- 1National Institute of Water and Atmospheric Research, Hamilton, New Zealand
- 2School of Science, University of Waikato, Hamilton, New Zealand
Seagrass meadows provide a range of important ecosystem functions that can be influenced by anthropogenic pressures. Sediment loading from coastal land use mismanagement can elevate turbidity and reduce seabed light levels, thereby impacting seagrass primary productivity and meadow health. Less understood is the impact of elevated turbidity on the nutrient removal capacity of seagrass, which is a key ecosystem function. Here, we use in situ benthic chambers to show that elevated turbidity is associated with lower nutrient (NH4+) removal within intertidal seagrass meadows. Our results suggest that reductions in sediment loading to improve water clarity may increase the nutrient removal capacity of intertidal seagrass meadows influenced by light limitation. When quantifying important ecosystem functions such as nutrient removal by seagrass it is important to consider this context dependency.
Introduction
Seagrasses are a key component of shallow coastal ecosystems that provide a range of ecosystem functions (Campagne et al., 2015; Mtwana Nordlund et al., 2016). They protect and stabilize coastlines (Christianen et al., 2013), and play a valuable role in the cycling and storage of carbon (Duarte et al., 2013) and nutrients (McGlathery et al., 2007). They also support diverse ecological communities of plants and animals and provide important habitats for fish, birds, and shellfish (Unsworth et al., 2010). There is world-wide concern about the loss of seagrass-associated ecosystem functions due to various anthropogenic stressors (Waycott et al., 2009), including excess nutrients or sediments which are the most common and significant causes of seagrass decline.
Seagrasses are estimated to store between 4.2 and 8.4 Pg carbon (Fourqurean et al., 2012) and denitrify 0.03 Tg N2O-N per year (Murray et al., 2015). Seagrasses take up inorganic nutrients from sediment porewater (via roots and rhizomes) and from the water column (via blades) (van der Heide et al., 2008). The water column is estimated to contribute approximately 50% of nutrients required for seagrass growth and survival (Lee et al., 2007). The relative importance of above and belowground biomass for carbon sequestration and nutrient uptake is highly variable, related to nutrient type, seagrass species, and other environmental factors (La Nafie et al., 2014; Sandoval-Gil et al., 2015).
Seagrass meadows are typically located adjacent to bare “unvegetated” sediments, which are colonized during seagrass expansion. Unvegetated sediments also often replace seagrass habitat when seagrass meadows naturally contract or are lost due to disturbance, stress or disease. However, despite the flat and relatively homogenous appearance of unvegetated sediments, this habitat type can be highly productive due to the presence of microphytobenthic (MPB) communities, comprised of microscopic algae and cyanobacteria living on the surface of sediment (MacIntyre et al., 1996). Seagrass meadows and MPB communities take up inorganic nutrients to support photosynthesis and produce organic biomass (Lee et al., 2007). The organic material is either exported from the system as detritus, buried in the sediment column, or remineralised (Hemminga et al., 1991). Many types of soft-sediment macrofauna bioturbate and irrigate the upper layers of the sediment column, thereby oxygenating sediment, consuming organic matter and excreting inorganic nutrients, which can fuel seagrass and MPB growth and production (Lohrer et al., 2016).
Photosynthetic biomass and primary production are generally significantly higher in seagrass meadows than bare sediments [approximately 2–4 times higher (Pratt et al., 2015; Gustafsson and Norkko, 2016; Lohrer et al., 2016)]. As nutrient uptake rates are related to rates of primary production in bare sediments (Thornton et al., 1999; Dalsgaard, 2003; Longphuirt et al., 2009; Rodil et al., 2011), and primary production is higher in seagrass meadows (Lohrer et al., 2016), it follows that seagrass meadows will have a greater capacity to remove inorganic nutrients than bare unvegetated sediments. In addition, because nutrient uptake rates are generally related to primary production rates, stressors that affect seagrass primary production, such as increased turbidity, may therefore affect the nutrient removal capacity of seagrass. Turbidity in the water column is inversely correlated with seabed light levels, and high turbidity is generally considered a stressor of seagrass (Petrou et al., 2013; Chartrand et al., 2016). By moderating seagrass primary production rates, high turbidity has the potential to affect the growth and vigor of individual plants and the extent and lushness of whole meadows (Goodman et al., 1995; Petrou et al., 2013; Chartrand et al., 2016), which in turn may result in reduced nutrient removal or retention (van der Heide et al., 2008).
We hypothesize that high water column turbidity has a significant effect on the nutrient removal capacity of seagrass, both due to a reduction in the rate of photosynthetically driven uptake, and due to reduced seagrass biomass. Here we tested this hypothesis by measuring nutrient fluxes in seagrass meadows and adjacent bare sediments at three sites spanning a significant turbidity gradient in a New Zealand estuary. The difference in nutrient efflux between a site’s seagrass meadow and its bare sediment was used to determine the nutrient removal capacity of seagrass at each site and how it varied with turbidity and benthic light availability. We chose this approach, rather than a direct comparison of nutrient fluxes in seagrass meadows across a turbidity gradient, to better control for biophysical variation among the three sites (e.g., variation in sediment mud and organic content, the abundance of large macrofaunal species, and MPB concentrations). Within sites, in habitats with and without seagrass that were <5 m apart, the influence of spatial variation in these parameters was reduced, and our ability to make inferences about turbidity’s effects on seagrass nutrient removal capacity was enhanced. As an illustration, the abundance of large bioturbating macrofauna such as bivalves is generally positively related to NH4+ efflux in intertidal systems (Lohrer et al., 2016). If bivalve abundance does not differ between seagrass meadows and adjacent bare sediment at an individual site, we may infer that bivalves are not responsible for the difference in nutrient flux at that site, even though the abundance of bivalves may vary significantly among sites, potentially confounding direct comparisons of nutrient efflux in seagrass beds across sites.
Materials and Methods
This study was conducted at three sites in the Kaipara Harbour, New Zealand: Mairetahi Creek (Mai C; -36.5477S, 174.3609E), Kakaraia Flats (KKF; 36.4188S, 174.3937E), and Tapora Bank (TPB; -36.3938S; 174.2930E). Sites were selected to represent a gradient of high, moderate and low suspended sediment concentration; informed by the output of a sediment transport model for the harbor (Pritchard, NIWA) and confirmed by total suspended solids and turbidity measurements during sampling. Sampling occurred on 3 days over a 4-day period in summer (February 26–29, 2016). The tidal range in the harbor during sampling was ∼2.7 m. Conditions over the 4-day period were typical of conditions expected during summer; 24–25°C air temperature and little or no rainfall during sampling.
Each site was located on an intertidal flat occupied by both seagrass and bare sediment. Sediment-water column fluxes of inorganic nutrients and oxygen were measured using paired light and dark benthic chambers (Figure 1). The methodology used has been described in previous studies (Lohrer et al., 2016). Briefly, a total of five light and dark sets of benthic chambers were deployed at each site within seagrass and adjacent bare sediment habitats. Chambers (50 cm × 50 cm × 15 cm height) were pressed approximately 5 cm into the substrate to enclose 0.25 m2 plots. During high tide these chambers were submerged by ∼1.5–2 m of water. Translucent Perspex domes were fitted to each chamber, sealing in approximately 30 L of water above the enclosed plots during tidal inundation. Dark chambers were covered by shade cloth while light chambers were exposed to ambient sunlight (to capture the impact of photosynthetic processes). A seawater stirrer (Sea Bird Electronics; recirculating pumping for 5 s every 30–40 s), dissolved oxygen logger (ZebraTech D-Opto; sampling at 1-min intervals), and temperature logger (HOBO; sampling every 30 s) were included within each chamber. Chambers were incubated for approximately 4 h during midday high tides. Water samples (60 ml) were drawn from a port in the lid of each chamber at the start, middle, and end of ∼4 h incubations to assess solute concentrations. Water depths were approximately 0.5–1 m at the start, 1.5–2 m at the middle, and 0.5–1 m at the end of sampling. Three light and dark bottles were filled with ambient bottom water and incubated at each site alongside the benthic chambers as a control for potential water column effects (i.e., pelagic processes, such as phytoplankton production) on chamber flux values. Dissolved oxygen (DO) concentrations were measured within each water sample (YSI ProODO Optical Dissolved Oxygen Instrument). The change in DO between the start and the end of an incubation was typically less than 50% (the lowest dark chamber value 49%; the highest light chamber value 138%). Water samples drawn from chambers and bottles were then filtered (0.8 μm glass fiber) and stored frozen in the dark before inorganic nutrient analysis (ammonium nitrogen, nitrate + nitrite nitrogen, dissolved reactive phosphorus). Dissolved inorganic nutrient analysis was performed using standard methods for seawater on an Astoria-Pacific 300 series segmented flow auto-analyzer with detection limits of 1 mg m-3 for N and P.
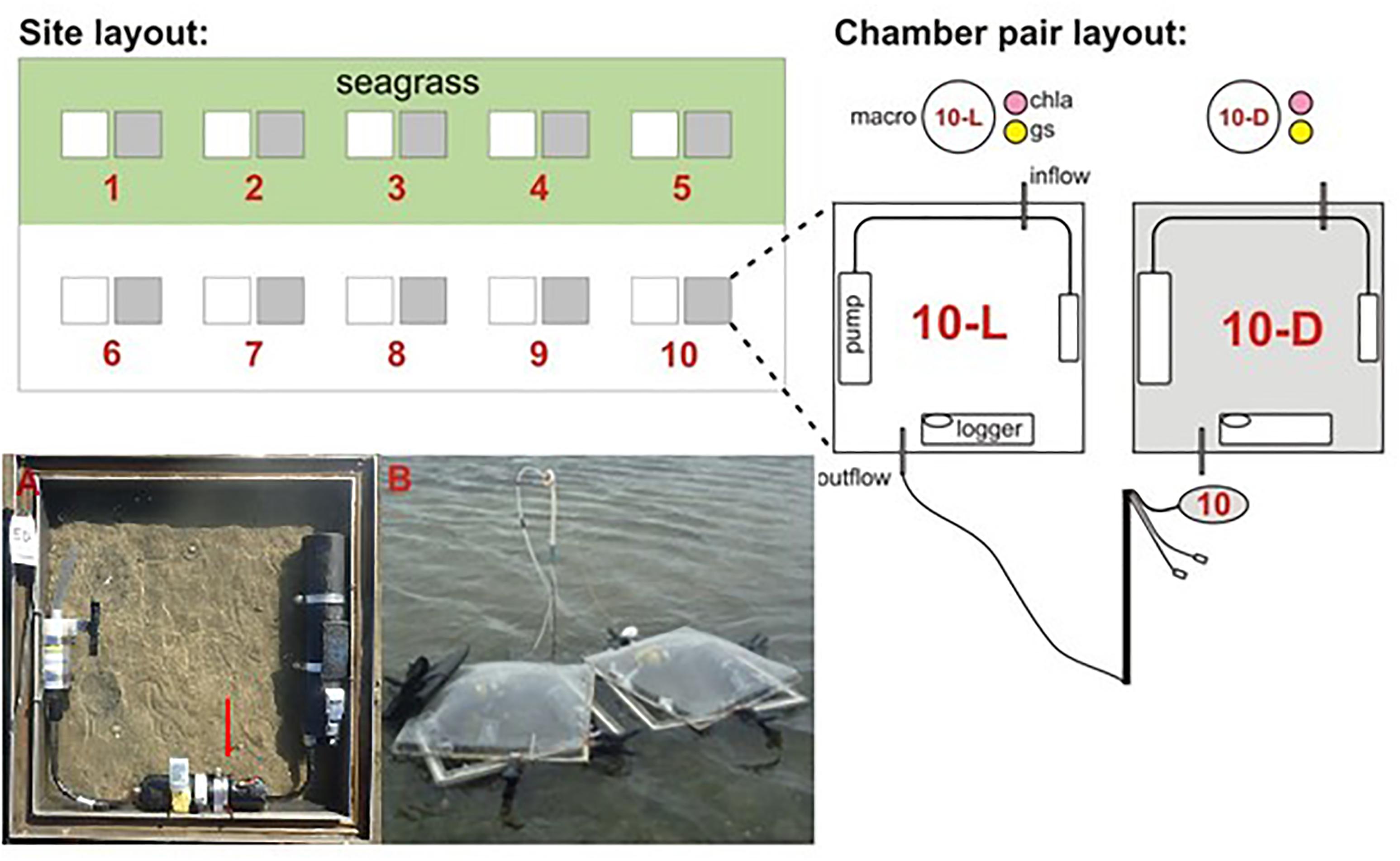
Figure 1. Description of field work site and chamber layout. (A) A light chamber, deployed, with dissolved oxygen logger and water stirring system under a clear Perspex dome, (B) the field site, pre-deployment, just prior to being fully immersed by the incoming tide. Chla, chlorophyll a; gs, grain size; macro, macrofaunal core.
Benthic photosynthetically active radiation (PAR) adjacent to chambers was measured with six light loggers (Odyssey, sampling at 5-min intervals) randomly distributed throughout each site. Water column samples were collected two times during each incubation to measure Total Suspended Solids (TSS). TSS was quantified based on the difference in dry weights of a Whatman glass fiber filter (GF/F, 0.8 μm pore size) before and after passing a set volume of sample water through it (e.g., 500 ml). A logger measuring water column turbidity was also deployed approximately 50 cm below the surface of the water column at each site (measuring turbidity at 1-min intervals). Turbidity values were calibrated based on TSS values to provide an estimate of TSS across the entire incubation period.
At the end of incubations, during low tide, a number of biological and physical variables were sampled. Three small sediment cores (2 cm dia., 2 cm depth) were taken within 50 cm of each plot and amalgamated for sediment mud content [<64 μm (Gatehouse, 1971)], sediment chlorophyll a (Moed and Hallegraeff, 1978), and organic matter content [by loss on ignition at 400°C (Sartory and Grobbelaar, 1984)]. Seagrass blades were avoided during the collection of these samples, even when sampling the seagrass habitats. One larger core (13 cm dia., 15 cm depth) was collected within each plot to quantify macrofaunal community structure and abundance. These samples did include seagrass (both above and below ground biomass) when present. Samples were sieved through a 500 μm mesh and macrofaunal were preserved in 70% isopropyl alcohol for processing in the laboratory. Cockles (Austrovenus stutchburyi) and wedge shells (Macomona liliana) are known to strongly influence sediment-water column fluxes of inorganic nutrients (Pratt et al., 2015; Lohrer et al., 2016) and were the species of interest within macrofaunal cores. Above and below-ground seagrass biomass was extracted from cores and dried in a 60°C oven for 4 days, or until weights stabilized.
Data Analysis
The flux of inorganic nutrients and oxygen in each chamber was calculated according to previously described methods (Lohrer et al., 2016) based on slope coefficients from the time series of concentration measurements collected from each chamber, corrected for chamber surface area and volume. Gross Primary Productivity (GPP) was calculated as light minus dark oxygen flux for each paired light and dark chamber. Flux differences between seagrass and adjacent bare sediments (denoted hereafter by Δ) were calculated by subtraction (seagrass flux minus bare sediment flux). A three factor PERMANOVA test (crossed design) was used to detect significant differences between sites, habitat type and light or dark chamber for inorganic nutrient and oxygen fluxes, and biological and physical characteristics. A two factor PERMANOVA (crossed design) was used to detected significant differences between sites and habitat types for GPP. A one factor PERMANOVA was used to detect significant differences between sites for turbidity, light availability, Δ NH4+ flux, Δ NO3- flux, Δ PO43- flux and Δ GPP. If significant differences were detected (p < 0.05), a pair-wise test was used to isolate differences. As we found no significant difference between light and dark inorganic nutrient fluxes (see Supplementary Table S2), fluxes were pooled for analysis of Δ fluxes. The lack of a significant difference in nutrient flux in seagrass beds in light and dark is consistent with previous observations in New Zealand (Lohrer et al., 2016) and our understanding of seagrass physiology (Touchette and Burkholder, 2000; Alexandre et al., 2016).
Backward multiple regression was used to assess combinations of factors affecting the significant nutrients fluxes obtained in the PERMANOVA analyses (SAS version 9.3; selection criterion α 0.15; final model significance level α 0.05). The initial full model contained the following explanatory variables: seagrass biomass, A. stutchburyi abundance (>5 mm shell width), M. liliana abundance ( >5 mm shell width), sediment organic matter content, sediment chlorophyll a content, sediment mud content, GPP, turbidity, benthic light availability and water temperature. The difference between seagrass and bare sediment (Δ) for each of the explanatory variables was used for ΔNH4+ efflux regressions. Collinearity diagnostics and variance inflation factors were examined, homogeneity of variance was evaluated by plotting residual vs. predicted values, and normality was assessed via normal probability plots to ensure that models met the assumptions of the tests. The relative influence of explanatory variables retained in final models was assessed by examining the significance, sign and magnitude of variable coefficients.
Results
Water column turbidity during sampling was highest at Mai, intermediate at KKF, and lowest at TPB, which corresponded to an inverse pattern in seabed light availability during periods of tidal inundation: lowest at Mai, intermediate at KKF, and highest at TPB (Figure 2A). The average benthic light availability at the two sites exposed to the highest turbidity was below that required for photosynthesis saturation in Zostera muelleri (≥195 μmol m-2 s-1; (Schwarz, 2004); dashed line), while light availability at the site exposed to the lowest turbidity exceeded saturation requirements (Figure 2A). Water temperature varied across sites/chambers by <1.5°C, with slightly lower temperatures at Mai (24.13 ± 0.13°C) than at KKF (25.09 ± 0.13°C) or TPB (25.26 ± 0.25°C), and slightly lower temperatures in dark chambers than in light chambers in seagrass meadows.
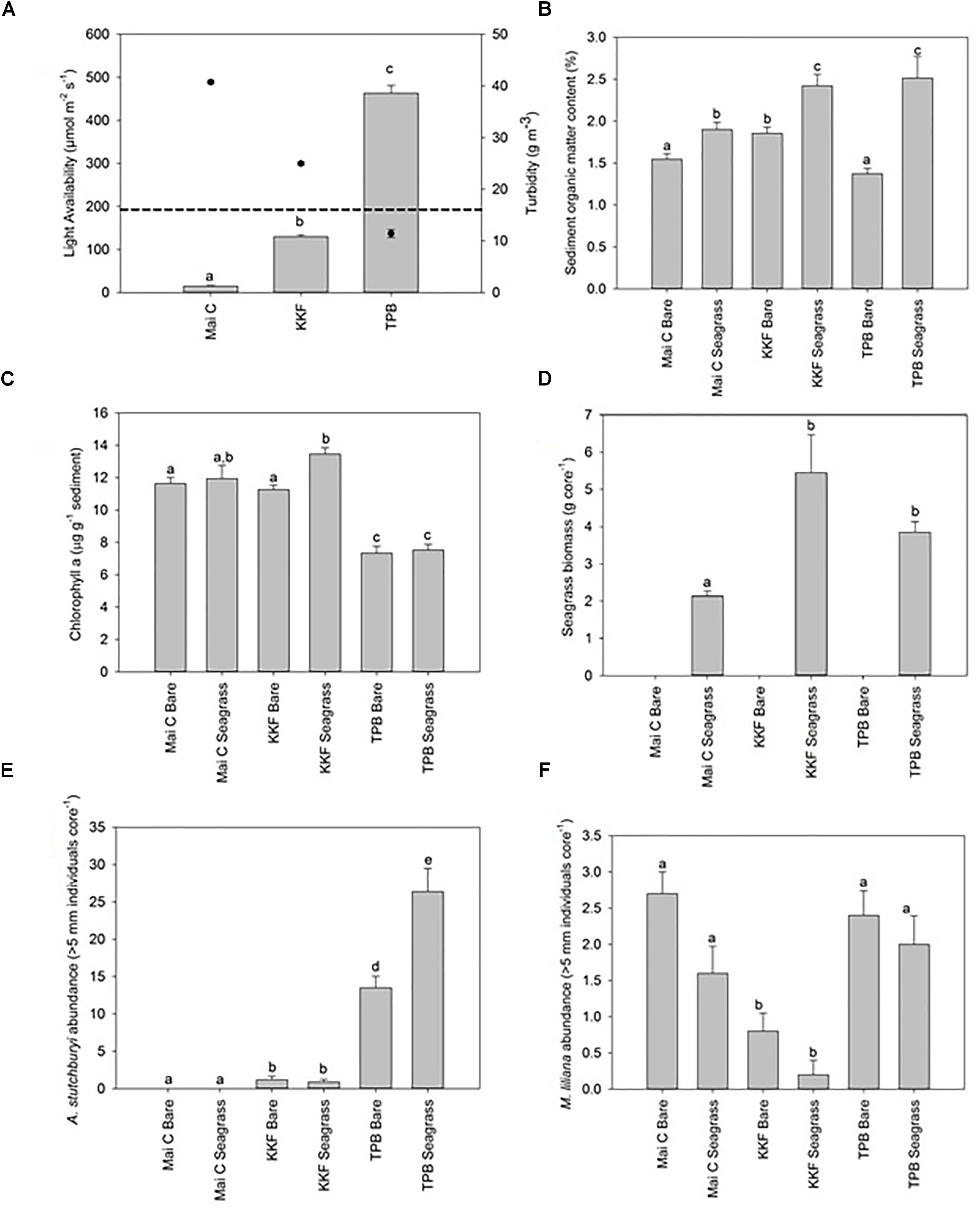
Figure 2. Physical and biological site characteristics ±1 standard error. n = 10 per site/habitat type. (A) Benthic light availability [Dashed line on light availability graph indicates the light required for photosynthesis saturation in Zostera muelleri (≥195 μmol m-2 s-1; Schwarz, 2004)]. Turbidity overlaid onto light availability graph as point data – right hand axis, (B) Sediment organic matter content, (C) Seagrass biomass, (D) Chlorophyll a concentration, (E) Austrovenus stutchburyi abundance, (F) Macomona liliana abundance. a,b,c same letter indicates no significant difference (p > 0.05).
Sediment organic matter content within seagrass meadows was lower at Mai than at KKF or TPB, and was always higher in seagrass meadows than in adjacent bare sediments (Figure 2B). Mud content was higher at Mai (8.57 ± 0.59% within seagrass meadows, 7.67 ± 0.62% within bare sediment) and TPB (9.84 ± 1.67% within seagrass meadows, 9.93 ± 2.0% within bare sediment) than KKF (6.25 ± 0.59% within seagrass meadows, 5.57 ± 0.32% within bare sediment). Sediment chlorophyll a content was lower at TPB than at Mai or KKF within seagrass meadows (Figure 2C).
Although the percent coverage of seagrass shoots at all three sites was 80–100%, seagrass biomass (above plus below ground) was lower at Mai (2.14 ± 0.13) than at KKF (2.80 ± 1.02) or TPB (3.70 ± 0.28) (Figure 2D). Species richness and abundance of macrofauna was highest at TPB, intermediate at KKF and lowest at Mai (Supplementary Table S1). All three sites were dominated numerically by polychaetes and bivalves. In terms of large bioturbating macrofauna (>5 mm in diameter), there was a gradient across sites driven by the abundance of cockles: A. stutchburyi were not observed at Mai C, occurred at low densities at KKF, and were at high densities at TPB (Figure 2E). Another bivalve, the tellinid Macomona liliana, was more abundant at TBP and Mai than it was at KKF (Figure 2F).
Solute Fluxes
Dissolved Oxygen (DO) fluxes from seagrass were positive in the light chambers at TPB (i.e., seagrass habitat was net autotrophic) and negative at KKF and Mai C. DO fluxes from bare sediments for light and dark chambers were negative at all sites (i.e., oxygen demand exceeded oxygen production). Gross primary production, which represents the rate of photosynthetic oxygen production after adjusting for community oxygen respiration (i.e., GPP = light DO flux minus dark DO flux), was higher in seagrass than in bare sediments at KKF and TPB, yet not significantly different at Mai C (Figure 3A). GPP in seagrass and bare sediment was higher at TPB than it was at the other two sites. ΔGPP was greater at TPB than at KKF, and both were greater than at Mai C (Figure 3B).
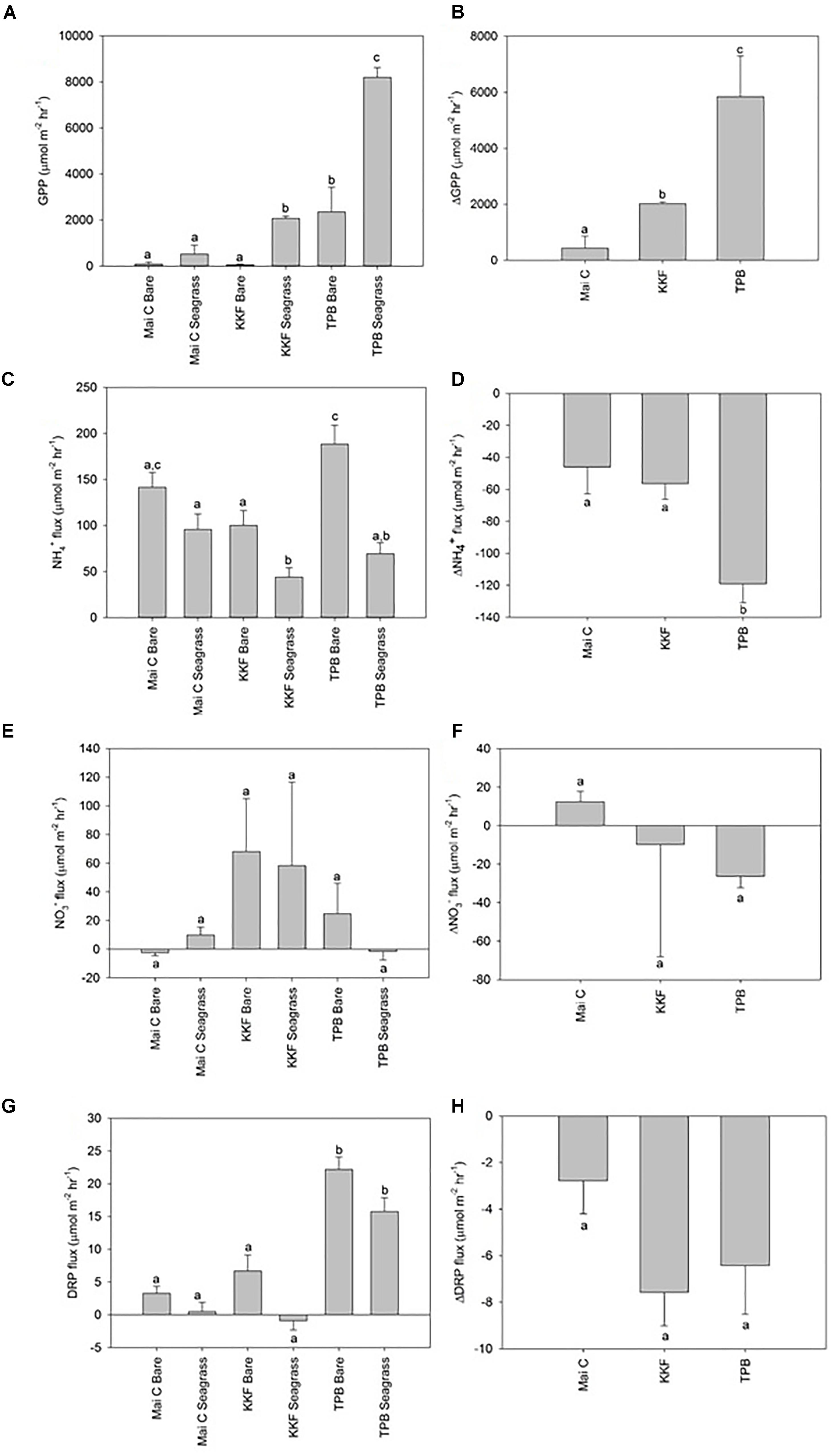
Figure 3. Dissolved oxygen and inorganic nutrient sediment-water column fluxes from seagrass and bare sediment at three sites ±1 standard error. Δ represent seagrass minus adjacent bare sediment fluxes at each site. (A) Gross Primary Production (GPP), (B) ΔGPP, (C) NH4+ flux, (D) ΔNH4+ flux, (E) NO3- flux, (F) ΔNO3- flux, (G) DRP flux, (H) ΔDRP flux. n = 10 per site/habitat type. a,b,c same letter indicates no significant difference (p > 0.05).
Ammonium (NH4+) efflux from seagrass was higher at Mai C than at KKF, yet neither site was significantly different to TPB. Within bare sediment, NH4+ efflux was higher at TPB than KKF. NH4+ efflux from seagrass was lower than adjacent bare sediment at TPB and KKF, however, no significant difference was observed between seagrass and bare sediment at Mai C (Figure 3C). ΔNH4+ between seagrass and adjacent bare sediment was greater at TPB than at Mai C or KKF (Figure 3D). Fluxes of Nitrate (NO3-) were generally lower and more variable than NH4+ fluxes, and no significant patterns were detected across sites or habitats (Figures 3E,F). Dissolved Reactive Phosphorus (DRP) flux was lower at Mai C and KKF than at TPB. Although ΔDRP was lower at Mai C than it was at the other two sites, the differences between the three sites and between habitats at each site were not significant (Figures 3G,H).
Backward regressions were run on NH4+ efflux and ΔNH4+ as NH4+ was the only nutrient which significantly varied in the PERMANOVA analysis (between sites crossed by habitat; Supplementary Table S2). Backward linear regression of the complete dataset (all sites, habitat types, light and dark treatments combined) revealed that seagrass biomass was inversely related to NH4+ efflux (r2 = 0.24, p = <0.001; NH4+ efflux = 131.208 - 12.977 ∗ seagrass biomass) and was the only significant explanatory variable among those entered into the initial full model. In other words, with increasing seagrass biomass, less ammonium moved across the sediment-water interface into the water column. Similarly, nutrient uptake capacity (ΔNH4+ efflux) was inversely correlated with ΔGPP—the gross primary productivity of seagrass relative to its adjacent bare sediment—and was again the only significant predictor of the response (r2 = 0.38, p = 0.01; ΔNH4+ efflux = -52.640 - 0.0141 ∗ ΔGPP).
Refer to Supplementary Table S1 for means ± SE for physical and biological site characteristics and solute fluxes. Refer to Supplementary Table S2 for PERMANOVA results.
Discussion
Our study found NH4+ efflux to be greatest in seagrass exposed to the highest turbidity and no significant difference in nutrient effluxes (NH4+, DRP, and NO3-) between seagrass and bare sediments under these conditions. In contrast, at the site with lowest turbidity and highest ΔGPP, nutrient removal capacity was greatest (significant difference in NH4+ and DRP efflux between seagrass and bare sediment, significant effect for ΔNH4+, consistent patterns for ΔDRP and ΔNO3-). The difference in NH4+ efflux was positively related to ΔGPP, suggesting that seagrass is less effective at intercepting nutrients moving out of the sea bed and into overlying water when turbidity is high and photosynthesis is reduced. Our study suggests that higher turbidity is associated with lower nutrient (NH4+) removal within intertidal seagrass meadows.
Water column turbidity at Mai C, the most turbid of our study sites, was in the upper range of estuarine turbidity values typically observed in New Zealand and overseas (Uncles et al., 2002; Dudley et al., 2017). We note that many of the impacts of turbidity on seagrass nutrient removal are indirect. Water column turbidity impacts seagrass by reducing the quantity and quality of light available for photosynthesis (Petrou et al., 2013; Chartrand et al., 2016). During our experiment, the average benthic light availability at Mai C, and also at KKF, was less than the amount required for photosynthetic saturation in Zostera muelleri [≥195 μmol m-2 s-1; (Schwarz, 2004)], whereas light availability at the site with the clearest water, TPB, exceeded saturation requirements. Increased primary production due to increased light availability is associated with nutrient uptake in MPB communities (Thornton et al., 1999; Dalsgaard, 2003; Longphuirt et al., 2009; Rodil et al., 2011), and results presented here suggest this also occurs in seagrass. Consistent with the literature (van der Heide et al., 2008), our results demonstrate a positive relationship between nutrient uptake rates and seagrass biomass. The site with the lowest nutrient uptake capacity, Mai C, likely had a reduced capacity for nutrient uptake due to a combination of relatively low seagrass biomass and a low potential for photosynthetic activity as a result of high water column turbidity [which in turn is associated with reduced seagrass biomass (Goodman et al., 1995; Petrou et al., 2013; Chartrand et al., 2016)].
As seagrass in this study was intertidal, and was shown to be more productive during tidal emergence at all three sites (Drylie et al., 2018), the access of intertidal seagrass to light during the low tide period (when turbidity is a non-factor) may allow it to persist even at the most highly turbid sites. However, the capacity of seagrass to take up nutrients may be influenced by light climate on a range of time scales. In addition to instantaneous uptake of nutrients to fuel photosynthetic processes (related to light availability and seagrass biomass), the light climate prior to our experiment may have influenced nutrient flux measurements. For instance, seagrass can take up nutrients in the dark by catabolizing starch reserves, however, this capacity is limited to relatively short time-frames (i.e., 8 h) (Touchette and Burkholder, 2000; Alexandre et al., 2016). During periods of high light availability, seagrass may deplete nutrients present in sediment pore-water, thereby reducing the amounts available for exchange with the water column. Low rates of nutrient uptake by seagrass may lead to the accrual of nutrients in sediment porewater, resulting in increased export of nutrients from sediment to water column [as has been observed in MPB communities (Thornton et al., 1999)]. In this study, we assessed fluxes in inundated habitats during the middle part of the day, after the habitats had experienced no or low light for 12–18 h (i.e., night and early morning). The turbidity of the water that inundated the sites as the tide came in likely affected the productivity of the seagrass and its nutrient uptake kinetics. High turbidity prior to the chamber incubations would tend to reduce rates of photosynthesis and starch replenishment and thereby limit nutrient uptake from pore water, consistent with our observation of greater proportions escaping from seabed to water column. The key finding of our study is that the nutrient removal capacity of seagrass – the reduction in NH4+ efflux from sediment to water column during tidal inundation—appears to be affected by high water column turbidity during high tide. Further, even though intertidal seagrass may survive at high turbid sites due to productivity gains around tidal emergence, turbidity still has the potential to limit their value due to a reduction in seagrass-mediated nutrient removal.
Other factors (such as differences in mud, chlorophyll a, organic matter content, bivalve abundance, and temperature) had the potential to influence nutrient fluxes at the study sites, although none were found to be significant in our statistical tests. Moreover, the expected impacts of these factors on nutrient fluxes generally ran counter to what was observed in our study. For example, organic matter content, bivalve abundance and temperature are generally expected to be positively related to sediment NH4+ efflux in intertidal ecosystems (Ziegler and Benner, 1999; Lohrer et al., 2016). The quantity of organic matter available in the sediment for microbial remineralization affects the stocks of NH4+ in pore water (Hemminga et al., 1991); bivalves mix and irrigate sediments and thereby contribute to the release of solutes such as ammonium from pore water to water column (Lohrer et al., 2016); and higher temperatures contribute to higher nutrient fluxes through increases in microbial remineralization rates and macrofaunal activity levels (Bulthuis, 1987). We note that Z. muelleri has a wide thermal optima for maximum photosynthesis, ranging from 16 to 31°C depending on location (Lee et al., 2007; Collier et al., 2017). Thermal optima for maximum photosynthesis have not been established for Z. muelleri in New Zealand. However, for subtropical Z.muelleri an increase of 1°C around the thermal optima for maximum photosynthesis was estimated to result in an increase in GPP of approximately 2.5% (Collier et al., 2017). This suggests a similar difference in temperature between sites/chambers observed during our study is likely to have had a relatively minor impact on fluxes. Therefore, by all accounts, the efflux of ammonium should have been highest in the seagrass beds at TPB. However, NH4+ efflux was relatively low in the seagrass bed at TPB, and was much lower than the level of NH4+ efflux in adjacent unvegetated sediments at TPB. The most parsimonious explanation for this is the higher rates of productivity and nutrient removal by seagrass in the comparatively clear waters of TPB, relative to lower rates of removal in the more turbid waters of KKF and Mai C (Figure 4).
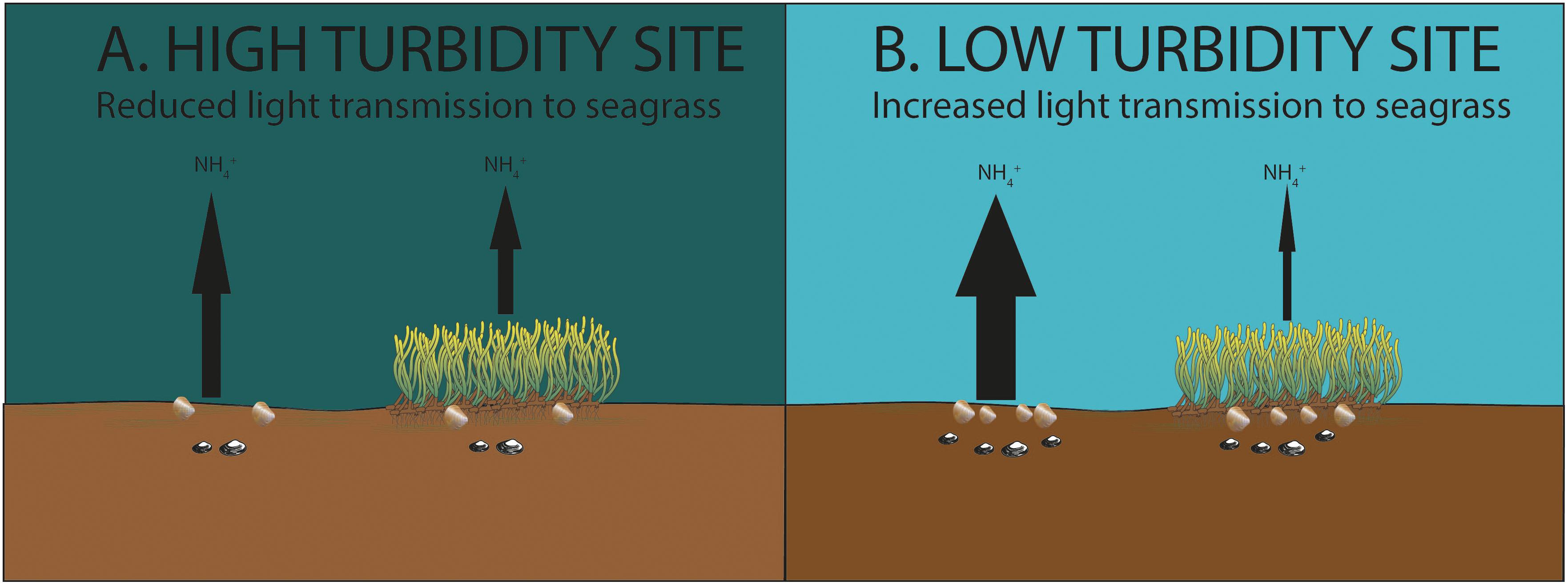
Figure 4. (A) Lower organic matter and bivalve abundance, higher chlorophyll a content (Site Mai C). (B) Higher organic matter and bivalve abundance, lower chlorophyll a content (Site TPB). At bare sediment sites higher sediment-water column efflux of inorganic nutrients (NH4+) was observed due to higher organic matter content, bivalve abundance, and lower chlorophyll a content. Despite these factors, lower inorganic nutrient efflux was observed at the seagrass sites exposed to lower turbidity, indicating higher nutrient removal by seagrass exposed to lower turbidity.
Conclusion
Our results suggest that improvements in water clarity may increase the nutrient removal capacity of intertidal seagrass meadows that experience light limitation. The effect of water column turbidity on seagrass-mediated nutrient removal illustrates how turbidity may impact upon the provisioning of ecosystem functions by seagrass meadows, even before seagrass biomass is lost and meadows contract. When quantifying seagrass ecosystem functions such as nutrient removal, it is important to consider this context dependency.
Author Contributions
RB assisted with conceptual design and led data analysis and write up. AL assisted with conceptual design, fieldwork, data analysis, and write up. MT and TD assisted with fieldwork and write up.
Funding
This research was supported by Ministry of Business Innovation and Employment (MBIE contract no. C01X1005, Management of Cumulative Effects of Stressors on Aquatic Ecosystems) and NIWA (Coasts and Oceans Research Programme 5, SCI 2017/18).
Conflict of Interest Statement
The authors declare that the research was conducted in the absence of any commercial or financial relationships that could be construed as a potential conflict of interest.
Acknowledgments
Many thanks to those at NIWA who contributed to sample processing (macrofaunal sorting and identifications, sediments, water chemistry), including Sarah Hailes, Kelly Carter, Samantha Parkes, Lisa McCartain, Rosalie Carter, Scott Edhouse, and Barry Greenfield. Thanks to Wright and the Integrated Kaipara Harbour Management Group (IKHMG) for granting us access to the sites in Kairpara Harbour, and Luke Connelly (Oruawharo Kaitiaki) and Te Uri o Hau particularly.
Supplementary Material
The Supplementary Material for this article can be found online at: https://www.frontiersin.org/articles/10.3389/fmars.2018.00462/full#supplementary-material
References
Alexandre, A., Silva, J., and Santos, R. (2016). Nitrogen uptake in light versus darkness of the seagrass Zostera noltei: integration with carbon metabolism. Mar. Ecol. 37, 1050–1056. doi: 10.1111/maec.12351
Bulthuis, D. A. (1987). Effects of temperature on photosynthesis and growth of seagrasses. Aquat. Bot. 27, 27–40. doi: 10.1016/0304-3770(87)90084-2
Campagne, C. S., Salles, J.-M., Boissery, P., and Deter, J. (2015). The seagrass Posidonia oceanica: ecosystem services identification and economic evaluation of goods and benefits. Mar. Pollut. Bull. 97, 391–400. doi: 10.1016/j.marpolbul.2015.05.061
Chartrand, K. M., Bryant, C. V., Carter, A. B., Ralph, P. J., and Rasheed, M. A. (2016). Light thresholds to prevent dredging impacts on the great barrier reef seagrass, Zostera muelleri ssp. capricorni. Front. Mar. Sci. 3:106. doi: 10.3389/fmars.2016.00106
Christianen, M. J. A., van Belzen, J., Herman, P. M. J., van Katwijk, M. M., Lamers, L. P. M., van Leent, P. J. M., et al. (2013). Low-canopy seagrass beds still provide important coastal protection services. PLoS One 8:e62413. doi: 10.1371/journal.pone.0062413
Collier, C. J., Ow, Y. X., Langlois, L., Uthicke, S., Johansson, C. L., O’Brien, K. R., et al. (2017). Optimum temperatures for net primary productivity of three tropical seagrass species. Front. Plant Sci. 8:1446. doi: 10.3389/fpls.2017.01446
Dalsgaard, T. (2003). Benthic primary production and nutrient cycling in sediments with benthic microalgae and transient accumulation of macroalgae. Limnol. Oceanogr. 48, 2138–2150. doi: 10.4319/lo.2003.48.6.2138
Drylie, T. P., Lohrer, A. M., Needham, H. R., Bulmer, R. H., and Pilditch, C. A. (2018). Benthic primary production in emerged intertidal habitats provides resilience to high water column turbidity. J. Sea Res. 142, 101–112. doi: 10.1016/j.seares.2018.09.015
Duarte, C. M., Losada, I. J., Hendriks, I. E., Mazarrasa, I., and Marbà, N. (2013). The role of coastal plant communities for climate change mitigation and adaptation. Nat. Clim. Chang. 3, 961–968. doi: 10.1038/nclimate1970
Dudley, B., Zeldis, J., and Burge, O. (2017). “New Zealand Coastal Water Quality Assessment”: Prepared for Ministry for the Environment. NIWA Client Report No. 2016093CH. Auckland: NIWA.
Fourqurean, J. W., Duarte, C. M., Kennedy, H., Marba, N., Holmer, M., Mateo, M. A., et al. (2012). Seagrass ecosystems as a globally significant carbon stock. Nat. Geosci. 5, 505–509. doi: 10.1038/ngeo1477
Gatehouse, J. (1971). “Sedimentary analysis,” in Procedures in Sedimentology and Petrology, ed. R. E. Carver (New York, NY: Wiley Interscience),59–94.
Goodman, J. L., Moore, K. A., and Dennison, W. C. (1995). Photosynthetic responses of eelgrass (Zostera marina L.) to light and sediment sulfide in a shallow barrier island lagoon. Aquat. Bot. 50, 37–47. doi: 10.1016/0304-3770(94)00444-Q
Gustafsson, C., and Norkko, A. (2016). Not all plants are the same: exploring metabolism and nitrogen fluxes in a benthic community composed of different aquatic plant species. Limnol. Oceanogr. 61, 1787–1799. doi: 10.1002/lno.10334
Hemminga, M. A., Harrison, P. G., and van Lent, F. (1991). The balance of nutrient losses and gains in seagrass meadows. Mar. Ecol. Prog. Ser. 71, 85–96. doi: 10.3354/meps071085
La Nafie, Y. A., Van Engeland, T., van Katwijk, M. M., and Bouma, T. J. (2014). Uptake of nitrogen from compound pools by the seagrass Zostera noltii. J. Exp. Mar. Biol. Ecol. 460, 47–52. doi: 10.1016/j.jembe.2014.06.007
Lee, K.-S., Park, S. R., and Kim, Y. K. (2007). Effects of irradiance, temperature, and nutrients on growth dynamics of seagrasses: a review. J. Exp. Mar. Biol. Ecol. 350, 144–175. doi: 10.1016/j.jembe.2007.06.016
Lohrer, A. M., Townsend, M., Hailes, S. F., Rodil, I. F., Cartner, K., Pratt, D. R., et al. (2016). Influence of New Zealand cockles (Austrovenus stutchburyi) on primary productivity in sandflat-seagrass (Zostera muelleri) ecotones. Estuar. Coast. Shelf Sci. 181, 238–248. doi: 10.1016/j.ecss.2016.08.045
Longphuirt, S. N., Lim, J. H., Leynaert, A., Claquin, P., Choy, E. J., Kang, C. K., et al. (2009). Dissolved inorganic nitrogen uptake by intertidal microphytobenthos: nutrient concentrations, light availability and migration. Mar. Ecol. Prog. Ser. 379, 33–44. doi: 10.3354/meps07852
MacIntyre, H. L., Geider, R. J., and Miller, D. C. (1996). Microphytobenthos: the ecological role of the “secret garden” of unvegetated, shallow-water marine habitats. I. Distribution, abundance and primary production. Estuaries 19, 186–201. doi: 10.2307/1352224
McGlathery, K. J., Sundback, K., and Anderson, I. C. (2007). Eutrophication in shallow coastal bays and lagoons: the role of plants in the coastal filter. Mar. Ecol. Prog. Ser. 348, 1–18. doi: 10.3354/meps07132
Moed, J. R., and Hallegraeff, G. M. (1978). Some problems in the estimation of chlorophyll-a and phaeopigments from pre- and post-acidification spectrophotometrie measurements. Int. Rev. Ges. Hydrobiol. 63, 787–800. doi: 10.1002/iroh.19780630610
Mtwana Nordlund, L., Koch, E. W., Barbier, E. B., and Creed, J. C. (2016). Seagrass ecosystem services and their variability across genera and geographical regions. PLoS One 11:e0163091. doi: 10.1371/journal.pone.0163091
Murray, R. H., Erler, D. V., and Eyre, B. D. (2015). Nitrous oxide fluxes in estuarine environments: response to global change. Glob. Chang. Biol. 21, 3219–3245. doi: 10.1111/gcb.12923
Petrou, K., Jimenez-Denness, I., Chartrand, K., McCormack, C., Rasheed, M., and Ralph, P. J. (2013). Seasonal heterogeneity in the photophysiological response to air exposure in two tropical intertidal seagrass species. Mar. Ecol. Prog. Ser. 482, 93–106. doi: 10.3354/meps10229
Pratt, D. R., Lohrer, A. M., Thrush, S. F., Hewitt, J. E., Townsend, M., Cartner, K., et al. (2015). Detecting subtle shifts in ecosystem functioning in a dynamic estuarine environment. PLoS One 10:e0133914. doi: 10.1371/journal.pone.0133914
Rodil, I. F., Lohrer, A. M., Chiaroni, L. D., Hewitt, J. E., and Thrush, S. F. (2011). Disturbance of sandflats by thin terrigenous sediment deposits: consequences for primary production and nutrient cycling. Ecol. Appl. 21, 416–426. doi: 10.1890/09-1845.1
Sandoval-Gil, J. M., Camacho-Ibar, V. F., del Carmen Ávila-López, M., Hernández-López, J., Zertuche-González, J. A., and Cabello-Pasini, A. (2015). Dissolved inorganic nitrogen uptake kinetics and δ15N of Zostera marina L. (eelgrass) in a coastal lagoon with oyster aquaculture and upwelling influence. J. Exp. Mar. Biol. Ecol. 472, 1–13. doi: 10.1016/j.jembe.2015.06.018
Sartory, D. P., and Grobbelaar, J. U. (1984). Extraction of chlorophyll a from freshwater phytoplankton for spectrophotometric analysis. Hydrobiologia 114, 177–187. doi: 10.1007/bf00031869
Schwarz, A. M. (2004). Contribution of photosynthetic gains during tidal emersion to production of Zostera capricorni in a North Island, New Zealand estuary. New Zeal. J. Mar. Freshw. Res. 38, 809–818. doi: 10.1080/00288330.2004.9517280
Thornton, D. C. O., Underwood, G. J. C., and Nedwell, D. B. (1999). Effect of illumination and emersion period on the exchange of ammonium across the estuarine sediment-water interface. Mar. Ecol. Prog. Ser. 184, 11–20. doi: 10.3354/meps184011
Touchette, B. W., and Burkholder, J. M. (2000). Review of nitrogen and phosphorus metabolism in seagrasses. J. Exp. Mar. Biol. Ecol. 250, 133–167. doi: 10.1016/S0022-0981(00)00195-7
Uncles, R. J., Stephens, J. A., and Smith, R. E. (2002). The dependence of estuarine turbidity on tidal intrusion length, tidal range and residence time. Cont. Shelf Res. 22, 1835–1856. doi: 10.1016/S0278-4343(02)00041-9
Unsworth, R. K. F., Cullen, L. C., Pretty, J. N., Smith, D. J., and Bell, J. J. (2010). Economic and subsistence values of the standing stocks of seagrass fisheries: potential benefits of no-fishing marine protected area management. Ocean Coast. Manag. 53, 218–224. doi: 10.1016/j.ocecoaman.2010.04.002
van der Heide, T., Smolders, A. J. P., Rijkens, B. G. A., van Nes, E. H., van Katwijk, M. M., and Roelofs, J. G. M. (2008). Toxicity of reduced nitrogen in eelgrass (Zostera marina) is highly dependent on shoot density and pH. Oecologia 158, 411–419. doi: 10.1007/s00442-008-1155-2
Waycott, M., Duarte, C. M., Carruthers, T. J. B., Orth, R. J., Dennison, W. C., Olyarnik, S., et al. (2009). Accelerating loss of seagrasses across the globe threatens coastal ecosystems. Proc. Natl. Acad. Sci. U.S.A. 106, 12377–12381. doi: 10.1073/pnas.0905620106
Keywords: seagrass, nutrients, turbidity, flux chamber, primary production, microphytobenthos, light availability
Citation: Bulmer RH, Townsend M, Drylie T and Lohrer AM (2018) Elevated Turbidity and the Nutrient Removal Capacity of Seagrass. Front. Mar. Sci. 5:462. doi: 10.3389/fmars.2018.00462
Received: 07 June 2018; Accepted: 16 November 2018;
Published: 10 December 2018.
Edited by:
Christian Grenz, UMR7294 Institut Méditerranéen d’Océanographie (MIO), FranceReviewed by:
Marina Dolbeth, Centro Interdisciplinar de Investigação Marinha e Ambiental (CIIMAR), PortugalW. Judson Kenworthy, Independent Researcher, Beaufort, United States
Copyright © 2018 Bulmer, Townsend, Drylie and Lohrer. This is an open-access article distributed under the terms of the Creative Commons Attribution License (CC BY). The use, distribution or reproduction in other forums is permitted, provided the original author(s) and the copyright owner(s) are credited and that the original publication in this journal is cited, in accordance with accepted academic practice. No use, distribution or reproduction is permitted which does not comply with these terms.
*Correspondence: Richard Hugh Bulmer, richard.bulmer@niwa.co.nz