- 1College of Science and Engineering, James Cook University, Townsville, QLD, Australia
- 2ARC Centre of Excellence for Coral Reef Studies, James Cook University, Townsville, QLD, Australia
Recent studies demonstrate that diel CO2 cycles, such as those prevalent in many shallow water habitats, can potentially modify the effects of ocean acidification conditions on marine organisms. However, whether the interaction between elevated CO2 and diel CO2 cycles is further modified by elevated temperature is unknown. To test this, we reared juvenile spiny damselfish, Acanthochromis polyacanthus, for 11 weeks in two stable (450 and 1000 μatm) and two diel- cycling elevated CO2 treatments (1000 ± 300 and 1000 ± 500 μatm) at both current-day (29°C) and projected future temperature (31°C). We measured the effects on survivorship, growth, behavioral lateralization, activity, boldness and escape performance (fast starts). A significant interaction between CO2 and temperature was only detected for survivorship. Survival was lower in the two cycling CO2 treatments at 31°C compared with 29°C but did not differ between temperatures in the two stable CO2 treatments. In other traits we observed independent effects of elevated CO2, and interactions between elevated CO2 and diel CO2 cycles, but these effects were not influenced by temperature. There was a trend toward decreased growth in fish reared under stable elevated CO2 that was counteracted by diel CO2 cycles, with fish reared under cycling CO2 being significantly larger than fish reared under stable elevated CO2. Diel CO2 cycles also mediated the negative effect of elevated CO2 on behavioral lateralization, as previously reported. Routine activity was reduced in the 1000 ± 500 μatm CO2 treatment compared to control fish. In contrast, neither boldness nor fast-starts were affected by any of the CO2 treatments. Elevated temperature had significant independent effects on growth, routine activity and fast start performance. Our results demonstrate that diel CO2 cycles can significantly modify the growth and behavioral responses of fish under elevated CO2 and that these effects are not altered by elevated temperature, at least in this species. Our findings add to a growing body of work that highlights the critical importance of incorporating natural CO2 variability in ocean acidification experiments to more accurately assess the effects of ocean climate change on marine ecosystems.
Introduction
Increasing atmospheric carbon dioxide (CO2) levels are driving ocean warming (OW) and a decline in seawater pH, a process referred to as ocean acidification (OA) (Collins et al., 2013). Both OA and OW are expected to significantly impact the performance of marine organisms, with severe consequences for community dynamics and ecosystem functioning (Schiel et al., 2004; Doney et al., 2012; Kroeker et al., 2012; Cheung et al., 2013; Wittmann and Pörtner, 2013; Nagelkerken and Connell, 2015). However, while there has been extensive research on the effects of OA and OW in isolation, comparatively less in known about their combined effects. Understating how multiple climate change drivers affect marine organisms is vital to accurately predict future impacts (Riebesell and Gattuso, 2015).
To date, most OA experiments have tested shallow water coastal species, yet have been conducted using stable elevated CO2 levels consistent with open ocean projections (McElhany and Busch, 2013; Wahl et al., 2016). Unlike the open ocean, CO2 levels in shallow water habitats are not in equilibrium with the atmosphere over short time scales, with many habitats experiencing substantial fluctuations in CO2 on a daily basis (Hofmann et al., 2011; Shaw et al., 2012; Duarte et al., 2013; Baumann et al., 2015; Challener et al., 2016). These diel CO2 cycles are driven primarily by the processes of photosynthesis and respiration across a day–night cycle, but are also influenced by a variety of local hydrodynamic factors (Falter et al., 2013; Waldbusser and Salisbury, 2014). Importantly, a number of studies have demonstrated that diel CO2 cycles can significantly modify organismal responses to OA, highlighting the critical importance or incorporating natural CO2 variability in OA experiments (e.g., Cornwall et al., 2013; Frieder et al., 2014; Ou et al., 2015; Jarrold et al., 2017; Enochs et al., 2018; Wahl et al., 2018). However, it is currently unknown if the interaction between elevated CO2 and diel CO2 cycles will be modified by elevated temperature.
Marine fish were initially expected to be resilient to OA as they can tightly defend their internal pH under elevated CO2 conditions via active control of acid-base relevant ions (Wood et al., 1990; Baker et al., 2009; Heuer and Grosell, 2014). However, the increased energetic costs associated with this compensatory acid-base regulation could have detrimental effects on growth and survival, particularly in early life stages where the cost of homeostasis is greater (Brauner, 2009). While some studies have indeed reported negative effects of OA on growth and survival in the early life stages of marine fish (e.g., Miller et al., 2012; Murray et al., 2014; Stiasny et al., 2016; Gobler et al., 2018), others have observed neutral (e.g., Munday et al., 2011; Bignami et al., 2013; Frommel et al., 2013; Watson et al., 2018) and even positive effects (e.g., Munday et al., 2009b; Cattano et al., 2017). To date, only two studies have investigated how diel CO2 cycles will interact with elevated CO2 levels to affect the survival and growth of marine fishes under OA conditions. Diel CO2 cycles (450–2000 μatm) were shown to alleviate the negative effects of elevated CO2 (1000 μatm) on growth of larval pink salmon, Oncorhynchus gorbuscha (Ou et al., 2015). In contrast, diel CO2 cycles (1000 ± 300 and 500 μatm) had no significant effect on growth and survival in two species of coral reef fish (Jarrold and Munday, 2018).
Some of the most notable effects of elevated CO2 on marine fish have been the impacts on ecologically important behaviors (Nagelkerken and Munday, 2016; Cattano et al., 2018). A wide array of behavioral responses, across a range of species, have now been shown to be altered under elevated CO2, including: predator avoidance/prey detection behavior (e.g., Pistevos et al., 2015; Sundin and Jutfelt, 2015; Munday et al., 2016; McMahon et al., 2018) escape responses (e.g., Allan et al., 2013; Munday et al., 2016), activity/boldness levels (e.g., Jutfelt et al., 2013; Hamilton et al., 2014; Munday et al., 2014) and lateralization (e.g., Jutfelt et al., 2013; Welch et al., 2014; Lopes et al., 2016; Schmidt et al., 2017). Nevertheless, species/trait-specific responses are also evident, with other studies reporting no impacts of elevated CO2 on fish behavior (e.g., Jutfelt and Hedgärde, 2013; Heinrich et al., 2016; Cattano et al., 2017; Schmidt et al., 2017; Sundin et al., 2017; Laubenstein et al., 2018). The changes in behavioral responses under elevated CO2 observed in laboratory experiments are expected to have significant ecological consequences for fish populations through effects on recruitment, dispersal, predator-prey/competitive interactions and habitat preference (Nagelkerken and Munday, 2016), although such effects may also be offset by compensatory and indirect effects in more diverse communities (Munday et al., 2014; Goldenberg et al., 2018). Only two studies have investigated how diel CO2 cycles will interact with elevated CO2 levels to affect the behavioral performance of marine fishes under OA conditions. Behavioral impairments in two species of coral reef fish under elevated CO2 were less serve, or absent, in the presence of diel-cycling CO2 regime (1000 ± 300 and 500 μatm) (Jarrold et al., 2017). In contrast, diel CO2 cycles (587–1066 μatm) had no significant effect on behavioral responses of juvenile blacksmith, Chromis punctipinnis (Kwan et al., 2017).
In contrast to elevated CO2, the effects of elevated temperature on the growth and survival of marine fish are more consistent and predictable. Increases in temperature can result in increased growth if the species is living below its thermal optimum (Green and Fisher, 2004; McLeod et al., 2015; Moyano et al., 2016; Gobler et al., 2018). By contrast, growth and survivorship are often reduced at temperatures above the thermal optimum, as metabolic demands exceed capacity (Pörtner and Knust, 2007; Munday et al., 2008; Todd et al., 2008; Neuheimer et al., 2011). Elevated temperatures have also been shown to impact a range of behavioral responses, in some cases having stronger effects than elevated CO2, particularly behaviors which are closely linked to physiological condition such as routine activity and escape performance (Biro et al., 2010; Allan et al., 2017; Schmidt et al., 2017; Laubenstein et al., 2018; Watson et al., 2018).
Considerably less is known about how OA and OW will interact to affect the performance of marine fish. However, a few studies have demonstrated that responses to one stressor can be modified by the presence of another. For example, the positive effects of elevated temperature on growth of larval flat fish, Solea senegalensis, were reduced when CO2 was also elevated (Pimentel et al., 2014). Furthermore, while elevated temperature was shown to reduce growth and survival in larval Atlantic herring, Clupea harengus, the effects of elevated CO2 were shown to be temperature dependent (Sswat et al., 2018; see also Gobler et al., 2018). From a behavioral perspective, elevated CO2 and temperature interacted synergistically on predation rate, but antagonistically on predator selectivity of the dottyback, Pseudochromis fuscus (Ferrari et al., 2015). Furthermore, elevated temperature reduced the effects of elevated CO2 on relative laterization in the damselfish Pomacentrus wardi (Domenici et al., 2014). Collectively, these studies highlight the importance of studying the combined effects of OA and OW if we are to accurately predict the impacts of climate change on marine fish and the associated ecological consequences. It is currently unknown how elevated temperature will modify the interaction between elevated CO2 and diel CO2 cycles to affect the performance of marine fishes.
Here, we investigated the effects of elevated CO2, diel CO2 cycles and elevated temperature on the survival, growth and behavior of a coral reef fish, the spiny damselfish, Acanthochromis polyacanthus. Diel CO2 cycles in some shallow coral reef habitats have been shown to range up to ±600 μatm around the mean (Shaw et al., 2012), although smaller ranges (<±200 μatm) are more common (Kayanne et al., 1995; Manzello, 2010; Albright et al., 2013; Kline et al., 2015). Importantly, diel CO2 cycles on coral reefs are predicted to be amplified up to threefold over the next century (Shaw et al., 2013), and thus cycles with greater magnitude may become more common by the year 2100. To test if elevated temperature alters the effects of elevated CO2 and diel CO2 cycles on reef fishes, we reared juvenile damselfish for 11 weeks at control (450 μatm), stable elevated (1000 μatm) and two diel-cycling elevated (1000 ± 300 μatm and 1000 ± 500 μatm) CO2 treatments at both 29°C (current-day average summer temperature) and 31°C (year 2100 prediction; Collins et al., 2013) (Table 1 and Supplementary Figure 1). We compared survivorship and growth among treatments. We also tested behavioral lateralization, routine activity, boldness and escape performance (i.e., fast starts) of fish from each treatment, as past research has shown that these traits can be affected by both elevated CO2 and temperature.
Materials and Methods
Study Species and Brood-Stock Maintenance
Acanthochromis polyacanthus is common on coral reefs in the Indo-west Pacific. They are demersal spawners, laying clutches of eggs within small caves and crevices in the reef matrix. Eggs hatch into small juveniles, with both parents providing care to the eggs and offspring for up to 45 days post-hatching (Kavanagh, 2000). A. polyacanthus can be bred and reared in captivity with high success, which has led to their establishment as a model for investigating the potential impacts of OA and OW on coral reef fishes (Munday et al., 2008, 2011; Donelson et al., 2012; Welch et al., 2014; Heuer et al., 2016; Jarrold et al., 2017).
Adult A. polyacanthus were collected using hand nets from the Bramble Reef area (site 1: 18°22′S, 146°40′E; site 2: 18°25′S, 146°40′E) of the Great Barrier Reef in July 2015. Fish were transported to an environmentally controlled aquarium research facility at James Cook University (JCU) (Townsville, QLD, Australia) where they were housed as breeding pairs in 60 L aquaria at temperature conditions matching the collection location. Breeding pairs were maintained under stable, ambient CO2 (∼490 μatm). Temperatures were increased at a rate of 0.5°C per week until the summer breeding temperature of 29°C was reached in the first week of December 2016. Breeding pairs were provided with half a terracotta pot to act as a shelter and spawning site. Aquaria were checked each morning for the presence of newly laid clutches of eggs. Pairs were fed ad libitum on commercial fish feed pellets (INVE Aquaculture Nutrition NRD 12/20) once daily outside the breeding season and twice daily during the breeding season.
Experimental Design
The experimental part of the study was carried out at the National Sea Simulator (SeaSim) facility at the Australian Institute of Marine Science (AIMS) (Cape Cleveland, QLD, Australia). Clutches of juveniles were transferred from JCU to the experimental system at AIMS at 1 day post hatch (dph) where they were reared for 11 weeks at two stable (450 and 1000 μatm) and two cycling (1000 ± 300 and 1000 ± 500 μatm) CO2 treatments at both 29 and 31°C. A total of four offspring clutches were used in the experiment, sourced from two breeding pairs (two clutches per pair). Each clutch was split between the CO2 and temperature treatments in duplicate tanks (15–25 fish per tank with tanks on different systems). Juvenile A. polyacanthus were fed 6mL of freshly hatched Artemia naupli (∼4000 mL-1) for the first 4 days post hatch (dph). From 5 to 28 dph they were fed daily (0.12 g) on a weaning fish feed (INVE Aquaculture Nutrition Wean-S 200–400 μm) and from 29 to 42 dph they were fed daily (0.15 g) on a small pellet fish feed (INVE Aquaculture Nutrition NRD 5/8). Feeding was increased to 0.2 g after 42 dph and to 0.35 g after 70 dph.
Fast start trials were performed 28–35 dph, lateralization trials were performed 42–49 dph and activity/boldness trials were performed 63–70 dph. Fast start and lateralization trials were only performed on three clutches due to logistic constraints. Behavioral trials took place across four consecutive days with the temperature treatment tested alternating between days. This allowed the room temperature to be adjusted accordingly, thus ensuring a stable testing temperature was maintained. Fish were randomly selected for each behavioral trial, which means that some fish were likely tested for more than one behavioral trial, and the time between trials was to ensure that they recovered from any stress between trails. To ensure a fish was not tested twice within a trial, fish were placed in an isolation chamber in their experimental tank after testing for the rest of the day. All behavioral trials were performed between 09:00 and 17:00. Fish were gently transferred to the behavioral arenas using a glass beaker to minimize handling stress. Fish from each CO2 treatment were tested at random times throughout the day to account for any possible time of day effects in the fluctuating treatments. At the end of the rearing period all fish were euthanised with clove oil anesthetic. Each fish was blotted dry, weighed (nearest mg) on an analytical balance (AX224, Sartorius, Bradford, PA, United States) and photographed in a lateral position next to a ruler. Standard length (SL) to the nearest 0.1 mm was estimated for each fish from the digital photographs using ImageJ software1. Research was carried out under approval of the James Cook University animal ethics committee (permit: A2210) and according to the University’s animal ethics guidelines.
Experimental System
The experimental setup used at SeaSim comprised of 16 independent flow-through systems (4 systems per CO2 treatment). Each system supplied ultra-filtered seawater (0.04 μm), at either 29 or 31°C, to three custom-made 50 L experimental tanks at the rate of 50 L h-1. Thus, there was total of 48 tanks (6 tanks per CO2/temp treatment). The experimental tanks were placed in individual temperature-controlled water baths to ensure temperature stability (±0.1°C). Treatments and tank replicates were randomly positioned in the experimental room. Supplementary Figure 2 shows a schematic diagram of an individual system.
pCO2 and temperature levels were controlled through a custom designed Model Predictive Control running on a micro-programmable logic controller (PLC) (Series S7-1500, Siemens, Australia). The micro-PLC was integrated with the general SeaSim control system, to provided SCADA (Siemens WinCC) accessibility and data archiving. The CO2 feedback for each of the replication lines was provided via non-dispersive infrared measurements. On each system, water from one tank was delivered to an equilibrator (Seasim, AIMS design, custom built) via an in-tank submersible pump (Universal Pump 1260, EHEIM, Deizisau, Germany) where the air space in the chamber maintains an equilibrium with the CO2 of the experimental water. The air was constantly delivered to a non-dispersive infrared CO2 analyzer (Telaire T6613, Amphenol, Australia) that provided live feedback to the PLC. The CO2 analyzers were calibrated monthly using certified calibration gas mixtures at 0, 600, and 2000 ppm. The control system delivered pure CO2 though Gas Mass Flow Controllers (GFC17 series, Aalborg, Orangeburg, NY, United States) according to the profiling schedule designed for the pCO2 treatment and the feedback signal coming from the experimental tanks. CO2 was dissolved in the flow-through water by membrane contactors (Membrana Liqui-Cel 2.5 × 8 Extra-Flow. 3M, United States). Throughout the experiment incoming coastal water had a pCO2 ranging between 500 and 550 μatm. Thus, to achieve a control pCO2 level closer to 450 μatm, membrane contactors (Membrana Liqui-Cel 4 × 28 Extra-Flow) were used to remove CO2, using CO2 – depleted air as sweep gas. Total alkalinity was measured from a random tank on half of the systems each week (the systems measured alternated each week) using Gran Titration (Metrohm 888 Titrando Titrator Metrohm AG, Switzerland) and certified reference material from Dr. A. G. Dickson (Scripps Institution of Oceanography). Mean values for seawater parameters are presented in Table 1. Supplementary Figure 1 illustrates the shape of the CO2 profiles used.
Behavioral Assays
Fast Starts
Fast start trials were performed using methods similar to those described by Munday et al. (2016). The setup consisted of a circular experimental arena (diameter 210 mm) placed inside an opaque rectangular container (550 × 410 × 320 mm). A polystyrene lid was placed on top of the container with a white PVC tube (40 mm × 300 mm) inserted through the middle. An electromagnet was attached to the top of the PVC tube to which a weighted stimulus was attached via a metal disk. The arena was illuminated by an LED strip placed around the outside of the container. The container was filled with water from the treatment fish were reared in to a depth of 50 mm to reduce movement in the vertical plane. Once introduced into the arena fish were given a 3 min habituation period. After this period, a fast start was elicited by turning off the electromagnet, thus releasing the stimulus. A length of fishing line attached to the weight caused it to stop when the tip of the weight only just touched the surface of the water after release from the magnet. To provide a sudden stimulation and allow calculation of the response latency the bottom edge of the PVC tube was placed just above the water level. This way there was no visual stimulation before mechanical stimulation. Fish were only startled when they were more than two body lengths from the arena’s edge to minimize edge effects on escape responses. Responses to the stimulus were filmed as a silhouette from below with a high-speed camera (Casio EX-ZR2000; 480 fps). To do this, the experimental setup was placed on a wooden frame with a mirror placed inside at 45° degrees. The front of the frame was covered with black plastic sheet, to minimize external disturbance, with a small hole cut out in the middle for the camera lens. Five to seven fish per tank were tested. Video recordings were analyzed with the observer blind to treatment using IMAGEJ software and calibrated against the base of the PVC tube. The very front of the fish was used as the reference point for tracking. From the videos, we quantified non-locomotor traits (response latency and directionality) and locomotor traits (turning rate, mean escape speed, maximum escape speed and escape distance). The moment the stimulus weight hit the water was benchmarked by the first detectable water disturbance in the video. Fish escape variables were only measured if a C-start was initiated.
Non-locomotor variables
(1) Response latency (in ms) was measured as the time interval between the stimulus onset and the first detectable movement leading to the escape of the animal.
(2) Directionality: escape responses were divided into ‘away’ and ‘toward’ responses when the first detectable movement of the head was oriented away and toward the stimulus, respectively.
Locomotor variables
(1) Turning rate (degrees ms-1) was measured as the angle turned before the fish swam away after the onset of the response.
(2) Mean response speed (ms-1) was measured as the distance covered within a fixed time (24 ms) which corresponds to the average duration of the first two tail flips of the tail (the first two axial bends, i.e., stages 1 and 2 defined based on Domenici and Blake (1997), which is the period considered crucial for avoiding predator ambush attacks).
(3) Maximum speed (ms-1) was measured as the maximum speed reached at any time during the response.
(4) Escape distance was measured as the straight-line distance between the position of the fish at the onset of a C-start and the position of the fish after 24 ms.
Behavioral Lateralization
Lateralization in juvenile A. polyacanthus was determined using a detour test in a two-way T-maze using methods described by Jarrold et al. (2017). The two-way T-maze consisted of an experimental arena (60 cm × 30 cm × 20 cm), with a runway in the middle (25 cm × 2 cm, length × width), and at both ends of the runway (2 cm ahead of the runway) an opaque barrier (12 cm × 12 cm × 1 cm) was positioned perpendicular to the runway. The maze was filled to a depth of 4 cm with the respective treatment water of the fish being tested, being changed after each trial. A single fish was placed at one end of the T-maze and given a 3 min habituation period, during which time it could explore the apparatus. At the end of the habituation period the fish was gently guided into the runway using a plastic rod with the observer standing directly behind the fish (the plastic rod was never placed closer than approximately twice the body length of the fish). At this point to minimize human interference affecting direction turned the observer slowly stepped back from the maze and the fish was allowed to swim to the end of the runway. In instances when a fish did not swim to the end, encouragement was provided by gently moving the plastic rod around at the beginning of the runway. Consecutive runs were carried out until 10 successful runs per fish had been recorded. Six to eight fish were tested per tank. To account for any possible asymmetry in the maze, turns were recorded alternately on the two ends of the runway. Turning preference (i.e., bias in left or right turns) at the population level was assessed using the relative lateralization index (LR, from -100 to +100, indicating complete preference for left and right turning, respectively) according to the following formula: LR = [(Turn to the right – Turn to the left)/(Turn to the right + Turn to the left)] ∗ 100. The strength of lateralization (irrespective of its direction) was also assessed at the individual-level using the absolute lateralization index LA [ranging from 0 (an individual that turned in equal proportion to the right and to the left) to 100 (an individual that turned right or left on all 10 trials)]. Trials were filmed, and fish were scored from video analysis with the observer blind to treatment. Direction choice was recorded as the first direction turned when the fish exited the runway.
Routine Activity and Boldness
Routine activity and boldness were determined using an open field test with methods similar to those described by Laubenstein et al. (2018). The setup consisted of a round, white plastic arena (26 cm diameter, 6 cm height) placed inside a white plastic bin (52 cm length, 32 cm width, 34 cm height), which was opaque to minimize visual disturbance for the fish but allowed light through for filming. Two wooden planks (L = 60 cm, H = 9 cm, W = 2 cm) were placed across the top of each plastic bin with a sheet of white corflute on top which had a small circular hole cut into its center, where a video camera (Casio EX-ZR2000) was placed. This was done so that the whole arena fitted into the camera’s field of view. Fish were tested in their treatment water at a depth of 5cm. To begin a trial, a fish was transferred into the center of the arena by gently transferring it with a beaker to minimize stress. The camera was turned on, and the fish was filmed for 15 min. Six to nine fish per tank were tested. Activity and space use in the tank were determined from the video using motion-tracking software (Lolitrack v4.1.0, Loligo Systems, Tjele, Denmark). Before each video analysis, a circular arena was drawn within the test arena, with the same central point, but which was 18 cm in diameter, or approximately 1.5 standard body lengths away from the edges of the test arena. This “center zone” was used to quantify boldness, based on the idea that an open field is considered dangerous, and that venturing into the inner zone represents boldness, or the willingness to undertake risk. Therefore, we quantified boldness by measuring the number of visits made to the center zone and time spent in the center zone. The routine activity parameters quantified by the software were: time active (%) and average swimming velocity (cm s-1). All videos were analyzed blind to treatment. The first 5 min of each video were discarded to allow some habituation time.
Data Accessibility
The datasets generated during the current study are available from the corresponding author on request or via the Tropical Research Data Hub (doi: 10.25903/5bd7c7f552897).
Statistical Analysis
All analyses were conducted using R version 3.4.0. (R Core Team, 2017) using the following packages: nlme, lme4 and glmmTBM. In all cases, except for survival data, mixed effects models were used so that random effects could be incorporated. In all mixed effects models, pCO2 and temperature treatment were fixed factors, with clutch and tank as random factors with tank nested within clutch. Initially, fully interactive models were run. However, non-significant interaction terms were gradually removed. Final model choice was confirmed based on Akaike information criterion (AIC). When a CO2 effect was observed pair-wise comparisons were conducted using Tukey’s tests using the ‘multcomp’ package. When a significant CO2 ∗ temperature interaction was found (survival data only) planned contrasts were performed. For planned comparison we compared pCO2 treatments across each temperature treatment separately and temperature treatments within a pCO2 treatment.
Survival and Growth
The effects of CO2 and temperature on juvenile survival were tested using a generalized linear model (GLM) with a binomial distribution (due to the data being proportional), weighted to the starting number of fish in each tank. The effects of CO2 and temperature on wet weight and standard length were analyzed using linear mixed effects models (LMEs). The number of fish remaining in each tank at the end of the experiment was included as a covariate. Additive models were used (number + CO2 + temperature).
Fast Starts
The effects of CO2 and temperature on latency to respond to the stimulus were tested using an LME with distance of the fish from the center of the experimental arena when the stimulus hit the water as a covariate. The effects of CO2 and temperature on direction turned were analyzed with a generalized linear mixed model (GLMM) with a binomial distribution. The effects of CO2 and temperature on turning rate, mean escape speed, maximum escape speed and escape distance were tested using LMEs with total length included as a covariate for mean and maximum escape speed and escape distance. Additive models were used for latency, direction turned, turning rate and escape distance. For mean and maximum escape speed the model tested the interactive effects between total length and temperature and the additive effect of CO2 (body length ∗ temperature + CO2).
Behavioral Lateralization
GLMMs with binomial distributions and weighted to the number of runs (10) were used to test the effects of CO2 and temperature on absolute lateralization (LA) and relative lateralization (LR) due to the proportional nature of the data. Additive models were used (CO2 + temp).
Routine Activity and Boldness
A GLMM with a binominal distribution and weighted to the length of time of the trial (10 min) was used to test the effects of CO2 and temperature on percentage time active. The effects of CO2 and temperature on velocity and time spent in the center zone (fish that did not visit the center zone were excluded from analysis) were tested using LMEs. Finally, a mixed-effects hurdles model was used to test the effects of CO2 and temperature on number of visits to the center zone due to the high number of zeros present in the dataset. Total length was included as a covariate for all traits. Additive models were used for time active, velocity and number of visits to the center zone. An interactive model was used for time spent in center zone (body length ∗ temperature ∗ CO2).
Results
Survival and Growth
Elevated temperature (Figure 1A, χ2 = 29.59, df = 1, P < 0.001) but not elevated CO2 (χ2 = 0.74, df = 3, P = 0.863) had an overall negative effect on survival of juvenile A. polyacanthus. Survivorship from hatching to 11 weeks post hatch decreased from 89.9% at 29°C to 78.2% at 31°C. In addition, a significant interactive effect between CO2 and temperature was observed (χ2 = 7.85, df = 3, P = 0.049). Specifically, elevated temperature had a negative effect on survival in the two diel-cycling CO2 treatments (max. P = 0.047), but not under control or stable elevated CO2 conditions (max. P = 0.194).
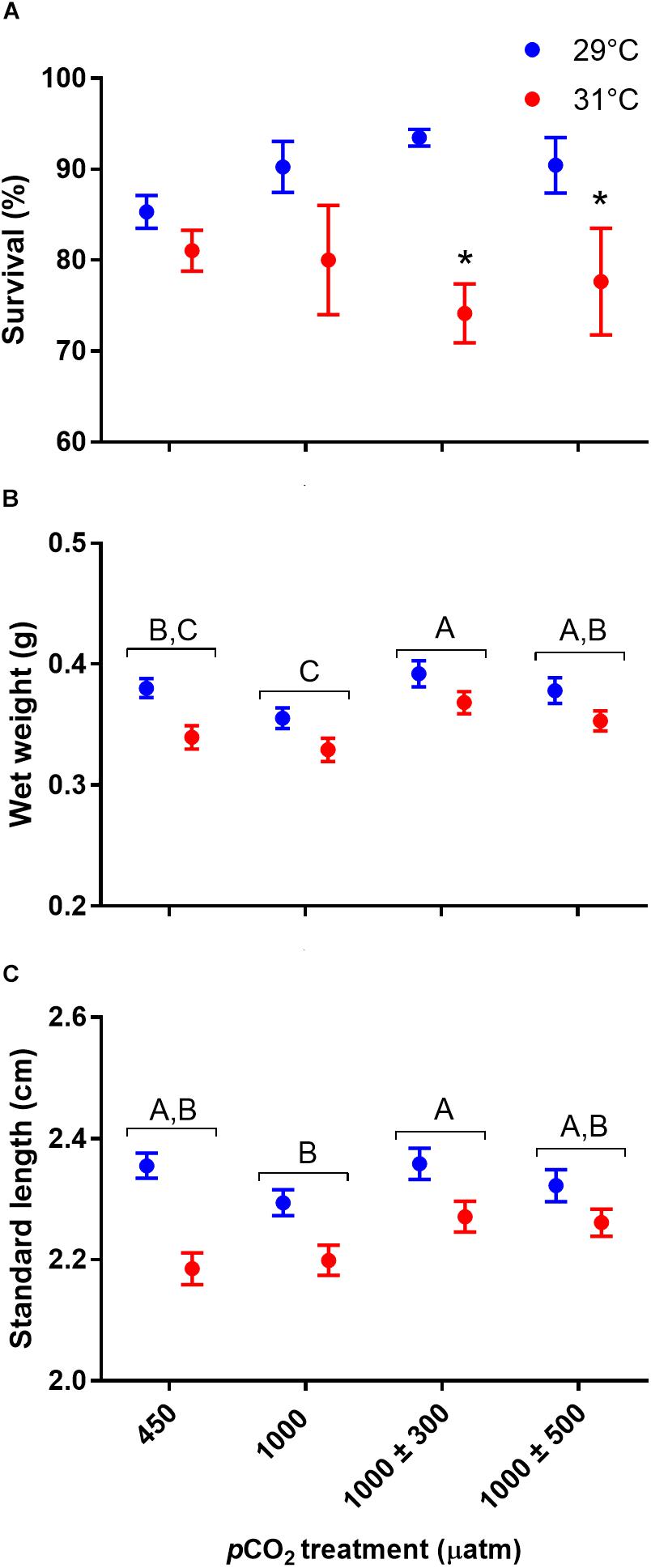
Figure 1. Effects of elevated CO2 and temperature on survival and growth of juvenile Acanthochromis polyacanthus. (A) Survival. (B) Wet weight. (C) Standard length. Different letters represent significant differences between pCO2 treatments (Tukey’s, P < 0.05). Points represent means ± SE. ∗Represent a significant difference between temperature treatments at the same pCO2 level.
Wet weight of juvenile A. polyacanthus was significantly affected by CO2 (Figure 1B, F3,54 = 6.32, P < 0.001) and temperature (Figure 1B, F1,54 = 58.90, P < 0.0001) treatment. There was a trend of decreased (-4.7%) wet weight of fish reared under stable elevated CO2 compared to those reared under control conditions, although this was non-significant (P = 0.506). However, fish reared under diel-cycling elevated CO2 conditions were significantly heavier (+11.2% for ± 300 μatm and +6.6% ± 500 μatm CO2 respectively) compared to those reared under stable elevated CO2 conditions (min. P = 0.035). Fish reared under the smaller CO2 fluctuations were also significantly heavier (+5.9%) compared to fish reared under control conditions (P = 0.033). Elevated temperature had a negative effect on wet weight of juvenile A. polyacanthus, decreasing it by 7.8% under 31°C. Finally, the number of fish remaining in the tank at the end of the experiment had a significant effect on wet weight, with heavier fish in tanks with fewer fish (F1,54 = 42.10, P < 0.0001).
Standard length of juvenile A. polyacanthus was significantly affected by CO2 (Figure 1C, F3,54 = 3.46, P = 0.023) and temperature (Figure 1C, F1,54 = 69.33, P < 0.0001) treatment. Fish reared at 1000 ± 300 μatm CO2 were significantly longer (+3.2%) compared to those reared under stable elevated CO2 (P = 0.022). However, neither were significantly different compared to control fish (min. P = 0.144). Finally, fish reared under 1000 ± 500 μatm CO2 were of comparable length to fish from all other CO2 treatments (min. P = 0.133). Elevated temperature had a significant negative effect on length of juvenile A. polyacanthus, decreasing it by 4.5% under 31°C. The number of fish remaining in the tank at the end of the experiment had a significant effect on standard length, with fish reaching a longer size in tanks with fewer fish (F1,54 = 18.08, P = 0.0001).
Behavioral Lateralization
Absolute lateralization (LA) was significantly influenced by CO2 treatment (Figure 2, χ2 = 17.57, df = 3, P < 0.001) but not temperature (χ2 = 0.37, df = 1, P = 0.541). Juveniles reared under stable elevated CO2 were less lateralized compared to those reared at control levels (P < 0.001). LA of juveniles reared at 1000 ± 300 μatm CO2 was partially restored being intermediate to fish reared under control and stable elevated CO2 (min. P = 0.060). LA of juveniles reared at 1000 ± 500 μatm CO2 was fully restored to control levels being significantly greater than those reared at stable elevated CO2 (P = 0.021). Relative lateralization (LR) was unaffected by CO2 (χ2 = 1.67, df = 3, P = 0.644) or temperature (χ2 = 0.15, df = 1, P = 0.697).
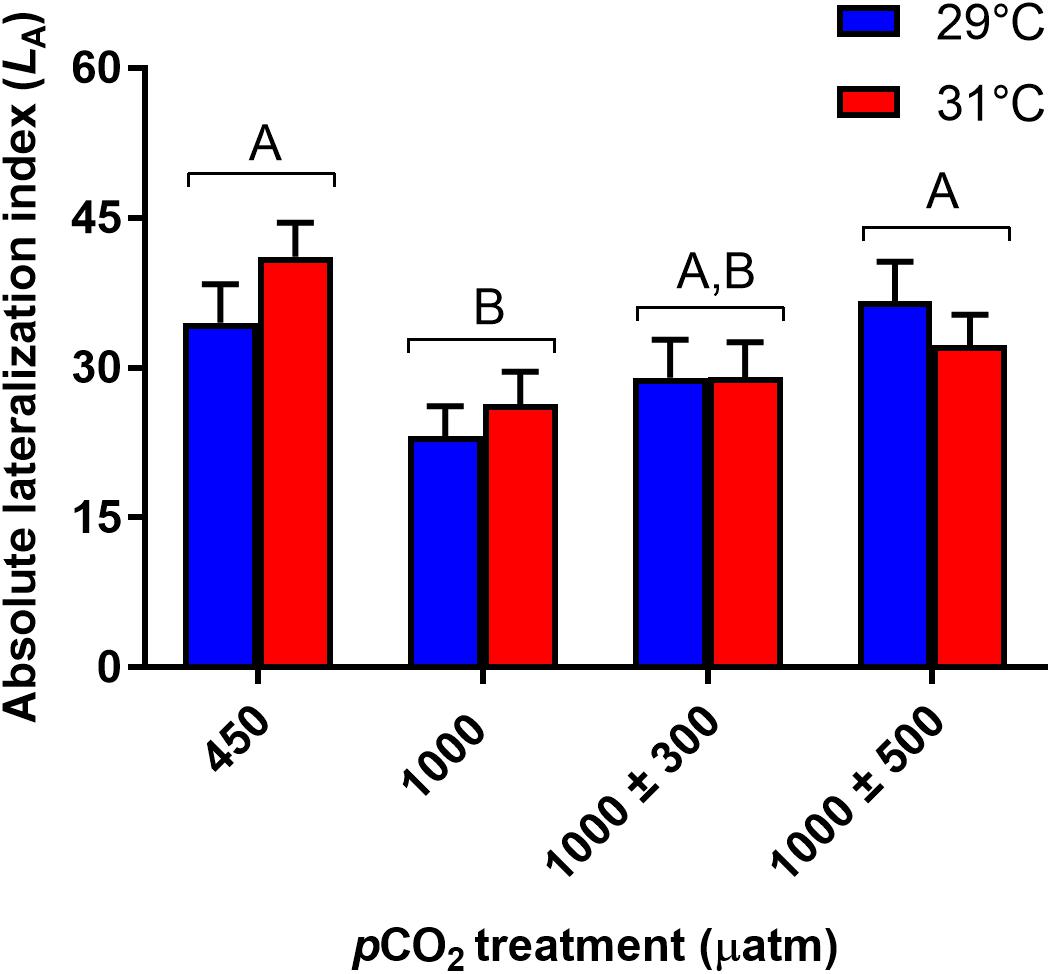
Figure 2. Effects of elevated CO2 on absolute lateralization (LA) in juvenile A. polyacanthus. Different letters represent significant differences between pCO2 treatments (Tukey, P < 0.05). Bars represent means ± SE.
Routine Activity and Boldness
Percentage time active was significantly influenced by CO2 (Figure 3A, χ2 = 11.99, df = 3, P = 0.007) and temperature (Figure 3A, χ2 = 25.51, df = 1, P < 0.001) treatments. Fish reared at 1000 ± 500 μatm CO2 were significantly less active (-10.2%) than fish reared under control conditions (P = 0.005). No other significant differences between pCO2 treatments were observed (min. P = 0.059). Elevated temperature had a negative effect on percentage time active, decreasing from 74.4% at 29°C to 64.9% at 31°C. Average velocity was also significantly affected by CO2 (Figure 3B, F3,441 = 5.15, P = 0.002) and temperature (Figure 3B, F1,441 = 16.66, P < 0.001) treatment. Fish reared at 1000 ± 500 μatm CO2 swam at lower speeds (-15.9%) compared to control fish (P = 0.001). Average velocity was negatively impacted by elevated temperature, decreasing by 14.4% at 31°C compared to fish reared at 29°C. Finally, total body length had a significant effect on average velocity (F1,441 = 12.46, P < 0.001), with longer fish swimming faster.
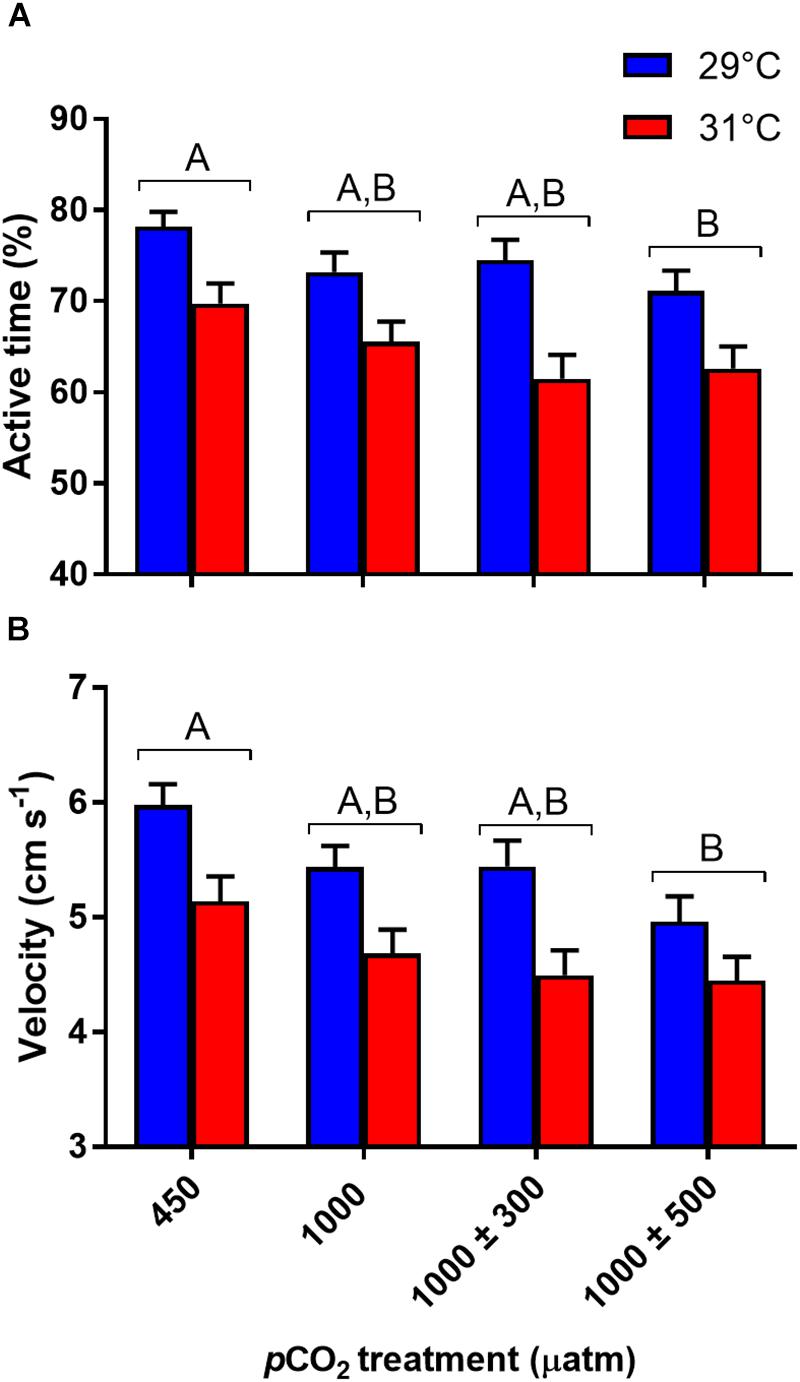
Figure 3. Effects of elevated CO2 and temperature on routine activity traits of juvenile A. polyacanthus. (A) % time active. (B) Average velocity. Different letters represent significant differences between pCO2 treatments (Tukey’s, P < 0.05). Bars represent means ± SE.
Number of visits to the center zone was not significantly influenced by CO2 treatment (Figure 4A, min. P = 0.130), but was affected by temperature (Figure 4A, P = 0.010). Fish reared at 31°C made fewer visits to the center zone. There was no effect of CO2 (Figure 4B, F3,58 = 0.07, P = 0.974) or temperature (Figure 4B, F1,336 = 0.001, P = 0.974) treatment on time spent in the center zone.
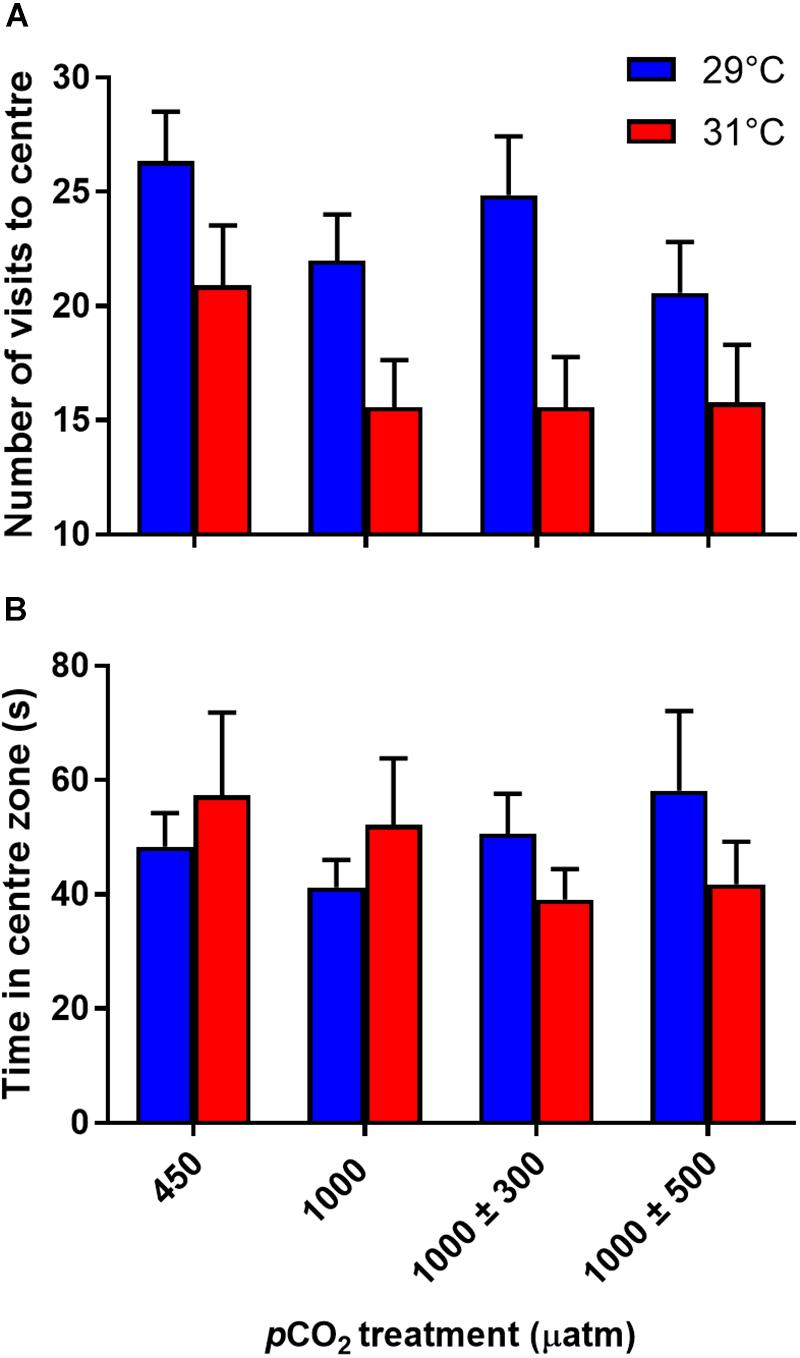
Figure 4. Effects of elevated CO2 and temperature on the boldness traits of juvenile A. polyacanthus. (A) Number of visits to center zone. (B) Time spent in center zone. Bars represent means ± SE.
Fast Starts
Latency in response to the stimulus was unaffected by CO2 (Figure 5A, F3,42 = 1.48, P = 0.234) or temperature (Figure 5A, F1,238 = 0.58, P = 0.448) treatment. The distance fish were from the center of the arena had a significant effect on escape latency with fish closer the center responding more quickly to the stimulus (F1,238 = 9.68, P = 0.002). Direction of response was also unaffected by CO2 (Figure 5B, χ2 = 4.08, df = 3, P = 0.252) or temperature (Figure 5B, χ2 = 0.00, df = 1, P = 0.988) treatment.
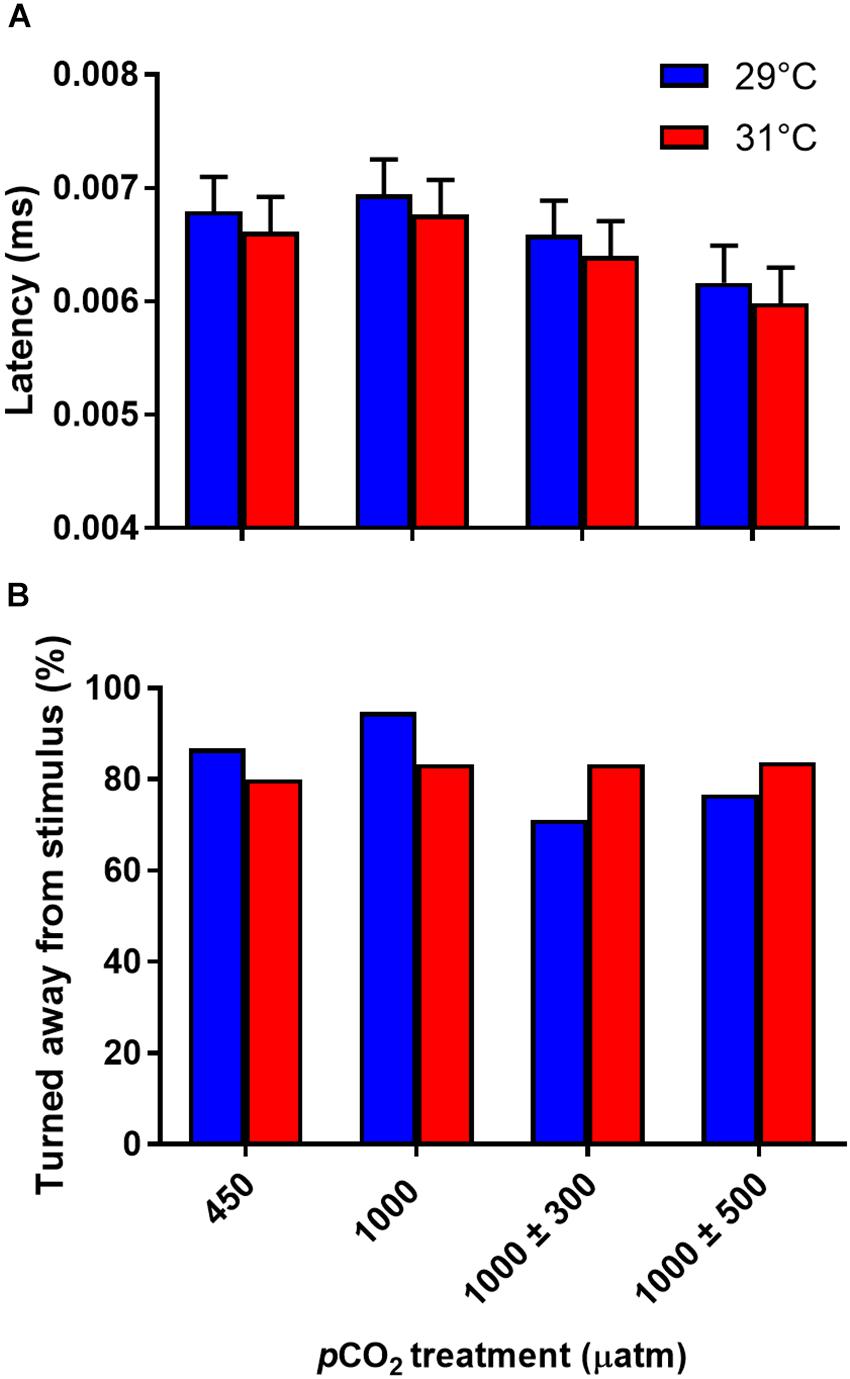
Figure 5. Effects of elevated CO2 and temperature on non-locomotor fast start traits of juvenile A. polyacanthus. (A) Response latency. (B) Direction turned. Bars represent means ± SE.
All locomotor traits (turning rate, mean escape speed, maximum escape speed and escape distance) were unaffected by CO2 treatment (max. F3,42 = 1.54, P = 0.217) but significantly affected by temperature (Figures 6A–D, min. F1,237 = 4.09, P = 0.044). Fish reared at 31°C turned at a faster rate (+8.1%), had a greater mean (+4.2%) and maximum (+5.2%) escape speed and a greater escape distance (+6.9%) compared to fish reared at 29°C. Total body length had a significant positive effect on mean escape speed, maximum escape speed and escape distance (min. F1,237 = 11.51, P < 0.001).
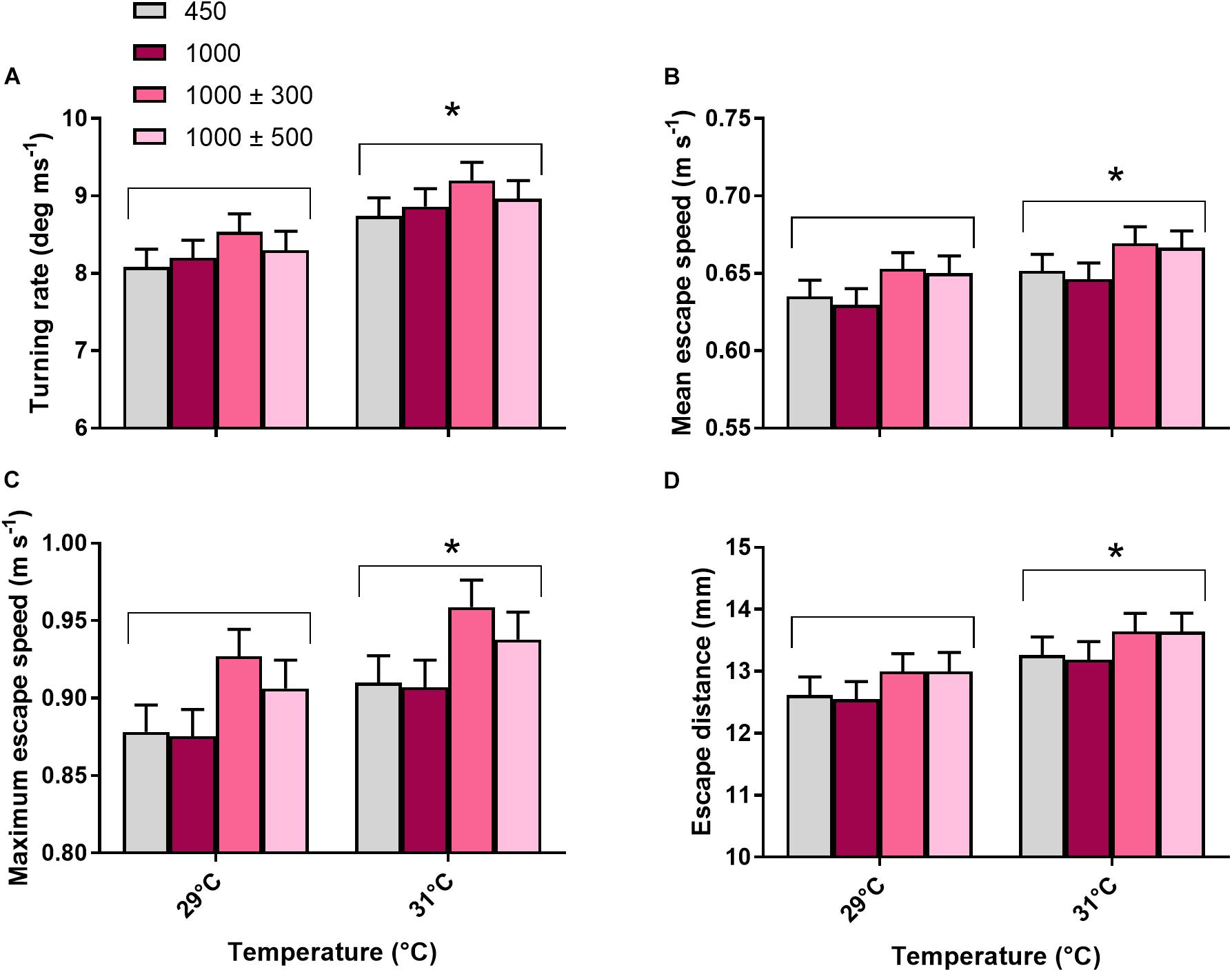
Figure 6. Effects of elevated CO2 and temperature on locomotor fast start traits of juvenile A. polyacanthus. (A) Turning rate. (B) Mean escape speed. (C) Maximum escape speed. (D) Escape distance. Bars represent means ± SE. ∗Indicates a significant difference between temperature treatments (P < 0.05).
Discussion
Recent studies have shown that diel CO2 cycles can significantly modify the effects of ocean acidification conditions on marine organisms. However, whether the interaction between elevated CO2 and diel CO2 cycles is further modified by elevated temperature is unknown. Here, we report several independent effects of elevated CO2, and interactions between elevated CO2 and diel CO2 cycles, that were mostly unaffected by elevated temperature. Our results show that diel CO2 cycles alleviated the negative effect elevated CO2 had on growth and absolute lateralization but had a negative impact on routine activity. A significant interaction between CO2 and temperature was only observed for survivorship, with lower survival in the two diel-cycling elevated CO2 treatments observed at elevated temperature (31°C). Finally, elevated temperature had significant independent effects of growth, routine activity and fast start performance.
Survival and Growth
CO2 Effects
Survival and growth of juvenile A. polyacanthus were not significantly affected by stable elevated CO2 conditions, although there was a trend of decreased growth compared with control fish. Previous experiments testing the effects of stable elevated CO2 on fish early life stages have produced highly variable and species-specific results, with negligible, negative and positive effects on survival and growth all reported (Heuer and Grosell, 2014). The sensitivity of marine fish to OA conditions has often been linked to the environmental CO2 levels they experience in natural habitats, with tolerant species generally occurring in areas that have daily and seasonal CO2 cycles, and thus may already be physiologically adapted to elevated CO2 (e.g., Munday et al., 2011; Bignami et al., 2013; Frommel et al., 2013; Perry et al., 2015). A recent meta-analysis lends some support to this theory by showing that mortality increased in pelagic species under elevated CO2, whereas mortality often decreased in benthic species, which experience higher and more variable CO2 compared with pelagic species (Cattano et al., 2018). Our results support this as A. polyacanthus is a benthic reef species that shelters within the reef-matrix at night where it is likely exposed to CO2 levels considerably higher than in the surrounding water column (e.g., Albright et al., 2013; Kline et al., 2015).
While natural CO2 cycles have been used to explain tolerance to future OA conditions in marine fish, very few studies have incorporated them in their experimental designs (but see Ou et al., 2015; Jarrold and Munday, 2018). Here, we show that diel CO2 cycles had a significant positive effect on growth of juvenile A. polyacanthus under elevated CO2. These beneficial effects were most apparent for weight wet, with fish reared under both the ±300 and ±500 μatm diel-cycling CO2 regimes being significantly heavier compared to fish reared under stable elevated CO2. We observed a similar positive effect of diel CO2 cycles on standard length, although there was only a significant difference between the ±300 μatm diel-cycling and the stable elevated CO2 treatments. These results suggest that the positive effects of diel CO2 cycles are magnitude dependent, with greater effects observed under the smaller ± 300 μatm diel-cycling CO2 regime compared with ±500 μatm CO2. This may reflect the closer match of the ±300 μatm CO2 treatment to the diel fluctuations these fish experience on the reef. Similar trends to those described above were observed in a previous study (Jarrold and Munday, 2018) carried out the same species and in which the same pCO2 treatments were used, although no significant differences were detected in that study, most likely due to the lower levels of replication (three clutches compared to the four in this study) (see Supplementary Table 1 for a summary comparison between the current and past studies). Positive effects of diel CO2 cycles on growth under elevated CO2 were also reported in the freshwater stage of larval pink salmon, O. gorbuscha, where the negative effect that 1000 μatm CO2 had on length was absent in the diel-cycling treatment (450–2000 μatm) (Ou et al., 2015).
The underlying physiological mechanism responsible for our observed positive effect of diel CO2 cycles on growth under elevated CO2 is unknown. However, our results and those of Ou et al. (2015) suggest that it is energetically less expensive for fish to live in a diel-cycling elevated CO2 environment compared to a stable elevated CO2 environment. Aerobic scope [the difference between resting metabolic rates (RMR) and maximal metabolic rates (MMR)] represents the energy budget available for an organism to undertake aerobic tasks (e.g., growth). Previous work has shown that exposure to stable elevated CO2 can alter metabolic rates in some fish, highlighting an increased cost of living (Munday et al., 2009a; Enzor et al., 2013; Strobel et al., 2013; Ou et al., 2015; Cattano et al., 2018; Laubenstein et al., 2018). Thus, we suggest that the positive effects of diel CO2 cycles on growth could be driven by increased aerobic scope under such conditions. In line with this, Ou et al. (2015) reported that maximal metabolic rates (MMR) of larval pink salmon after transition to saltwater were negatively impacted by stable elevated CO2 (1600 μatm), but not a by diel-cycling conditions (450–1600 μatm), with resting metabolic rates (RMR) being similar in both treatments. However, because the mean CO2 also differed between the stable and fluctuating treatments in that experiment it is not possible to be certain whether the changes in MMR were being driven primarily by the cycling CO2 regime or change in mean CO2. Alterations in aerobic capacity under elevated CO2 may be linked to the increased metabolic costs associated with defending acid-base status (Perry and Gilmour, 2006; Baker and Brauner, 2012). Thus, we hypothesize that the cost of acid-base regulation under elevated CO2 is reduced by the presence of diel CO2 cycles, ultimately resulting in more energy available for life-history processes such as growth.
Temperature Effects
Elevated temperature (31°C) negatively impacted both survival and growth of juvenile A. polyacanthus. This demonstrates that 31°C is above the optimal temperature for this species and is consistent with previous findings (Munday et al., 2008; Rodgers et al., 2018). The negative impacts of elevated temperature on growth and survival were most likely correlated to increased energetic demands associated with increased metabolic costs. Indeed, previous work on coral reef fish, including A. polyacanthus, has shown that exposure to 31°C causes a significant increase in RMR and subsequent decrease in aerobic scope (Munday et al., 2009a; Nilsson et al., 2009; Donelson et al., 2012). While elevated temperature (31°C) had an overall negative effect on survival of juvenile A. polyacanthus, CO2 treatment dependent effects were present. There was a trend of decreased survival in all the CO2 treatments at 31°C; however, significant differences were only observed in the two diel-cycling elevated CO2 treatments. Most mortality was observed in the mornings, indicating that deaths were occurring at night during the high CO2 peaks, within the first week of the experiment. These observations suggest that for newly hatched fish, which might not have fully developed acid-base regulation capacity, and for which the cost of homeostasis is greater (Brauner, 2009), the metabolic demands to tolerate the higher CO2 levels caused by diel cycles become too great under elevated temperature. The effects of elevated temperature on fish early life growth have been varied. For example, temperatures above the natural preference range were shown to enhance growth of larval yellowtail kingfish, Seriola lalandi (Watson et al., 2018). In contrast, elevated temperature negatively impacted the growth and survival of larval Atlantic herring, Clupea harengus, despite the elevated temperature still being within the species preferred thermal range (Sswat et al., 2018). Differences between studies could potentially be linked to food availability, with studies reporting positive effects usually providing a continuous supply of high-density food that enabled energetic demands to be met. In this study, juvenile A. polyacanthus were fed a fixed ration of food daily, however, previous work on this species has shown that the effects of 31°C on growth were no different depending on whether fish were fed a high or low quality diet (Munday et al., 2008). Thus, we are confident that our feeding regime had little impact on the observed response.
Behavior
CO2 Effects
Behavioral abnormalities in fish under OA conditions are thought to be linked to the effects of acid-base regulation on the function of type A γ-aminobutyric acid (GABAA) neurotransmitter receptors which have specific conductance for HCO3- and Cl- (Nilsson et al., 2012; Hamilton et al., 2014; Heuer et al., 2016). Under elevated CO2 fishes increase intracellular and extracellular HCO3- concentrations, with a corresponding decrease in Cl-, to prevent plasma and tissue acidosis (Wood et al., 1990; Baker et al., 2009; Heuer and Grosell, 2014). This altered ion gradient is thought to turn some GABAA receptors from inhibitory to excitatory, ultimately leading to behavioral impairments (Heuer et al., 2016). Importantly, acid-base regulation in fish is under circadian control (Peterson and Gilmore, 1988). In a recent study on A. polyacanthus, variation in behavioral tolerance to stable elevated CO2 was linked to the differential expression of genes related to circadian rhythm control and indicated that behaviorally sensitive fish may have been displaying more pronounced acid-base regulation (Schunter et al., 2016). This suggests that diel CO2 cycles, and associated circadian rhythms, are likely to have an important influence on the sensitivity of reef fishes to elevated CO2.
Consistent with previous work, we observed that absolute lateralization of juvenile A. polyacanthus was significantly reduced under stable elevated CO2 (Welch et al., 2014; Jarrold et al., 2017). Similar results have been reported in a range of other species (Domenici et al., 2012; Jutfelt et al., 2013; Green and Jutfelt, 2014; Lopes et al., 2016; Schmidt et al., 2017). Furthermore, in agreement with a past study, we show that the severity of behavioral impairment under elevated CO2 is reduced by the presence of diel CO2 cycles, with partial restoration in the smaller diel-cycling treatment and full restoration in the larger diel-cycling treatment (Jarrold et al., 2017; see Supplementary Table 1 for a summary comparison between studies). This is an important finding given the ecological consequences of behavioral abnormalities and that past research has shown a limited capacity for acclimation/adaptation of behavioral traits to stable elevated CO2 (Welch et al., 2014; Welch and Munday, 2017). Our lateralization results suggest that GABAA receptors were functioning more normally under elevated CO2 when a diel cycling CO2 regime was present. This could indicate that fish kept under diel CO2 cycles underwent less acid-base compensation compared to those reared under stable elevated CO2. For coral reef fish it appears that complete acid-base regulation in the brain under stable elevated CO2 levels takes between 24 and 96 h, as this is the exposure period required before behavioral abnormalities manifest (Munday et al., 2010). Our results suggest that for fish reared under diel-cycling CO2, exposure to lower CO2 levels for several hours each day is enough to prevent the physiological changes that would normally occur at a stable high CO2 (see discussion in Jarrold et al., 2017 for more detail). It is possible that changes in acid-base regulation under diel-cycling elevated CO2 is linked to altered expression of circadian rhythm genes. Further work is needed to determine if and how acid-base regulation is altered under elevated CO2 when diel CO2 cycles are present.
Routine activity and boldness of juvenile A. polyacanthus were unaffected by exposure to stable elevated CO2, which is consistent with some previous work (Nowicki et al., 2012; Heinrich et al., 2016; Sundin et al., 2017). Yet, these observations contrast with other studies on coral reef fish where significant effects of elevated CO2 on routine activity have been documented (Munday et al., 2010, 2014; Cripps et al., 2011; Devine et al., 2012; McCormick et al., 2013). Instead, our findings are more consistent with what has been observed in non-tropical species (Maneja et al., 2015; Sundin and Jutfelt, 2015; Duteil et al., 2016; Schmidt et al., 2017). The underlying explanation for discrepancies between studies is unclear but may be related to methodological differences. In general, the studies on coral reef fish which have reported significant effects of stable elevated CO2 on activity/boldness tested fish in the field on natural reef structures, or in an artificial tank environment which had a shelter. In contrast, many of the studies reporting no affects, including this one, conducted open field tests in an artificial environment, generally, without any shelter and/or with minimal acclimation time. Thus, it could be that without the option of a ‘safe place’ and/or insufficient acclimation time the impacts of elevated CO2 are masked by the effects of a general stress response.
While routine activity was unaffected by stable elevated CO2, it was altered by the presence of a ± 500 μatm CO2 diel cycle, with fish in the cycling elevated CO2 treatment being less active (-10.2%) and swimming slower (-15.9%) compared to controls. Impaired metabolic performance can likely be ruled out a as a potential underlying mechanism for the observed decrease in routine activity because increased growth was observed under these conditions. We propose that this decrease in routine activity was associated with altered anxiety levels. Activity in a novel environment can be an indicator of anxiety, whereby reduced activity is usually associated with increased anxiety (Egan et al., 2009; Maximino et al., 2010). Increased anxiety/reduced boldness in stable elevated CO2 conditions has been observed in juvenile Californian rockfish, Sebastes diploproa, (Hamilton et al., 2014) and three-spined stickleback, Gasterosteus aculeatus, (Jutfelt et al., 2013), but why we only observed altered anxiety in the largest diel-cycling elevated CO2 treatment is unclear. Anxiety in fish is linked to GABAA receptor functioning, with normal function acting to reduce anxiety (Stewart et al., 2011; Hamilton et al., 2014). Our behavioral laterization data and previous work (Jarrold et al., 2017), where diel CO2 cycles had a positive effect on behavior, suggests that GABAA receptor functioning is closer to normal under elevated CO2 when diel CO2 cycles are present. Thus, it appears that another physiological mechanism, other than the GABAA pathway, may be responsible for our observed reduced activity in the 1000 ± 500 μatm CO2 treatment. One possible explanation is that the higher CO2 peaks may have altered stress hormone levels, such as cortisol, which are known to directly correlate with anxiety behaviors in fish (Egan et al., 2009; Cachat et al., 2010). The link between behavioral responses and stress hormones in fish under elevated CO2 conditions is currently unknown and would be an interesting area for future work.
All fast start non-locomotor and locomotor variables in juvenile A. polyacanthus were unaffected by exposure to elevated CO2, regardless of whether a stable or cycling regime was experienced. This contrasts with most previous studies on coral reef fish were significant effects of elevated CO2 on fast starts have been observed (Allan et al., 2013, 2014; Munday et al., 2016). Negative effects of elevated CO2 on fast start performance were also observed in the temperate species yellowtail kingfish, S. lalandi (Watson et al., 2018). Our results are comparable to those reported for the coral reef damselfish Pomacentrus wardi, where elevated CO2 was shown to have no effect on escape speed and distance (Allan et al., 2017). The methods and CO2 levels used in all studies, including this one, were very similar and so differences are most likely due to species-specific responses.
Temperature Effects
Elevated temperature had a clear negative effect on routine activity and boldness of juvenile A. polyacanthus. These results contrast with previous experiments on coral reef damselfish were activity and boldness were generally shown to increase at higher temperatures (Biro et al., 2010; McCormick and Meekan, 2010). However, the temperatures used in these studies were within the species preferred thermal range. When this is the case fish become more active to seek food so that increased metabolic costs associated with higher temperatures can be met (Biro et al., 2010). In contrast, when temperatures exceed a species optimum, as was the case in this study, reduced activity could represent an energy-saving strategy (Johansen and Jones, 2011). Additionally, elevated temperatures have been shown to increase stress hormone levels in fish (Davis, 2004) which is potentially another mechanism driving the observed reduction in activity and boldness, although additional testing is needed to confirm this.
In contrast to routine activity, elevated temperature had a small positive effect (4–8%) on fast start locomotor variables. Allan et al. (2017) also showed that elevated temperature had a stonger effect on fast start performance compared to elevated CO2, although in this case the effects were negative. Watson et al. (2018) observed enhanced fast start performance in larval yellowtail kingfish at temperatures above their preferred range, although this was attributed to increased larval size under elevated temperature. While elevated temperature had a negative effect on size of juvenile A. polyacanthus in this study there was no difference in the size of fish selected for fast start trials between treatments. The increased locomotory performance observed in this study may therefore have been linked to the combined effects of reduced viscosity and increased muscle efficiency in warmer water (Fuiman and Batty, 1997; Wieser and Kaufmann, 1998). The underlying reason for the opposing effects elevated temperature had on routine activity and fasts starts is unknown but may be related to the fact routine activity is under aerobic control whereas fast starts are driven by anaerobic metabolism (Domenici and Blake, 1997).
Summary
Diel CO2 cycles had a significant effect on three ecologically important traits in A. polycanthus. Firstly, diel CO2 cycles were shown to mediate the negative effects of elevated CO2 on absolute lateralization, confirming results of a previous study. Secondly, diel CO2 cycles had a significant positive effect on growth under elevated CO2. Finally, routine activity of fish from the larger diel-cycling CO2 treatment was significantly lower compared to control fish. Importantly, these effects were observed at both current-day and future elevated temperatures, demonstrating that higher temperature did not significantly alter the effects of diel CO2 cycles in the traits measured here. These findings highlight the importance of incorporating natural CO2 variability in experiments to more accurately assess the impacts of future OA on shallow water coastal organisms (Cornwall et al., 2013; Frieder et al., 2014; Ou et al., 2015; Jarrold et al., 2017; Wahl et al., 2018).
In contrast to elevated CO2, elevated temperature had a significant effect on every trait except behavioral lateralization. Furthermore, in all cases when a trait was significantly affected by both climate change drivers’ temperature had the strongest affect. While this is unsurprising for survival and growth, it is perhaps unexpected for behavioral traits where elevated CO2 has often been shown to have a significant impact. Our results suggest that behavioral responses that involve decision making (e.g., lateralization and response to cues) may indeed be more impacted by elevated CO2, whereas responses which are governed by energetic constraints (e.g., activity, fast starts and competitive interactions) will be more impacted by elevated temperature. This is consistent with other recent studies where elevated temperature had a stronger effect on fast start performance and swimming activity compared to elevated CO2 (Allan et al., 2017; Watson et al., 2018).
A limitation of this study was that we were unable to include a diel-cycling control treatment. It is possible that fish reared under a diel-cycling control may perform differently than to those reared under a stable control (Cornwall et al., 2013). For example, as was observed under elevated CO2, it is possible that fish reared under a diel-cycling control may perform better compared to those reared under a stable control and thus the negative effects of elevated CO2 may still be present. However, based on our current understanding of the impacts elevated CO2 has on fishes we do not believe this would be case, although further work is needed to confirm this. In this study we only incorporated diel cycles with elevated CO2 as this was a main stressor of interest. However, shallow water habitats also experience diel cycles in temperature (e.g., Kline et al., 2015), which are also known to affect fish performance (e.g., Hokanson et al., 1977). Importantly, the highest level of temperature and CO2 cycles occurs at opposite times of the day, with CO2 cycles having their high peak during the night and temperature cycles having their high peak during the day. Thus, the responses of fish to a cycling CO2 and temperature environment could be different to a static one, or when one stressor is static as was the case in this study. Future experiments should incorporate both diel-cycling CO2 and temperature to further our understating on how marine fish will respond to climate change.
Author Contributions
MJ and PM contributed to conception and design of the study. MJ performed the experiments and analyses. MJ wrote the first draft of the manuscript with input from PM.
Funding
This project was funded by the AustralianResearch Council (PLM). MJ was supported by an International Postgraduate Research Scholarship.
Conflict of Interest Statement
The authors declare that the research was conducted in the absence of any commercial or financial relationships that could be construed as a potential conflict of interest.
Acknowledgments
We thank the SeaSim team for their technical support throughout the experiment at AIMS. Additionally, we thank Rhondda Jones for statistical support.
Supplementary Material
The Supplementary Material for this article can be found online at: https://www.frontiersin.org/articles/10.3389/fmars.2018.00458/full#supplementary-material
Footnotes
References
Albright, R., Langdon, C., and Anthony, K. R. N. (2013). Dynamics of seawater carbonate chemistry, production, and calcification of a coral reef flat, Central Great Barrier Reef. Biogeosciences 10, 6747–6758. doi: 10.5194/bgd-10-7641-2013
Allan, B. J. M., Domenici, P., McCormick, M. I., Watson, S.-A., and Munday, P. L. (2013). Elevated CO2 affects predator-prey interactions through altered performance. PLoS One 8:e58520. doi: 10.1371/journal.pone.0058520
Allan, B. J. M., Domenici, P., Watson, S. A., Munday, P. L., and McCormick, M. I. (2017). Warming has a greater effect than elevated CO2 on predator–prey interactions in coral reef fish. Proc. R. Soc. B Biol. Sci. 284:20170784. doi: 10.1098/rspb.2017.0784
Allan, B. J. M., Miller, G. M., McCormick, M. I., Domenici, P., and Munday, P. L. (2014). Parental effects improve escape performance of juvenile reef fish in a high-CO2 world. Proc. R. Soc. B Biol. Sci. 281:20132179. doi: 10.1098/rspb.2013.2179
Baker, D. W., and Brauner, C. J. (2012). Metabolic changes associated with acid-base regulation during hypercarbia in the CO2-tolerant chondrostean, white sturgeon (Acipenser transmontanus). Comp. Biochem. Physiol. A Mol. Integr. Physiol. 161, 61–68. doi: 10.1016/j.cbpa.2011.09.002
Baker, D. W., Matey, V., Huynh, K. T., Wilson, J. M., Morgan, J. D., and Brauner, C. J. (2009). Complete intracellular pH protection during extracellular pH depression is associated with hypercarbia tolerance in white sturgeon, Acipenser transmontanus. Am. J. Physiol. Regul. Integr. Comp. Physiol. 296, 1868–1880. doi: 10.1152/ajpregu.90767.2008
Baumann, H., Wallace, R. B., Tagliaferri, T., and Gobler, C. J. (2015). Large natural pH, CO2 and O2 fluctuations in a temperate tidal salt marsh on diel, seasonal, and interannual time scales. Estuaries Coast. 38, 220–231. doi: 10.1007/s12237-014-9800-y
Bignami, S., Sponaugle, S., and Cowen, R. K. (2013). Response to ocean acidification in larvae of a large tropical marine fish, Rachycentron canadum. Glob. Chang. Biol. 19, 996–1006. doi: 10.1111/gcb.12133
Biro, P. A., Beckmann, C., and Stamps, J. A. (2010). Small within-day increases in temperature affects boldness and alters personality in coral reef fish. Proc. R. Soc. B Biol. Sci. 277, 71–77. doi: 10.1098/rspb.2009.1346
Brauner, C. J. (2009). “Acid-base balance,” in Fish Larval Physiology, eds R. N. Finn and B. G. Kapoor (Enfield: Science Publishers), 185–198. doi: 10.1016/j.tree.2006.11.002
Cachat, J., Stewart, A., Grossman, L., Gaikwad, S., Kadri, F., Chung, K. M., et al. (2010). Measuring behavioral and endocrine responses to novelty stress in adult zebrafish. Nat. Protoc. 5, 1786–1799. doi: 10.1038/nprot.2010.140
Cattano, C., Calò, A., Di Franco, A., Firmamento, R., Quattrocchi, F., Sdiri, K., et al. (2017). Ocean acidification does not impair predator recognition but increases juvenile growth in a temperate wrasse off CO2 seeps. Mar. Environ. Res. 132, 33–40. doi: 10.1016/j.marenvres.2017.10.013
Cattano, C., Claudet, J., Domenici, P., and Milazzo, M. (2018). Living in a high CO2 world: a global meta-analysis shows multiple trait-mediated responses of fish to ocean acidification. Ecol. Monogr. 88, 320–335. doi: 10.1002/ecm.1297
Challener, R. C., Robbins, L. L., and Mcclintock, J. B. (2016). Variability of the carbonate chemistry in a shallow, seagrass-dominated ecosystem: implications for ocean acidification experiments. Mar. Freshw. Res. 67, 163–172. doi: 10.1071/MF14219
Cheung, W. W. L., Sarmiento, J. L., Dunne, J., Frölicher, T. L., Lam, V. W. Y., Palomares, M. L. D., et al. (2013). Shrinking of fishes exacerbates impacts of global ocean changes on marine ecosystems. Nat. Clim. Chang. 3, 254–258. doi: 10.1038/nclimate1691
Collins, M., Knutti, R., Arblaster, J., Dufresne, J.-L., Fichefet, T., Friedlingstein, P., et al. (2013). “Long-term climate change: projections, commitments and irreversibility,” in Climate Change 2013: The Physical Science Basis. Contribution of Working Group I to the Fifth Assessment Report of the Intergovernmental Panel on Climate Change, eds T. F. Stocker, D. Qin, G.-K. Plattner, M. Tignor, S. K. Allen, J. Boschung, et al. (New York, NY: Cambridge University Press), 1029–1136.
Cornwall, C. E., Hepburn, C. D., McGraw, C. M., Currie, K. I., Pilditch, C. A., Hunter, K. A., et al. (2013). Diurnal fluctuations in seawater pH influence the response of a calcifying macroalga to ocean acidification. Proc. R. Soc. B Biol. Sci. 280:20132201. doi: 10.1098/rspb.2013.2201
Cripps, I. L., Munday, P. L., and McCormick, M. I. (2011). Ocean acidification affects prey detection by a predatory reef fish. PLoS One 6:e22736. doi: 10.1371/journal.pone.0022736
Davis, K. B. (2004). Temperature affects physiological stress responses to acute confinement in sunshine bass (Morone chrysops × Morone saxatilis). Comp. Biochem. Physiol. A Mol. Integr. Physiol. 139, 433–440. doi: 10.1016/j.cbpb.2004.09.012
Devine, B. M., Munday, P. L., and Jones, G. P. (2012). Homing ability of adult cardinalfish is affected by elevated carbon dioxide. Oecologia 168, 269–276. doi: 10.1007/s00442-011-2081-2
Domenici, P., Allan, B., McCormick, M. I., and Munday, P. L. (2012). Elevated carbon dioxide affects behavioural lateralization in a coral reef fish. Biol. Lett. 8, 78–81. doi: 10.1098/rsbl.2011.0591
Domenici, P., Allan, B. J. M., Watson, S.-A., McCormick, M. I., and Munday, P. L. (2014). Shifting from right to left: the combined effect of elevated CO2 and temperature on behavioural lateralization in a coral reef fish. PLoS One 9:e87969. doi: 10.1371/journal.pone.0087969
Domenici, P., and Blake, R. W. (1997). The kinematics and performance of fish fast-start swimming. J. Exp. Biol. 200, 1165–1178.
Donelson, J. M., Munday, P. L., McCormick, M. I., and Pitcher, C. R. (2012). Rapid transgenerational acclimation of a tropical reef fish to climate change. Nat. Clim. Chang. 2, 30–32. doi: 10.1038/nclimate1323
Doney, S., Ruckelshaus, M., Duffy, E., Barry, J., Chan, F., English, C., et al. (2012). Climate change impacts on marine ecosystems. Ann. Rev. Mar. Sci. 4, 11–37. doi: 10.1146/annurev-marine-041911-111611
Duarte, C. M., Hendriks, I. E., Moore, T. S., Olsen, Y. S., Steckbauer, A., Ramajo, L., et al. (2013). Is ocean acidification an open-ocean syndrome? Understanding anthropogenic impacts on seawater pH. Estuaries Coast. 36, 221–236. doi: 10.1007/s12237-013-9594-3
Duteil, M., Pope, E. C., Pérez-Escudero, A., de Polavieja, G. G., Fürtbauer, I., Brown, M. R., et al. (2016). European sea bass show behavioural resilience to near-future ocean acidification. R. Soc. Open Sci. 3:160656. doi: 10.1098/rsos.160656
Egan, R. J., Bergner, C. L., Hart, P. C., Cachat, J. M., Canavello, P. R., Elegante, M. F., et al. (2009). Understanding behavioral and physiological phenotypes of stress and anxiety in zebrafish. Behav. Brain Res. 205, 38–44. doi: 10.1016/j.bbr.2009.06.022
Enochs, I. C., Manzello, D. P., Jones, P. J., Aguilar, C., Cohen, K., Valentino, L., et al. (2018). The influence of diel carbonate chemistry fluctuations on the calcification rate of Acropora cervicornis under present day and future acidification conditions. J. Exp. Mar. Biol. Ecol. 506, 135–143. doi: 10.1016/j.jembe.2018.06.007
Enzor, L. A., Zippay, M. L., and Place, S. P. (2013). High latitude fish in a high CO2 world: synergistic effects of elevated temperature and carbon dioxide on the metabolic rates of Antarctic notothenioids. Comp. Biochem. Physiol. A Mol. Integr. Physiol. 164, 154–161. doi: 10.1016/j.cbpa.2012.07.016
Falter, J. L., Lowe, R. J., Zhang, Z., and Mcculloch, M. (2013). Physical and biological controls on the carbonate chemistry of coral reef waters: effects of metabolism, wave forcing, sea level, and geomorphology. PLoS One 8:e53303. doi: 10.1371/journal.pone.0053303
Ferrari, M. C. O., Munday, P. L., Rummer, J. L., McCormick, M. I., Corkill, K., Watson, S.-A., et al. (2015). Interactive effects of ocean acidification and rising sea temperatures alter predation rate and predator selectivity in reef fish communities. Glob. Chang. Biol. 21, 1848–1855. doi: 10.1111/gcb.12818
Frieder, C. A., Gonzalez, J. P., Bockmon, E. E., Navarro, M. O., and Levin, L. A. (2014). Can variable pH and low oxygen moderate ocean acidification outcomes for mussel larvae? Glob. Chang. Biol. 20, 754–764. doi: 10.1111/gcb.12485
Frommel, A. Y., Schubert, A., Piatkowski, U., and Clemmesen, C. (2013). Egg and early larval stages of Baltic cod, Gadus morhua, are robust to high levels of ocean acidification. Mar. Biol. 160, 1825–1834. doi: 10.1007/s00227-011-1876-3
Fuiman, L., and Batty, R. (1997). What a drag it is getting cold: partitioning the physical and physiological effects of temperature on fish swimming. J. Exp. Biol. 200, 1745–1755.
Gobler, C. J., Merlo, L. R., Morrell, B. K., and Griffith, A. W. (2018). Temperature, acidification, and food supply interact to negatively affect the growth and survival of the forage Fish, Menidia beryllina (Inland Silverside), and Cyprinodon variegatus (Sheepshead Minnow). Front. Mar. Sci. 5:86. doi: 10.3389/fmars.2018.00086
Goldenberg, S. U., Nagelkerken, I., Marangon, E., Bonnet, A., Ferreira, C. M., and Connell, S. D. (2018). Ecological complexity buffers the impacts of future climate on marine consumers. Nat. Clim. Chang. 8, 229–233. doi: 10.1038/s41558-018-0086-0
Green, B. S., and Fisher, R. (2004). Temperature influences swimming speed, growth and larval duration in coral reef fish larvae. J. Exp. Mar. Biol. Ecol. 299, 115–132. doi: 10.1016/j.jembe.2003.09.001
Green, L., and Jutfelt, F. (2014). Elevated carbon dioxide alters the plasma composition and behaviour of a shark. Biol. Lett. 10:20140538. doi: 10.1098/rsbl.2014.0538
Hamilton, T. J., Holcombe, A., and Tresguerres, M. (2014). CO2 -induced ocean acidification increases anxiety in Rockfish via alteration of GABA A receptor functioning. Proc. R. Soc. 281:20132509. doi: 10.1098/rspb.2013.2509
Heinrich, D. D. U., Watson, S.-A., Rummer, J. L., Brandl, S. J., Simpfendorfer, C. A., Heupel, M. R., et al. (2016). Foraging behaviour of the epaulette shark Hemiscyllium ocellatum is not affected by elevated CO2. ICES J. Mar. Sci. 73, 633–640. doi: 10.1093/icesjms/fss153
Heuer, R. M., and Grosell, M. (2014). Physiological impacts of elevated carbon dioxide and ocean acidification on fish. Am. J. Physiol. Regul. Integr. Comp. Physiol. 307, R1061–R1084. doi: 10.1152/ajpregu.00064.2014
Heuer, R. M., Welch, M. J., Rummer, J. L., Munday, P. L., and Grosell, M. (2016). Altered brain ion gradients following compensation for elevated CO2 are linked to behavioural alterations in a coral reef fish. Sci. Rep. 6:33216. doi: 10.1038/srep33216
Hofmann, G. E., Smith, J. E., Johnson, K. S., Send, U., Levin, L. A., Paytan, A., et al. (2011). High-frequency dynamics of ocean pH: a multi-ecosystem comparison. PLoS One 6:e28983. doi: 10.1371/journal.pone.0028983
Hokanson, K. E. F., Kleiner, C. F., and Thorslund, T. W. (1977). Effects of constant temperatures and diel temperature fluctuations on specilic growth and mortality rates and yield of juvenile rainbow trout. J. Fish. Res. Board Canada 34, 639–648.
Jarrold, M. D., Humphrey, C., McCormick, M. I., and Munday, P. L. (2017). Diel CO2 cycles reduce severity of behavioural abnormalities in coral reef fish under ocean acidification. Sci. Rep. 7:10153. doi: 10.1038/s41598-017-10378-y
Jarrold, M. D., and Munday, P. L. (2018). Diel CO2 cycles do not modify juvenile growth, survival and otolith development in two coral reef fish under ocean acidification. Mar. Biol. 165:49. doi: 10.1007/s00227-018-3311-5
Johansen, J. L., and Jones, G. P. (2011). Increasing ocean temperature reduces the metabolic performance and swimming ability of coral reef damselfishes. Glob. Chang. Biol. 17, 2971–2979. doi: 10.1111/j.1365-2486.2011.02436.x
Jutfelt, F., Bresolin de Souza, K., Vuylsteke, A., and Sturve, J. (2013). Behavioural disturbances in a temperate fish exposed to sustained high-CO2 levels. PLoS One 8:e65825. doi: 10.1371/journal.pone.0065825
Jutfelt, F., and Hedgärde, M. (2013). Atlantic cod actively avoid CO2 and predator odour, even after long-term CO2 exposure. Front. Zool. 10:81. doi: 10.1186/1742-9994-10-81
Kavanagh, K. D. (2000). Larval brooding in the marine damselfish Acanthochromis polyacanthus (Pomacentridae) is correlated with highly divergent morphology, ontogeny and life-history traits. Bull. Mar. Sci. 66, 321–337.
Kayanne, H., Suzuki, A., and Saito, H. (1995). Diurnal changes in the partial pressure of carbon dioxide in coral reef water. Science 269, 214–216.
Kline, D. I., Teneva, L., Hauri, C., Schneider, K., Miard, T., Chai, A., et al. (2015). Six month in situ high-resolution carbonate chemistry and temperature study on a coral reef flat reveals asynchronous pH and temperature anomalies. PLoS One 10:e0127648. doi: 10.1371/journal.pone.0127648
Kroeker, K. J., Micheli, F., and Gambi, M. C. (2012). Ocean acidification causes ecosystem shifts via altered competitive interactions. Nat. Clim. Chang. 3, 156–159. doi: 10.1038/nclimate1680
Kwan, G. T., Hamilton, T. J., and Tresguerres, M. (2017). CO2-induced ocean acidification does not affect individual or group behaviour in a temperate damselfish. R. Soc. Open Sci. 4:170283. doi: 10.1098/rsos.170283
Laubenstein, T., Rummer, J., Nicol, S., Parsons, D., Pether, S., Pope, S., et al. (2018). Correlated effects of ocean acidification and warming on behavioral and metabolic traits of a large pelagic fish. Diversity 10:35. doi: 10.3390/d10020035
Lopes, A. F., Morais, P., Pimentel, M., Rosa, R., Munday, P. L., Goncalves, E. J., et al. (2016). Behavioural lateralization and shoaling cohesion of fish larvae altered under ocean acidification. Mar. Biol. 163:243. doi: 10.1007/s00227-016-3026-4
Maneja, R. H., Frommel, A. Y., Browman, H. I., Geffen, A. J., Folkvord, A., Piatkowski, U., et al. (2015). The swimming kinematics and foraging behavior of larval Atlantic herring (Clupea harengus L.) are unaffected by elevated pCO2. J. Exp. Mar. Biol. Ecol. 466, 42–48. doi: 10.1016/j.jembe.2015.02.008
Manzello, D. P. (2010). Ocean acidification hot spots: spatiotemporal dynamics of the seawater CO2 system of eastern Pacific coral reefs. Limnol. Oceanogr. 55, 239–248. doi: 10.4319/lo.2010.55.1.0239
Maximino, C., de Brito, T. M., da Silva Batista, A. W., Herculano, A. M., Morato, S., and Gouveia, A. (2010). Measuring anxiety in zebrafish: a critical review. Behav. Brain Res. 214, 157–171. doi: 10.1016/j.bbr.2010.05.031
McCormick, M. I., and Meekan, M. G. (2010). The importance of attitude: the influence of behaviour on survival at an ontogenetic boundary. Mar. Ecol. Prog. Ser. 407, 173–185. doi: 10.3354/meps08583
McCormick, M. I., Watson, S.-A., and Munday, P. L. (2013). Ocean acidification reverses competition for space as habitats degrade. Sci. Rep. 3:3280. doi: 10.1038/srep03280
McElhany, P., and Busch, S. D. (2013). Appropriate pCO2 treatments in ocean acidification experiments. Mar. Biol. 160, 1807–1812. doi: 10.1007/s00227-012-2052-0
McLeod, I. M., McCormick, M. I., Munday, P. L., Clark, T. D., Wenger, A. S., Brooker, R. M., et al. (2015). Latitudinal variation in larval development of coral reef fishes: implications of a warming ocean. Mar. Ecol. Prog. Ser. 521, 129–141. doi: 10.3354/meps11136
McMahon, S. J., Donelson, J. M., and Munday, P. L. (2018). Food ration does not influence the effect of elevated CO2 on antipredator behaviour of a reef fish. Mar. Ecol. Prog. Ser. 586, 155–165. doi: 10.3354/meps12397
Miller, G. M., Watson, S.-A., Donelson, J. M., McCormick, M. I., and Munday, P. L. (2012). Parental environment mediates impacts of increased carbon dioxide on a coral reef fish. Nat. Clim. Chang. 2, 858–861. doi: 10.1038/nclimate1599
Moyano, M., Illing, B., Peschutter, P., Huebert, K. B., and Peck, M. A. (2016). Thermal impacts on the growth, development and ontogeny of critical swimming speed in Atlantic herring larvae. Comp. Biochem. Physiol. Part A Mol. Integr. Physiol. 197, 23–34. doi: 10.1016/j.cbpa.2016.02.020
Munday, P. L., Cheal, A. J., Dixson, D. L., Rummer, J. L., and Fabricius, K. E. (2014). Behavioural impairment in reef fishes caused by ocean acidification at CO2 seeps. Nat. Clim. Chang. 4, 487–492. doi: 10.1038/nclimate2195
Munday, P. L., Crawley, N. E., and Nilsson, G. E. (2009a). Interacting effects of elevated temperature and ocean acidification on the aerobic performance of coral reef fishes. Mar. Ecol. Prog. Ser. 388, 235–242. doi: 10.3354/meps08137
Munday, P. L., Donelson, J. M., Dixson, D. L., and Endo, G. G. K. (2009b). Effects of ocean acidification on the early life history of a tropical marine fish. Proc. R. Soc. B Biol. Sci. 276, 3275–3283. doi: 10.1098/rspb.2009.0784
Munday, P. L., Dixson, D. L., McCormick, M. I., Meekan, M., Ferrari, M. C. O., and Chivers, D. P. (2010). Replenishment of fish populations is threatened by ocean acidification. Proc. Natl. Acad. Sci. U.S.A. 107, 12930–12934. doi: 10.1073/pnas.1004519107
Munday, P. L., Gagliano, M., Donelson, J. M., Dixson, D. L., and Thorrold, S. R. (2011). Ocean acidification does not affect the early life history development of a tropical marine fish. Mar. Ecol. Prog. Ser. 423, 211–221. doi: 10.3354/meps08990
Munday, P. L., Kingsford, M. J., O’Callaghan, M., and Donelson, J. M. (2008). Elevated temperature restricts growth potential of the coral reef fish Acanthochromis polyacanthus. Coral Reefs 27, 927–931. doi: 10.1007/s00338-008-0393-4
Munday, P. L., Welch, M. J., Allan, B. J. M., Watson, S.-A., McMahon, S. J., and McCormick, M. I. (2016). Effects of elevated CO2 on predator avoidance behaviour by reef fishes is not altered by experimental test water. PeerJ 4:e2501. doi: 10.1101/050062
Murray, C., Malvezzi, A., Gobler, C., and Baumann, H. (2014). Offspring sensitivity to ocean acidification changes seasonally in a coastal marine fish. Mar. Ecol. Prog. Ser. 504, 1–11. doi: 10.3354/meps10791
Nagelkerken, I., and Connell, S. D. (2015). Global alteration of ocean ecosystem functioning due to increasing human CO2 emissions. Proc. Natl. Acad. Sci. U.S.A. 112, 13272–13277. doi: 10.1073/pnas.1510856112
Nagelkerken, I., and Munday, P. L. (2016). Animal behaviour shapes the ecological effects of ocean acidification and warming: moving from individual to community-level responses. Glob. Chang. Biol. 22, 974–989. doi: 10.1111/gcb.13167
Neuheimer, A. B., Thresher, R. E., Lyle, J. M., and Semmens, J. M. (2011). Tolerance limit for fish growth exceeded by warming waters. Nat. Clim. Chang. 1, 110–113. doi: 10.1038/nclimate1084
Nilsson, G. E., Crawley, N., Lunde, I. G., and Munday, P. L. (2009). Elevated temperature reduces the respiratory scope of coral reef fishes. Glob. Chang. Biol. 15, 1405–1412. doi: 10.1111/j.1365-2486.2008.01767.x
Nilsson, G. E., Dixson, D. L., Domenici, P., McCormick, M. I., Sørensen, C., Watson, S.-A., et al. (2012). Near-future carbon dioxide levels alter fish behaviour by interfering with neurotransmitter function. Nat. Clim. Chang. 2, 201–204. doi: 10.1038/nclimate1352
Nowicki, J. P., Miller, G. M., and Munday, P. L. (2012). Interactive effects of elevated temperature and CO2 on foraging behavior of juvenile coral reef fish. J. Exp. Mar. Biol. Ecol. 412, 46–51. doi: 10.1016/j.jembe.2011.10.020
Ou, M., Hamilton, T. J., Eom, J., Lyall, E. M., Gallup, J., Jiang, A., et al. (2015). Responses of pink salmon to CO2-induced aquatic acidification. Nat. Clim. Chang. 5, 950–955. doi: 10.1038/NCLIMATE2694
Perry, D. M., Redman, D. H., Widman, J. C. Jr., Meseck, S., King, A., and Pereira, J. J. (2015). Effect of ocean acidification on growth and otolith condition of juvenile scup, Stenotomus chrysops. Ecol. Evol. 5, 4187–4196. doi: 10.1002/ece3.1678
Perry, S. F., and Gilmour, K. M. (2006). Acid-base balance and CO2 excretion in fish: unanswered questions and emerging models. Respir. Physiol. Neurobiol. 154, 199–215. doi: 10.1016/j.resp.2006.04.010
Peterson, M. S., and Gilmore, R. G. (1988). Hematocrit, osmolality and ion concentration in fishes: consideration of circadian patterns in the experimental design. J. Exp. Mar. Biol. Ecol. 121, 73–78.
Pimentel, M. S., Faleiro, F., Dionisio, G., Repolho, T., Pousao-Ferreira, P., Machado, J., et al. (2014). Defective skeletogenesis and oversized otoliths in fish early stages in a changing ocean. J. Exp. Biol. 217, 2062–2070. doi: 10.1242/jeb.092635
Pistevos, J. C. A., Nagelkerken, I., Rossi, T., Olmos, M., and Connell, S. D. (2015). Ocean acidification and global warming impair shark hunting behaviour and growth. Sci. Rep. 5:16293. doi: 10.1038/srep16293
Pörtner, H. O., and Knust, R. (2007). Climate change affects marine fishes through the oxygen limitation of thermal tolerance. Science 315, 95–97. doi: 10.1126/science.1135471
R Core Team (2017). R: A Language and Environment for Statistical Computing. Vienna: R Foundation for Statistical Computing.
Riebesell, U., and Gattuso, J. P. (2015). Lessons learned from ocean acidification research. Nat. Clim. Chang. 5, 12–14. doi: 10.1038/nclimate2456
Rodgers, G. G., Donelson, J. M., McCormick, M. I., and Munday, P. L. (2018). In hot water: sustained ocean warming reduces survival of a low-latitude coral reef fish. Mar. Biol. 165:73. doi: 10.1007/s00227-018-3333-z
Schiel, D. R., Steinbeck, J. R., and Foster, M. S. (2004). Ten years of induced ocean warming causes comprehensive changes in marine benthic communities. Ecology 85, 1833–1839. doi: 10.1890/03-3107
Schmidt, M., Gerlach, G., Leo, E., Kunz, K. L., Swoboda, S., Pörtner, H. O., et al. (2017). Impact of ocean warming and acidification on the behaviour of two co-occurring gadid species, Boreogadus saida and Gadus morhua, from Svalbard. Mar. Ecol. Prog. Ser. 571, 183–191. doi: 10.3354/meps12130
Schunter, C., Welch, M. J., Ryu, T., Zhang, H., Berumen, M. L., Nilsson, G. E., et al. (2016). Molecular signatures of transgenerational response to ocean acidification in a species of reef fish. Nat. Clim. Chang. 6, 1014–1018. doi: 10.1038/NCLIMATE3087
Shaw, E. C., McNeil, B. I., and Tilbrook, B. (2012). Impacts of ocean acidification in naturally variable coral reef flat ecosystems. J. Geophys. Res. 117:C03038. doi: 10.1029/2011JC007655
Shaw, E. C., McNeil, B. I., Tilbrook, B., Matear, R., and Bates, M. L. (2013). Anthropogenic changes to seawater buffer capacity combined with natural reef metabolism induce extreme future coral reef CO2 conditions. Glob. Chang. Biol. 19, 1632–1641. doi: 10.1111/gcb.12154
Sswat, M., Stiasny, M. H., Jutfelt, F., Riebesell, U., and Clemmesen, C. (2018). Growth performance and survival of larval Atlantic herring, under the combined effects of elevated temperatures and CO2. PLoS Biol. 13:e0191947. doi: 10.1371/journal.pone.0191947
Stewart, A., Wu, N., Cachat, J., Hart, P., Gaikwad, S., Wong, K., et al. (2011). Pharmacological modulation of anxiety-like phenotypes in adult zebrafish behavioral models. Prog. Neuropsychopharmacol. Biol. Psychiatry 35,1421–1431. doi: 10.1016/j.pnpbp.2010.11.035
Stiasny, M. H., Mittermayer, F. H., Sswat, M., Voss, R., Jutfelt, F., Chierici, M., et al. (2016). Ocean acidification effects on Atlantic cod larval survival and recruitment to the fished population. PLoS One 11:e0155448. doi: 10.1371/journal.pone.0155448
Strobel, A., Graeve, M., Poertner, H. O., and Mark, F. C. (2013). Mitochondrial acclimation capacities to ocean warming and acidification are limited in the Antarctic Notothenioidei Fish, Notothenia rossii and Lepidonotothen squamifrons. PLoS One 8:e68865. doi: 10.1371/journal.pone.0068865
Sundin, J., Amcoff, M., Mateos-González, F., Raby, G. D., Jutfelt, F., and Clark, T. D. (2017). Long-term exposure to elevated carbon dioxide does not alter activity levels of a coral reef fish in response to predator chemical cues. Behav. Ecol. Sociobiol. 71:108. doi: 10.1007/s00265-017-2337-x
Sundin, J., and Jutfelt, F. (2015). 9–28 d of exposure to elevated pCO2 reduces avoidance of predator odour but had no effect on behavioural lateralization or swimming activity in a temperate wrasse (Ctenolabrus rupestris). ICES J. Mar. Sci. 73, 620–632. doi: 10.1093/icesjms/fsv101
Todd, C. D., Hughes, S. L., Marshall, C. T., MacLean, J. C., Lonergan, M. E., and Biuw, E. M. (2008). Detrimental effects of recent ocean surface warming on growth condition of Atlantic salmon. Glob. Chang. Biol. 14, 958–970. doi: 10.1111/j.1365-2486.2007.01522.x
Wahl, M., Saderne, V., and Sawall, Y. (2016). How good are we at assessing the impact of ocean acidification in coastal systems? Limitations, omissions and strengths of commonly used experimental approaches with special emphasis on the neglected role of fluctuations. Mar. Freshw. Res. 67, 25–36. doi: 10.1071/MF14154
Wahl, M., Schneider Covachã, S., Saderne, V., Hiebenthal, C., Müller, J. D., Pansch, C., et al. (2018). Macroalgae may mitigate ocean acidification effects on mussel calcification by increasing pH and its fluctuations. Limnol. Oceanogr. 63, 3–21. doi: 10.1002/lno.10608
Waldbusser, G. G., and Salisbury, J. E. (2014). Ocean acidification in the coastal zone from an organism’s perspective: multiple system parameters, frequency domains, and habitats. Ann. Rev. Mar. Sci. 6, 221–247. doi: 10.1146/annurev-marine-121211-172238
Watson, S. A., Allan, B. J. M., Mcqueen, D. E., Nicol, S., Parsons, D. M., Pether, S. M. J., et al. (2018). Ocean warming has a greater effect than acidification on the early life history development and swimming performance of a large circumglobal pelagic fish. Glob. Chang. Biol. doi: 10.1111/gcb.14290 [Epub ahead of print].
Welch, M. J., and Munday, P. L. (2017). Heritability of behavioural tolerance to high CO2 in a coral reef fish is masked by nonadaptive phenotypic plasticity. Evol. Appl. 10, 682–693. doi: 10.1111/eva.12483
Welch, M. J., Watson, S.-A., Welsh, J. Q., McCormick, M. I., and Munday, P. L. (2014). Effects of elevated CO2 on fish behaviour undiminished by transgenerational acclimation. Nat. Clim. Chang. 4, 1086–1089. doi: 10.1038/nclimate2400
Wieser, W., and Kaufmann, R. (1998). A note on interactions between temperature, viscosity, body size and swimming energetics in fish larvae. J. Exp. Biol. 201, 1369–1372.
Wittmann, A. C., and Pörtner, H. (2013). Sensitivities of extant animal taxa to ocean acidification. Nat. Clim. Chang. 3, 995–1001. doi: 10.1038/nclimate1982
Keywords: ocean acidification, ocean warming, lateralization, activity, fast starts, pH variability, CO2 fluctuations
Citation: Jarrold MD and Munday PL (2018) Elevated Temperature Does Not Substantially Modify the Interactive Effects Between Elevated CO2 and Diel CO2 Cycles on the Survival, Growth and Behavior of a Coral Reef Fish. Front. Mar. Sci. 5:458. doi: 10.3389/fmars.2018.00458
Received: 25 July 2018; Accepted: 14 November 2018;
Published: 12 December 2018.
Edited by:
Christopher Edward Cornwall, Victoria University of Wellington, New ZealandReviewed by:
Hans O. Pörtner, Helmholtz-Gemeinschaft Deutscher Forschungszentren (HZ), GermanyKai G. Schulz, Southern Cross University, Australia
Copyright © 2018 Jarrold and Munday. This is an open-access article distributed under the terms of the Creative Commons Attribution License (CC BY). The use, distribution or reproduction in other forums is permitted, provided the original author(s) and the copyright owner(s) are credited and that the original publication in this journal is cited, in accordance with accepted academic practice. No use, distribution or reproduction is permitted which does not comply with these terms.
*Correspondence: Michael D. Jarrold, bWljaGFlbC5qYXJyb2xkQG15LmpjdS5lZHUuYXU=