- 1Graduate Program in Oceanography, Department of Oceanography, University of Concepción, Concepción, Chile
- 2Instituto Milenio de Oceanografía, University of Concepción, Concepción, Chile
- 3Departamento de Oceanografía, Facultad de Ciencias Naturales y Oceanográficas, Universidad de Concepción, Concepción, Chile
- 4Instituto Español de Oceanografía, Centro Oceanográfico de A Coruña, A Coruña, Spain
Biochemical conditions and taxonomic composition of size-fractioned mesozoo-plankton were studied after a cruise conducted in September 2015 between the Chilean coast (70°W) and Easter Island (110°W) within the central south Pacific gyre. Taxonomy was assessed with an automated method based on image analysis and biochemical conditions assessed by analyses of C and N contents and stable isotope composition. Based on surface Chlorophyll-a levels, four regions were distinguished across the zonal gradient: eutrophic (Chilean upwelling zone), mesotrophic (Coastal Transition Zone), oligotrophic (open ocean water) and ultraoligotrophic (central south Pacific gyre). The zones had marked differences in temperature, oxygen, salinity and Chlorophyll-a, and they also exhibited significant differences in zooplankton composition, C/N ratios and δ13C and δ15N for all size fractions of zooplankton. Variability in the sources of C and N, linked to biogeochemical processes, such as new production and denitrification in the upwelling zone, potential diazotrophy, highly regenerated C and N and extreme oligotrophy (N-deficiency) in oceanic areas, are suggested as the key drivers of these differences. Our findings also suggest a strong coupling between taxonomic and size zooplankton-diversity and the sources of nutrients that fuel phytoplankton, the major food source for zooplankton. Although multiple factors and processes can modulate C and N and their isotopes composition of zooplankton biomass, our study shows that changes in community structure are linked to different biogeochemical regions across the zonal gradient, providing the basis for ecological zonation associated with nutrient utilization at lower trophic levels.
Introduction
The eastern South Pacific region is characterized by a strong coastal-offshore oceanographic gradient from the highly productive (eutrophic) coastal upwelling zone toward the oligotrophic and even ultraoligotrophic region in the central south Pacific gyre (Raimbault and Garcia, 2008; Von Dassow and Collado-Fabbri, 2014; Moutin et al., 2017).
A substantial amount of research has been conducted on the functioning and structure of the eastern boundary upwelling system (EBUS) off Chile (e.g., Cury et al., 2000; Daneri et al., 2000; Hormazabal et al., 2001), including the mesotrophic coastal transition zone (CTZ) (Morales et al., 1999, 2010). However, the vast oceanic oligotrophic region remains largely unknown (Von Dassow and Collado-Fabbri, 2014). A few expeditions have reported the plankton composition in the region (Fagetti and Fisher, 1964; Hernández et al., 2005; Palma and Silva, 2006), although there is a lack of knowledge on how these pelagic communities can be sustained in terms of their sources of organic C and N, considering the very low levels of primary production (Raimbault and Garcia, 2008).
From the highly productive Chilean coastal zone to the oceanic Easter Island (∼4,500 km from shore), and over this oceanographic gradient, the plankton community is subject to gradual, and sometimes abrupt changes in temperature, oxygenation, salinity, pH, food quality and quantity, and possibly to a variety of ecological and biogeochemical processes, such as primary production, diazotrophy, denitrification among others, altogether modulating their sources of nutrients supporting the production of organic C and N (Raimbault and Garcia, 2008; Fernandez et al., 2011; Farías et al., 2013). However, in spite of the strong variability in oceanographic conditions observed over the zonal gradient of the eastern South Pacific, only two biogeochemical provinces have been suggested for the whole area which are characterized by globally unique and distinguishable biogeochemical processes, corresponding to the provinces of the Humboldt current coast and South Pacific gyre (Oliver and Irwin, 2008; Longhurst, 2010; Reygondeau et al., 2013). Within these provinces it is then possible to distinguish ecoregions, being defined on the basis of species composition and ecological dynamics. These features within ecoregions are relatively homogeneous, and stable with respect to the adjacent regions. In the eastern South Pacific the suggested ecoregions are Central Peru, Humboldtian, Central Chile, Araucanian, Juan Fernández and Desventuradas (Spalding et al., 2007). Nevertheless, the ecological and biogeochemical partitioning of the entire zonal gradient is unclear from the point of view of the plankton community. For instance, the interaction between the physical/biogeochemical gradient and the zooplankton community structure is unknown for this large region covering the area from the coastal upwelling zone up to the central Pacific gyre. Understanding such interaction can provide insights on the ecological and evolutionary processes controlling diversity and adaptability of plankton to a changing ocean, both in space and time.
Zooplankton plays an important role in the pelagic zone because they are a key element in the transfer of organic matter from primary producers to higher levels (Saiz et al., 2007). In this respect, the study of the stable isotopes composition of C and N in zooplankton can provide highly valuable information to determine the sources of C and N being transferred through the food web (Peterson and Fry, 1987). Furthermore, isotopes are widely used as markers for depicting the food web and trophic position of organisms, due to the enrichment of heavy isotopes in the tissue of predators with respect to their prey, since the light isotopes are mobilized with greater speed in chemical reactions (Post, 2002; El-Sabaawi et al., 2013; Wang et al., 2014). An important advantage of these analyses is that they can also provide a quantitative characterization of the trophic niche of species, communities or ecosystems (Layman et al., 2007, 2012).
In this study, we assessed the mesozooplankton community structure (size and taxonomic composition) along the coastal-offshore gradient, their size-fractionated C and N contents, and corresponding (δ13C and δ15N) in relation with the zonal variability in temperature, salinity, oxygen and Chlorophyll-a. We aimed at elucidating how changing oceanographic conditions across the coastal-offshore gradient and different biogeochemistry processes determining the sources of C and N affecting the food resource of zooplankton, can influence their biochemical conditions and community structure.
Materials and Methods
Oceanographic Survey
The CIMAR-21 cruise was carried out during 01–30 October 2015 onboard the Chilean R/V Cabo de Hornos. The cruise surveyed the transect between Caldera (27° S, 70°50′ W) and Easter Island (27°10′ S, 109°30′ W) covering 30 oceanographic stations (Figure 1A). We performed zooplankton sampling in 17 stations as indicated in Figure 1. At each station a Rosette-CTDO was deployed to a maximum depth of 1,500 m to obtain vertical profiles of temperature, salinity and dissolved oxygen. Water samples were also obtained at 15 depths for chemical analyses of oxygen, salinity and nutrients. At the zooplankton stations a Hydrobios Multinet Midi type of 0.25 m2 opening mouth was vertically hauled from 800 m to surface. The Multinet had five nets 200 μm mesh-size which were electronically opened and closed at five depth strata: 0–100, 100–200, 200–400, 400–600 and 600–800 m. Hauling speed was 1 ms-1 and the samples were collected at different times of the day and likely different light conditions (Table 1). There were five stations sampled at nighttime conditions Once onboard, samples were collected in buckets and depending on the amount of zooplankton they were split in two fractions; one fraction was immediately preserved in 10% formalin and the second fraction was frozen at -20°C for C and N measurements. Small samples were frozen as whole.
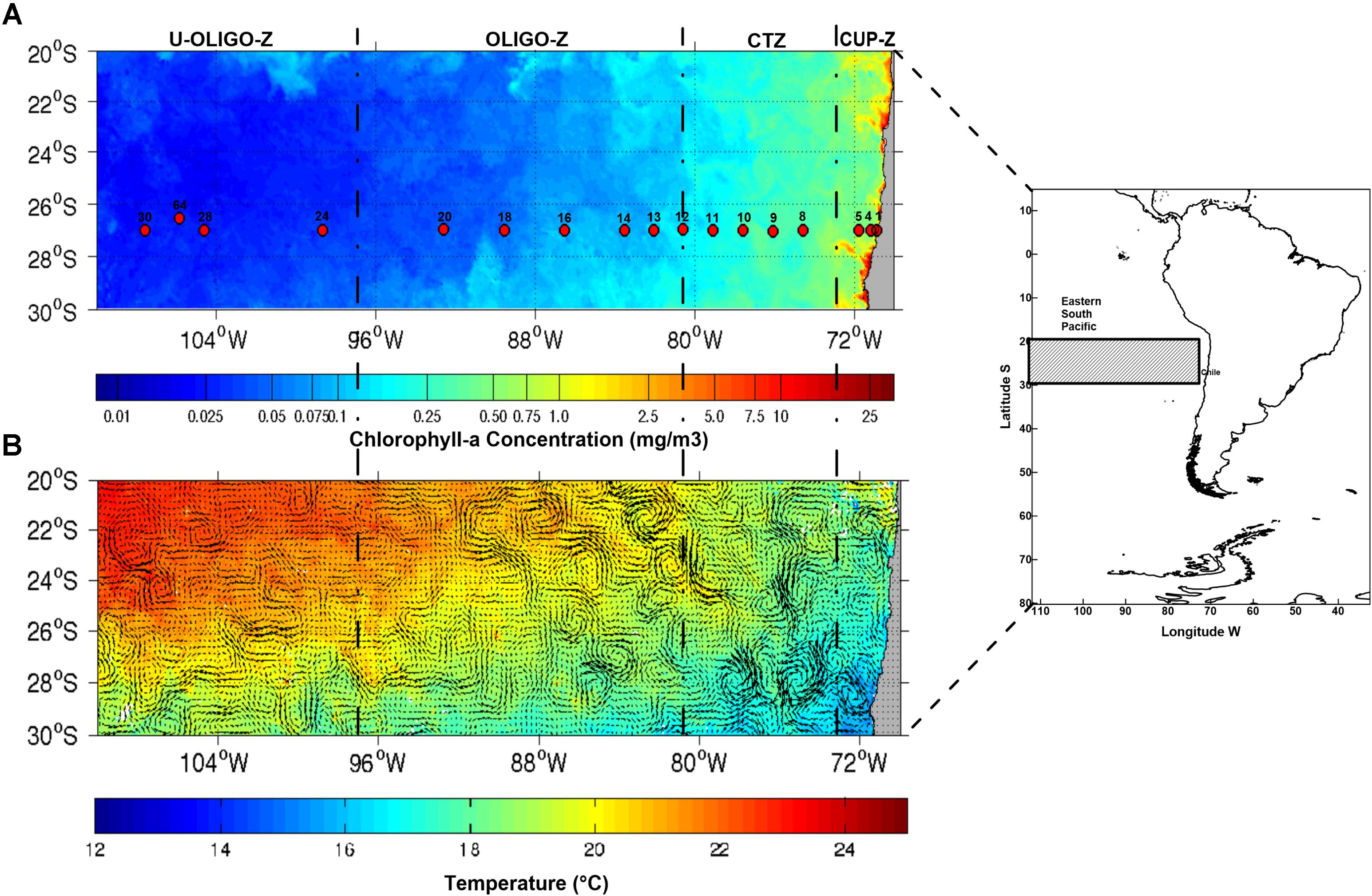
Figure 1. The eastern south Pacific region where the CIMAR-21 cruise was carried out in October 2015: (A) representation of the sampling stations across the four study zones defined from satellite chlorophyll-a levels as: coastal upwelling area (CUP-Z), the mesotrophic coastal transition zone (CTZ), the oligotrophic area (OLIGO-Z) and the ultra-oligotrophic region (U-OLIGO-Z), (B) satellite surface temperature and geostrophic surface currents during the cruise.
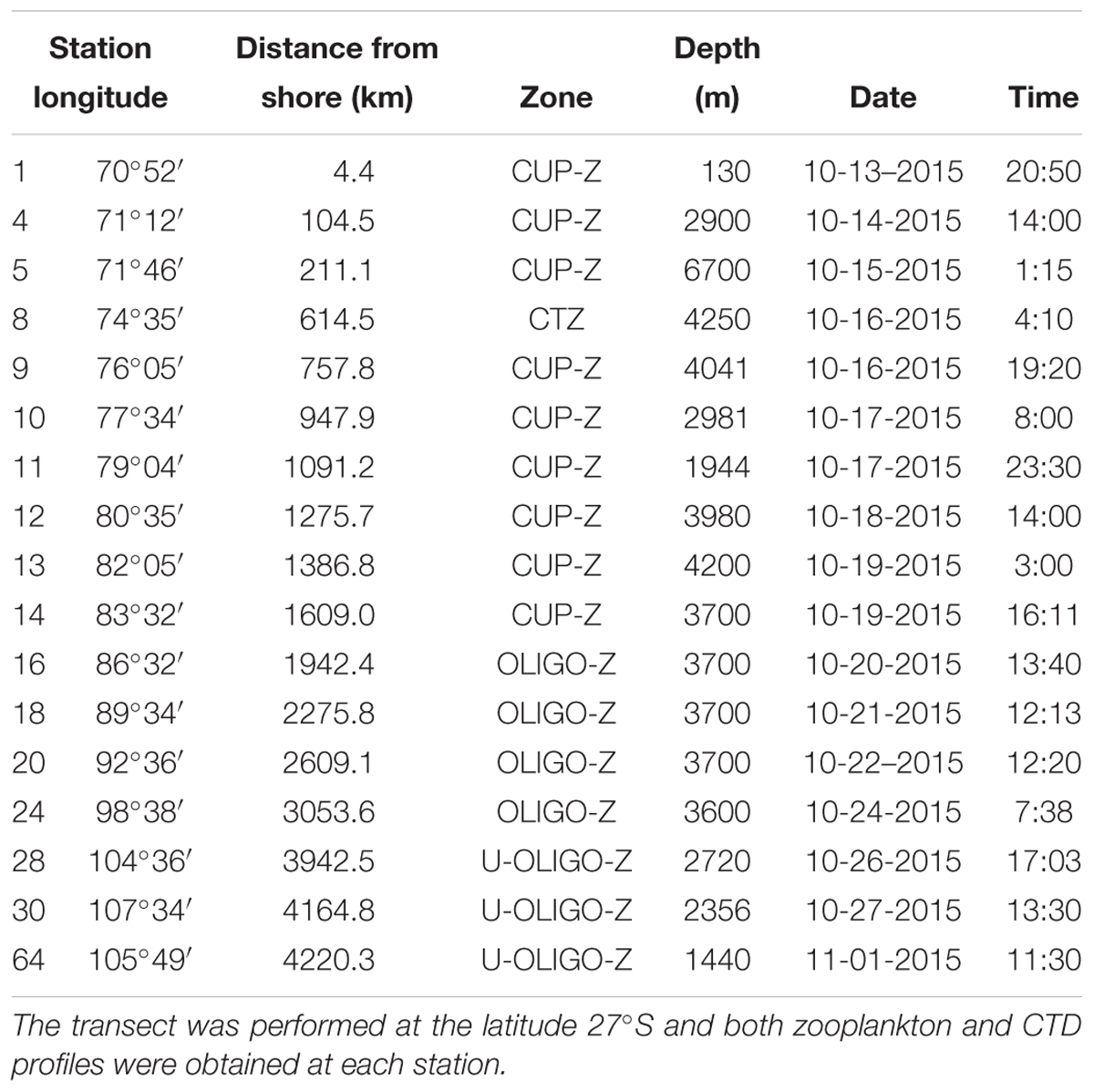
Table 1. Description of the sampling stations used for the study from the coast to open ocean waters.
Zooplankton Analyses
In our study, we used samples from the two upper layers, i.e., 0–100 and 100–200 m. Frozen samples from these strata were first thawed and both mixed to increase the amount of zooplankton. Half of this sample was preserved in formalin for analysis of composition. Samples were first diluted with filtered seawater (GF/C filters) and then fractioned in five size classes using sieves. The sieves were 2,000, 1,000, 500, 200 and 60 μm, so that the following size classes were defined: <200 μm, 200–500 μm, 500–1,000 μm, 1,000–2,000 μm, >2,000 μm. All fractionated samples were then received onto pre-weighed GF/C filters, dried in an oven at 70°C for 48 h. Each filter sample was removed with a spatula and homogenized with a pestle to have a dry weight of about 1 mg to feed an elemental analyzer (Flash EA2000) coupled with a mass spectrometer isotope ratio (DELTA V The IRMS). Abundance values of natural stable isotopes were reported as δ15N or δ13C (‰) relative to atmospheric nitrogen and Vienna Pee Dee Belemnite, respectively (Coplen, 2011). The associated isotopic error was 0.218 and 0.43‰ for Nitrogen and Carbon, respectively. In turn, the error associated in the elemental analysis was 0.001 and 0.01 mg for Nitrogen and Carbon correspondingly, as assessed by a linear regression. The standard used for this calculation was Acetanilide, which contains 71.10% carbon and 10.36% nitrogen. For the calculation of the isotopic proportion, we used four previously standardized internal standards, Acetanilide, Atropine, Caffeine, Organic Sediment and Glutamic Acid. Determination of the isotopic composition and elemental contents were performed by LABASI Laboratory of Biogeochemistry and Applied Stable Isotopes of the Pontificia Universidad Católica (Chile). A high variation in lipid content in zooplankton samples was obtained, as shown in the value of the ratio C:N > 3.5. Therefore, δ13C values were normalized (δ13Ccorr) using an empirical relationship with the sample C:N value (by weight) as determined by meta-analysis with data from aquatic animals (Post et al., 2007): δ13Ccorr = δ13C -3.32 + 0.99 C:N.
All the samples preserved in formalin were analyzed by a ZooScan Hydroptic to obtain digitized images of samples which were thereafter processed with ZooProcess software V1.5. This automated method to analyze zooplankton is based on the RAPID approach (Benfield et al., 2007) which allows us to obtain size distribution of zooplankton and identification and counting of major dominant taxonomic categories. For this, samples are scanned with a resolution of 2,400 dpi with no staining.
Data Analysis
Using satellite data of Chlorophyll-a (Chla), available for the cruise period from (https://oceancolor.gsfc.nasa.gov/), we defined four zones in according to observed ranges of surface Chla: the eutrophic zone corresponding to the coastal upwelling area off Chile with Chla >0.5 mg m-3 (CUP-Z), the mesotrophic CTZ with a Chla range of 0.1–0.5 mg m-3, the oligotrophic area (OLIGO-Z) with a Chla range of 0.05–0.10 mg m-3, and the ultra-oligotrophic region (U-OLIGO-Z) corresponding to the central south Pacific gyre with Chla concentrations <0.05 mg m-3 (Figure 1A). Satellite surface Chla were obtained. Complementary satellite data on sea surface temperature was obtained from the NASA’s Ocean Biology Processing Group (OBPG) https://oceancolor.gsfc.nasa.gov/, and surface geostrophic current also from www.aviso.altimetry.fr (Figure 1B). All data used for the Chla, temperature and geostrophy maps were daily averages for the sampling period, 13–27 October 2015, and covered the area surrounding the oceanographic transect.
Variability in zooplankton abundance, biomass, size-fractions, C and N contents and δ15N and δ13C, as well as for oceanographic variables, across the four zones were examined, and differences tested by means of Kruskal–Wallis and GLM (General Linear Models) using the software SYSTAT 12 (Systat Software Inc., San Jose, CA, United States). In most cases, log-normalized variables were used for GLM and in other cases we used the non-parametric test Kruskal–Wallis, due to significant deviations from normality and homogeneity of variance, as examined by the Shapiro–Wilks and Levene tests, respectively. The post hoc tests used were those of the Pgirmess package of R, which performs multiple Tukey’s range comparisons after Kruskal–Wallis test (Dickinson and Chakraborti, 2003) and the Games-Howell Test to perform paired comparisons in the case GLM.
The use of different resources by zooplankton size classes in each zone was investigated by estimations of the isotopic niche. For this analysis, the niche amplitude was calculated from the space delimited by the values of δ15N vs δ13Ccorr for all study areas and size classes with the SIBER package developed in the R program (Jackson et al., 2011). The standard ellipses calculated could thus represent the distinct food sources and their utilization (Layman et al., 2007, 2012). Layman metrics (Layman et al., 2007) were estimated to describe these ellipses, as follows: NR: range of δ15N (diversity of trophic levels and nitrogen sources), CR: range of δ13Ccorr (diversity of carbon sources), TA: total area of the convex hull encompassing all data points (trophic diversity and niche size), CD: centroid distance (average measure of trophic diversity among consumers), MNND: mean distance to the nearest neighbor (trophic redundancy, lower when isotopic niches are similar) and SDNND: SD of distance to the nearest neighbor (trophic equality). Maximum likelihood estimates of ellipse areas were used for graphical representation while estimates of niche overlap were made from ellipses encompassing 95% of data. Niche width was estimated using Bayesian inference of the standard ellipse areas to account for the uncertainty in sample data (Jackson et al., 2011) after 104 estimations per ellipse.
The variability in community structure across the study area was studied from the taxonomic categories provided from the ZooScan analysis, expressed in individuals m-3. The community structure was assessed by means of multivariate analyses performed with PRIMER v.7 (Clarke and Gorley, 2006). First, we evaluated similarities within and between zones in terms of relative abundance. A cluster analysis and multidimensional scaling (NMDS) were done after a fourth root transformation of data, with the Bray-Curtis index as a distance measurement. Second, ANOSIM was used to test the hypothesis that community structure and abundance of zooplankton differed among zones. Finally, the differences or similarities between the study areas were further assessed with SIMPER (percentages of similarity).
Results
Oceanographic Characterization
Temperature of the water column throughout the coast-offshore gradient (Figure 2A) showed that the upper layer ranging between surface and 50–300 m, depending on the region, accounted for most of the variation. This layer was approximately delimited by the 14°C isotherm. The CUP-Z had the lowest near-surface temperature (<15°C) over a narrow upper layer of less than 100 m. The CTZ showed a temperature range between 16 and 18°C within the upper 200 m with presence of a surface front at about 78° W. The OLIGO-Z was more extensive and its temperature in the upper 200 m varied between 18 and 19°C, whereas the U-OLIGO-Z exhibited a surface warming reaching down to 300 m depth, and a temperature maximum of about 21°C at the surface. Mean temperature at 10 m depth was significantly different between zones (p < 0.001) and post hoc tests indicated that OLIGO-Z and U-OLIGO-Z were not different in temperature (Table 2). Contrasting with temperature, salinity exhibited a greater variation, both over the horizontal and vertical gradients in the upper 1,000 m, characterized by a large and very extensive low salinity (<34.4) water mass located between ca. 300 and 1,000 m, becoming shallower toward the coast. In the upper 200 m, low salinity water (<34.6) prevailed in the CUP-Z and in the CTZ, whereas in the U-OLIGO-Za high salinity (>35.5) water mass dominated the upper 200 m intruding the western part of the OLIGO-Z (Figure 2B). Surface salinity among zones was also significantly different (test, p < 0.001), although post hoc tests showed no differences between OLIGO-Z and U-OLIGO-Z (Table 2). Distribution of dissolved oxygen showed a strong variation within the CUP-Z and the CTZ in the 400 m, reflecting the presence and distribution of the oxygen minimum zone (OMZ) in these areas and marking the western limit of the CTZ (Figure 2C). Mean oxygen in the upper 100 m was significantly different among zones (test, p < 0.001), except that CUP-Z and U-OLIGO-Z had no difference and the same was found for OLIGO-Z and U-OLIGO-Z, in according to post hoc tests (Table 2).
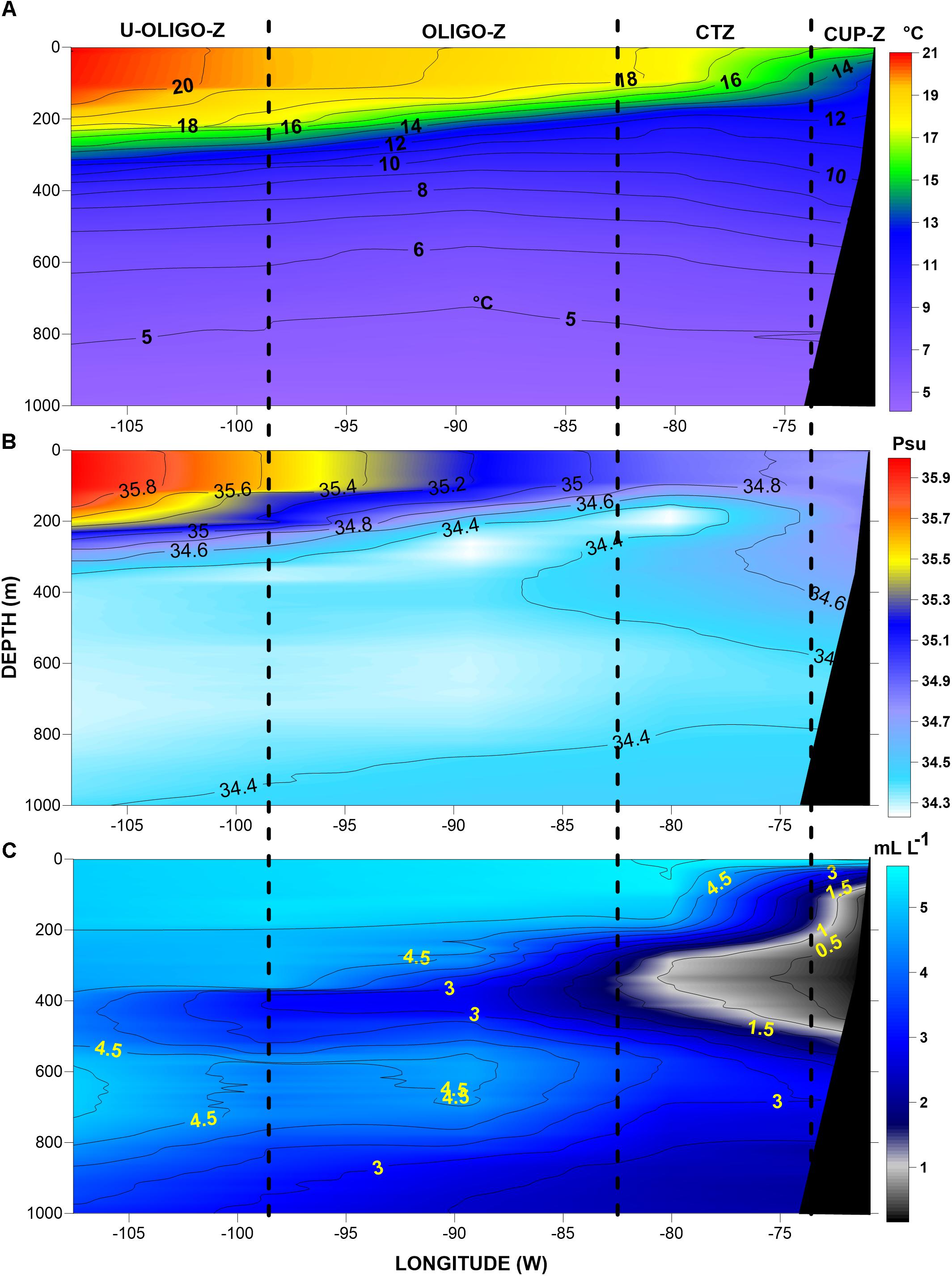
Figure 2. Hydrographic conditions of the water column across the zonal gradient from the Chilean coast to Easter Island from CTDO profiles to 1,000 m: (A) temperature, (B) salinity and (C) dissolved oxygen (mL L-1).
Maximal zooplankton biomass was found in the upwelling zone (Table 2), decreasing abruptly toward the central gyre with extremely low values (<1% compared to the upwelling zone). Temperature, salinity and oxygen all increased toward the oceanic region, while surface Chla sharply decreased down to less than 0.1 mg m-3 in the OLIGO-Z and U-OLIGO-Z. Fractionated biomass showed that large-sized classes (>1,000 μm) had lower values than small ones (Table 3). Mean C:N ratios tended to appear higher (>7) in the small size class, but also in the largest one, whereas intermediate size classes had C:N mean ratios <6. Mean values varied in rather narrow ranges of -18.0 and -19.7 and 9.0 and 11.0, respectively (Table 3).
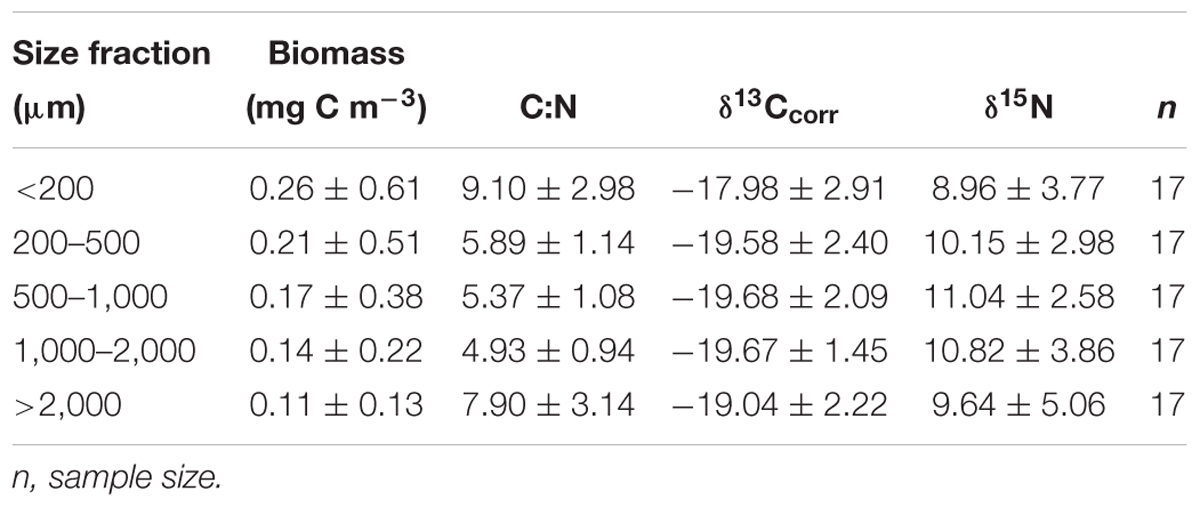
Table 3. Size-fractioned zooplankton biomass (mean ± SD) and their isotopes composition of C and N. Biomass is in terms of C, the C/N ratio and isotopic composition of δ13C and δ15N.
When including all sampling stations from the four zones, a GLM showed that zooplankton differed significantly in the C:N ratio (p = 0.01), δ13Ccorr (p = 0.01) and δ15N (p = 0.00) between zones (Table 4). However, size fractions of zooplankton only showed significant differences in the C:N ratio (p < 0.01) (Table 4). For instance, the C:N ratio strongly varied across the zonal gradient in all size classes, characterized by lower C:N ratios in the coastal zone with values between 4 and 7, and then incrementing toward offshore, reaching maximum values of ca. 15 in the OLIGO-Z in the >2,000 μm fraction, although an abrupt decrease in C:N was detected in the 1,000–2,000 μm fraction at the station near Easter Island (Figure 3A). In general, small size fractions (<500 μm) showed greater C:N ratios (7.5–14.0), whereas larger size classes (>500 μm) showed more variation between stations within zones. When comparing C:N ratios between zones, it was found that CUP-Z and CTZ were not different to each other (Test, p > 0.05), but CUP-Z was different from the U-OLIGO-Z (Test, p = 0.05), as well as CTZ from the U-OLIGO-Z (Test, p < 0.05). Pairwise comparisons between zones for isotopes composition showed that for δ13C even though there significant differences among zones as found by GLM (Table 4) the Games-Howell Test was unable to detect the pairwise differences (Test, p > 0.05), possibly because the variance within zones was too high. In the case of δ15N the pairwise comparisons test showed that OLIGO-Z and U-OLIGO-Z did not differ (Test, p > 0.05), while all the others significantly differed to each other (Test, p < 0.05).
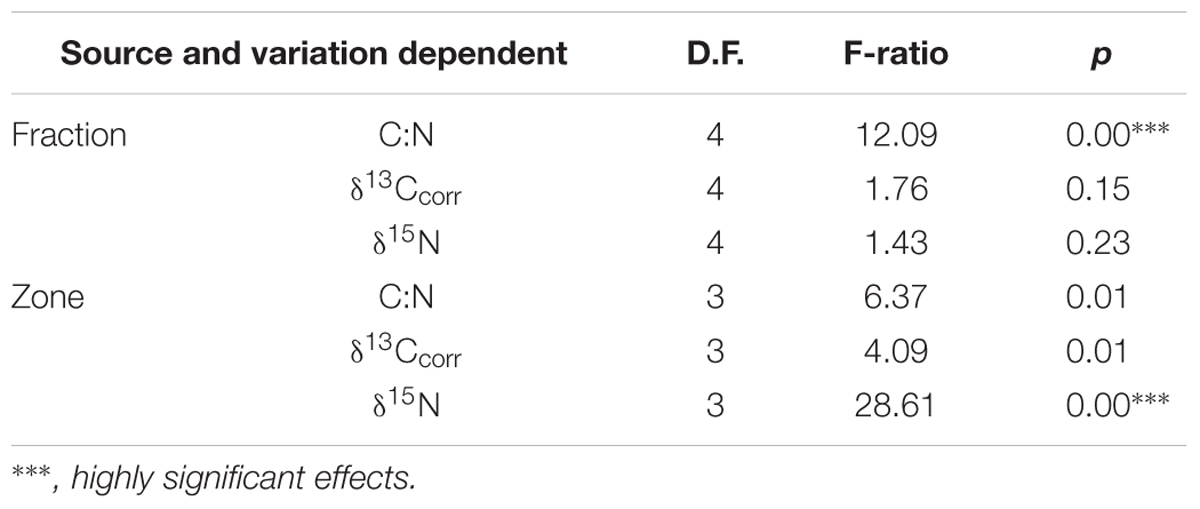
Table 4. A Generalized Linear Model (GLM) to test the effect of size fractions and zone on C:N ratio and isotopes composition of C and N of mesozooplankton across a zonal gradient in the eastern south Pacific.
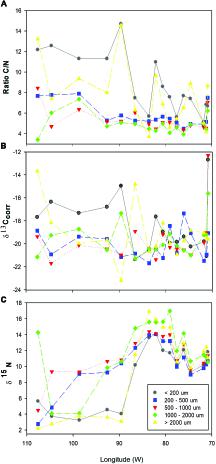
Figure 3. Biochemical variables of size-fractioned zooplankton across the zonal gradient: (A) C:N ratio, (B) stable carbon isotopes (δ13C), (C) stable nitrogen isotopes (δ15N).
The distribution of δ13C across the zonal gradient showed variable patterns, depending on the size fraction, although most fractions exhibited a greater value at the upwelling, a reduction in the CTZ and then higher values again toward the open ocean (Figure 3B). Different patterns were observed in the zonal distribution of δ15N, with a downward trend toward offshore observed in all size fractions (Figure 3C). There was a remarkable sharp decrease in δ15N from the last portion of the CTZ to the OLIGO-Z and U-OLIGO-Z areas with most values of <5‰. A similar pattern through all size classes suggested the same source of N for zooplankton in these areas.
From GLM analysis differences in C:N ratios and isotopes composition of C and N size fractions were compared. Within the different size fractions, significant differences were observed only in C:N ratio (p = 0.00). Post hoc analyzes indicated that the fraction <200 μm was significantly different from the other four fractions (Test, p ≤ 0.05). Also significant differences were observed between the fractions >2,000 μm and the intermediates size classes (1,000–2,000 and 500–100 μm) (Test, p ≤ 0.05).
Isotopic Niches
The samples projected on the isospace of δ15N and δ13C, showed distinct patterns between zones, with a greater range in δ13C in CUP-Z and U-OLIGO-Z, and a maximum of δ15N in CTZ (Figure 4). In the CUP-Z and CTZ zones, values of δ15N were >10‰, but in other zones, such as OLIGO-Z and U-OLIGO-Z some samples could reach values <2‰. The ellipses of the five fractions for the four zones revealed different distributions, depending on functional groups (size fractions) and the zone, but it also evidenced a large overlapping among ellipses, with the exception of the U-OLIGO-Z (Figure 4).
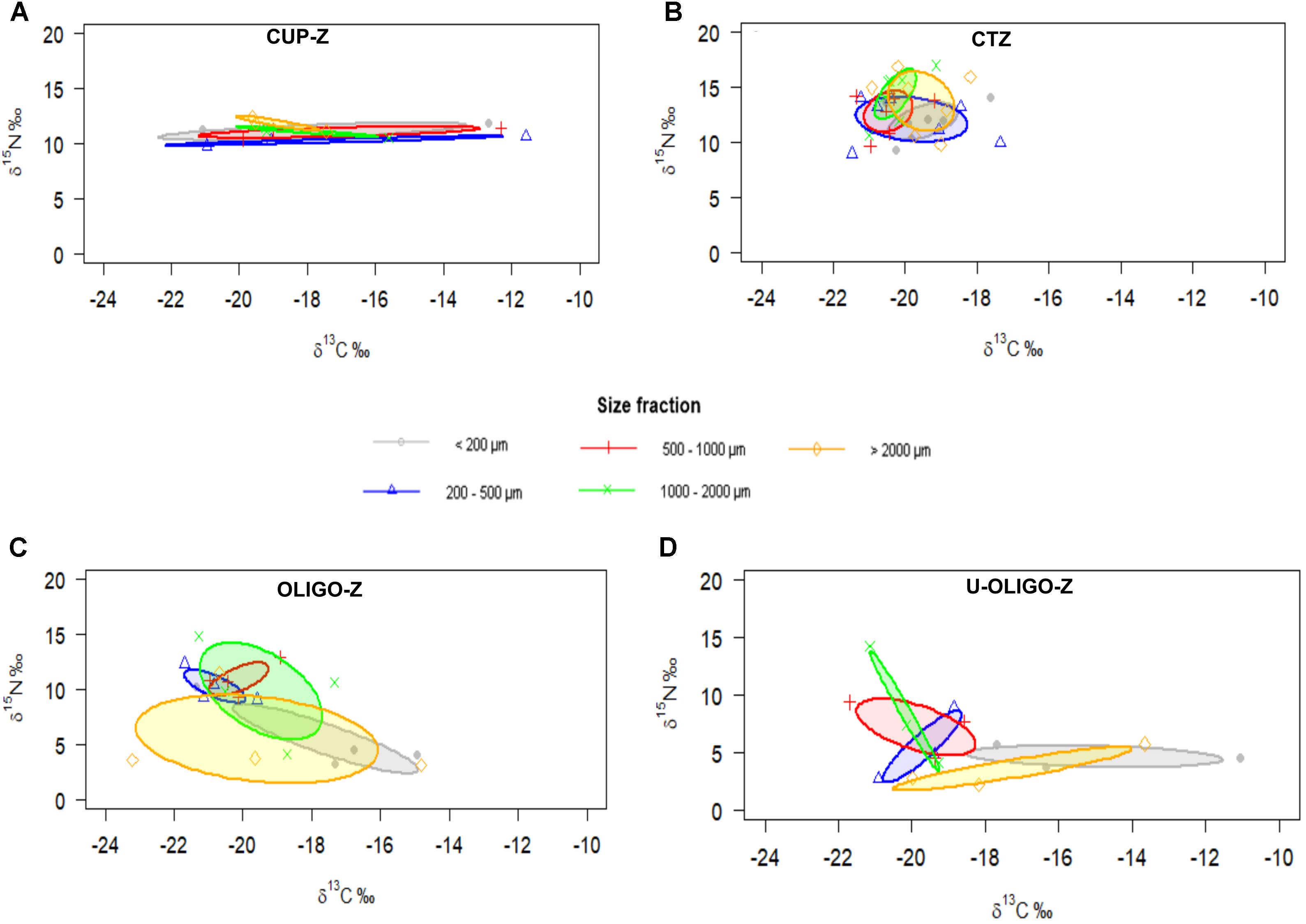
Figure 4. Layer isospaces of five size fractions for δ13C and δ15N, including the individual measurements of the samples for each zone: (A) CUP-Z, (B) CTZ, (C) OLIGO-Z and (D) U-OLIGO-Z. The maximum likelihood calculated of standard ellipse areas for each size fraction (continuous line).
The Layman metrics, of the Bayesian approximation of the ellipses for the four study areas, revealed that in the OLIGO-Z and U-OLIGO-Z zones there is a potential greater number of trophic levels, and nitrogen sources when compared to the other zones (higher NR). In terms of C sources, only the region U-OLIGO-Z showed large variability (higher CR). From the TA values, the OLIGO-Z and U-OLIGO-Z zones, revealed that these regions have a large trophic diversity and niche amplitude (higher TA), and thus differing from the coastal area. The mean trophic diversity among consumers (CD) showed higher values mainly in U-OLIGO-Z, being slightly higher in the OLIGO-Z. The highest similarity in isotopic niches (low MNND) was observed in the CUP-Z and CTZ zones, while the more oceanic areas showed greater values. The distribution of trophic diversity (SDNND) was more uniform in the U-OLIGO-Z zone, and to a lesser extent in the OLIGO-Z with respect to the other ones (Table 5). As most of the variation in isotope composition was found between zones, Bayesian estimates of the ellipse areas by zones (all size-classes combined) indicated a large similarity of the isotopic niche between the coastal zones on one side and between the oligotrophic zones on the other side (Figure 5). Niche overlap was generally <20% for most zones but increased for neighboring zones, and even exceeded 50% in the oligotrophic region (Table 6).
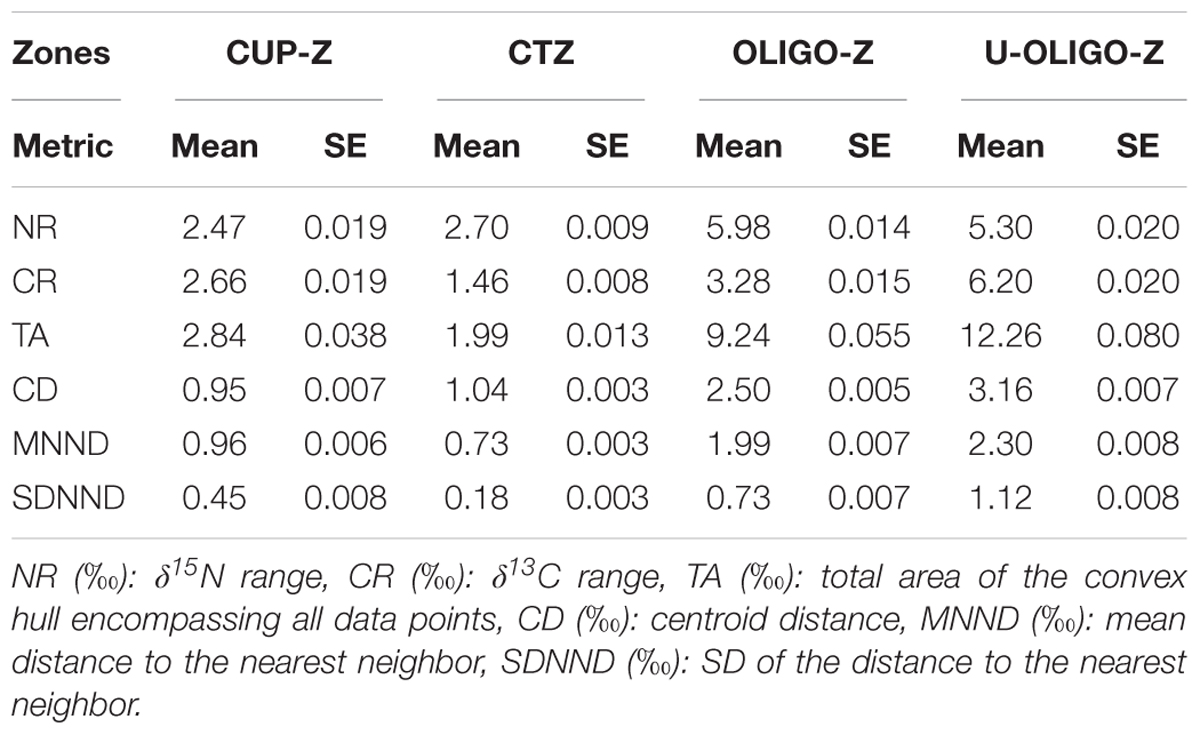
Table 5. Layman metrics (mean ± SE) estimated by a Bayesian approach from the area defined by δ15N and δ13C of mesozooplankton for four study zones (all size-clases combined).
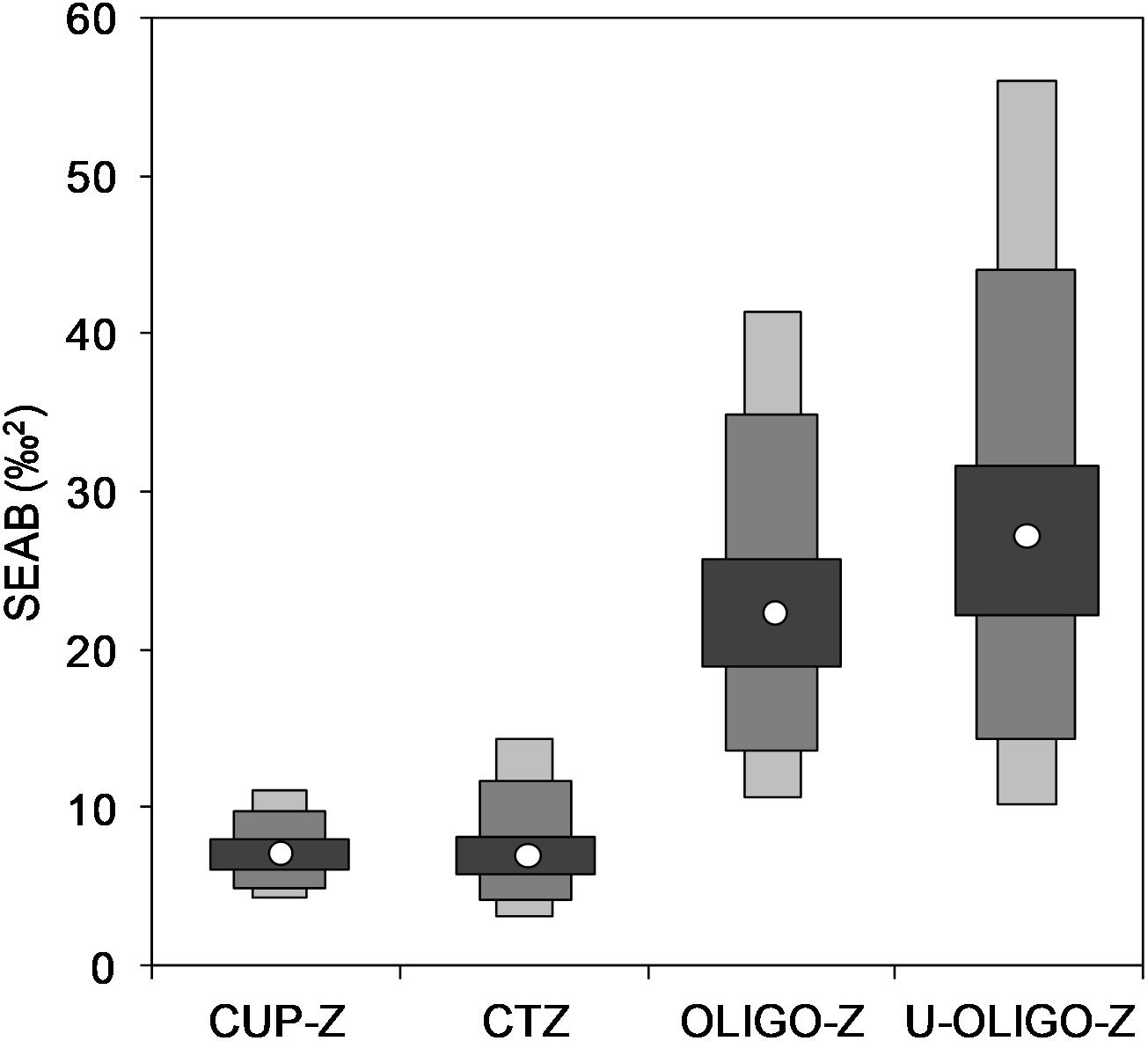
Figure 5. The standard ellipse areas (SEAB) for the plankton communities considered in this study and computed from the Bayesian multivariate distributions fitted to the original isotope data for each zone (all size-classes combined). The modes (white dots) and the intervals encompassing 50, 95, and 99% of the estimations (shaded boxes) are indicated.
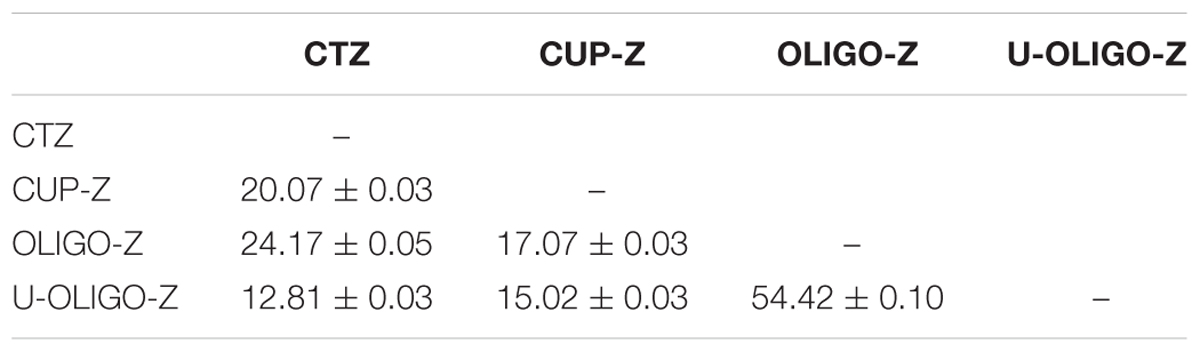
Table 6. Mean ± SE overlap [expressed as a fraction (%) of the non-overlapping area] between estimates of ellipses encompassing 95% of isotope data (all size-classes combined) of the different zones showed in Figure 4.
Zooplankton Communities
When analyzing the zooplankton community by the automated approach using a ZooScan, it was found that 19 taxonomic categories could properly the composition in terms of major groups. However, 9 of them appeared as mostly controlling the total abundance for all samples. Small sized copepods (<1.5 mm), followed by large size copepods (mainly > 2 mm), were the most abundant organisms at all regions, and with greater abundances in the CUP-Z and CTZ (Figure 6). In terms of relative abundance, the prevalence of small copepods and salps increased in open ocean waters. Some taxonomic groups did not show large variations in their abundance across of the study area, such as chaetognatha, appendicularia and eggs (mainly fish eggs). While others were more abundant in specific areas, such as euphausiids in the CUP-Z region (Figure 6).
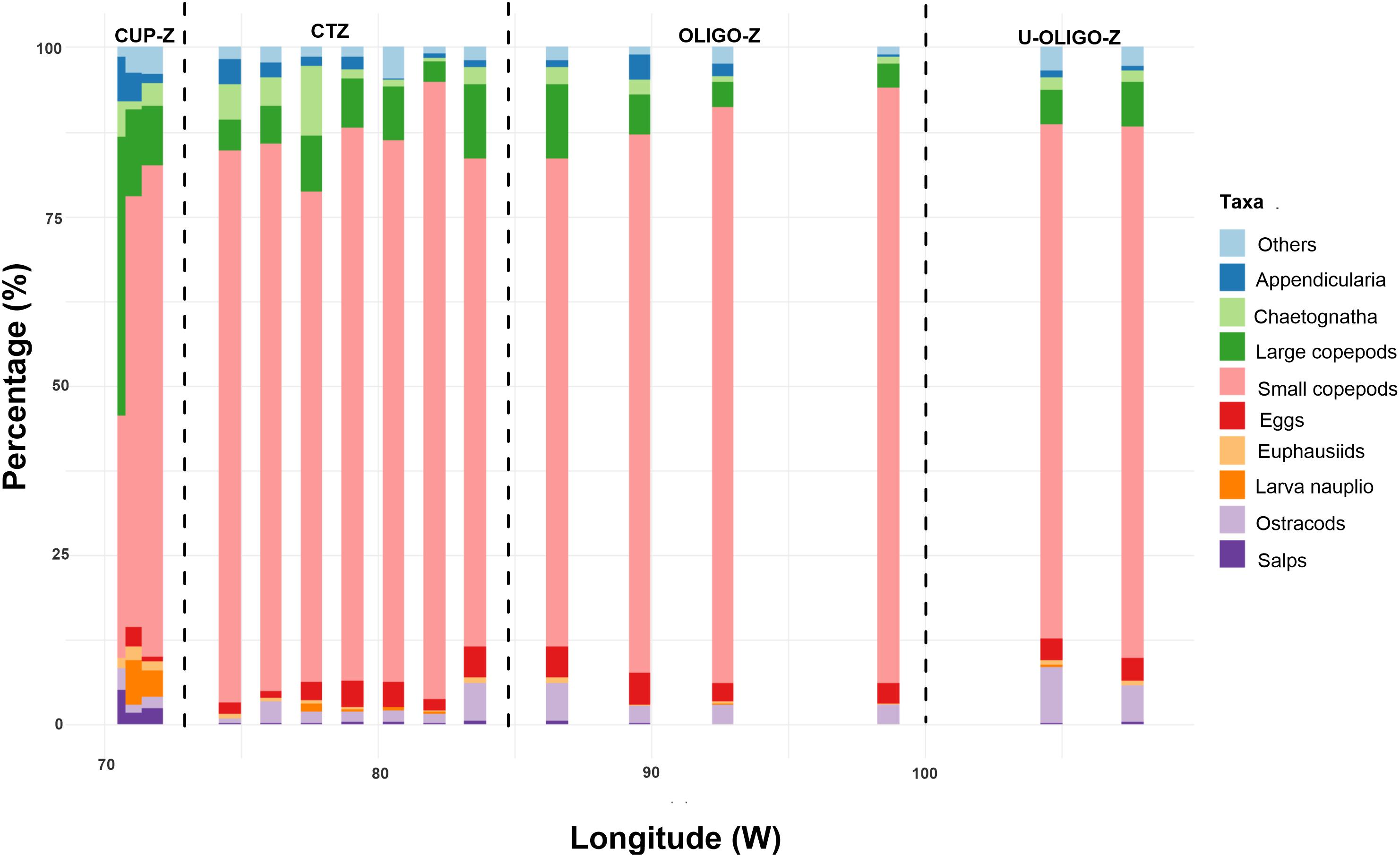
Figure 6. Variation in relative abundance (%) of the most abundant taxonomic categories sampled during the cruise CIMAR-21 from the Chilean coast to Easter Island for a depth strata 0–200 m. The taxonomic categories were assessed by automated analysis (ZooScan). Four study zone were defined: CUP-Z, CTZ, OLIGO-Z and U-OLIGO-Z.
For a more detailed analysis of the zooplankton community structure, associated with the four regions, a multivariate community analysis was performed with PRIMER (V. 7). Cluster analysis (Figure 7A) showed that the CUP-Z clearly separates from the other zones, but also with strong internal variation, whereas the CTZ exhibited a more homogeneous pattern (>80% similarity). The OLIGO-Z tended to mix with CTZ and U-OLIGO-Z. The latter also formed a separate cluster, although it became mixed with the OLIGO-Z. ANOSIM showed significant segregation of the four zones (Figure 7B) with R = 0.482 and a significant level of 0.08%, indicating that the community structure between zones are different, as based on the automated taxonomic analysis.
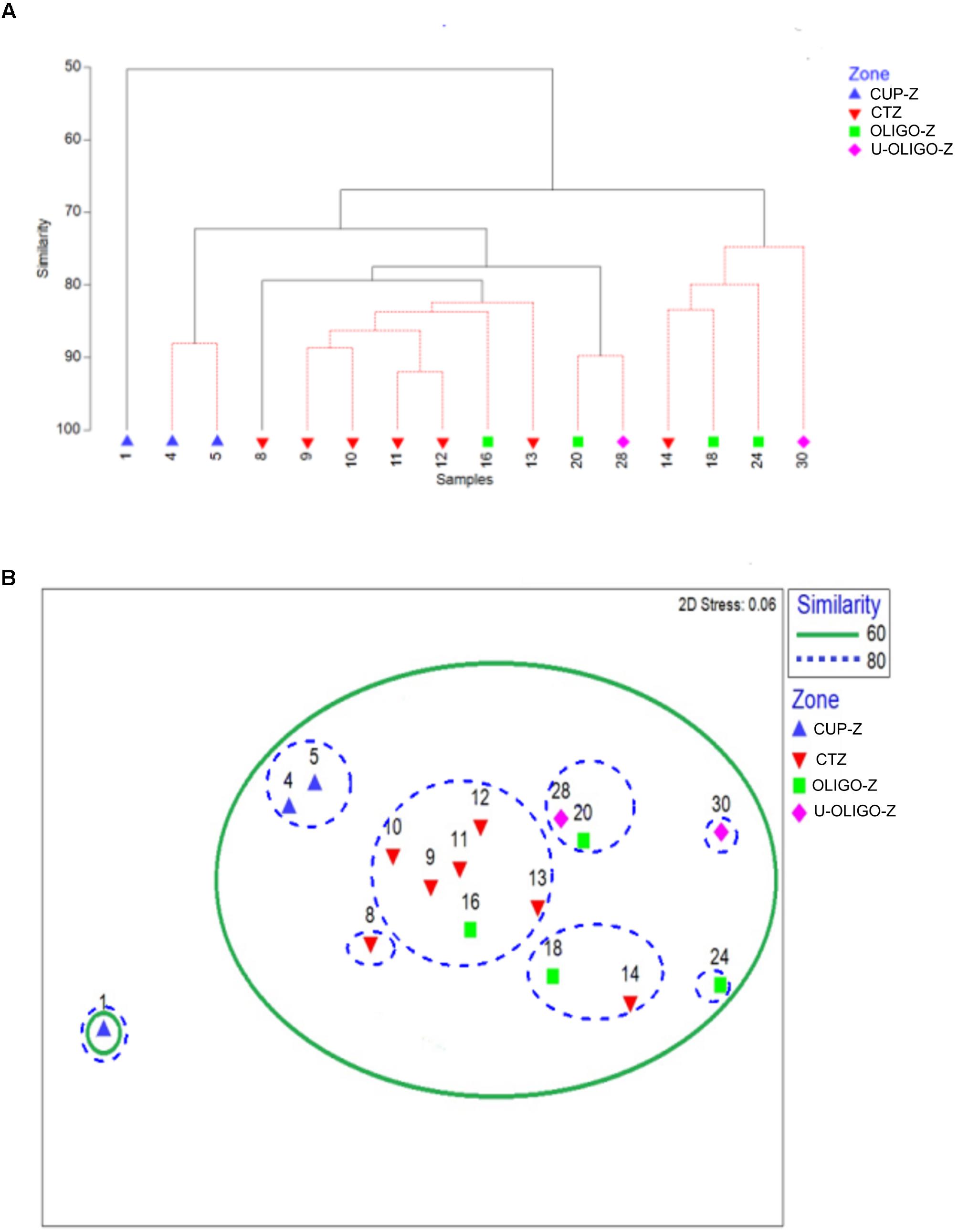
Figure 7. Cluster analysis of taxonomic groups of zooplankton from the upper 0–200 m over the zonal gradient from the Chilean coast to Easter Island (A) similarity distance was Bray Curtis. Non-metric multidimensional scaling graph (NMDS) based on the Bray-Curtis distance index of transformed from the abundance data of the taxonomic groups (B). Both analyses were associated with four zones across the zonal gradient: Eutrophic (CUP-Z), Mesotrophic (CTZ), Oligotrophic (OLIGO-Z) and Ultra-oligotrophic (U-OLIGO-Z).
Discussion
Our observations clearly showed a heterogeneous environment across the coast-offshore gradient, characterized by either gradual or abrupt changes in physical, chemical and biological variables. These results are consistent with previous observations (e.g.,: Palma and Silva, 2006; Dolan et al., 2007; Raimbault and Garcia, 2008; Cornejo et al., 2015). It is important to consider that boundaries of our proposed zones were arbitrary and based on surface Chla levels, so that the areas occupied by each zone were very different. For instance, the CUP-Z represented only 5% of the sampled region, the CTZ 38%, the OLIGO-Z 72% and the U-OLIGO-Z 7%. This arbitrary division of zones was intended to represent potential biogeochemical regions, in the sense that distinct processes could determine the sources of C and N. These processes refer for example to nitrogen fixation (Montoya et al., 2004; Espinasse et al., 2014; Hunt et al., 2015), denitrification (Dalsgaard et al., 2012), oligotrophy vs eutrophy and the ratio between new and regenerated production (Dugdale and Goering, 1967), and outgassing driven upwelling, among others.
However, variability in biochemical conditions of zooplankton may not only depend on the sources of nutrients, but also on the prevailing environmental conditions and also on some ecological processes. For example, physiological and nutritional conditions of organisms can be modified by factors, such as oxygenation (Seibel, 2011), temperature (Atkinson, 1994), pH (Yamada and Ikeda, 1999). In the same context, food conditions are critical for determining physiology and nutrition of zooplankton. Both food quantity and quality play a key role in that sense (e.g., Hirst and Bunker, 2003; Vargas et al., 2006), through food availability, feeding behavior and selectivity (DeNiro and Epstein, 1978). Body composition and structure (e.g., quitinous/gelatinous) may also affect nutrient composition, including the isotopes signals (Webb et al., 1998). Life cycles and ontogenia can also cause variation in physiological and nutritional conditions (Tibbets et al., 2008). All these integrated processes may ultimately affect the C:N ratios and isotopes composition. However, we postulated that the main sources of C and N, which in turn depend on the above defined biogeochemical regions, are the key driving force for determining such biochemical features. As supporting this hypothesis, we found that C:N ratios, for all size fractions, were similar between CUP-Z and CTZ, but different when those of the OLIGO-Z and U-OLIGO-Z which exhibited much higher values. Elevated C:N ratios in zooplankton tissue in both oligotrophic areas may be explained by a N-deficiency upon more sable levels of C. N-deficiency is a well-known characteristic in open ocean areas of the Eastern South Pacific (Altabet et al., 2012; Stramma et al., 2013; Cornejo et al., 2015). However, C:N ratios >3.5 in all fractions can indicate excess of lipids in animal body (Post, 2002; Smyntek et al., 2007). Lipid storage is probably a strategy against food shortages (Lee et al., 2006), resulting in higher C:N ratios in oceanic regions. In both cases, N-deficiency and food shortage are processes triggered by an extreme oligotrophic condition which is one of our criteria to define biogeochemical zonation.
When comparing the zones in terms of isotopes composition, the patterns varied between δ13C and δ15N. From the isospaces and Layman metrics (Figure 4 and Table 5) it was found that the range of δ13C differed between zones, suggesting a variable origin of C for zooplankton biomass across the zonal gradient. A highly regenerated source of C for zooplankton biomass may be expected in the open ocean (Williams, 1981; Wollast, 1998; Del Giorgio and Duarte, 2002), compared to the upwelling zone where the major source of C is freshly uptaken from an atmospheric source (Gruber et al., 1999; Mompeán et al., 2013). Also in the upwelling zone greater δ13C can be found after dominance of diatoms which exhibit a range between -15 and -19 compared to -21 and -25 in dinoflagellates which are more abundant in the open ocean (Nakatsuka et al., 1992). The large variability in δ13C (but not in δ15N) observed in this zone for all size fractions can be interpreted as the consequence of the dynamics of upwelling, stimulating diatom blooms near the coast but also introducing phytoplankton species from the neighboring CTZ region, where diatom abundance is expected to be lower. Finally, higher values of CR found in the oceanic areas show a variable source of C. However, it was not possible to differentiate if the source of C was preferentially from diatoms or dinoflagellates, as in other studies (e.g., Perry et al., 1999), because in the oligotrophic zone the phytoplankton may not cover the required carbon demand by zooplankton (Zhang et al., 1995), and therefore zooplankton compensate this deficiency by feeding on microzooplankton (Kleppel, 1993; Calbet and Landry, 1999; Calbet and Saiz, 2005) which would translate in changes in δ13C. Moreover, potential advection of organisms from coastal areas could also cause variability in the source of C by mixing processes (Gruber et al., 1999).
In turn, δ15N clearly decreased toward the open ocean suggesting a changing source of N for zooplankton across the zonal gradient. A variable source of N might be related to the type of food for zooplankton feeding. Even though our zooplankton size fractions may be comprised by a variety of trophic behaviors (carnivorous or herbivorous) the ultimate N source should mostly be phytoplankton, probably derived from diazotrophy, as reflected in the low values of δ15N. This can thus be interpreted as evidence for presence of atmospheric nitrogen being fixed by phytoplankton and channeled to the tropic web (McClelland et al., 2003; Mompeán et al., 2016). There are, however, other processes potentially modifying δ15N. For instance, in oligotrophic environments nitrate inputto the photic zone may occur by diffusion through the pycnocline (Mouriño-Carballido et al., 2011; Fernández-Castro et al., 2015) providing N for new production with low δ15N. Furthermore, diel vertical migration (DVM) of large-size zooplankton would facilitate the access to different preys causing variability in isotopic composition of zooplankton in large-size classes compared to smaller fractions (Cartes et al., 2007; Agersted et al., 2014). In any case, phytoplankton composition may have a key role for determining biochemical and nutritional conditions of nitrogen in zooplankton. Across the coastal-offshore gradient it is known thatthe processes that modulate the availability of nitrogen in the coastal zone differ to those of more oceanic areas, for example, presence of a shallow OMZ promotes the denitrification process in the upwelling zone (Thamdrup et al., 2006; Galán et al., 2009; Dalsgaard et al., 2013), favoring an enrichment in δ15N.
With respect to ecological zonation across the zonal gradient, we found that the zooplankton composition changes significantly from the CUP-Z toward the U-OLIGO-Z. Our automated analysis of zooplankton does not provide information on the species composition, but only on the proportion and abundance of different taxonomic categories. This proportion changes from one zone to the other and most likely the species also do so from the coastal area to the oceanic region, as seen for example in euphausiids species (Riquelme-Bugueño et al., 2012) and cnidaria (Palma and Silva, 2006). Species composition may also influence the isotopic values of zooplankton due to variability in trophic position in different zooplankton assemblages (Matthews and Mazumder, 2003), and thus some of the variation in δ15N may relate to changing species composition. Sampling method is another potential source of variation for the zooplankton composition. Depending on the sampling gear, mesh-size of the plankton net and light conditions, DVM cannot be depicted properly (see Harris et al., 2000 for review). Our Multinet targets the mesozooplankton community which is mostly dominated by copepods. In this group DVM is mostly restricted to the upper 200 m and even shallower upon presence of the OMZ (Escribano et al., 2009). More extensive DMV is mainly performed by micronekton, including euphausiids and fish larvae (Harris et al., 2000) which cannot be properly sampled by the Multinet. Therefore, within the upper 200 m we have assumed that our mesozooplankton community is adequately represented by our sampling protocol. In sum, changes in community structure can be driven by a variety of denso-dependent and denso-independent ecological processes, including potential sources of variation due to sampling methods, all of them shaping the observed spatial pattern of species distribution and diversity.
Because of the large difference in productivity and resources between the zones identified, the size of the isotopic niche was also very variable. Relatively small niches were obtained for the plankton communities samples in the coastal zones, as the availability of feeding resources will allow for specialized feeding on prey of similar isotopic composition. Grazing on phytoplankton is a dominant process in these zones and this is indicated by the composition of the copepod community (Morales et al., 2010). Indeed, our results showed a relatively large homogeneity in composition for the CTZ. In contrast, oligotrophic zones had large isotopic niches, mainly due to a large variability in δ13C, and resulted also more variable in taxonomic composition than the coastal zones. Opportunistic feeding on prey that are scarce (i.e., low plankton biomass) and diverse would cause large variations in the isotopic composition of both prey and consumers, and therefore would produce large isotopic niches. Similar results were found in plankton from deep layers of the Atlantic, where the communities living in tropical, oligotrophic zones showed isotopic niches of large size (Bode and Hernández-León, 2018). From an ecological perspective, it can be suggested that the U-OLIGO-Z, representing a special ecosystem of the South Pacific central gyre, may be less sensitive to a changing environment upon a greater plasticity of species to exploit highly diverse resources.
In spite of the recognition that multiple factors and processes can modulate C and N and their isotopes composition of the zooplankton community, it is relevant to show that a changing community structure and isotopic niche characteristics appear linked to different biogeochemical zones, providing the basis for ecological zonation and revealing that basic processes governing nutrient recycling and availability can modulate the food web at least at lower trophic levels. Lower availability of prey and the prevalence of regenerated or atmospheric nitrogen sources in the open ocean would likely explain these differences in isotopic niches, and that would reflect mostly the opportunistic feeding of zooplankton in oligotrophic regions. The proposed trophic zones identified by both the isotopic and taxonomic approach could serve as a framework for comparison of pelagic food webs across large ocean regions.
Author Contributions
All authors have contributed to the work. CG as leading author organized data and the structure of the MS, RE and AB assisted paper writing and WS analyzed and described oceanographic part.
Funding
This work was funded by grant CIMAR-21 of CONA-Chile and the Millennium Institute of Oceanography (IMO, Grant IC120019). CG work is supported by CONICYT Scholarship No. 21160714.
Conflict of Interest Statement
The authors declare that the research was conducted in the absence of any commercial or financial relationships that could be construed as a potential conflict of interest.
Acknowledgments
We are grateful to D. Toledo for sampling assistance and E. Navarro for helping us with satellite data. L. Cubillos and R. Riquelme-Bugueño helped us with statistical analyses.
References
Agersted, M. D., Bode, A., and Nielsen, T. G. (2014). Trophic position of coexisting krill species: a stable isotope approach. Mar. Ecol. Progr. Ser. 516, 139–151. doi: 10.3354/meps11055
Altabet, M. A., Ryabenko, E., Stramma, L., Wallace, D. W., Frank, M., Grasse, P., et al. (2012). An eddy-stimulated hotspot for fixed nitrogen-loss from the Peru oxygen minimum zone. Biogeosciences 9, 4897–4908. doi: 10.5194/bg-9-4897-2012
Atkinson, D. (1994). Temperature and organism size: a biological law for ectotherms? Adv. Ecol. Res. 25, 1–58. doi: 10.1016/S0065-2504(08)60212-3
Benfield, M. C., Grosjean, P., Culverhouse, P. F., Irigoien, X., Sieracki, M. E., Lopez-Urrutia, A., et al. (2007). RAPID: Research on Automated Plankton Identification. Oceanography 20, 172–187. doi: 10.5670/oceanog.2007.63
Bode, A., and Hernández-León, S. (2018). Trophic diversity of plankton in the epipelagic and mesopelagic layers of the tropical and equatorial atlantic determined with stable isotopes. Diversity 10:48. doi: 10.3390/d10020048
Calbet, A., and Landry, M. R. (1999). Mesozooplankton influences on the microbial food web: direct and indirect trophic interactions in the oligotrophic open ocean. Limnol. Oceanogr. 44, 1370–1380. doi: 10.4319/lo.1999.44.6.1370
Calbet, A., and Saiz, E. (2005). The ciliate-copepod link in marine ecosystems. Aquat. Microb. Ecol. 38, 157–167. doi: 10.3354/ame038157
Cartes, J. E., Huguet, C., Parra, S., and Sánchez, F. (2007). Trophic relationships in deep-water decapods of Le Danois bank (Cantabrian Sea, NE Atlantic): trends related with depth and seasonal changes in food quality and availability. Deep Sea Res. Part I Oceanogr. Res. Papers 54, 1091–1110. doi: 10.1016/j.dsr.2007.04.012
Coplen, T. B. (2011). Guidelines and recommended terms for expression of stable-isotope-ratio and gas-ratio measurement results. Rapid Commun. Mass Spectrom. 25, 2538–2560. doi: 10.1002/rcm.5129
Cornejo, M., Bravo, L., Ramos, M., Pizarro, O., Karstensen, J., Gallegos, M., et al. (2015). Biogeochemical characteristics of a long-lived anticyclonic eddy in the eastern South Pacific Ocean. Biogeosci. Discuss. 12, 2971–2979. doi: 10.5194/bgd-12-14481-2015
Cury, P., Bakun, A., Crawford, R. J., Jarre, A., Quinones, R. A., Shannon, L. J., et al. (2000). Small pelagics in upwelling systems: patterns of interaction and structural changes in “wasp-waist” ecosystems. ICES J. Mar. Sci. 57, 603–618. doi: 10.1006/jmsc.2000.0712
Dalsgaard, T., Thamdrup, B., Farías, L., and Revsbech, N. P. (2012). Anammox and denitrification in the oxygen minimum zone of the Eastern South Pacific. Limnol. Oceanogr. 57, 1331–1346. doi: 10.4319/lo.2012.57.5.1331
Dalsgaard, T., De Brabandere, L., and Hall, P. O. (2013). Denitrification in the water column of the central Baltic Sea. Geochim. Cosmochim. Acta 106, 247–260. doi: 10.1016/j.gca.2012.12.038
Daneri, G., Dellarossa, V., Quiñones, R., Jacob, B., Montero, P., and Ulloa, O. (2000). Primary production and community respiration in the Humboldt Current System off Chile and associated oceanic areas. Mar. Ecol. Prog. Ser. 197, 41–44. doi: 10.3354/meps197041
Del Giorgio, P. A., and Duarte, C. M. (2002). Respiration in the open ocean. Nature 420, 379–384. doi: 10.1038/nature01165
DeNiro, M. J., and Epstein, S. (1978). Carbon isotopic evidence for different feeding patterns in two hyrax species occupying the same habitat. Science 201, 906–908. doi: 10.1126/science.201.4359.906
Dickinson, G. J., and Chakraborti, S. (2003). Nonparametric Statistical Inference, Vol. 168. Abingdon: Taylor & Francis.
Dolan, J. R., Ritchie, M. E., and Ras, J. (2007). The” neutral” community structure of planktonic herbivores, tintinnid ciliates of the microzooplankton, across the SE Tropical Pacific Ocean. Biogeosci. Discuss. 4, 561–593. doi: 10.5194/bgd-4-561-2007
Dugdale, R. C., and Goering, J. J. (1967). Uptake of new and regenerated forms of nitrogen in primary productivity 1. Limnol. Oceanogr. 12, 196–206. doi: 10.4319/lo.1967.12.2.0196
El-Sabaawi, R., Trudel, M., and Mazumder, A. (2013). Zooplankton stable isotopes as integrators of bottom-up variability in coastal margins: a case study from the Strait of Georgia and adjacent coastal regions. Prog. Oceanogr. 115, 76–89. doi: 10.1016/j.pocean.2013.05.010
Escribano, R., Hidalgo, P., and Krautz, C. (2009). Zooplankton associated with the oxygen minimum zone system in the northern upwelling region of Chile during March 2000. Deep Sea Res. Part II 56, 1049–1060. doi: 10.1016/j.dsr2.2008.09.009
Espinasse, B., Harmelin-Vivien, M., Tiano, M., Guilloux, L., and Carlotti, F. (2014). Patterns of variations in C and N stable isotope ratios in size-fractionated zooplankton in the Gulf of Lion, NW Mediterranean Sea. J. Plankton Res. 36, 1204–1215. doi: 10.1016/j.jenvrad.2015.02.019
Fagetti, E., and Fisher, W. (1964). Resultados cuantitativos del zooplancton colectado frente a la costa chilena por la expedición ìMar Chile. Montemar 4, 137–200.
Farías, L., Faúndez, J., Fernández, C., Cornejo, M., Sanhueza, S., and Carrasco, C. (2013). Biological N2O fixation in the Eastern South Pacific Ocean and marine cyanobacterial cultures. PLoS One 8:e63956. doi: 10.1371/journal.pone.0063956
Fernandez, C., Farías, L., and Ulloa, O. (2011). Nitrogen fixation in denitrified marine waters. PLoS One 6:e20539. doi: 10.1371/journal.pone.0020539
Fernández-Castro, B., Mouriño-Carballido, B., Marañón, E., Chouciño, P., Gago, J., Ramírez, T., et al. (2015). Importance of salt fingering for new nitrogen supply in the oligotrophic ocean. Nat. Commun. 6, 8002. doi: 10.1038/ncomms9002
Galán, A., Molina, V., Thamdrup, B., Woebken, D., Lavik, G., Kuypers, M. M., et al. (2009). Anammox bacteria and the anaerobic oxidation of ammonium in the oxygen minimum zone off northern Chile. Deep Sea Res. Part II Top. Stud. Oceanogr. 56, 1021–1031. doi: 10.1016/j.dsr2.2008.09.016
Gruber, N., Keeling, C. D., Bacastow, R. B., Guenther, P. R., Lueker, T. J., Wahlen, M., et al. (1999). Spatiotemporal patterns of carbon-13 in the global surface oceans and the oceanic Suess effect. Glob. Biogeochem. Cycles 13, 307–335. doi: 10.1029/1999GB900019
Harris, R. P., Wiebe, P. H., Lenz, J., et al. (2000). ICES Zooplankton Methodology Manual. New York, NY: Academic Press.
Hernández, C. E., Moreno, R., and Rozbaczylo, N. (2005). Biogeographical patterns and Rapoport’s rule in southeastern Pacific benthic polychaetes of the Chilean coast. Ecography 28, 1–11. doi: 10.1111/j.0906-7590.2005.04013.x
Hirst, A. G., and Bunker, A. J. (2003). Growth of marine planktonic copepods: global rates and patterns in relation to chlorophyll a, temperature, and body weight. Limnol. Oceanogr. 48, 1988–2010. doi: 10.4319/lo.2003.48.5.1988
Hormazabal, S., Shaffer, G., Letelier, J., and Ulloa, O. (2001). Local and remote forcing of sea surface temperature in the coastal upwelling system off Chile. J. Geophys. Res. Oceans 106, 16657–16671. doi: 10.1029/2001JC900008
Hunt, B. P., Allain, V., Menkès, C., Lorrain, A., Graham, B., Rodier, M., et al. (2015). A coupled stable isotope-size spectrum approach to understanding pelagic food-web dynamics: a case study from the Southwest sub-tropical Pacific. Deep Sea Res. Part II Top. Stud. Oceanogr. 113, 208–224. doi: 10.1016/j.dsr2.2014.10.023
Jackson, A. L., Inger, R., Parnell, A. C., and Bearhop, S. (2011). Comparing isotopic niche widths among and within communities: SIBER–Stable Isotope Bayesian Ellipses in R. J. Anim. Ecol. 80, 595–602. doi: 10.1111/j.1365-2656.2011.01806.x
Kleppel, G. S. (1993). On the diets of calanoid copepods. Mar. Ecol. Prog. Ser. 99, 183–183. doi: 10.3354/meps099183
Layman, C. A., Araujo, M. S., Boucek, R., Hammerschlag-Peyer, C. M., Harrison, E., Jud, Z. R., et al. (2012). Applying stable isotopes to examine food-web structure: an overview of analytical tools. Biol. Rev. 87, 545–562. doi: 10.1111/j.1469-185X.2011.00208.x
Layman, C. A., Arrington, D. A., Montaña, C. G., and Post, D. M. (2007). Can stable isotope ratios provide for community-wide measures of trophic structure? Ecology 88, 42–48.
Lee, R. F., Hagen, W., and Kattner, G. (2006). Lipid storage in marine zooplankton. Mar. Ecol. Prog. Ser. 307, 273–306. doi: 10.3354/meps307273
Matthews, B., and Mazumder, A. (2003). Compositional and interlake variability of zooplankton affect baseline stable isotope signatures. Limnol. Oceanogr. 48, 1977–1987. doi: 10.4319/lo.2003.48.5.1977
McClelland, J. W., Holl, C. M., and Montoya, J. P. (2003). Relating low δ15N values of zooplankton to N2-fixation in the tropical North Atlantic: insights provided by stable isotope ratios of amino acids. Deep Sea Res. Part I Oceanogr. Res. Papers 50, 849–861. doi: 10.1016/S0967-0637(03)00073-6
Mompeán, C., Bode, A., Benítez-Barrios, V. M., Domínguez-Yanes, J. F., Escánez, J., and Fraile-Nuez, E. (2013). Spatial patterns of plankton biomass and stable isotopes reflect the influence of the nitrogen-fixer Trichodesmium along the subtropical North Atlantic. J. Plankton Res. 35, 513–525. doi: 10.1093/plankt/fbt011
Mompeán, C., Bode, A., Latasa, M., Fernández-Castro, B., Mouriño-Carballido, B., and Irigoien, X. (2016). The influence of nitrogen inputs on biomass and trophic structure of ocean plankton: a study using biomass and stable isotope size-spectra. J. Plankton Res. 38, 1163–1177. doi: 10.1093/plankt/fbw052
Montoya, J. P., Holl, C. M., Zehr, J. P., Hansen, A., Villareal, T. A., and Capone, D. G. (2004). High rates of N 2 fixation by unicellular diazotrophs in the oligotrophic Pacific Ocean. Nature 430, 1027. doi: 10.1038/nature02824
Morales, C. E., Hormazábal, S. E., and Blanco, J. (1999). Interannual variability in the mesoscale distribution of the depth of the upper boundary of the oxygen minimum layer off northern Chile (18–24S): implications for the pelagic system and biogeochemical cycling. J. Mar. Res. 57, 909–932. doi: 10.1357/002224099321514097
Morales, C. E., Torreblanca, M. L., Hormazabal, S., Correa-Ramírez, M., Nuñez, S., and Hidalgo, P. (2010). Mesoscale structure of copepod assemblages in the coastal transition zone and oceanic waters off central-southern Chile. Prog. Oceanogr. 84, 158–173. doi: 10.1016/j.pocean.2009.12.001
Mouriño-Carballido, B., Graña, R., Fernández, A., Bode, A., Varela, M., Domínguez, J., et al. (2011). Importance of N2 fixation vs. nitrate eddy diffusion along a latitudinal transect in the Atlantic Ocean. Limnol. Oceanogr. 56, 999–1007. doi: 10.4319/lo.2011.56.3.0999
Moutin, T., Doglioli, A. M., Verneil, A. D., and Bonnet, S. (2017). Preface: the oligotrophy to the UlTra-oligotrophy Pacific experiment (OUTPACE cruise, 18 February to 3 April 2015). Biogeosciences 14, 3207–3220. doi: 10.5194/bg-14-3207-2017
Nakatsuka, T., Handa, N., Wada, E., and Wong, C. S. (1992). The dynamic changes of stable isotopic ratios of carbon and nitrogen in suspended and sedimented particulate organic matter during a phytoplankton bloom. J. Mar. Res. 50, 267–296. doi: 10.1357/002224092784797692
Oliver, M. J., and Irwin, A. J. (2008). Objective global ocean biogeographic provinces. Geophys. Res. Lett. 35, 1–6. doi: 10.1029/2008GL034238
Palma, S., and Silva, N. (2006). Epipelagic siphonophore assemblages associated with water masses along a transect between Chile and Easter Island (eastern South Pacific Ocean). J. Plankton Res. 28, 1143–1151. doi: 10.1093/plankt/fbl044
Perry, R. I., Thompson, P. A., Mackas, D. L., Harrison, P. J., and Yelland, D. R. (1999). Stable carbon isotopes as pelagic food web tracers in adjacent shelf and slope regions off British Columbia, Canada. Can. J. Fish. Aquat. Sci. 56, 2477–2486. doi: 10.1139/f99-194
Peterson, B. J., and Fry, B. (1987). Stable isotopes in ecosystem studies. Ann. Rev. Ecol. Syst. 18, 293–320. doi: 10.1146/annurev.es.18.110187.001453
Post, D. M. (2002). Using stable isotopes to estimate trophic position: models, methods, and assumptions. Ecology 83, 703–718. doi: 10.1890/0012-9658(2002)083[0703:USITET]2.0.CO;2
Post, D. M., Layman, C. A., Arrington, D. A., Takimoto, G., Quattrochi, J., and Montana, C. G. (2007). Getting to the fat of the matter: models, methods and assumptions for dealing with lipids in stable isotope analyses. Oecologia 152, 179–189. doi: 10.1007/s00442-006-0630-x
Raimbault, P., and Garcia, N. (2008). Evidence for efficient regenerated production and dinitrogen fixation in nitrogen-deficient waters of the South Pacific Ocean: impact on new and export production estimates. Biogeosciences 5, 323–338. doi: 10.5194/bg-5-323-2008
Reygondeau, G., Longhurst, A., Martinez, E., Beaugrand, G., Antoine, D., and Maury, O. (2013). Dynamic biogeochemical provinces in the global ocean. Glob. Biogeochem. Cycles 27, 1046–1058. doi: 10.1002/gbc.20089
Riquelme-Bugueño, R., Núñez, S., Jorquera, E., Valenzuela, L., Escribano, R., and Hormazábal, S. (2012). The influence of upwelling variation on the spatially-structured euphausiid community off central-southern Chile in 2007–2008. Prog. Oceanogr. 92, 146–165. doi: 10.1016/j.pocean.2011.07.003
Saiz, E., Calbet, A., Atienza, D., and Alcaraz, M. (2007). Feeding and production of zooplankton in the Catalan Sea (NW Mediterranean). Prog. Oceanogr. 74, 313–328. doi: 10.1016/j.pocean.2007.04.004
Seibel, B. A. (2011). Critical oxygen levels and metabolic suppression in oceanic oxygen minimum zones. J. Exp. Biol. 214, 326–336. doi: 10.1242/jeb.049171
Smyntek, P. M., Teece, M. A., Schulz, K. L., and Thackeray, S. J. (2007). A standard protocol for stable isotope analysis of zooplankton in aquatic food web research using mass balance correction models. Limnol. Oceanogr. 52, 2135–2146. doi: 10.4319/lo.2007.52.5.2135
Spalding, M. D., Fox, H. E., Allen, G. R., Davidson, N., Ferdaña, Z. A., Finlayson, M. A. X., et al. (2007). Marine ecoregions of the world: a bioregionalization of coastal and shelf areas. AIBS Bull. 57, 573–583. doi: 10.1641/B570707
Stramma, L., Bange, H. W., Zeschel, R., Lorenzo, A., and Frank, M. (2013). On the role of mesoscale eddies for the biological productivity and biogeochemistry in the eastern tropical Pacific Ocean off Peru. Biogeosciences 10, 7293–7306. doi: 10.5194/bg-10-7293-2013
Thamdrup, B., Dalsgaard, T., Jensen, M. M., Ulloa, O., Farías, L., and Escribano, R. (2006). Anaerobic ammonium oxidation in the oxygen-deficient waters off northern Chile. Limnol. Oceanogr. 51, 2145–2156. doi: 10.4319/lo.2006.51.5.2145
Tibbets, T. M., Wheeless, L. A., and Del Rio, C. M. (2008). Isotopic enrichment without change in diet: an ontogenetic shift in δ15N during insect metamorphosis. Funct. Ecol. 22, 109–113.
Vargas, C. A., Escribano, R., and Poulet, S. (2006). Phytoplankton food quality determines time windows for successful zooplankton reproductive pulses. Ecology 87, 2992–2999. doi: 10.1890/0012-9658(2006)87[2992:PFQDTW]2.0.CO;2
Von Dassow, P., and Collado-Fabbri, S. (2014). Biological oceanography, biogeochemical cycles, and pelagic ecosystem functioning of the east-central South Pacific Gyre: focus on Easter Island and Salas y Gómez Island. Latin Am. J. Aquat. Res. 42, 703–742. doi: 10.3856/vol42-issue4-fulltext-4
Wang, S. W., Budge, S. M., Gradinger, R. R., Iken, K., and Wooller, M. J. (2014). Fatty acid and stable isotope characteristics of sea ice and pelagic particulate organic matter in the Bering Sea: tools for estimating sea ice algal contribution to Arctic food web production. Oecologia 174, 699–712. doi: 10.1007/s00442-013-2832-3
Webb, S. C., Hedges, R. E., and Simpson, S. J. (1998). Diet quality influences the (&dgr) 13C and (& dgr) 15N of locusts and their biochemical components. J. Exp. Biol. 201, 2903–2911.
Williams, P. J. L. (1981). Microbial contribution to overall marine plankton metabolism-direct measurements of respiration. Oceanol. Acta 4, 359–364.
Wollast, R. (1998). Evaluation and comparison of the global carbon cycle in the coastal zone and in the open ocean. Sea 10, 213–252.
Yamada, Y., and Ikeda, T. (1999). Acute toxicity of lowered pH to some oceanic zooplankton. Plankton Biol. Ecol. 46, 62–67.
Keywords: zooplankton, community structure, stable isotopes, carbon, nitrogen, ecoregions
Citation: González CE, Escribano R, Bode A and Schneider W (2019) Zooplankton Taxonomic and Trophic Community Structure Across Biogeochemical Regions in the Eastern South Pacific. Front. Mar. Sci. 5:498. doi: 10.3389/fmars.2018.00498
Received: 13 September 2018; Accepted: 11 December 2018;
Published: 08 January 2019.
Edited by:
Cinzia Corinaldesi, Università Politecnica delle Marche, ItalyReviewed by:
André Ricardo Araújo Lima, Federal University of Pernambuco, BrazilEmanuela Fanelli, Università Politecnica delle Marche, Italy
Copyright © 2019 González, Escribano, Bode and Schneider. This is an open-access article distributed under the terms of the Creative Commons Attribution License (CC BY). The use, distribution or reproduction in other forums is permitted, provided the original author(s) and the copyright owner(s) are credited and that the original publication in this journal is cited, in accordance with accepted academic practice. No use, distribution or reproduction is permitted which does not comply with these terms.
*Correspondence: Carolina E. González, Y2Fyb2xnb256YWxlekB1ZGVjLmNs