threatening corals (Meissner et al., 2012). However, temperature stress on Caribbean reefs might be less in coming decades than in recent years (1990–2015). According to historical ocean temperature oscillations it has been suggested that in the following decades these oscillations will shift from a warm phase (the present) to a cold phase (Klöwer et al., 2014; McCarthy et al., 2015; Frajka-Williams et al., 2017; Page, 2017). In spite of this, models indicate that most coral reefs will suffer marked deterioration over the next few decades, even reducing global warming to 2°C and under the optimistic assumption of corals undergoing thermal adaptation (Frieler et al., 2012). Calcification might be oscillating in response to the AMO, however, the decline in Ωarag will be critical for coral growth. Consequently, the prediction of future responses of Caribbean reefs has to consider the combined role of warming, AMO variability and ocean acidification on coral growth and calcification processes.
LL-S processed and analyzed the cores, performed statistical analyses, and contributed to manuscript writing. ÁM-G contributed to core processing and analysis. ML-V collected the samples, processed and analyzed the cores, and contributed to manuscript writing. AR-R analyzed the cores, performed statistical analyses, contributed to manuscript writing, and directed the work. All authors participated in study conception and design.
This work was funded by the Pontificia Universidad Javeriana Cali. The field trip was conducted during the Seaflower Scientific Expedition-Serrana 2016, supported by the Colombian Government, under the leadership of the Colombian Oceans’ Commission (CCO), and with logistic support from the Colombian Navy. Additional funding was provided by the Administrative Department of Science, Technology and Innovation (Colciencias), under the Colombia-BIO program (Agreement 341–2016, between Universidad de los Andes and Comisión Colombiana del Océano).
The authors declare that the research was conducted in the absence of any commercial or financial relationships that could be construed as a potential conflict of interest.
We thank the Colombian authorities leading the Scientific Expeditions to the Seaflower Biosphere Marine Reserve, in particular the Colombian Oceans’ Commission (CCO), the Colombian Navy, and the Corporation for the Sustainable Development of the Archipelago of San Andrés, Providencia and Santa Catalina (Coralina). Of great importance was the logistic support during the field trip provided by the crew members from Coralina, and by Juliana Sintura. The Hospital Universitario del Valle (HUV) and its personnel kindly helped us with CT-scan images acquisition. We thank Adam Suchley for his comments on a previous version of this manuscript. This research was performed under the framework of a collaborative research between the Global Change Institute, the University of Queensland, and the Department of Natural Sciences and Mathematics, from the Pontificia Universidad Javeriana Cali.
The Supplementary Material for this article can be found online at: https://www.frontiersin.org/articles/10.3389/fmars.2019.00038/full#supplementary-material
Albright, R., Takeshita, Y., Koweek, D. A., Ninokawa, A., Wolfe, K., Rivlin, T., et al. (2018). Carbon dioxide addition to coral reef waters suppresses net community calcification. Nature 555, 516–519. doi: 10.1038/nature25968
Anderson, M., Gorley, R. N., and Clarke, K. (2008). PERMANOVA+ for Primer: Guide to Software and Statistical Methods. Plymouth: PRIMER-E Ltd.
Anderson, M. J. (2003). Distlm Forward: A Fortran Computer Program to Calculate a Distance-Based Multivariate Analysis for a Linear Model using Forward Selection. Auckland: Department of Statistics, University of Auckland.
Barnes, D. J., and Lough, J. M. (1996). Coral skeletons: storage and recovery of environmental information. Glob. Chang. Biol. 2, 569–582. doi: 10.1111/j.1365-2486.1996.tb00068.x
Bessat, F., and Buigues, D. (2001). Two centuries of variation in coral growth in a massive Porites colony from moorea (French Polynesia): a response of ocean-atmosphere variability from south central Pacific. Palaeogeogr. Palaeoclimatol. Palaeoecol. 175, 381–392. doi: 10.1016/S0031-0182(01)00381-9
Cantin, N. E., Cohen, A. L., Karnauskas, K. B., Tarrant, A. M., and McCorkle, D. C. (2010). Ocean warming slows coral growth in the central red sea. Science 329, 322–325. doi: 10.5061/dryad.9g182
Carilli, J. E., Norris, R. D., Black, B., Walsh, S. M., and Mcfield, M. (2010). Century-scale records of coral growth rates indicate that local stressors reduce coral thermal tolerance threshold. Glob. Chang. Biol. 16, 1247–1257. doi: 10.1111/j.1365-2486.2009.02043.x
Carilli, J. E., Norris, R. D., Black, B. A., Walsh, S. M., and McField, M. (2009a). Local stressors reduce coral resilience to bleaching. PLoS One 4:e6324. doi: 10.1371/journal.pone.0006324
Carilli, J. E., Prouty, N. G., Hughen, K. A., and Norris, R. D. (2009b). Century-scale records of land-based activities recorded in mesoamerican coral cores. Mar. Pollut. Bull. 58, 1835–1842. doi: 10.1016/j.marpolbul.2009.07.024
Carricart-Ganivet, J. P. (2004). Sea surface temperature and the growth of the west atlantic reef-building coral Montastraea annularis. J. Exp. Mar. Bio. Ecol. 302, 249–260. doi: 10.1016/j.jembe.2003.10.015
Carricart-Ganivet, J. P. (2007). Annual density banding in massive coral skeletons: result of growth strategies to inhabit reefs with high microborers’ activity? Mar. Biol. 153, 1–5. doi: 10.1007/s00227-007-0780-3
Carricart-Ganivet, J. P., and Barnes, D. J. (2007). Densitometry from digitized images of X-radiographs: methodology for measurement of coral skeletal density. J. Exp. Mar. Bio. Ecol. 344, 67–72. doi: 10.1016/j.jembe.2006.12.018
Carricart-Ganivet, J. P., Cabanillas-Terán, N., Cruz-Ortega, I., and Blanchon, P. (2012). Sensitivity of calcification to thermal stress varies among genera of massive reef-building corals. PLoS One 7:e32859. doi: 10.1371/journal.pone.0032859
Carricart-Ganivet, J. P., and Merino, M. (2001). Growth responses of the reef-building coral Montastraea annularis along a gradient of continental influence in the southern gulf of Mexico. Bull. Mar. Sci. 68, 133–146.
Castillo, K. D., Ries, J. B., and Weiss, J. M. (2011). Declining coral skeletal extension for forereef colonies of Siderastrea siderea on the mesoamerican barrier reef system, southern belize. PLoS One 6:e14615. doi: 10.1371/journal.pone.0014615
Castillo, K. D., Ries, J. B., Weiss, J. M., and Lima, F. P. (2012). Decline of forereef corals in response to recent warming linked to history of thermal exposure. Nat. Clim. Chang. 2, 756–760. doi: 10.1038/nclimate1577
Cooper, T. F., De’ath, G., Fabricius, K. E., and Lough, J. M. (2008). Declining coral calcification in massive Porites in two nearshore regions of the northern great barrier reef. Glob. Chang. Biol. 14, 529–538. doi: 10.1111/j.1365-2486.2007.01520.x
Crook, E. D., Cohen, A. L., Rebolledo-Vieyra, M., Hernandez, L., and Paytan, A. (2013). Reduced calcification and lack of acclimatization by coral colonies growing in areas of persistent natural acidification. Proc. Natl. Acad. Sci. U.S.A. 110, 11044–11049. doi: 10.1073/pnas.1301589110
Cruz-Piñón, G., Carricart-Ganivet, J. P., and Espinoza-Avalos, J. (2003). Monthly skeletal extension rates of the hermatypic corals Montastraea annularis and Montastraea faveolata: biological and environmental controls. Mar. Biol. 143, 491–500. doi: 10.1007/s00227-003-1127-3
De’ath, G., Lough, J. M., and Fabricius, K. E. (2009). Declining coral calcification on the great barrier reef. Science 323, 116–119. doi: 10.1126/science.1165283
Dodge, R. E., and Brass, G. W. (1984). Skeletal extension, density and calcification of the reef coral, Montastrea annularis: St. Croix, U.S. Virgin Islands. Bull. Mar. Sci. 34, 288–307.
D’Olivo, J. P., McCulloch, M. T., and Judd, K. (2013). Long-term records of coral calcification across the central great barrier reef: assessing the impacts of river runoff and climate change. Coral Reefs 32, 999–1012. doi: 10.1007/s00338-013-1071-8
Enfield, D. B., Mestas-NuÑez, A. M., and Trimble, P. J. (2001). The Atlantic multidecadal oscillation and its relationship to rainfall and river flows in the continental U.S.A. Geophys. Res. Lett. 28, 2077–2080. doi: 10.1029/2000GL012745
Fabricius, K. E. (2005). Effects of terrestrial runoff on the ecology of corals and coral reefs: review and synthesis. Mar. Pollut. Bull. 50, 125–146. doi: 10.1016/j.marpolbul.2004.11.028
Fantazzini, P., Mengoli, S., Pasquini, L., Bortolotti, V., Brizi, L., Mariani, M., et al. (2015). Gains and losses of coral skeletal porosity changes with ocean acidification acclimation. Nat. Commun. 6:7785. doi: 10.1038/ncomms8785
Frajka-Williams, E., Beaulieu, C., and Duchez, A. (2017). Emerging negative atlantic multidecadal oscillation index in spite of warm subtropics. Sci. Rep. 7:11224. doi: 10.1038/s41598-017-11046-x
Frieler, K., Meinshausen, M., Golly, A., Mengel, M., Lebek, K., Donner, S. D., et al. (2012). Limiting global warming to 2°C is unlikely to save most coral reefs. Nat. Clim. Chang. 3, 165–170. doi: 10.1038/nclimate1674
Gischler, E., and Oschmann, W. (2005). Historical climate variation in Belize (Central America) as recorded in scleractinian coral skeletons. Palaios 20, 159–174. doi: 10.2210/palo.2004.p04-09
Gledhill, D. K., Wanninkhof, R., Millero, F. K., and Eakin, M. (2008). Ocean acidification of the greater caribbean region 1996-2006. J. Geophys. Res. 113, C10031. doi: 10.1029/2007JC004629
Grove, C. A., Zinke, J., Peeters, F., Park, W., Scheufen, T., Kasper, S., et al. (2013). Madagascar corals reveal a multidecadal signature of rainfall and river runoff since 1708. Clim. Past 9, 641–656. doi: 10.5194/cp-9-641-2013
Guzman, H. M., Cipriani, R., and Jackson, J. B. C. (2008). Historical decline in coral reef growth after the panama canal. Ambio 37, 342–346. doi: 10.1579/07-A-372.1
Helmle, K. P., and Dodge, R. E. (2011). “Sclerochronology,” in Encyclopedia of Modern Coral Reefs, ed. D. Hopley (Berlin: Springer), 958–966. doi: 10.1007/978-90-481-2639-2_22
Helmle, K. P., Dodge, R. E., Swart, P. K., Gledhill, D. K., and Eakin, C. M. (2011). Growth rates of florida corals from 1937 to 1996 and their response to climate change. Nat. Commun. 2:215. doi: 10.1038/ncomms1222
Hetzinger, S., Pfeiffer, M., Dullo, W. C., Keenlyside, N., Latif, M., and Zinke, J. (2008). Caribbean coral tracks atlantic multidecadal oscillation and past hurricane activity. Geology 36, 11–14. doi: 10.1130/G24321a.1
Hughes, T. P., Anderson, K. D., Connolly, S. R., Heron, S. F., Kerry, J. T., Lough, J. M., et al. (2018). Spatial and temporal patterns of mass bleaching of corals in the Anthropocene. Science 359, 80–83. doi: 10.1126/science.aan8048
Hughes, T. P., Kerry, J. T., Álvarez-Noriega, M., Álvarez-Romero, J. G., Anderson, K. D., Baird, A. H., et al. (2017). Global warming and recurrent mass bleaching of corals. Nature 543, 373–377. doi: 10.1038/nature21707
Jones, P. D., Briffa, K. R., Osborn, T. J., Lough, J. M., van Ommen, T. D., Vinther, B. M., et al. (2009). High-resolution palaeoclimatology of the last millennium: a review of current status and future prospects. Holocene 19, 3–49. doi: 10.1177/0959683608098952
Jones, P. D., Jonsson, T., and Wheeler, D. (1997). Extension to the north atlantic oscillation using early instrumental pressure observations from gibraltar and south-west Iceland. Int. J. Climatol. 17, 1433–1450. doi: 10.1002/(SICI)1097-0088(19971115)17:13<1433::AID-JOC203>3.0.CO;2-P
Kleypas, J. A. (1999). Geochemical consequences of increased atmospheric carbon dioxide on coral reefs. Science 284, 118–120. doi: 10.1126/science.284.5411.118
Kleypas, J. A., and Yates, K. K. (2009). Coral reefs and ocean acidification. Oceanography 22, 108–117. doi: 10.5670/oceanog.2009.101
Klöwer, M., Latif, M., Ding, H., Greatbatch, R. J., and Park, W. (2014). Atlantic meridional overturning circulation and the prediction of north atlantic sea surface temperature. Earth Planet. Sci. Lett. 406, 1–6. doi: 10.1016/j.epsl.2014.09.001
Kwiatkowski, L., Cox, P. M., Economou, T., Halloran, P. R., Mumby, P. J., Booth, B. B. B., et al. (2013). Caribbean coral growth influenced by anthropogenic aerosol emissions. Nat. Geosci. 6, 362–366. doi: 10.1038/ngeo1780
Legendre, P., and Anderson, M. J. (1999). Distance-based redundancy analysis: testing multispecies responses in multifactorial ecological experiments. Ecol. Monogr. 69, 1–24. doi: 10.1890/0012-9615(1999)069[0001:DBRATM]2.0.CO;2
Liu, G., Strong, A. E., Skirving, W. J., and Arzayus, L. F. (2006). “Overview of NOAA coral reef watch program’s near-real-time satellite global coral bleaching monitoring activities,” in Proceedings of the 10th International Coral Reef Symposium, (Okinawa), 1783–1793.
Lough, J. M. (2008). Coral calcification from skeletal records revisited. Mar. Ecol. Prog. Ser. 373, 257–264. doi: 10.3354/meps07398
Lough, J. M., and Barnes, D. J. (1992). Comparisons of skeletal density variations in Porites from the central great barrier reef. J. Exp. Mar. Bio. Ecol. 155, 1–25. doi: 10.1016/0022-0981(92)90024-5
Lough, J. M., and Barnes, D. J. (2000). Environmental controls on growth of the massive coral Porites. J. Exp. Mar. Bio. Ecol. 245, 225–243. doi: 10.1016/S0022-0981(99)00168-9
Lough, J. M., and Cantin, N. E. (2014). Perspectives on massive coral growth rates in a changing ocean. Biol. Bull. 226, 187–202. doi: 10.1086/BBLv226n3p187
Lough, J. M., and Cooper, T. F. (2011). New insights from coral growth band studies in an era of rapid environmental change. Earth Sci. Rev. 108, 170–184. doi: 10.1016/j.earscirev.2011.07.001
Manzello, D. P., Enochs, I. C., Kolodziej, G., and Carlton, R. (2015). Recent decade of growth and calcification of Orbicella faveolata in the florida keys: an inshore-offshore comparison. Mar. Ecol. Prog. Ser. 521, 81–89. doi: 10.3354/meps11085
Marubini, F., Ferrier-Pages, C., Furla, P., and Allemand, D. (2008). Coral calcification responds to seawater acidification: a working hypothesis towards a physiological mechanism. Coral Reefs 27, 491–499. doi: 10.1007/s00338-008-0375-6
McCarthy, G. D., Haigh, I. D., Hirschi, J., Grist, J. P., and Smeed, D. A. (2015). Ocean impact on decadal atlantic climate variability revealed by sea-level observations. Nature 521, 508–512. doi: 10.1038/nature14491
Meissner, K. J., Lippmann, T., and Sen Gupta, A. (2012). Large-scale stress factors affecting coral reefs: open ocean sea surface temperature and surface seawater aragonite saturation over the next 400 years. Coral Reefs 31, 309–319. doi: 10.1007/s00338-011-0866-8
Mollica, N. R., Guo, W., Cohen, A. L., Huang, K.-F., Foster, G. L., Donald, H. K., et al. (2018). Ocean acidification affects coral growth by reducing skeletal density. Proc. Natl. Acad. Sci. U.S.A. 115, 1754–1759. doi: 10.1073/pnas.1712806115
Page, N. J. (2017). The coming cooling: usefully accurate climate forecasting for policy makers. Energy Environ. 28, 330–347. doi: 10.1177/0958305X16686488
Pandolfi, J. M. (2011). “The Paleoecology of Coral Reefs,” in Coral Reefs: An Ecosystem in Transition, eds Z. Dubinsky and N. Stambler (Berlin: Springer), 13–24. doi: 10.1007/978-94-007-0114-4_2
Rayner, N. A., Parker, D. E., Horton, E. B., Folland, C. K., Alexander, L. V., and Rowell, D. P. (2003). Global analyses of sea surface temperature, sea ice, and night marine air temperature since the late nineteenth century. J. Geophys. Res. 108:4407. doi: 10.1029/2002JD002670
Ropelewski, C. F., and Jones, P. D. (1987). An extension of the tahiti–darwin southern oscillation index. Mon. Weather Rev. 115, 2161–2165. doi: 10.1175/1520-0493(1987)115<2161:AEOTTS>2.0.CO;2
Saenger, C., Cohen, A. L., Oppo, D. W., Halley, R. B., and Carilli, J. E. (2009). Surface-temperature trends and variability in the low-latitude north atlantic since 1552. Nat. Geosci. 2, 492–495. doi: 10.1038/ngeo552
Tambutté, E., Venn, A. A., Holcomb, M., Segonds, N., Techer, N., Zoccola, D., et al. (2015). Morphological plasticity of the coral skeleton under CO2-driven seawater acidification. Nat. Commun. 6:7368. doi: 10.1038/ncomms8368
Tanzil, J., Brown, B., Tudhope, A., and Dunne, R. (2009). Decline in skeletal growth of the coral Porites lutea from the andaman sea, south thailand between 1984 and 2005. Coral Reefs 28, 519–528. doi: 10.1007/s00338-008-0457-5
Tanzil, J. T., Brown, B. E., Dunne, R. P., Lee, J. N., Kaandorp, J. A., and Todd, P. A. (2013). Regional decline in growth rates of massive Porites corals in Southeast Asia. Glob. Chang. Biol. 19, 3011–3023. doi: 10.1111/gcb.12279
van Veghel, M. L., and Bosscher, H. (1995). Variation in linear growth and skeletal density within the polymorphic reef building coral Montastraea annularis. Bull. Mar. Sci. 56, 902–908.
van Woesik, R., van Woesik, K., and van Woesik, L. (2013). Effects of ocean acidification on the dissolution rates of reef-coral skeletons. PeerJ 1, e208. doi: 10.7717/peerj.208
Vásquez-Bedoya, L. F., Cohen, A. L., Oppo, D. W., and Blanchon, P. (2012). Corals record persistent multidecadal SST variability in the Atlantic Warm Pool since 1775 AD. Paleoceanography 27, A3231. doi: 10.1029/2012PA002313
Yan, H., Sun, L., Wang, Y., Huang, W., Qiu, S., and Yang, C. (2011). A record of the southern oscillation index for the past 2,000 years from precipitation proxies. Nat. Geosci. 4, 611–614. doi: 10.1038/ngeo1231
Keywords: coral growth parameters, Atlantic Multi-decadal Oscillation, ocean acidification, coral reefs, Orbicella faveolata, Serrana Atoll
Citation: Lizcano-Sandoval LD, Marulanda-Gómez Á, López-Victoria M and Rodriguez-Ramirez A (2019) Climate Change and Atlantic Multidecadal Oscillation as Drivers of Recent Declines in Coral Growth Rates in the Southwestern Caribbean. Front. Mar. Sci. 6:38. doi: 10.3389/fmars.2019.00038
Received: 19 September 2018; Accepted: 23 January 2019;
Published: 08 February 2019.
Edited by:
Sonia Bejarano, Leibniz Centre for Tropical Marine Research (LG), GermanyReviewed by:
Chiara Lombardi, Italian National Agency for New Technologies, Energy and Sustainable Economic Development (ENEA), ItalyCopyright © 2019 Lizcano-Sandoval, Marulanda-Gómez, López-Victoria and Rodriguez-Ramirez. This is an open-access article distributed under the terms of the Creative Commons Attribution License (CC BY). The use, distribution or reproduction in other forums is permitted, provided the original author(s) and the copyright owner(s) are credited and that the original publication in this journal is cited, in accordance with accepted academic practice. No use, distribution or reproduction is permitted which does not comply with these terms.
*Correspondence: Mateo López-Victoria, bWFsb3ZAamF2ZXJpYW5hY2FsaS5lZHUuY28=; bWFsb3ZAcHVqLmVkdS5jbw==
†Present address: Luis D. Lizcano-Sandoval, College of Marine Science, University of South Florida, St. Petersburg, FL, United States; Ángela Marulanda-Gómez Department of Freshwater and Marine Ecology, Institute of Biodiversity and Ecosystem Dynamics (IBED), The University of Amsterdam, Amsterdam, Netherlands; Alberto Rodriguez-Ramirez, Marine Palaeoecology Lab, School of Biological Sciences, The University of Queensland, St. Lucia, QLD, Australia
Disclaimer: All claims expressed in this article are solely those of the authors and do not necessarily represent those of their affiliated organizations, or those of the publisher, the editors and the reviewers. Any product that may be evaluated in this article or claim that may be made by its manufacturer is not guaranteed or endorsed by the publisher.
Research integrity at Frontiers
Learn more about the work of our research integrity team to safeguard the quality of each article we publish.