- 1Institute of Marine Research, Matredal, Norway
- 2Uni Research Environment, Bergen, Norway
The fish gill is subject to an osmorespiratory compromise in physiologically demanding situations where conditions that favor gas exchange may compromise osmotic balance, especially when large gradients between the blood and the aquatic environment are present. Fish in isosmotic water should therefore be less restricted by an osmorespiratory compromise, which should improve aerobic performance. To investigate this hypothesis, Atlantic salmon were acclimated for minimum 3 weeks to freshwater, near isosmotic brackish water or sea water, and tested in groups of 10 in a large swim tunnel respirometer to assess metabolic rates, swimming capacity and hematological parameters. Oxygen uptake rates and the critical swimming speed were similar between treatments. However, osmolality and plasma [ions] before and after swim trials, and subsequent recovery differed. Fish in sea water experienced a substantially larger osmotic disturbance in the swim trials, which had increased further 3 h post-fatigue, while fish in lower salinities were approaching full recovery. Swim trials increased plasma cortisol levels, which may modulate increased gas transfer and facilitate beneficial ion regulation in both low and high salinities. Swimming also increased hematocrit and hemoglobin concentration that returned to control levels after recovery, suggesting recruitment of erythrocytes via splenic contraction. These results show that Atlantic salmon do not elicit a clear salinity optimum in terms of metabolic and locomotory advantages. Although, swimming in sea water imposes larger osmoregulatory challenges which may have implications for repeated swim challenges. Hence, Atlantic salmon are well-equipped to minimize the potential restrictions of an osmorespiratory compromise on aerobic performance, and more so in brackish and freshwater.
Summary Statement
Metabolic rates and swimming capacity were similar in Atlantic salmon acclimated to different salinities. However, osmotic disturbances from swimming differed substantially with salinity where seawater imposed greater osmoregulatory challenges.
Introduction
Fish have adapted to an aquatic existence over a wide range of salinities, spanning from freshwater lakes to hypersaline estuaries, where some species are stenohaline, while others are euryhaline (Nelson, 2016). Through the gills fish are in close contact with the surrounding water, which often differs substantially from their blood plasma in ion concentrations. Therefore, fish need to continuously osmoregulate to maintain proper water and salt balance. In freshwater, fish are hyperosmotic to their environment and rely on active branchial uptake of Na+ and Cl− from the external water while producing large volumes of dilute urine (Krogh, 1937; Marshall, 2002). In sea water, however, fish are hypoosmotic to their environment where Na+ and Cl− are actively excreted at the gills while water is absorbed over the intestines (Keys, 1931; Marshall, 2002).
It has been attempted to quantify the metabolic costs of osmoregulation in several species of fish, and estimates range from above 30% to only a few per cent of resting metabolic rates (reviewed by Ern et al., 2014). Theoretically, these energetic requirements should be minimized in brackish water where the osmotic gradient between the blood and the surrounding environment is reduced. Among the species investigated so far, no obvious pattern between O2 and salinity has been found, although most studies report lowest
O2 at the salinity they typically occupy (Ern et al., 2014). However, some euryhaline species do show reduced metabolic rates in near isosmotic water (e.g., Farmer and Beamish, 1969; Nordlie, 1978; Gaumet et al., 1995), and these energy savings may be associated with improved growth conditions (Gaumet et al., 1995; Boeuf and Payan, 2001).
The fish gill is a multifunctional organ responsible for gas exchange, osmoregulation, acid-base balance and excretion of nitrogenous waste (Evans et al., 2005). Conditions that favor gas exchange such as high surface areas, reduced diffusion distances and increased blood flow can be detrimental to osmoregulation when large ionic concentration gradients are present between the blood and the surrounding water. Conversely, the maximum rate of gas exchange may be compromised when osmoregulation is prioritized. Hence, the fish gill is subject to an osmorespiratory compromise in situations that challenges the physiological capacity of either function (Sardella and Brauner, 2007). The osmorespiratory compromise is therefore a key concept in understanding the physiological constraints of fish performance in general and under specific environmental challenges such as hypoxia, and changes in temperature and salinity (Wood and Randall, 1973a; Nilsson and Sundin, 1998; Sardella and Brauner, 2007; Iftikar et al., 2010). For instance, in FW-acclimated rainbow trout (Oncorhynchus mykiss), high intensity swimming caused Na+ efflux to increase by 70% through passive diffusion together with an increase in net branchial water entry (Wood and Randall, 1973a,b). In SW, the osmorespiratory compromise during exercise may similarly be inferred from opposing effects such as an increase in plasma Na+ and Cl− concentrations (Brauner et al., 1992; Wagner et al., 2006; Hvas et al., 2017b).
The maximum metabolic rate (MMR) is the highest rate of aerobic metabolism and resting metabolic rate, termed standard metabolic rate (SMR) in fish and other ectotherms, correspond to the minimum energy required to maintain basal homeostasis. The difference between these is termed the aerobic scope (AS), and is a useful capacity measure of how well fish will perform in specific environmental conditions (Priede, 1985; Claireaux and Lefrancois, 2007). Since high rates of branchial oxygen uptake are associated with an osmotic disturbance in both freshwater and sea water, AS may be restricted in suboptimal salinities. Also, AS may be higher at salinities where the energetic requirements of osmoregulation are minimized.
Interestingly, only a few studies have systematically measured metabolic rates of fish swimming at varying intensities in different salinities (Rao, 1968; Farmer and Beamish, 1969; Febry and Lutz, 1987; Wagner et al., 2006). These have not revealed whether AS and swimming capacity elicits a salinity dependent optimum. However, in two species of tilapia, oxygen uptake were found to be lower in near isosmotic salinities at a range of steady swimming intensities (Farmer and Beamish, 1969; Febry and Lutz, 1987). This suggests that the osmorespiratory compromise is less restricting in brackish water which may improve the aerobic capacity.
Atlantic salmon (Salmo salar) is a suitable model organism to study osmorespiratory compromise related to salinity and aerobic performance, since it is a euryhaline fish that migrates between freshwater and sea water during its lifecycle. After smoltification, Atlantic salmon and other salmonids efficiently acclimate to different salinities within days by altering ion-transport properties of epithelium in the gills and the gut, where the state of acclimation can be inferred from changing levels of branchial Na+-K+-ATPase (NKA) activity activity (McCormick and Saunders, 1987; Morgan and Iwama, 1998). In addition, Atlantic salmon and other salmonids are fast long-distance swimmers with high aerobic capacities (Farrell, 2007; Hvas and Oppedal, 2017).
The purpose of the present study was to reveal whether Atlantic salmon post-smolts have an optimum salinity with regards to metabolic scope and swimming capacity, and if such observations could be explained by the nature of exercise induced osmotic disturbances in accordance with an osmorespiratory compromise. To achieve this, swim tunnel respirometry was performed on groups of Atlantic salmon acclimated to either (SW, 34.7 ppt), near isosmotic brackish water (BW, 11 ppt) or freshwater (FW, 0.2 ppt). Here, rates of oxygen uptake (O2) were used as a proxy of aerobic metabolism and measured at stepwise increasing activity levels until critical swimming speed (Ucrit) was reached, at the point of fatigue. In addition, blood and gill samples were taken at rest, fatigue and 3 h recovery time points for the measurement of plasma ions, lactate, cortisol, glucose, hematological parameters and NKA activity. It was hypothesized that AS and Ucrit would be highest in near isosmotic BW owing to osmoregulatory advantage.
Materials and Methods
Animals and Acclimation
After smoltification, hatchery-reared Atlantic salmon were maintained in the Tank Environmental Laboratory facilities at the Institute of Marine Research, Matre, Norway. Large tanks (5.3 m3) in an open water system with a continuous inflow of 120 l min−1 were used, which ensured high oxygen levels and prevented waste products from accumulating. Through custom software (SD Matre software, Normatic, Nordfjordeid, Norway), temperature was set at 13°C, the light regime was a simulated natural photoperiod, and feed (Nutra, 3 mm pellet size, Skretting, Norway) was given daily in excess through automatic feeding devices from 9:00 to 13:00 h.
The fish were kept in full strength SW of 34.7 ppt for 5 months prior to experimentation and acclimation to other salinities. SW was supplied from 90 m depth in the nearby fjord, where the year round temperature is stable at ∼9°C, and was filtered and UVC treated. Part of this ambient water supply was either warmed up to 20°C or cooled to 2°C in separate reservoirs such that desired temperatures in fish tanks could be achieved by automatic mixing via the custom software. In addition, after thermal mixing the water was aerated in a header tank to ensure that individual fish tanks received water of a constant quality.
Approximately 150 fish were moved to new tanks with either FW or BW with corresponding salinities of 0.2 or 11 ppt, respectively. Here, they were acclimated for 3 weeks before swim trial experiments. FW used for FW and BW water qualities was supplied from two nearby rivers, filtered and UVC treated, buffered with SW from an initial conductivity of ∼100 mS to 1200 mS, and divided into separate thermal reservoirs in a similar way as to the SW supply.
Brackish water was made by controlled mixing of SW and FW supplied from both ambient and heated reservoirs, which caused some cyclical fluctuations in water parameters in the header tank. However, water parameters remained stable within the BW fish tanks, which were confirmed by CTD measurements prior to acclimation procedures (SD 208 – CTD, SAIV A/S Environmental Sensors & Systems, Norway).
The experiments in the present study were performed in March and April 2017 under permit number 9776 in accordance with the Norwegian laws and regulations for procedures on live animals.
Swim Tunnel Setup
A large custom-built Brett-type swim tunnel respirometer specifically designed to assess natural swimming behavior in groups of larger salmonids was used in this study. This setup has been thoroughly described (Remen et al., 2016; Hvas et al., 2017a). Briefly summarized, the swim section of the tunnel was 248 cm long with an internal diameter of 36 cm. At the rear end, the top lid could be easily removed for accessing the swim section, while a camera and an oxygen sensor (RINKO ARO-FT, JFE Advanced Co., Japan) were deployed behind the rear grid to observe undisturbed fish behavior and to measure O2 of the fish, respectively. Desired water currents were generated with a motor-driven propeller (Flygt 4630, 11° propeller blade, Xylem Water Solutions Norge AS, Nesttun, Norway) controlled by a frequency converter ((ITT Monitoring and Control, Norway), after calibration of current velocities with a Doppler velocimeter (Vectrino Lab Velocimeter, Nortek AS, Rud, Norway). Water was provided from the same header tanks used for the holding tanks through a wide hose connected via an adjustable inlet, which allowed for controlled flushing of the setup. Owing to cyclical fluctuations in temperature and salinity in the BW header tank, it was necessary to setup an additional water reservoir to ensure stable water parameters when supplying water to the swim tunnel in the BW trials.
Experimental Protocols
In each swim trial, 10 fish were gently netted from the holding tanks and quickly moved to the swim tunnel setup. Here they were allowed to acclimate to the experimental environment overnight at a modest current velocity of 15 cm s−1 corresponding to ∼0.5 body lengths s−1. Fish were not fed during this acclimation period to avoid confounding metabolic effects from digestion in the swim trials. The following day, current velocity was increased incrementally by 15 cm s−1 every 30 min until all fish reached fatigue. Fatigue was defined as when fish were no longer able to remove themselves from the rear grid of the swim tunnel, even when encouraged to do so with tactile stimulation. Time of fatigue was recorded and individual fish were then quickly removed from the swim tunnel through the opening at the rear end, ensuring that minimum interference was made with remaining fish that were still swimming.
Fatigued fish were either stunned with a blow to the head or moved to a recovery tank in an alternating order. In stunned fish, a 2 ml blood sample was immediately drawn from the caudal vein with a heparinized syringe and momentarily stored on ice. Then, weight (W) and fork length (Lf) was recorded.
After 3 h in the recovery tank, fish were lightly sedated in 5 mg l−1 metomidate hydrochloride (AquaCalm vet., Scanvacc, Norway) within the tanks to minimize handling stress, whereafter they were netted without resistance. As in fatigued fish, blood samples were drawn from the caudal vein with a heparinized syringe, and W and Lf was recorded. Four replicate swim trials were performed for each salinity treatment, which provided 20 blood samples of fish in both a fatigue and a recovery condition for each salinity. Furthermore, for each salinity treatment, 20 fish were sampled directly from the holding tanks in a similar way to recovery groups to provide controls. Finally, in all experimental fish, a segment of the second right gill arch was dissected out, cleaned for blood, and stored in a SEI buffer (pH of 7.3) at −80°C for later analyses of NKA activity.
Calculation of Oxygen Uptake and Critical Swimming Speed
During swim trials, the setup was kept closed off in the first 20 min of any given current velocity, and subsequently flushed for the remaining 10 min, ensuring that oxygen levels never dropped below 90% saturation. O2 could then be calculated from the linear decrease in O2 in the water during closed periods for each swimming speed using the formula:
Where ΔO2/Δt is the change in oxygen over time, Vsys is the volume of the system, while Vb and Wb are the volume and weight of the fish where a density of 1 kg l−1 is assumed. SMR was estimated by fitting O2 as an exponential function of swimming speed, and extrapolating back to a theoretical swimming speed of zero (Brett, 1964; Beamish, 1978; Hvas et al., 2017a).
The MMR was defined as the highest O2 measured while all fish still remained in the swim tunnel. This corresponded to the swimming speed just before or when fish started to become fatigued, meaning that MMR occasionally was based on a reduced measurement period. After removal of the first fatigued fish, the remaining fish would either fatigue later at the same swimming speed, or at the beginning of the next increment, meaning that the reported MMR should be a representative group average of the 10 fish tested in each trial (Hvas et al., 2017a). The AS could then be calculated as the difference between MMR and SMR.
Prolonged swimming capacity of individual fish was expressed as Ucrit, defined by Brett (1964), and calculated as:
Where Uf is the last completed current velocity, tf is the time spent at the current velocity where fatigue was reached, ti is the time spent at each velocity and Ui is the magnitude of the velocity increment. Since individual fish tested had a small cross sectional area relative to the large swim tunnel (< 3%), and since the fish generally were evenly spread out in the tunnel during swimming, solid blocking effects were not corrected for Bell and Terhune (1970).
Hematological Analyses
Within minutes following blood sampling, the haematocrit (Hct) was measured in duplicates as the fraction of red blood cells after small subsamples were centrifuged in capillary tubes in a haematocrit centrifuge (StatSpin MP Centrifuge). Concentration of hemoglobin (Hb) was measured in 10 μl of blood with an assay kit (MAK115, Sigma-Aldrich). The mean corpuscular hemoglobin concentration (MCHC) could then be calculated as [Hb] divided by the fractional Hct.
At the same time, 1.5 mL blood was centrifuged for 5 min at 3000 g in Eppendorf tubes. The blood plasma was then stored at −80°C for later analyses of ions and stress parameters.
The concentrations of Na+, K+, and Cl− in the plasma samples were measured using ion selective electrodes in a Cobas 9180 electrolyte analyzer (Roche Diagnostics). Osmolality was measured via freeze point determination with a Fiske 210 Micro-Sample Osmometer (Advanced Instruments). Lactate and glucose concentrations were measured spectrophotometrically with MaxMat PL (MaxMat), and cortisol was quantified with an ELISA assay kit (IBL International GmbH) and a Sunrise microplate reader (Tecan).
The Na+/K+ ATPase Activity
Gill NKA activity was determined by the method of McCormick (1993). Briefly, this kinetic assay is enzymatically coupled to the conversion of NADH to NAD+ by pyruvate kinase and lactic dehydrogenase with or without the addition of ouabain, a specific inhibitor of NKA. Reduction of NADH was measured spectrophotometrically at 340 nm over a period of 10 min at 25°C using a Tecan Sunrise 96 well microplate reader. Protein in homogenate was determined by a bicinchoninic acid method (Smith et al., 1985), and NKA activity was expressed as mM ADP μg protein−1 h−1.
Statistics
A one-way ANOVA was used to test for differences between salinity treatments in O2, Ucrit, and to test for differences in hematological parameters and NKA activity between sampling conditions within each salinity treatment. A Tukey’s post hoc test was used to determine which groups or sampling points differed. In some cases, the data did not show equal variance from Levene’s test, even after a log transformation, in which case an ANOVA on ranks was used instead. Statistical analyses and figures were made with Sigmaplot 12.3, Systat Software. Significance level was set to 0.05 and data are presented as means ± s.e.m.
Results
The osmolality and concentrations of Na+, Cl−, and K− in the water of the different salinity treatments are summarized in Table 1. During the 1st week of acclimation to FW the fish were not observed to eat, but in the 2nd week they showed renewed interest in feed, and appetite was back to normal in the 3rd week. No change in appetite was observed while acclimating to BW. Fish sizes were similar between treatments, with weights of ∼400 g and Lf of ∼34 cm at the time of the swim trials (Table 2).
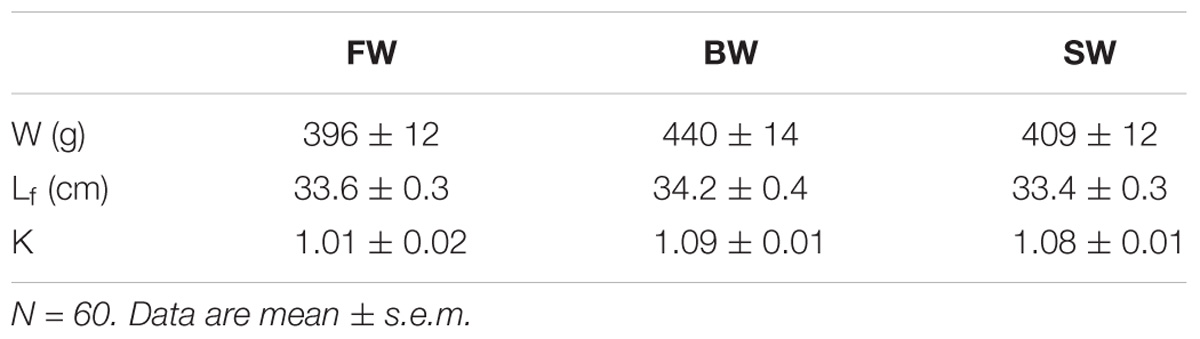
TABLE 2. Weight (W), fork length (Lf), and condition factor (K) of experimental fish acclimated to different salinities.
Oxygen Uptake Rates and Swimming Capacity
SMR and MMR were not statistically different between salinity treatments (Figure 1A). However, in SW, the SMR was slightly higher while MMR was slightly lower compared to the other treatments. This resulted in an AS of 400 ± 4 mg O2 kg−1 h−1 in SW that was statistically lower compared to in the other salinity treatments, where AS was 436 ± 5 and 445 ± 11 mg O2 kg−1 h−1 in FW and BW, respectively (Figure 1A). O2 was statistically identical between salinity treatments at all measured swimming speeds (Figure 1B). The Ucrit was 2.80 ± 0.08, 2.83 ± 0.04, and 2.78 ± 0.04 body lengths s−1 in FW, BW, and SW, respectively (Figure 1C). These values were statistically identical.
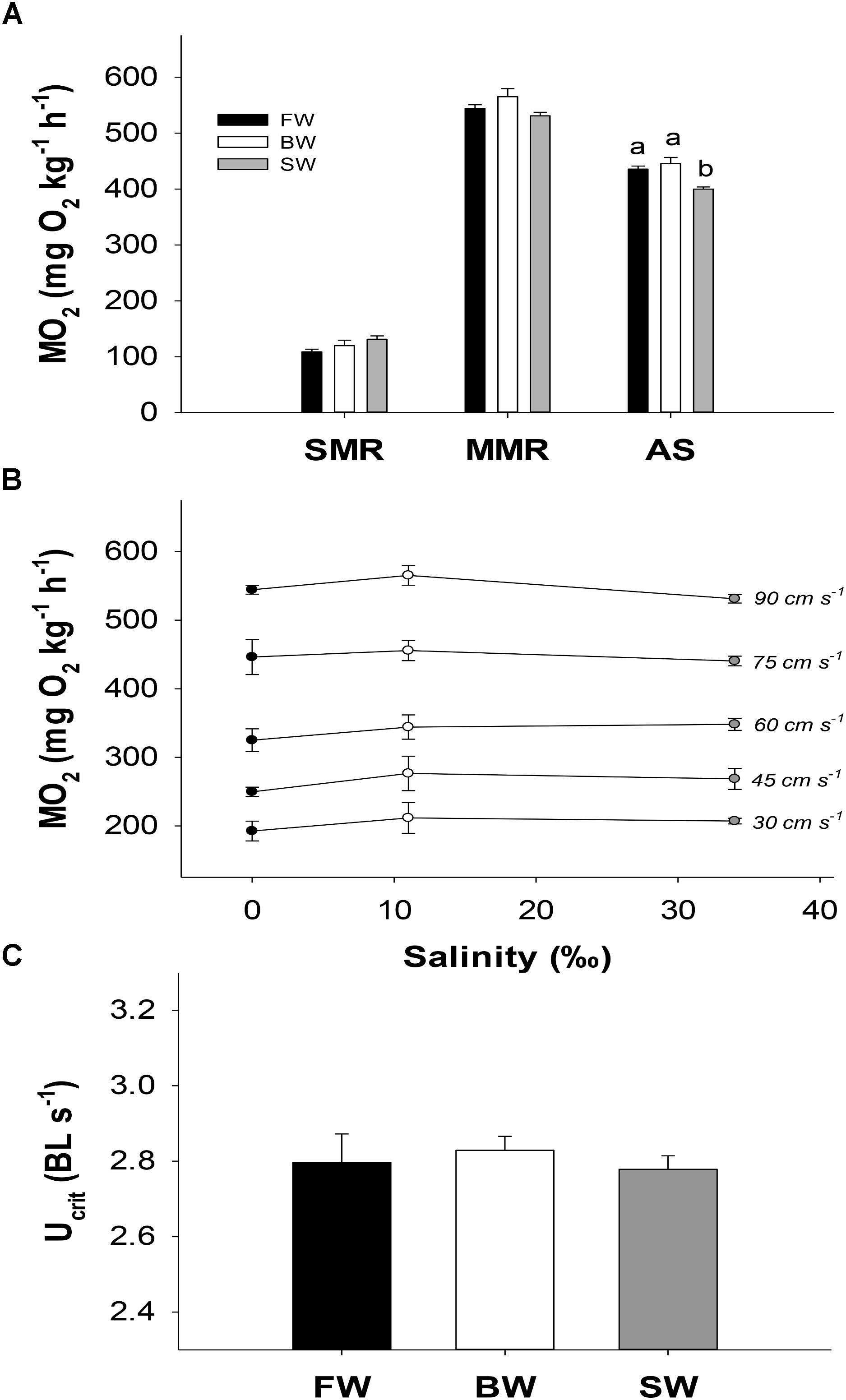
FIGURE 1. Metabolic rates and swimming performance in different salinities. SMR, Standard metabolic rate; MMR, maximum metabolic rate; and AS, aerobic scope (A), oxygen uptake rate (O2) in different swimming speeds (B), and the critical swimming speed (Ucrit) in body lengths s−1 (C). Different letters indicate significant differences between groups. Data are mean ± s.e.m.
Plasma Ions
Exercising to fatigue caused plasma osmolality to increase significantly regardless of salinity treatment, and this increase was highest in SW (Δ51 mOsm kg−1), intermediate in BW (Δ40 mOsm kg−1) and lowest in FW (Δ26 mOsm kg−1). A further 3 h of recovery increased osmolality in SW, while it decreased in the other groups, although this decrease was only significant in FW. Fish in SW showed an elevated osmolality compared to the other salinities at all sampling points. This was most notable during recovery, when plasma osmolality was 438 ± 8 mOsm kg−1 in SW, compared to only 334 ± 5 and 350 ± 5 mOsm kg−1 in FW and BW, respectively (Figure 2A).
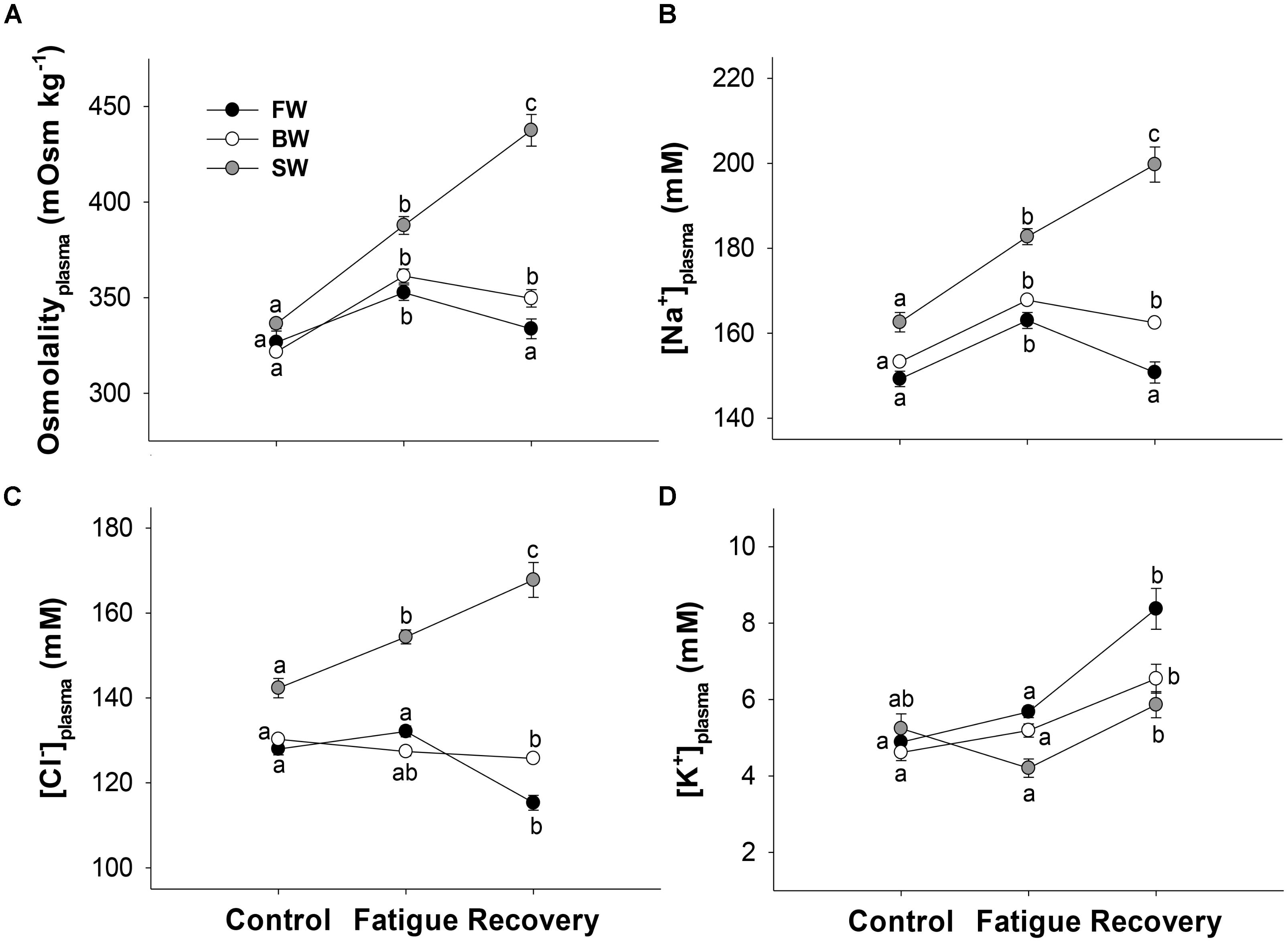
FIGURE 2. Plasma osmolality (A), sodium (B), chloride (C), and potassium (D) at different salinities and sampling times. Control, fatigue, and recovery refer to measurements taken directly from the holding tanks, at Ucrit, and following 3 h of rest, respectively. Different letters indicate significant differences between sampling conditions within a specific salinity group. Data are mean ± s.e.m.
Similar statistical patterns for plasma osmolality were seen for plasma [Na+] (Figure 2B). Exercising to fatigue in SW increased plasma [Cl−] significantly, and a further increase was seen after 3 h of recovery. However, in FW and BW plasma [Cl−] remained statistically similar between controls and fatigued fish, and recovery caused [Cl−] to significantly decrease in FW and BW compared to controls (Figure 2C). Fish in SW showed elevated [Cl−] and [Na+] compared to the other salinities in all sampling conditions (Figures 2D,C).
Plasma [K+] did not follow similar patterns to that of osmolality, [Na+] and [Cl−]. Instead [K+] was statistically similar between controls and fatigued fish regardless of salinity treatment, while recovery caused a significant increase in [K+] of 2.7, 1.4, and 1.7 mM in FW, BW, and SW, respectively, compared to in fatigue condition (Figure 2D).
The Na+/K+ ATPase Activity
Overall, NKA activity was highest in SW, intermediate in BW and lowest in FW regardless of sampling condition (Figure 3). In FW, the NKA activity remained unaffected by exercise and subsequent recovery with a pooled average value of 5.7 ± 0.5 μm ADP mg protein−1 h−1. In BW, exercising to fatigue caused NKA activity to decrease significantly from 10.7 ± 0.8 to 7.3 ± 1.0 μm ADP mg protein−1 h−1. Subsequent recovery caused NKA activity to increase again to an intermediate value of 9.0 ± 1.1 5 μm ADP mg protein−1 h−1. An opposite pattern was measured in SW acclimated fish, where exercising to fatigue significantly increased NKA activity from 12.8 ± 0.7 to 17.5 ± 1.5, where it remained elevated at similar levels after 3 h of recovery.
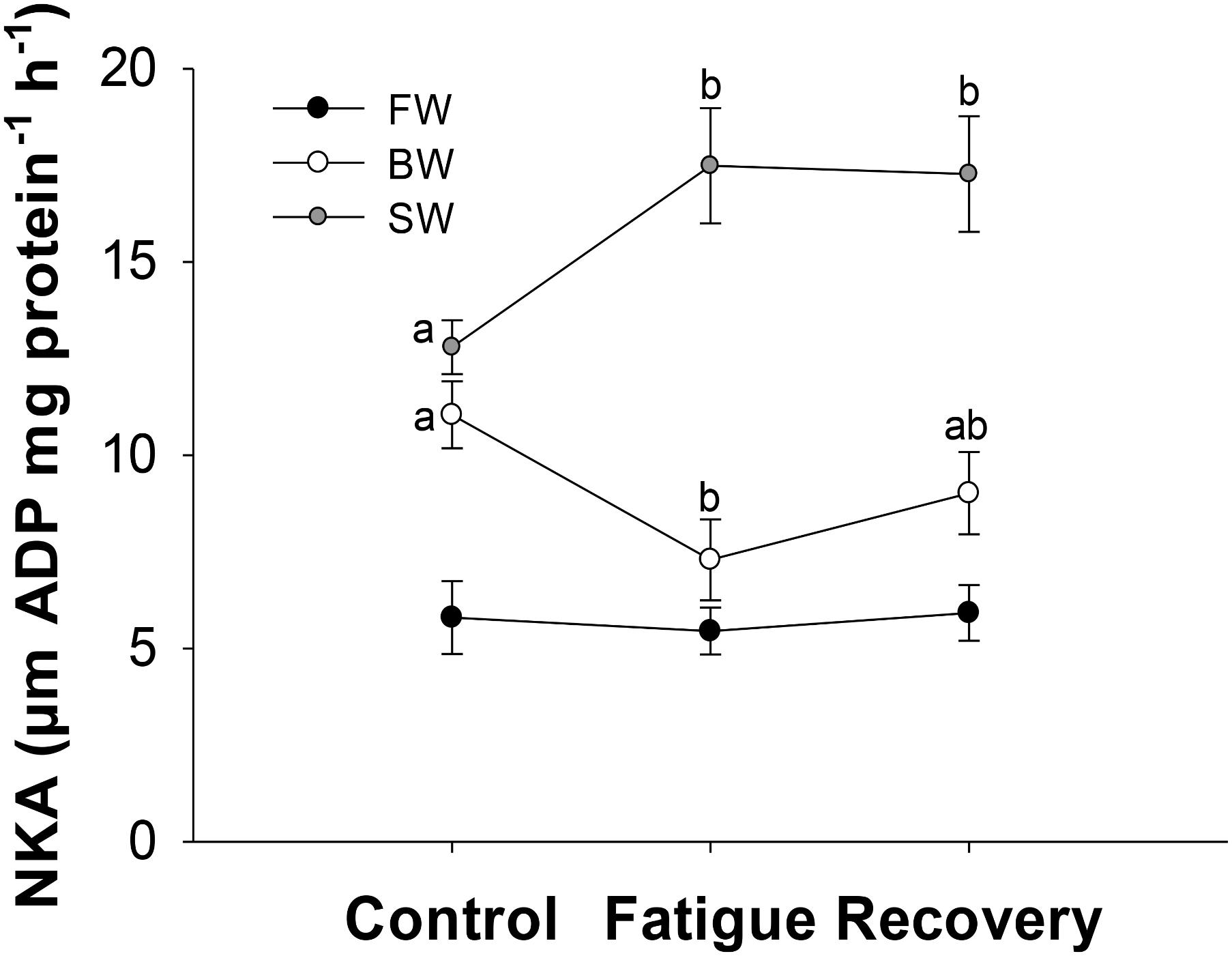
FIGURE 3. Na+/K+ ATPase activity (NKA) at different salinities and sampling conditions. Different letters indicate significant differences between sampling conditions within a specific salinity group. Data are mean ± s.e.m.
Lactate, Cortisol and Glucose Concentrations
Plasma lactate was significantly higher in fatigued fish compared to controls, and increased further at 3 h post-fatigue in all salinity treatments. However, this additional post-exercise increase was only significant in SW and FW (Figure 4A). Plasma cortisol in fatigued fish was 392, 452, and 349 ng ml−1 higher compared to controls in FW, BW, and SW, respectively. After recovery, cortisol was still similarly elevated in FW and SW, while it had decreased significantly to an intermediate value in BW (Figure 4B). Plasma glucose increased significantly with exercise and was still elevated at similar values 3 h post-fatigue in all salinity treatments (Figure 4C).
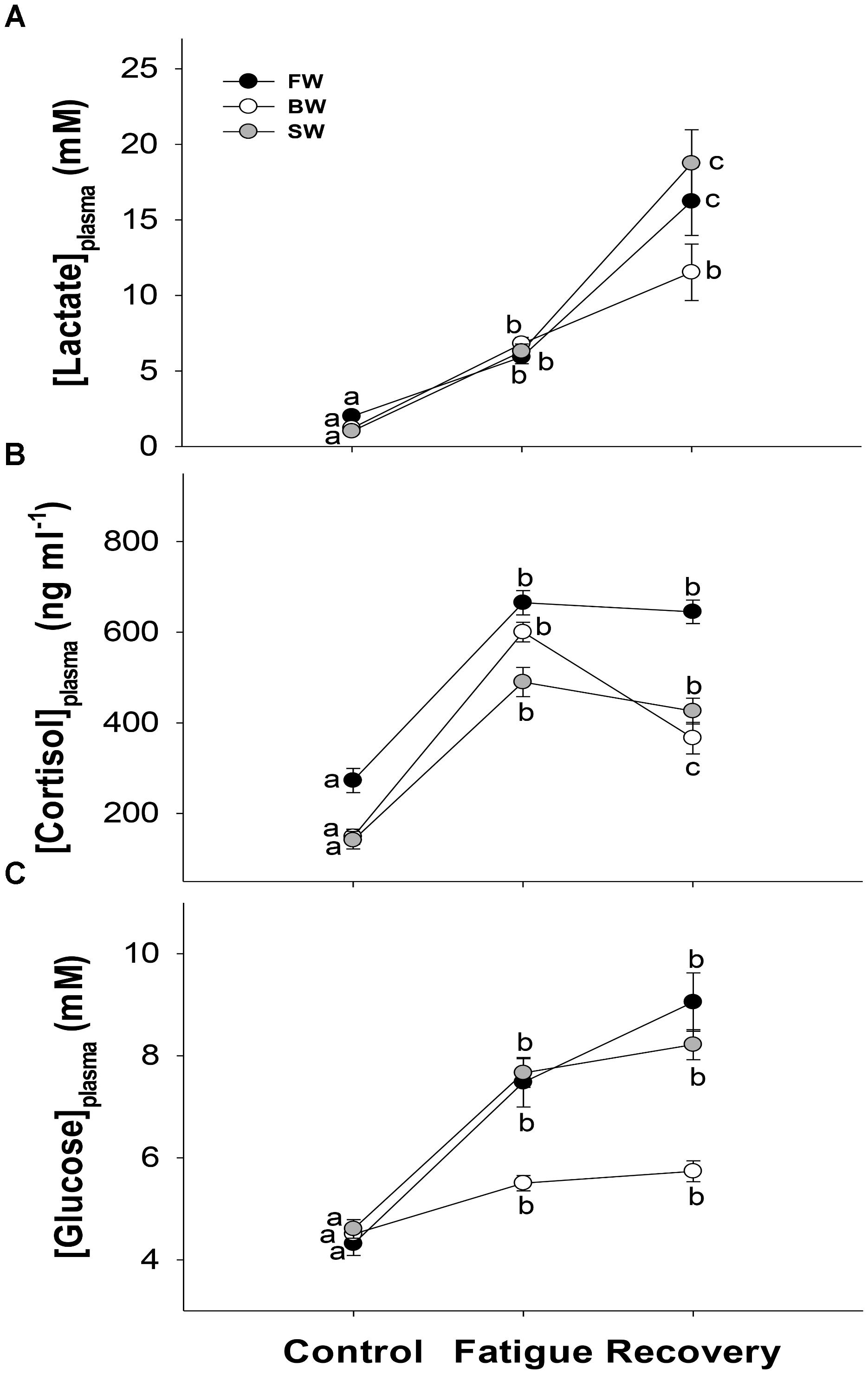
FIGURE 4. Stress parameters. Plasma lactate (A), cortisol (B), and glucose (C) in controls, after fatigue and following 3 h of recovery at different salinity acclimations. Different letters indicate significant differences between sampling conditions within a specific salinity group. Data are mean ± s.e.m.
Haematocrit and Hemoglobin
Swimming to exhaustion increased Hct significantly by 7.3, 7.6, and 6.7% in FW, BW, and SW, respectively. A subsequent 3 h of recovery, decreased Hct to statistically similar levels as the control fish in all salinity treatments (Figure 5A). The same statistical pattern between controls, fatigue and recovery was also seen for [Hb] (Figure 5B). Consequently, MCHC remained unaffected by exercising and recovery in all treatment groups (Figure 5C). However, MCHC appeared higher in SW owing to slightly lower Hct and slightly higher [Hb], which indicates some general shrinkage of red blood cells in sea water relative to in the other salinities.
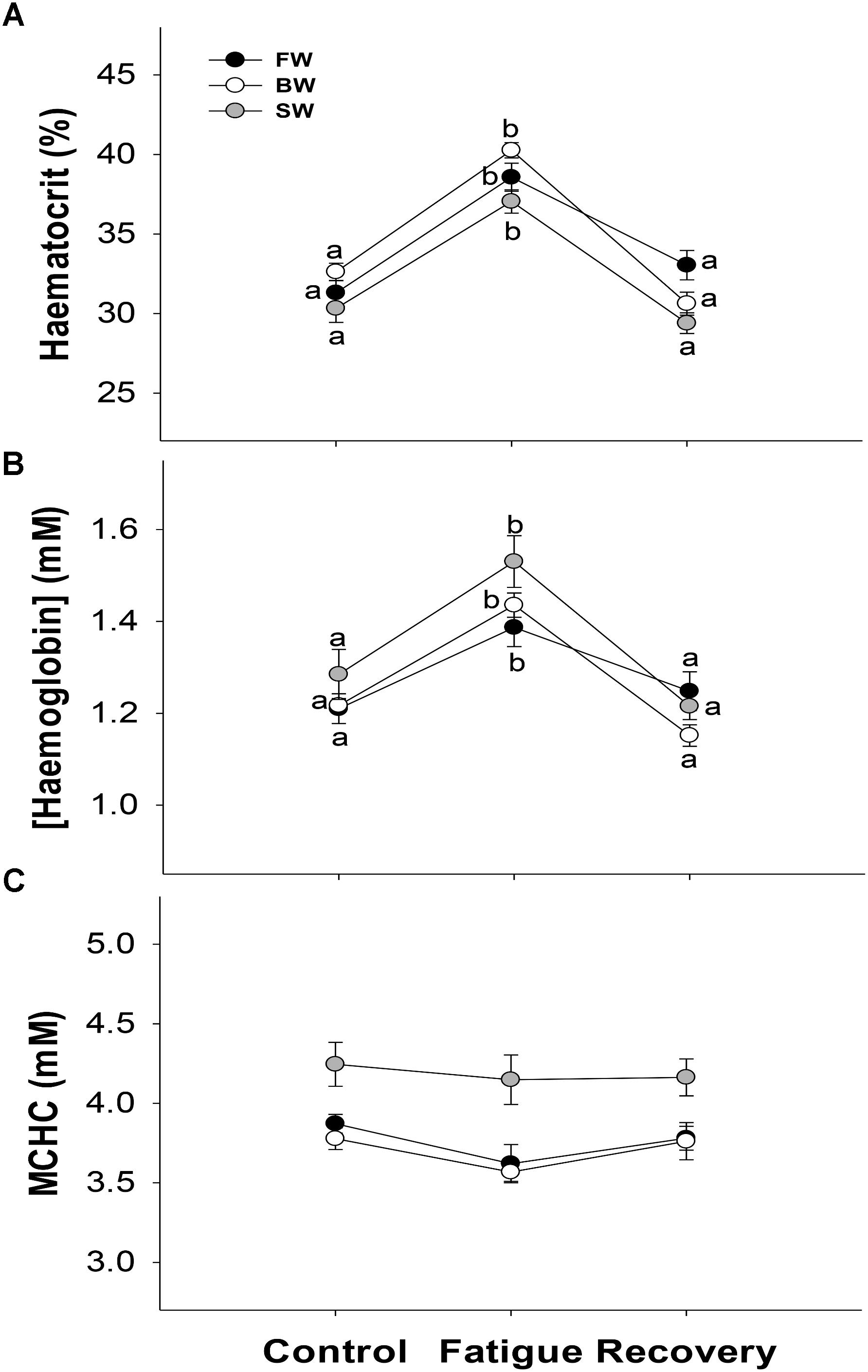
FIGURE 5. Hematological parameters. The haematocrit (A), hemoglobin concentration (B), and mean corpuscular hemoglobin concentration (MCHC) (C) in controls, after fatigue and following 3 h of recovery at different salinity acclimations. Different letters indicate significant differences between sampling conditions within a specific salinity group. Data are mean ± s.e.m.
Discussion
Salinity Effects on Metabolic Rates and Critical Swimming Speed
From the assumption that minimum energy is required for osmoregulation in near isosmotic BW, it was expected that SMR should be reduced in these conditions. With statistically similar SMR between all three salinity groups, this hypothesis was not confirmed. The metabolic requirements for osmoregulation must therefore be a fairly minor component of SMR in Atlantic salmon due to the similar values found in fish acclimated to FW, near isosmotic BW and SW.
In another salmonid, O. clarkii, O2 was measured in isolated gill tissues from both SW and FW acclimated fish, for a direct measurement of the energetic costs of branchial osmoregulation, where it was estimated to be less than 4% of whole-animal
O2 in both salinities (Morgan and Iwama, 1999). The cost of osmoregulation therefore appears to be a small part of the total energy budget in properly acclimated salmonids in general. Furthermore, such subtle differences will be difficult to detect with whole-animal
O2 measurements.
High intensity swimming is associated with osmotic disturbances in both FW and SW in salmonids (e.g., Wood and Randall, 1973a; Wagner et al., 2006). Hence, while SMR appeared unaffected in the present study, the increased metabolic demand during steady swimming at increasingly higher speeds could impose salinity dependent differences in O2 that reflects different costs in preserving osmotic integrity during activity. Such patterns were seen in two species of tilapia (Farmer and Beamish, 1969; Febry and Lutz, 1987). However, this was not the case for Atlantic salmon (Figure 1C), which further corroborates that the costs of osmoregulation are a minor component of whole-animal metabolic rate in this species.
MMR may be compromised in suboptimal salinities (Swanson, 1998; Sardella and Brauner, 2007). However, the highest measured O2 in the present study did not differ significantly with salinity treatment. Although, non-significant differences in SMR and MMR added up to a significantly lower AS in SW compared to both FW and BW. Hence, while
O2 was generally found to be independent of salinity acclimation, the AS may still be slightly reduced in SW.
The Ucrit was unaffected by salinity treatment. This shows that Atlantic salmon post-smolts are able to maintain similar swimming capacities in both hyper- and hypoosmotic environments, and that reduced osmotic gradients between branchial blood and the aquatic environment does not translate into improved performance.
Therefore, these data on O2 and Ucrit do not demonstrate an optimal salinity with regards to metabolic and locomotory advantages. This adds further merit to the findings of Atlantic salmon having an impressive flexibility to cope well physiologically over a wide range of environmental conditions, which also include large seasonal fluctuations in temperature (Hvas et al., 2017a).
Salinity Effects on Osmotic Balance and Subsequent Disturbance Following Exercise
The differences in NKA activity between salinity treatments, the similarity in O2, as well as a return to normal appetite in the 3rd week for the FW fish, indicated proper salinity acclimation in all groups. A similar positive relationship between NKA activity and salinity has been reported for Atlantic salmon previously (McCormick et al., 1989; Nilsen et al., 2007, 2010), while other salmonid and non-salmonid species may show lower NKA activity in near isosmotic BW (Kültz et al., 1992; Morgan and Iwama, 1998). A salinity dependent homeostasis in ion balance was evident from the differences in plasma osmolality, [Na+] and [Cl−] in control conditions. Fish in SW showing higher plasma [Na +] and [Cl−] compared to in FW has previously been reported in some salmonids (e.g., Brauner et al., 1992; Wagner et al., 2006), while others have found no such effects at a range of salinities (e.g., McCormick et al., 1989; Morgan and Iwama, 1998). However, all these studies are difficult to compare directly owing to differences in fish size, acclimation history, direction in acclimation, and life stage. Regardless, adjusting Na+ and Cl− to lower concentrations in FW, intermediate concentrations in BW and higher concentrations in SW, as seen here for Atlantic salmon post-smolts, seems reasonable from an energetic point of view, and may aid in explaining similar SMR between treatments.
Swimming to exhaustion caused salinity dependent differences in osmolality, where fish in SW showed a greater increase in plasma [Na+] and [Cl−] compared to the lower salinity treatments. An increase in these ions was an expected consequence of swimming in SW according to the osmorespiratory compromise of the gill, since higher O2 should favor passive uptake of ions from the hyperosmotic aquatic environment. Similar increases of plasma [Na+] and [Cl−] after an exhaustive swim trial in SW has previously been reported for Coho salmon parr (O. kitsutch) and Atlantic salmon post-smolts (Brauner et al., 1992; Hvas et al., 2017b).
However, in adult sockeye salmon (O. nerka) only a slight non-significant increase in both plasma [Na+] and [Cl−] was found 30 min post-fatigue (Wagner et al., 2006). In addition, when swimming at similar speeds, O2 was higher in SW compared to in FW, and Ucrit was lower in SW (Wagner et al., 2006). This suggests these adult sockeye salmon were spending excess energy on preserving osmotic integrity during swimming in SW and that it was prioritized over maximizing swimming performance. Reduced osmoregulatory capacity in SW for mature salmonids can be explained by reduced branchial NKA activity initiated from preparation for upriver migration (Uchida et al., 1997; Wagner et al., 2006). Curiously, at this specific ontogenetic stage, salmonids are essentially acclimated to FW despite still being in SW, which consequently impair their aerobic capacities in SW.
Swimming in FW was expected to decrease osmolality through the passive loss of ions and net-gain of water. However, an increased osmolality, mainly from elevated plasma [Na+], was rather found. This suggests that Atlantic salmon are able to mitigate the osmotic challenges associated with increasing O2 in FW, and therefore are less restricted by an osmorespiratory compromise in hypoosmotic environments.
Rainbow trout also show increased plasma [Na+] after exercising in FW (Wood and Randall, 1973a; Wood et al., 1983), while ion balance was unaffected in coho salmon and sockeye salmon (Brauner et al., 1992; Wagner et al., 2006). Increased plasma [Na+] in FW may be explained by increased urination rates (Wood and Randall, 1973a,b). Furthermore, fluid shifts from exercising muscles to the blood plasma is known to occur during exhaustive swimming (Wood, 1991; Kieffer, 2000), and may explain a part of the increased plasma osmolality at fatigue regardless of salinity treatment.
Interestingly, fatigued fish in BW showed the same response as FW fish with a similar increase in plasma [Na+], but no significant change in [Cl−]. In the BW treatment, the osmolality was 322 mOsm kg−1 in the plasma of the control fish, and 327 mOsm kg−1 in the water. In addition, plasma [Na+] and [Cl−] were slightly higher in the control fish compared to in the water. The osmotic gradient between the blood and water was therefore negligible compared to in the other salinity treatments, while an active net uptake of plasma Na+ and Cl− likely occurred. Hence, the exercise induced changes in osmolality and plasma ions in BW cannot be explained by the gradient between the blood and the external water, and must therefore be caused by a combination of increased active ion transport, fluid shifts between body compartments, and increased production of dilute urine.
After a 3 h recovery period, the osmotic disturbance was approaching control values in both FW and BW, while SW fish showed a dramatic further increase in [Na+] and [Cl−]. A continuous increase in plasma ions indicate a prolonged elevation of O2 after swim trials ended in SW, which shows that physiological recovery is substantially slower in SW. This could limit Atlantic salmon in how often high intensity swimming can be performed compared to in lower salinities.
Significant changes in NKA activity were seen between sampling points for both SW and BW fish. Increased NKA activity in SW could reflect a greater need for active osmoregulation, while the decrease in BW could accommodate an opposite function. It is unclear how Atlantic salmon were able to dynamically change NKA activity during swim trials. It is unlikely new proteins were synthesized and made functional within hours, despite transient increases in gill NKA activity 3 h post-transfer to SW have been previously observed in Fundulus heteroclitus (Mancera and McCormick, 2000). Instead, changes in ion gradients and/or post-transcriptional modifications may have stimulated NKA activity (Poulsen et al., 2010), while cyclic–AMP also is known to rapidly modify NKA activity in salmonids (Tipsmark and Madsen, 2001).
Stress Response in Different Salinities
Swimming to exhaustion increased plasma lactate, cortisol and glucose in all salinity groups. This corresponds to an expected exercise-induced stress response for salmonids and other fish (Nakano and Tomlinson, 1967; Wood, 1991; Wendelaar Bonga, 1997). Hyperglycemia shows that additional energy gets mobilized to accommodate the costs of swimming. Increasing plasma lactate concentrations show that a substantial anaerobic component was required at the final swimming speeds, and that proper physiological fatigue was reached. Lactate removal is generally slow in fish, and may be even slower in the presence of cortisol (Wood, 1991; Pagnotta et al., 1994). Accordingly, lactate was further elevated in blood plasma 3 h post-fatigue, indicating continuous transport from anaerobic white muscle fibers to the blood for quite some time after the swim trials.
High lactate concentrations must have caused a severe metabolic acidosis post-fatigue in all groups, but unfortunately acid-base status was not measured in this study. The decrease in plasma [Cl−] after 3 h of recovery in FW suggests regulatory pH compensation from modulation of branchial HCO3−/Cl− transport (Marshall, 2002; Evans et al., 2005), while this mechanism may have been masked by passive Cl− uptake in the higher salinities. Also, post-fatigue lactate was higher in SW, which in conjunction with the substantially larger osmotic disturbances further suggests that exercise recovery is less efficient here relative to in lower salinities.
Cortisol increases the permeability of the gill surface epithelia, which increases gas transfer (Wendelaar Bonga, 1997). Hence, high cortisol levels during intensive swimming accommodates a higher O2, but could potentially also aid in jeopardizing osmotic integrity by a passive loss or gain of ions, depending on the salinity. However, cortisol is also known to stimulate ion regulation of the gill in both high and low salinities (Evans, 2002). Specifically, cortisol plays a role in adapting to SW by stimulating increased abundance of ion transporters in the gills of Atlantic salmon (reviewed by McCormick, 2001). Although the timeframe of the swim trials in the present study were probably too short for synthesizing new beneficial transport proteins. Opposingly, in FW rainbow trout, cortisol increases chloride cell surface area, stimulates active uptake of Na+ and Cl−, while also increasing transepithelial resistance in pavement cells (Laurent and Perry, 1990; Kelly and Wood, 2001). In FW adapted salmonids, cortisol may therefore aid in countering some of the osmotic disturbances from exercise by reducing the passive loss of ions and facilitating higher active uptake rates at the gills. Together with diuresis and fluid shifts, this further explains why plasma osmolality and [Na+] had increased in fatigued FW Atlantic salmon in the present study, when an opposite effect was expected owing to passive ion loss and net water across the gills.
Recruitment of Red Blood Cells During Exercise
Regardless of salinity treatment, the Hct and Hb increased at fatigue and then returned to control values following 3 h recovery. This is an expected response in salmonids and other fish, and is caused by contraction of the spleen, which acts as a dynamic reservoir of red blood cells (Yamamoto, 1988). The amount of erythrocytes released may correspond to an increase in Hct of 25% in rainbow trout (Kita and Itazawa, 1989), which is similar to the increase found here for fatigued Atlantic salmon. Splenic contraction is therefore an important mechanism to accommodate increased energetic requirements during exercise, since the oxygen carrying capacity of the blood gets boosted substantially. This has previously been demonstrated in rainbow trout where splenectomy reduced Ucrit (Pearson and Don Stevens, 1991).
Increased Hct following exhaustive exercise stress may also be caused by swelling of erythrocytes owing to fluid shifts from extra- to intracellular compartments (Wood, 1991; Kieffer, 2000). However, Hb changed similarly to Hct, meaning that the MCHC remained unaffected by exercise and recovery at all salinities. This suggests that red blood cell recruitment was the primary cause for an increase in Hct.
Regardless of sampling condition, SW fish tended to have higher MCHC due to both slightly lower Hct and slightly higher Hb. Hence, an apparent relative shrinkage of red blood cells probably reflects the general homeostatic condition of Atlantic salmon acclimated to SW.
Conclusion
The main hypothesis of this study was that near isosmotic BW should improve AS and Ucrit through osmoregulatory advantages in Atlantic salmon post-smolts. This hypothesis was not confirmed since FW and BW fish showed similar respiratory, locomotory and osmoregulatory capabilities. Interestingly, despite having identical O2 over a range of activity levels and similar swimming capacities as for the lower salinity treatments, SW fish experienced a substantially more severe osmotic disturbance that had increased further after a recovery period. An ecological consequence of this is that repeated high-intensity swimming will be more difficult in SW. However, during the SW phase Atlantic salmon are known to follow ocean currents in their foraging routes (Dadswell et al., 2010), meaning that swimming needs should be less demanding. On the contrary, up-river migration in low salinities likely relies on repeated usage of the full aerobic capacity. Hence, being able to better restrict osmotic disturbances during exercise may be an important adaptation for migrating Atlantic salmon in the BW to FW stage.
Author Contributions
This study was conceived and designed by all the authors. MH conducted swim tunnel experiments and hematological measurements, while TN measured NKA activity. MH analyzed the data and wrote the first draft of the manuscript, with all co-authors providing constructive and valuable input before approving the final version.
Funding
This work was funded by the Norwegian Research Council primarily through the Center for Research-based innovation in Aquaculture Technology, “Exposed” (237790) and partly through the project “Future Welfare (267800), while TN was funded through the project #237856/O30.
Conflict of Interest Statement
The authors declare that the research was conducted in the absence of any commercial or financial relationships that could be construed as a potential conflict of interest.
Acknowledgments
We would like to thank Tone Vågseth for precise monitoring of salinity and temperature in the fish tanks, and Karen Anita Kvestad for assisting in hematological analyses.
Abbreviations
AS, aerobic scope; BW, brackish water; FW, freshwater; MCHC, mean corpuscular hemoglobin concentration; MMR, maximum metabolic rate; O2, oxygen uptake rate; NKA, Na+/K+-ATPase; SMR, standard metabolic rate; SW, seawater; Ucrit, the critical swimming speed.
References
Beamish, F. W. H. (1978). “Swimming capacity,” in Fish Physiology, eds W. S. Hoar and D. J. Randall (New York, NY: Academic Press), 101–187.
Bell, W. H., and Terhune, L. D. B. (1970). Water Tunnel Design for Fisheries Research. Technical Report No. 195. Ottawa, ON: Fisheries Research Board of Canada.
Boeuf, G., and Payan, P. (2001). How should salinity influence fish growth? Comp. Biochem. Physiol. C 130, 411–423.
Brauner, C. J., Shrimpton, J. M., and Randall, D. J. (1992). Effect of short-duration seawater exposure on plasma ion concentrations and swimming performance in coho salmon (Oncorhynchus kisutch) parr. Can. J. Fish. Aquat. Sci. 49, 2399–2405. doi: 10.1139/f92-265
Brett, J. R. (1964). The respiratory metabolism and swimming performance of young sockeye salmon. J. Fish. Res. Board Can. 21, 1183–1226. doi: 10.1139/f64-103
Claireaux, G., and Lefrancois, C. (2007). Linking environmental variability and fish performance: integration through the concept of scope for activity. Philos. Trans. R. Soc. B Biol. Sci. 362, 2031–2041. doi: 10.1098/rstb.2007.2099
Dadswell, M. J., Spares, A. D., Reader, J. M., and Stokesbury, M. J. (2010). The North Atlantic subpolar gyre and the marine migration of Atlantic salmon Salmo salar: the ’Merry-Go-Round’ hypothesis. J. Fish Biol. 77, 435–467. doi: 10.1111/j.1095-8649.2010.02673.x
Ern, R., Houng, D. T. T., Cong, N. V., Bayley, M., and Wang, T. (2014). Effect of salinity on oxygen consumption in fishes: a review. J. Fish Biol. 84, 1210–1220. doi: 10.1111/jfb.12330
Evans, D. H. (2002). Cell signaling and ion transport across the fish gill epithelium. J. Exp. Zool. 293, 336–347. doi: 10.1002/jez.10128
Evans, D. H., Piermarini, P. M., and Choe, K. P. (2005). The multifunctional fish gill: dominant site of gas exchange, osmoregulation, acid-base regulation, and excretion of nitrogenous waste. Physiol. Rev. 85, 97–177. doi: 10.1152/physrev.00050.2003
Farmer, G. J., and Beamish, F. W. H. (1969). Oxygen consumption of Tilapia nilotica in relation to swimming speed and salinity. J. Fish. Res. Board Can. 26, 2807–2821. doi: 10.1139/f69-277
Farrell, A. P. (2007). Cardiorespiratory performance during prolonged swimming tests with salmonids: a perspective on temperature effects and potential analytical pitfalls. Philos. Trans. R. Soc. B 362, 2017–2030. doi: 10.1098/rstb.2007.2111
Febry, R., and Lutz, P. (1987). Energy partitioning in fish - the activity-related cost of osmoregulation in a euryhaline cichlid. J. Exp. Biol. 128, 63–85.
Gaumet, F., Boeuf, G., Severe, A., Leroux, A., and Mayergonstan, N. (1995). Effects of salinity on the ionic balance and growth of juvenile turbot. J. Fish Biol. 47, 865–876. doi: 10.1111/j.1095-8649.1995.tb06008.x
Hvas, M., Folkedal, O., Imsland, A., and Oppedal, F. (2017a). The effect of thermal acclimation on aerobic scope and critical swimming speed in Atlantic salmon. Salmo salar. J. Exp. Biol. 220, 2757–2764. doi: 10.1242/jeb.154021
Hvas, M., Karlsbakk, E., Mæhle, S., Wright, D. W., and Oppedal, F. (2017b). The gill parasite Paramoeba perurans compromises aerobic scope, swimming capacity and ion balance in Atlantic salmon. Conserv. Physiol. 5:cow066. doi: 10.1093/conphys/cox066
Hvas, M., and Oppedal, F. (2017). Sustained swimming capacity of Atlantic salmon. Aquacult. Environ. Interact 9, 361–369. doi: 10.3354/aei00239
Iftikar, F. I., Matey, V., and Wood, C. M. (2010). The Ionoregulatory responses to hypoxia in the freshwater rainbow trout Oncorhynchus mykiss. Physiol. Biochem. Zool. 83, 343–355. doi: 10.1086/648566
Kelly, S. P., and Wood, C. M. (2001). Effect of cortisol on the physiology of cultured pavement cell epithelia from freshwater trout gills. Am. J. Physiol. Regul. Integr. Comp. Physiol. 281, R811–R820. doi: 10.1152/ajpregu.2001.281.3.R811
Keys, A. B. (1931). Chloride and water secretion and absorption by the gills of eels. J. Comp. Physiol. 15, 364–388.
Kieffer, J. D. (2000). Limits to exhaustive exercise in fish. Comp. Biochem.Physiol. 126A, 161–179. doi: 10.1016/S1095-6433(00)00202-6
Kita, J., and Itazawa, Y. (1989). Release of erythrocytes from the spleen during exercise and splenic constriction by adrenaline infusion in rainbow trout. Icthyol. Res. 36, 48–52.
Krogh, A. (1937). Osmotic regulation in freshwater fishes by active absorption of chloride ions. Z. Vergl. Physiol. 24, 656–666. doi: 10.1007/BF00592303
Kültz, D., Bastrop, R., Jürss, K., and Siebers, D. (1992). Mitochondria-rich (MR) cells and the activities of Na + /K + -ATPase and carbonic anhydrase in the gill and opercular epithelium of O. mossambicus adapted to various salinities. Comp. Biochem. Physiol. 102B, 293–301.
Laurent, P., and Perry, S. F. (1990). Effects of cortisol on gill chloride cell morphology and ionic uptake in the freshwater trout, Salmo gairdneri. Cell Tissue Res. 259, 429–442. doi: 10.1007/BF01740769
Mancera, J. M., and McCormick, S. D. (2000). Rapid activation of gill Na +, K + -ATPase in the euryhaline teleost Fundulus heteroclitus. J. Exp. Zool. 287, 263–274. doi: 10.1002/1097-010X(20000901)287:4<263::AID-JEZ1>3.0.CO;2-I
Marshall, W. S. (2002). Na +, Cl-, Ca2 + and Zn2 + transport by fish gills: retrospective review and prospective synthesis. J. Exp. Zool. 293, 264–283. doi: 10.1002/jez.10127
McCormick, S. D. (1993). Methods for nonlethal gill biopsy and measurement of Na +, K + -Atpase activity. Can. J. Fish. Aquat. Sci. 50, 656–658. doi: 10.1139/f93-075
McCormick, S. D. (2001). Endocrine control of osmoregulation in teleost fish. Am. Zool. 41, 781–794. doi: 10.1093/icb/41.4.781
McCormick, S. D., Moyes, C. D., and Ballantyne, J. S. (1989). Influence of salinity on the energetics of gill and kidney of Atlantic salmon (Salmo salar). Fish Physiol. Biochem. 6, 243–254. doi: 10.1007/BF01875027
McCormick, S. D., and Saunders, R. L. (1987). Preparatory physiological adaptations for marine life of salmonids: osmoregulation, growth, and metabolism. Am. Fish. Soc. Symp. 1, 211–229.
Morgan, J. D., and Iwama, G. K. (1998). Salinity effects on oxygen consumption, gill Na +, K + -ATPase and ion regulation in juvenile coho salmon. J. Fish Biol. 53, 1110–1119.
Morgan, J. D., and Iwama, G. K. (1999). Energy cost of NaCl transport in isolated gills of cutthroat trout. Am. J. Physiol. Regul. Integr. Comp. Physiol. 277, R631–R639. doi: 10.1152/ajpregu.1999.277.3.R631
Nakano, T., and Tomlinson, N. (1967). Catecholarnine and carbohydrate concentrations in rainbow trout (Salmo gairdneri) in relation to physical disturbance. J. Fish. Res. Bd. Can. 24, 1701–1715. doi: 10.1139/f67-140
Nelson, J. S. (2016). Fishes of the World, 5th Edn. Hoboken, NJ: John Wiley & Sons, Inc. doi: 10.1002/9781119174844
Nilsen, T. O., Ebbesson, L. O. E., Kverneland, O. G., Kroglund, F., Finstad, B., and Stefansson, S. O. (2010). Effects of acidic water and aluminum exposure on gill Na +, K + ATPase – α subunit isoforms, enzyme activity, physiology and return rates in Atlantic salmon (Salmo salar L.). Aquat. Toxicol. 97, 250–259. doi: 10.1016/j.aquatox.2009.12.001
Nilsen, T. O., Ebbesson, L. O. E., Madsen, S. S., McCormick, S. D., Andersson, E., Björnsson, B., et al. (2007). Differential expression of gill Na +, K + -ATPase α & β subunits, Na +, K +, 2Cl- cotransporter and CFTR anion channel in juvenile anadromous and landlocked Atlantic salmon (Salmo salar). J. Exp. Biol. 210, 2885–2896. doi: 10.1242/jeb.002873
Nilsson, S., and Sundin, L. (1998). Gill blood flow control. Comp. Biochem. Physiol. 119A, 137–147. doi: 10.1016/S1095-6433(97)00397-8
Nordlie, F. G. (1978). Influence of environmental salinity on respiratory oxygen demands in euryhaline teleost, Ambassis interrupta Bleeker. Comp. Biochem. Physiol. A 59, 271–274. doi: 10.1016/0300-9629(78)90160-3
Pagnotta, A., Brooks, L., and Milligan, L. (1994). The potential regulatory roles of cortisol in recovery from exhaustive exercise in rainbow trout. Can. J. Zool. 72, 2136–2146. doi: 10.1139/z94-286
Pearson, M. P., and Don Stevens, E. (1991). Splenectomy impairs aerobic swim performance in trout. Can. J. Zool. 69, 2089–2092. doi: 10.1139/z91-291
Poulsen, P., Morth, P., Egebjerg, J., and Nissen, P. (2010). Phosphorylation of the Na +, K +, -ATPase and the H +, K + -ATPase. FEBS Lett. 584, 2589–2595. doi: 10.1016/j.febslet.2010.04.035
Priede, I. G. (1985). “Metabolic scope in fish,” in Fish Energetics: New Perspectives, eds P. Tyler and P. Calow (London: Croom Helm), 33–64.
Rao, G. M. M. (1968). Oxygen consumption of rainbow trout (Salmo gairdneri). Can. J. Zool. 46, 781–786. doi: 10.1139/z68-108
Remen, M., Solstorm, F., Bui, S., Klebert, P., Vågseth, T., Solstorm, D., et al. (2016). Critical swimming speed in groups of Atlantic salmon Salmo salar. Aqat. Environ. Int. 8, 659–664. doi: 10.1242/jeb.154021
Sardella, B. A., and Brauner, C. J. (2007). “The osmo-respiratory compromise in fish,” in Fish Respiration and Environment, eds M. N. Fernandes, F. T. Rantin, M. L. Glass, and B. G. Kapoor (Boca Raton, FL: CRC Press), 147–165.
Smith, P. K., Krohn, R. I., Hermanson, G. T., Mallia, A. K., Gartner, F. H., Provenzano, M. D., et al. (1985). Measurement of protein using bicinchoninic acid. Anal. Biochem. 150, 76–85. doi: 10.1016/0003-2697(85)90442-7
Swanson, C. (1998). Interactive effects of salinity on metabolic rate, activity, growth and osmoregulation in the euryhaline milkfish (Chanos chanos). J. Exp. Biol. 201, 3355–3366.
Tipsmark, C. K., and Madsen, S. S. (2001). Rapid modulation of Na + /K + -ATPase activity in osmoregulatory tissues of a salmonid fish. J. Exp. Biol. 204, 701–709.
Uchida, K., Kaneko, T., Yamaguchi, A., Ogasawara, T., and Hirano, T. (1997). Reduced hypoosmoregulatory ability and alteration in gill chloride cell distribution in mature chum salmon (Oncorhynchus keta) migrating upstream for spawning. Mar. Biol. 129, 247–253. doi: 10.1007/s002270050165
Wagner, G. N., Kuchel, L. J., Lotto, A., Patterson, D. A., Shrimpton, J. M., Hinch, S. G., et al. (2006). Routine and active metabolic rates of migrating adult wild sockeye salmon (Oncorhynchus nerka Walbaum) in seawater and freshwater. Physiol. Biochem. Zool. 79, 100–108. doi: 10.1086/498186
Wendelaar Bonga, S. E. (1997). The stress response of fish. Physiol. Rev. 77, 591–625. doi: 10.1152/physrev.1997.77.3.591
Wood, C. M. (1991). Acid-base and ion balance, metabolism, and their interactions, after exhaustive exercise in fish. J. Exp. Biol. 160, 285–308.
Wood, C. M., and Randall, D. J. (1973a). The influence of swimming activity on water balance in the rainbow trout (Salmo gairdneri). J. Comp. Physiol. 82, 257–276. doi: 10.1007/BF00694239
Wood, C. M., Turner, J. D., and Graham, M. S. (1983). Why do fish die after severe exercise? J. Fish. Biol. 22, 189–201. doi: 10.1111/j.1095-8649.1983.tb04739.x
Wood, M. C., and Randall, D. J. (1973b). The influence of swimming activity on sodium balance in the rainbow trout (Salmo gairdneri). J. Comp. Physiol. 82, 207–233. doi: 10.1007/BF00694237
Keywords: Ucrit, aerobic scope, swim tunnel respirometry, osmorespiratory compromise, stress response
Citation: Hvas M, Nilsen TO and Oppedal F (2018) Oxygen Uptake and Osmotic Balance of Atlantic Salmon in Relation to Exercise and Salinity Acclimation. Front. Mar. Sci. 5:368. doi: 10.3389/fmars.2018.00368
Received: 05 June 2018; Accepted: 25 September 2018;
Published: 16 October 2018.
Edited by:
José Luis Soengas, University of Vigo, SpainReviewed by:
Juan Miguel Mancera, University of Cádiz, SpainJuan Antonio Martos-Sitcha, University of Cádiz, Spain
Copyright © 2018 Hvas, Nilsen and Oppedal. This is an open-access article distributed under the terms of the Creative Commons Attribution License (CC BY). The use, distribution or reproduction in other forums is permitted, provided the original author(s) and the copyright owner(s) are credited and that the original publication in this journal is cited, in accordance with accepted academic practice. No use, distribution or reproduction is permitted which does not comply with these terms.
*Correspondence: Malthe Hvas, bWFsdGhlLmh2YXNAaW1yLm5v