- 1Horn Point Laboratory, University of Maryland Center for Environmental Science, Cambridge, MD, United States
- 2Department of Biology, University of Akron, Akron, OH, United States
Polar marine ecosystems are characterized by low water temperatures, sea ice cover, and extreme annual variation in solar irradiance and primary productivity. A review of the available information from the Arctic suggests that mixotrophy (i.e., the combination of photosynthetic and phagotrophic modes of nutrition in one cell) is wide spread among plankton. In the central Arctic Ocean (AO) in summer, mixotrophic flagellates such as Micromonas and Dinobryon can account for much of bacterivory. Planktonic ciliates with acquired phototrophy form the bulk of microzooplankton biomass in both the ultra-oligotrophic deep basins of AO and its productive shelf seas. With the exception of the diatom bloom in the marginal ice zone, mixotrophic ciliates often dominate total chlorophyll in the mixed layer in summer taking advantage of the 24-h insolation. Their relatively high growth rates at low temperatures indicate that they are an important component of primary and secondary production. The key Arctic copepod species preferentially feed on chloroplast-bearing ciliates, which form an important link in the planktonic food web. The limited available year round data indicate that mixotrophic plankton persist in the water column during the long polar winter when irradiance is low or absent and ice cover further reduces light penetration. These observations suggest that at high latitudes an alternative food web based on mixotrophy may dominate the pelagic lower food web during much of the year.
Introduction
Mixotrophy, defined as the combination of phagotrophy and photosynthesis in an individual cell, is increasingly recognized as an important trophic mode among planktonic protists, both “phytoplankton” and “microzooplankton,” in the global ocean (reviewed in Caron, 2017; Leles et al., 2017; Selosse et al., 2017; Stoecker et al., 2017) but in polar waters mixotrophy has not received much attention. In fact, this mixed mode of nutrition is so wide-spread that the above dichotomy may be no longer suitable for an accurate description of the role of unicellular eukaryotes in the ocean (Flynn et al., 2013). Ecosystem models that include mixotrophy indicate that this trophic mode has profound effects on biogeochemical cycling, including increasing carbon fixation, decreasing loss of dissolved organic carbon and increasing vertical carbon flux (Mitra et al., 2014; Ward and Follows, 2016). Mixotrophy also influences the structure and function of food webs. Modeling indicates that mixotrophy can increase mean organism size and trophic transfer, potentially resulting in increased production at upper levels in the food web (Mitra et al., 2014; Ward and Follows, 2016).
It is particularly important to understand the effects of mixotrophy in the Arctic because its marine environment is changing rapidly. The Arctic Ocean may have lost over 50% of its sea ice volume (Kwok and Rothrock, 2009), with the largest decreases in the Barents, Kara, and Siberian sectors, particularly over the continental shelf (Pabi et al., 2008). In just 12 years, the open-water growing season has increased by 45 days, promoting a pan-Arctic 20% increase in net primary production (Brown and Arrigo, 2012). Climate change is causing a patchwork of altered environmental conditions in the Arctic. Decreases in sea ice thickness, extensions in the length of the ice free season, increased water temperatures, and freshening have led to increased stratification in some seas, whereas increased mixing due to storms have been observed in other areas (Metfies et al., 2016; Blais et al., 2017; Oziel et al., 2017). Although the rising trend in primary production (Slagstad et al., 2011) may be hindered by stronger stratification and nutrient limitation in offshore areas (Ardyna et al., 2011, 2017; Brown and Arrigo, 2012), these changes are expected to bolster the pelagic system at the expense of the benthic components, with profound impacts on trophic structure and carbon fluxes (Wassmann and Reigstad, 2011). Recent studies in the Arctic indicate that a major portion of pelagic primary production is channeled to the higher trophic levels through unicellular grazers such as ciliates and dinoflagellates (Olson and Strom, 2002; Verity et al., 2002; Calbet et al., 2011b; Sherr et al., 2013; Stoecker et al., 2014; Franzè and Lavrentyev, 2017). This warrants investigation of the effects of climate change on microbial plankton as even minor effects at the base of food webs could be amplified through trophic chains (Sarmento et al., 2010). Given the rapid changes in Arctic ecosystems, it is increasingly important to determine the trophic modes of plankton in Arctic seas and predict how trophic modes may change with the environment and how this may affect ecosystem processes.
The Objectives and Material
Few attempts have been made to directly examine the physiological rates of mixotrophic plankton in the Arctic due to logistical and methodological constraints (Putt, 1990; Sanders and Gast, 2012; Franzè and Lavrentyev, 2014). Nevertheless, there is evidence in the literature that mixotrophic plankton play an important role(s) in the Arctic marine ecosystems. Our objectives are to review existing data, identify data gaps, and present hypotheses about the roles of mixotrophy in Arctic Seas and how these may be altered due to changes in the physical environment.
In this review we primarily focus on the following contrasting regions of the Arctic: the deep oligotrophic Canada Basin of the Arctic Ocean, the relatively shallow and productive Barents and Kara shelf seas, and the main entrance for the Atlantic water into the Arctic Ocean, the Fram Strait (Figure 1). In addition we consider data from the productive shelf of the sub-Arctic Bering Sea, which has been subjected to similar climatic changes as the Arctic shelf seas (Hunt and Megrey, 2005). Each of the above polar regions is unique in terms of bathymetry, circulation, sea-ice cover, and food webs (Carmack and Wassmann, 2006). Thus, they are ideally suited for a pan-Arctic comparison.
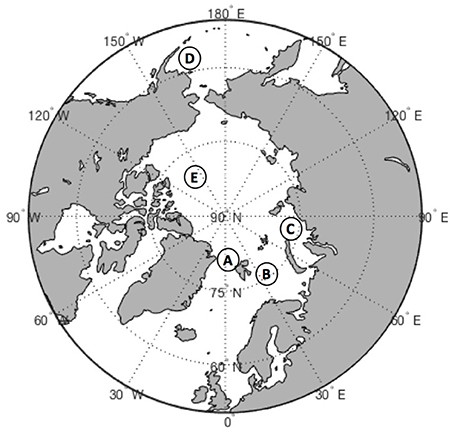
Figure 1. Map of the Arctic Ocean showing the main study locations: A, Fram Strait; B, Barents Sea; C, Kara Sea; D, Bering Sea; E, Canada Basin.
Review
Evidence for Mixotrophy Among Arctic Plankton
Although mixotrophy among planktonic protists is well documented in temperate and tropical waters, less is known about mixotrophy in Arctic seas. Phagotrophy by phytoflagellates is usually underestimated, particularly in remote locations such as the Arctic, where field experiments are logistically difficult or which are difficult to sample in all seasons. Ingestion of prey by small, pigmented cells is difficult to detect and is often underestimated, even in experimental studies designed to measure it (Anderson et al., 2017). Ciliates with plastids are more readily distinguished from heterotrophs than phagotrophic phytoflagellates from strict autotrophs. Measurements of feeding and photosynthesis in both mixotrophic flagellates and ciliates are rare. However, there are ample reasons to hypothesize that mixotrophy is common among Arctic plankton. Below we list mixotrophic flagellate and ciliate taxa reported from Arctic Seas and provide evidence that the taxa are mixotrophic. The evidence for mixotrophy ranges from observation of food vacuoles or plastids in preserved specimens to field or laboratory measurements of feeding and photosynthesis. For some species the evidence is very limited, whereas other species have been studied intensively. For well-studied species, we have included only a few key references.
Chrysophyta
Arctic plankton assemblages include nanoplanktonic chrysophytes such as Ochromonas spp. that are often bacterivorous as well as photosynthetic (Estep et al., 1986; Andersson et al., 1989; Keller et al., 1994). The colonial chrysophyte, Dinobryon balticum, is a common component of the microplankton in Arctic and sub-Arctic seas (Table 1) and is often associated with low irradiance and the deep chlorophyll maximum (DCM) layer (McKenzie et al., 1995). The colonies are a significant contributor to particle flux during summer in the Barents Sea (Olli et al., 2002) and probably other coastal Arctic seas. Although D. balticum has plastids and requires light, this species supplements it nutrition with bacterivory (McKenzie et al., 1995).
Cryptophyta
Cryptophytes are another algal group often associated with low light conditions and that are known to contribute to bacterivory (Table 1). In phytoplankton investigations and in situ studies of phagotrophy by algae, cryptophytes have rarely been identified to species. Teleaulax amphioxeia, a widespread species found in temperate and Arctic waters (Table 1) has been shown to be mixotrophic (Yoo et al., 2017).
Haptophyta (Prymnesiophyta)
Although many haptophytes are mixotrophic and often dominate the mixotrophic phytoplankton assemblage in temperate waters (Unrein et al., 2014), the dominant haptophyte in Arctic waters, Phaeocystis pouchetii, is yet to be shown to be phagotrophic. Another photosynthetic prymnesiophyte genus found in Arctic water is Chrysochromulina (Table 1). At low irradiances, feeding can enhance growth in some Chrysochromulina species (Hansen and Hjorth, 2002).
Chlorophyta
A well-documented case of mixotrophy among Arctic chlorophyte flagellates is bacterivory by Micromonas sp. This picoplankton-sized member of the Prasinophyceae is widely distributed and abundant in the Arctic (Not et al., 2005). During autumn, when the Beaufort Sea and Canada Basin were highly oligotrophic, photosynthetic picoflagellates numerically dominated the phytoplankton and accounted for as much or sometimes more bacterivory than heterotrophic flagellates (Sanders and Gast, 2012). Genetic analysis indicated that Micromonas was a common and probably abundant component of the picoflagellate assemblage (Sanders and Gast, 2012). In laboratory experiments with a culture of Arctic Micromonas, McKie-Krisberg and Sanders (2014) observed the greatest bacterivory under conditions of high light and inorganic nutrient limitation, conditions similar to the oligotrophic polar seas in summer.
Dinophyta
Dinoflagellates possess feeding structures that allow them to consume prey of equal to or greater than their own cell size. Most plastidic dinoflagellates are probably mixotrophic (reviewed in Jeong et al., 2010; Hansen, 2011). Mixotrophic as well as heterotrophic dinoflagellates are common in the Arctic (Table 1). Nanoplanktonic dinoflagellates have often been overlooked, but they appear to be important components of the polar plankton. For example, plastidic nano-sized gymnodiniids formed 55–85% of total dinoflagellate abundance in the Barents Sea (Franzè and Lavrentyev, 2017). Among the small size mixotrophic dinoflagellates are Karlodinium and small Gymnodinium-like spp. Some taxa, like Karlodinium, can ingest prey most of the time although inorganic nutrient limitation often stimulates feeding (Li et al., 1999, 2000; Calbet et al., 2011a). A small photosynthetic dinoflagellate that occurs in the Arctic as well as in temperate low salinity waters is Heterocapsa rotundata (Table 1). In temperate waters it blooms in winter and is stimulated to feed by light limitation (Millette et al., 2017). H. rotundata may be an important mixotrophic alga in low salinity, turbid waters, such as the coastal Beaufort Sea and Kara Sea. Among the larger thecate photosynthetic dinoflagellates, many species have been observed to capture and digest prey about their own size or larger, including diatoms, other flagellates, and ciliates (reviewed in Hansen, 2011). This group includes Alexandrium and Tripos (formerly Ceratium) spp. that have been observed in the Arctic (Table 1). Mixotrophic Dinophysis spp. prey on ciliates and obtain their plastids from ingestion of photosynthetic Mesodinium spp. (Park et al., 2006; Hansen, 2011) and are reported in Arctic Seas (Table 1).
Ciliophora
Phagotrophic protists that feed on phytoplankton can also be mixotrophic due to acquired phototrophy; among marine ciliates this usually involves sequestration of plastids and sometimes other organelles from their algal prey (reviewed in Stoecker et al., 2009). In field studies, presence of plastids in ciliates is easier to recognize than phagotrophy in phytoplankton. Epifluorescence microscopy is used to detect the chlorophyll (plastids) in fresh or aldehyde fixed samples that have been stored under conditions (refrigeration and darkness) that minimize chlorophyll degradation. However, many field studies of microzooplankton rely solely on acid Lugol's fixed samples. Thus, mixotrophy among the ciliates often is overlooked.
Among the oligotrich ciliates, plastid-retention is a species-specific attribute. In investigations that lack data from epifluorescence microscopy, the presence of certain species is a good indicator of mixotrophy among the oligotrichs. Laboea strobila, a very distinctive plastid-retaining species, several plastidic Strombidium spp. and Tontonia spp. which always seem to be plastidic, are reported from many Arctic Seas (Table 2). In addition, several plastidic prostomatid and haptorid ciliates such as Askenasia, Cyclotrichium, Didinium, Prorodon, and Urotricha occur in ice-covered waters and open waters of the Barents Sea and Kara Sea (Lavrentyev, 2012; Franzè and Lavrentyev, 2014, 2017). In field studies in which epifluorescence microscopy is utilized, plastid-retaining oligotrichs usually comprise 30–40% of the oligotrich assemblage in the euphotic zone (Stoecker et al., 2017), but in the Arctic they often dominate the ciliate assemblage (Table 3).
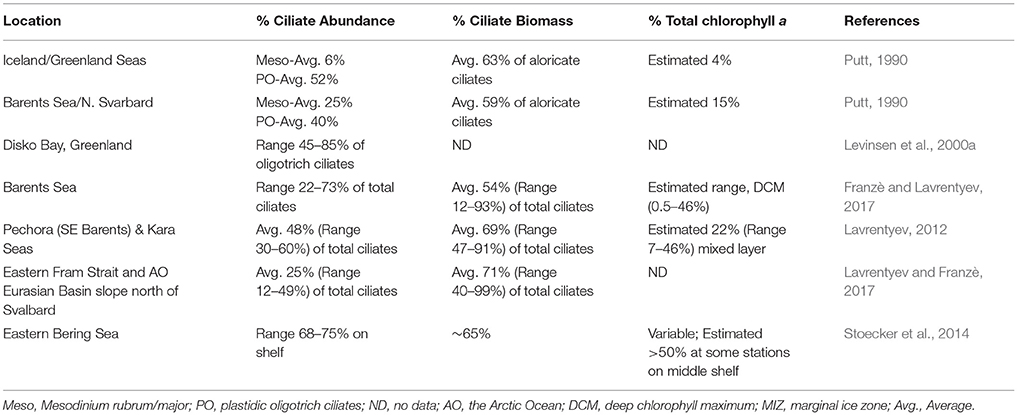
Table 3. Relative contributions of mixotrophic ciliates to ciliate abundance, biomass, and total chlorophyll a (chl a) in the Arctic during summer.
Contributions of mixotrophic ciliates to chlorophyll are highly variable even in ecosystems in which they are abundant (Table 3), partially because of the large variations in total chlorophyll a. Putt (1990) estimated that mixotrophic ciliates contributed ~15% of the total chlorophyll a at 2 m in the Barents Sea. In the deep chlorophyll maximum (DCM) of the Barents Sea, Franzè and Lavrentyev (2017) estimated that mixotrophic ciliates (predominantly plastidic oligotrichs) contributed between 0.5 and 46% of the chlorophyll a. The contribution of mixotrophs to ciliate abundance and total chlorophyll a peaked at the Polar Front primarily due to populations of the large mixotrophic oligotrichs L. strobila, Strombidium conicum, and S. wulffi (Figure 2). In the productive eastern Bering Sea during summer, mixotrophic ciliates abundance (also predominantly plastid oligotrichs) and their estimated contribution to total chlorophyll a was also very variable. The contribution of mixotrophs to ciliate abundance was relatively low at stations near the shelf edge and in upwelling around the Pribilof Islands. However, mixotrophic ciliate abundance was greatest and estimated contribution of ciliates to total chlorophyll was often 50% or more in highly stratified waters in the middle of the northern shelf (Stoecker et al., 2014). Similarly, in the shallow waters of the Kara Sea, mixotrophic ciliates were responsible for up to 46% of total chlorophyll a (Lavrentyev, 2012). In the western Fram Strait, mixotrophic oligotrichs formed a spring bloom in the mixed layer within the Atlantic Drift (>200 μg C L−1, Lavrentyev and Franzè, 2017); this is the highest ciliate biomass recorded so far in the Arctic.
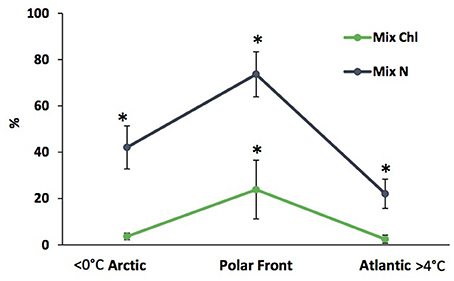
Figure 2. Relative abundance and contribution to total chlorophyll a of mixotrophic ciliates in the deep chlorophyll layer along the latitudinal gradient in the Barents Sea (modified from Franzè and Lavrentyev, 2017). Vertical bars, standard deviation; asterisks denote significant difference (p < 0.05) based on ANOVA and Fisher LSD test. Mix N, relative abundance of mixotrophic ciliates; Mix Chl, estimated contribution of mixotrophic ciliates to total chlorophyll a.
The above estimates of mixotrophic contributions are conservative. Mixotrophic chlorophyll a content was calculated based on ciliate volume (Montagnes et al., 1994), assuming that the volume to chlorophyll a relationship is similar to that in autotrophic plankton (Dolan and Perez, 2000). The chlorophyll a cellular quota calculated based on these assumptions for S. conicum (30,000 μm3, 55 pg chlorophyll cell−1) was similar to that measured directly in the Barents Sea (48 pg chlorophyll a cell−1, Putt, 1990). However, oligotrich chlorophyll a content can be much higher (Stoecker et al., 1988; McManus et al., 2012). Laboratory and field studies have demonstrated that plastidic ciliates graze on pico- and nano-phytoplankton, can have about the same biovolume specific chlorophyll a content as their phytoplankton prey and are photosynthetic (reviewed in Stoecker et al., 2009, 2017). One of the few field studies in which chlorophyll a content and photosynthesis were measured in oligotrichs from natural assemblages was conducted by Putt (1990) in Arctic seas. In Strombidium sp. “A,” carbon: chlorophyll ratios varied from 87 to 103 and in L. strobila from 200 to 232. In Strombidium sp. “A,” light saturated photosynthetic rates (Pmax) averaged 2.1 pg C (pg chlorophyll a)−1h−1, which was equivalent ~2.8% of body carbon h−1 (Putt, 1990; Table 3).
The “red” ciliates Mesodinium rubrum and M. major are important mixotrophic ciliates in coastal and upwelling waters globally (Johnson et al., 2016). In Arctic waters they are a common component of the microplankton (Table 2). M. major was described as a separate species from M. rubrum relatively recently (Garcia-Cuetos et al., 2012) so in Table 2 we refer to them together. In some cases, red Mesodinium are responsible for dense blooms in the Arctic (Johnson et al., 2016). In contrast with the plastid retaining oligotrichs, that can retain plastids from several algal taxa (reviewed in Stoecker et al., 2009; McManus et al., 2012), the red Mesodinium spp. are specialized and primarily retain plastids, nuclei and other cell components from phycoerythrin-containing cryptophytes in the Teleaulax and Plagioselmis species complex (Johnson et al., 2016). The metabolism of M. rubrum/M. major is primarily photosynthetic, although polar strains are capable of survival in the dark for long periods (Johnson and Stoecker, 2005). M. rubrum is often considered to be more a component of the phytoplankton than the microzooplantkon because of their high chlorophyll content, high rates of photosynthesis and ability to form blooms (reviewed in Crawford, 1989). In the Arctic, as elsewhere, red Mesodinium spp. are routinely a significant component of the protist plankton in meso- and eutrophic waters, particularly under low light conditions which also favor their cryptophyte prey. Blooms are usually associated with fronts or upwelling events and can be “hotspots” of primary and secondary production which influence biogeochemical cycling (Herfort et al., 2012).
Factors Influencing Mixotrophy in the Arctic
Although there have been fewer studies of mixotrophy in the Arctic than in temperate seas, the environmental conditions in the Arctic should often favor mixotrophs over strict heterotrophs or autotrophs. Mixotrophy is advantageous in environments in which resource availability is highly variable and/or does not support balanced growth (reviewed in Mitra et al., 2016; Stoecker et al., 2017). Mixotrophic strategies vary among planktonic protists (Mitra et al., 2016). For example, many small phytoflagellates and photosynthetic dinoflagellates can grow as strict autotrophs, using light and dissolved inorganic nutrients for growth, but nitrogen (N), phosphorus (P), and iron limitation or skewed N:P ratios induce or increase phagotrophy (Maranger et al., 1998; Li et al., 2000; Smalley et al., 2003). Less well-known are marine phytoflagellates and photosynthetic dinoflagellates that are stimulated to feed by light or carbon limitation. A freshwater mixotrophic Ochromonas sp. (chrysophyte) primarily obtains carbon and nitrogen from ingestion of bacteria and only assimilates inorganic nutrients to appreciable degree when bacterial abundances are very low (Terrado et al., 2017). The same strategy may occur in some marine Ochromonas spp. However, at least one marine Ochromonas sp. is primarily photosynthetic and is stimulated to feed by low light and low nutrients (Keller et al., 1994). In the marine dinoflagellate H. rotundata, light limitation stimulates ingestion of bacteria (Millette et al., 2017) but it is unclear how common this response is in photosynthetic dinoflagellates. Interaction of light and inorganic nutrient availability is important in regulating the relative contributions of phototrophy and phagotrophy to survival and growth in most mixotrophic flagellates. For example, species-specific responses of both photosynthesis and feeding to light and nutrients were observed in cultured Antarctic mixotrophic flagellates (McKie-Krisberg et al., 2015).
Among the microzooplankton with acquired phototrophy (which in the Arctic plankton, is primarily mixotrophic ciliates), most use photosynthesis as a carbon and energy supplement (reviewed in Stoecker et al., 2009). Except in the case of M. rubrum, which has nitrate reductase, most plastidic ciliates obtain most of their nitrogen from food. In the plastidic oligotrichs, photosynthate is used primarily to cover respiratory demands for carbon and thus can increase survival times during starvation and, particularly when prey are limiting, increase gross growth efficiency and growth rate (McManus et al., 2012; reviewed in Stoecker et al., 2017).
Environmental parameters, including temperature, inorganic nutrient availability, and light availability are all expected to influence mixotrophy among both “phytoplankton” and “microzooplankton” mixotrophs in Arctic Seas.
Temperature
Sub-zero sea temperatures typical for the Arctic Ocean may be conducive to mixotrophy, particularly among microzooplankton such as ciliates, because photosynthetic plankton are thought to be less constrained by low temperatures than their heterotrophic consumers (Rose and Caron, 2007). The latitudinal trends in mixotrophic ciliate growth rates in the Barents Sea seem to support this idea (Franzè and Lavrentyev, 2014). However, mixotrophic ciliates also peaked at relatively warm summer temperatures in the Kara (Lavrentyev, 2012) and Bering Seas (Stoecker et al., 2014). In the Fram Strait the highest concentration of ciliates in the Arctic was found in the Atlantic drift warm core (Lavrentyev and Franzè, 2017).
Little information is available on latitudinal or temperature gradients in mixotrophy among phytoflagellates. Laboratory experiments with a freshwater mixotrophic flagellate suggest that some mixotrophs become more heterotrophic with increases in temperature (Wilken et al., 2013). However, it is not clear that this is a general response among mixotrophic flagellates.
Oligotrophy
Oligotrophic conditions favor bacterivorous pico- and nanoplankton in tropical and temperate seas (Zubkov and Tarran, 2008; Hartmann et al., 2012; Mitra et al., 2016). In temperate seas, mixotrophic flagellates are often most abundant during summer stratification which can result in inorganic nutrient limitation of phytoplankton growth in the surface mixed layer (Mitra et al., 2014). Oligotrophic conditions are common during the ice-free season in some parts of the Arctic including the central Arctic Ocean, Beaufort Sea and parts of the Amundsen Sea.
Seasonally low phytoplankton biomass and small phytoplankton cell size can, in turn, select for mixotrophic oligotrich ciliates over strictly heterotrophic microzooplankton (Mitra et al., 2016). In large ciliates, photosynthetic carbon could cover a significant fraction of their metabolism (reviewed in Stoecker et al., 2009, 2017) due to relatively low volume-specific respiration rates (Dolan and Perez, 2000). The Barents Sea Polar Front, where the relative abundance of mixotrophic ciliates peaked, has relatively low phytoplankton biomass in summer compared to receding ice edges and does not stimulate phytoplankton productivity in summer (Reigstad et al., 2011; Erga et al., 2014). However, large mixotrophic oligotrichs are not restricted to oligotrophic conditions in the Arctic. Laboea strobila was abundant under phytoplankton bloom conditions in the Bering Sea (Olson and Strom, 2002), Kara Sea (Lavrentyev, 2012), and the Fram Straight (Lavrentyev and Franzè, 2017). In the Gulf of Ob, M. rubrum reached its maximum of 75 μg C L−1 at total chlorophyll a >17 μg L during a cyanobacterial bloom (Lavrentyev, 2012). Mixotrophs contributed up to 46% of total chlorophyll a in the deep chlorophyll maximum (DCM) at the pycnocline in the Barents Sea, where phytoplankton accumulate in summer (Franzè and Lavrentyev, 2017).
Light
In contrast to temperate and especially low latitudes, the Arctic is characterized by extremes in solar irradiance. During the polar day, 24-h insolation may favor mixotrophic metabolism over strictly heterotrophic metabolism in planktonic protists. For example, given the same prey concentration, a mixotrophic oligotrich grew faster under luxury light (McManus et al., 2012). The combination of high irradiance and low inorganic nutrients stimulates phagotrophy in many mixotrophic flagellates and dinoflagellates (reviewed in Stoecker et al., 2017). The proportion of phagotrophic and autotrophic-derived carbon in a mixotrophic dinoflagellate diet changed dynamically in response to light conditions and food availability (Riisgaard and Hansen, 2009). Mixotrophic ciliate abundance often peaks in the upper part of the mixed layer (Putt, 1990; Rat'kova and Wassmann, 2002; Lavrentyev, 2012; Franzè and Lavrentyev, 2014; Jiang et al., 2015). Some mixotrophic ciliates and dinoflagellates can migrate between prey or nutrient rich deep chlorophyll maximum layers and well-lit surface waters (Crawford and Lindholm, 1997; Ji and Franks, 2007).
During winter and in/under sea-ice, prolonged dark or low irradiance may also favor mixotrophic phytoplankton over strict autotrophs. Many mixotrophic phytoflagellates and some dinoflagellates use phagotrophy to supplement carbon budgets when light limited (Czypionka et al., 2011; McKie-Krisberg et al., 2015; Millette et al., 2017). Phagotrophy has been hypothesized to be an important mechanism supporting survival and limited growth of phytoflagellates during the polar winter (Zhang et al., 2003).
Although mixotrophy would not appear to be an advantage in ciliates during the dark polar winter, the plastidic ciliates L. strobila and M. rubrum persist under the ice in West Greenland (Levinsen et al., 2000a). The advantages of mixotrophy during the summer may outweigh the disadvantages of obtaining and maintaining chloroplasts during the winter. Levinsen et al. (2000a) suggested that mixotrophic ciliates survive long periods of darkness due to reduced metabolic demands at low winter temperatures. During the polar winter, photo-degradation is non-existent or minimal, which may contribute to long survival times of plastids in the ciliates. Although mixotrophic oligotrichs have been thought to require both algal prey and light (reviewed in Stoecker et al., 2017), under winter conditions some species may be able to survive and grow as heterotrophs. McManus et al. (2012) observed that a temperate mixotrophic ciliate could grow for several weeks in the dark when supplied with a small dinoflagellate as food, although the dinoflagellate did not support good growth of the ciliate in the light.
Role of Mixotrophy in the Pelagic Food Webs
Mixotrophy has important consequences for primary and secondary production and transfer of carbon to upper levels in Arctic planktonic food webs. Major differences between an Arctic food web with mixotrophy and the microbial loop sensu Azam et al. (1983) are conceptualized in Figure 3. In the traditional microbial loop, bacteria are consumed by heterotrophic nanoflagllates, which are then consumed by larger grazers such as ciliates. Most carbon collected by bacteria is respired in the subsequent trophic steps. In the mixotrophic microbial loop bacteria are consumed by both photosynthetic (exemplified by mixotrophic Micromonas) and heterotrophic nanoflagellates. The flagellates then become food for ciliates, which in addition to phagotrophy also use functional chloroplasts acquired from their photosynthetic prey. Thus, at each trophic step, energy dissipated through respiration is partially or completely replaced by solar energy. This is particularly important under oligotrophic conditions, when inorganic nutrient supply limits primary production. With mixotrophy among the photosynthetic plankton, primary production at the base of the food web can be enhanced (Zubkov and Tarran, 2008; Mitra et al., 2014). Studies in freshwater ecosystems indicate that mixotrophy can stabilize the stoichiometry (C:N:P) of the primary producers, which may in turn stabilize the efficiency of trophic transfer to secondary producers under varying nutrient regimes (Moorthi et al., 2017). Another benefit may be enhanced survival of plastidic ciliates and flagellates in a physiologically active state during the polar winter (Levinsen et al., 2000a; Zhang et al., 2003). When light becomes available, mixotrophic plankton can rapidly respond with high growth rates despite low sea temperatures (Franzè and Lavrentyev, 2014).
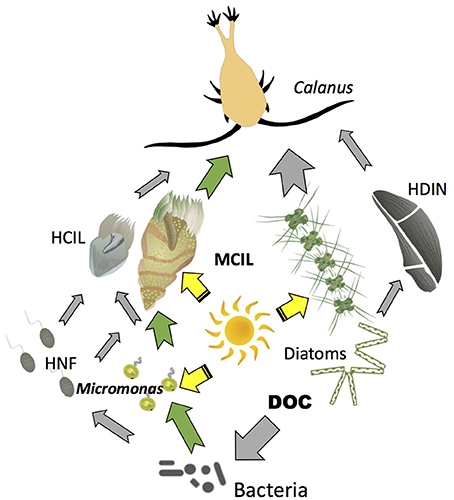
Figure 3. Conceptual model of Arctic food web with mixotrophy. Yellow arrows indicate flow of energy from the sun to photosynthetic organisms (autotrophs and mixotrophs); Gray arrows indicate flow of carbon to heterotrophs; Green arrows indicate major pathways of carbon flow to or from mixotrophs. HCIL, Strictly heterotrophic ciliates; MCIL, Mixotrophic ciliates; HNF, Heterotrophic nanoflagellates; DOC, Dissolved organic carbon; HDIN, Heterotrophic dinoflagellates.
Laboratory studies indicate that plastidic oligotrichs ingest and digest phytoplankton and, at least in temperate waters under moderate light intensities, derive much of their carbon, and probably almost all of their nitrogen and phosphorus from phagotrophy (Stoecker et al., 1988; McManus et al., 2012; Schoener and McManus, 2012). Particularly under prey limitation, photosynthesis can increase their gross growth efficiency (McManus et al., 2012) probably because photosynthesis can cover all or part of their respiratory demand for carbon in the light (Stoecker and Michaels, 1991). In the food web with mixotrophy, the sun's energy is directly transferred to relatively large size microzooplankton (the mixotrophic ciliates) as well as to traditional phytoplankton (such as diatoms and phytoflagellates). This augments microzooplankton production, which is important because microzooplankton are a large component of the diet of large size Arctic copepods, such as Calanus spp. (Campbell et al., 2009; Saiz et al., 2013). Mixotrophic ciliates in particular are a preferred prey of copepods (Levinsen et al., 2000b; Dutz and Peters, 2008). Such mixotrophic interactions have the potential to temporarily sustain mesozooplankton under low phytoplankton biomass conditions such as occur pre- and post-bloom in many polar seas. Modeling indicates that mixotrophy has the potential to increase food web transfer efficiencies, help retain inorganic nutrients (nitrogen and phosphorous) in the upper water column, and enhance transfer of carbon to larger size organisms (Mitra et al., 2014; Ward and Follows, 2016).
Potential Significance of Mixotrophy in the Changing Arctic
Below we outline three specific examples in which mixotrophs may be affected by changing climate and or how this could affect Arctic ecosystems now and in the future:
1. McMinn and Martin (2013) have suggested that phytoplankton will need to survive the same period of seasonal darkness, but at higher temperatures in the future, thus exhausting stored metabolic resources more quickly. In dark survival, phagotrophic phytoflagellates can have an advantage over diatoms (Zhang et al., 2003; Millette et al., 2017). This could result in changes in the composition of spring blooms, with an increase in phytoflagellates over diatoms.
2. Some areas of the Arctic are becoming more oligotrophic due to increased stratification. For example, offshore areas in the Western Arctic Ocean are becoming more oligotrophic. These changes have already resulted in the phytoplankton composition shift toward small sized-taxa in the Canada Basin (Li et al., 2009). Under such conditions, the presence of mixotrophy among photosynthetic flagellates and ciliates may moderate the negative climate change impacts on energy transfer to higher trophic levels and on particle flux out of surface waters (Figure 3).
3. Mixotrophy can facilitate blooms of unpalatable or harmful algae (Burkholder et al., 2008). Incursions of warmer waters, containing potentially bloom-forming, harmful species, already occur in the Arctic. Toxic Alexandrium, Dinophysis, Karlodinium, and Chrysochromulina spp. are all mixotrophic taxa that often proliferate in highly stratified or freshened waters and have been reported from the Arctic (Table 1).
To understand plankton dynamics in a changing Arctic environment, we obviously need information on the magnitude and spatial distribution of the physical and chemical changes. To understand and predict how these changes will alter the structure and function of Arctic planktonic food webs, we need information about alterations near the base of the food web, among the microbial plankton (bacteria and protists) because these changes will affect higher trophic levels through prey availability, palatability and size and alterations to biogeochemical cycles. In the Arctic, as elsewhere in aquatic food webs, many planktonic protists are not strict autotrophs or heterotrophs and this can have profound effects primary production and secondary production. We need to incorporate mixotrophy into our thinking, into our work on cruises and laboratory studies of Arctic “phytoplankton” and “microzooplankton,” and into models of Arctic marine ecosystems.
Conclusions and Questions for Future Research
In summary, many mixotrophic flagellates are found in Arctic waters, but there is only one reported field investigation (Sanders and Gast, 2012) of mixotrophy from Arctic Seas. Most of the data on Arctic phytoplankton relate to bloom-forming diatom species and Phaeocystis pouchetti but little data exist on the distribution and abundance of potentially mixotrophic phytoflagellate taxa. Mixotrophy by taxa that are traditionally categorized as “phytoplankton” has been overlooked in most Arctic seas where it may be particularly important in oligotrophic waters during summer and in light limiting conditions for phytoplankton growth year round. Under-ice phytoplankton blooms appear to be increasing in the Arctic (Arrigo et al., 2014); mixotrophs as well as diatoms may be a component of some of these blooms. Field investigations in the Antarctic have shown that mixotrophic flagellates are an important component of sea-ice algal assemblages (Moorthi et al., 2009) but the role of mixotrophs in this habitat in the Arctic appears to be unexplored.
An important task for future research is to determine if mixotrophy among flagellates is widespread in the Arctic and its role in planktonic food webs. For example, in the study by Sanders and Gast (2012), Micromonas and other mixotrophic flagellates preferred inert beads over the commonly used fluorescently labeled bacteria. In real life, they may consume a variety of prokaryotic and eukaryotic microorganisms. Genomic techniques for identification and quantification of populations will need to be combined with innovative measurements of both feeding and photosynthesis to accomplish this task (Anderson et al., 2017; Terrado et al., 2017). The degree of mixotrophy in protists (i.e., phagotrophy vs. photosynthesis) can be difficult to establish and its investigation requires novel approaches, involving both field and laboratory experiments. One important question is the effect of warming water temperatures and stratification on the balance between heterotrophy and autotrophy both within species and within assemblages of phytoplankton. Will mixotrophic flagellates increase in dominance because they can survive winter darkness better than strictly autotrophic phytoplankton at higher temperatures? Will stronger stratification and oligotrophy in summer in some regions increasingly favor mixotrophs over strictly autotrophic phytoplankton? How will this influence zooplankton populations and higher trophic levels?
Mixotrophic ciliates are undoubtedly a major component of pelagic food webs across the Arctic and surprisingly, also a major component of photosynthetic biomass. The routine methods of chlorophyll a collection need to be revised in the light of recent data showing the importance of green ciliates in total photosynthetic biomass. Specifically, vacuum filtration through membrane filters may result in significant losses of mixotrophic chlorophyll as fragile plankton cells are destroyed and release their cellular content to the filtrate. We still know little about the physiological ecology of mixotrophs in polar waters and a number of important questions remain to be answered. Is high mixotrophic ciliate biomass linked to blooms of particular types of phytoplankton as a source of nutrients and plastids? Are Micromonas sp. and other mixotrophic flagellates such as cryptophytes the primary prey and source of plastids for ciliates? This appears to be true for Mesodinium spp. which consume certain cryptophytes (Johnson et al., 2016), but is it true for plastid-retaining oligotrichs as well? Micromonas is a good source of plastids for at least some species of plastid-retaining oligotichs (Stoecker et al., 1988/1989). How does seasonal 24-h light or darkness influence their survival and growth? What effects do mixotrophs have on primary and secondary production in the Arctic? How does mixotrophy among protistan prey influence trophic transfer efficiencies to macrozooplankton and fish? Does a mixotrophic microbial food web, starting with small mixotrophic flagellates that are in turn consumed large mixotrophic ciliates, support zooplankton production during most of the year? To answer these questions will take a variety of approaches: field sampling to quantify populations of mixotrophic and non-mixotrophic planktonic protists, laboratory and field experiments to determine the contributions of phagotrophy and photosynthesis to the metabolism and growth of Arctic mixotrophs, experimental manipulations to determine the effects of temperature, irradiance, and inorganic nutrient concentrations and ratios on the balance of heterotrophy and autotrophy within mixotrophs as well as the contribution of strict heterotrophs, strict autotrophs and mixotrophs to plankton assemblages. Modeling will be necessary to understand the effects of mixotrophy on food webs and biogeochemical cycling in Arctic planktonic ecosystems and to predict what effects climate change might have on mixotrophs and their roles in Arctic Seas.
Author Contributions
DS and PL co-wrote the manuscript. DS had primary responsibility for constructing the tables, PL for drafting the figures.
Conflict of Interest Statement
The authors declare that the research was conducted in the absence of any commercial or financial relationships that could be construed as a potential conflict of interest.
Acknowledgments
DS thanks UMCES, Horn Point Laboratory for providing the resources needed to prepare this contribution and MD Johnson for suggesting we review mixotrophy in polar ecosystems.
References
Anderson, R., Jurgens, K., and Hansen, P. J. (2017). Mixotrophic phytoflagellate bacterivory field measurements strongly biased by standard approaches: a case study. Front. Microbiol. 8:1398. doi: 10.3389/fmicb.2017.01398
Andersson, A., Falk, S., Samuelsson, G., and Hagström, A. (1989). Nutritional characteristics of a mixotrophic nanoflagellate, Ochromonas sp. Microb. Ecol. 17, 251–262. doi: 10.1007/BF02012838
Ardyna, M., Babin, M., Devred, E., Forest, A., Grosselin, M., Raimbault, P., et al. (2017). Shelf-basin gradients shape ecological phytoplankton niches and community composition in the coastal Arctic Ocean (Beaufort Sea). Limnol. Oceanogr. 62, 2113–2132. doi: 10.1002/lno.10554
Ardyna, M., Gosselin, M., Michel, C., Poulin, M., and Tremblay, J. E. (2011). Environmental forcing of phytoplankton community structure and function in the Canadian High Arctic: contrasting oligotrophic and eutrophic regions. Mar. Ecol. Prog. Ser. 442, 37–57. doi: 10.3354/meps09378
Arrigo, K. R., Perovich, D. K., Pickart, R. S., Brown, Z. W., van Dijken, G. L., Lowry, K. E., et al. (2014). Phytoplankton blooms beneath the sea ice in the Chukchi sea. Deep Sea Res. II 105, 1–16. doi: 10.1016/j.dsr2.2014.03.018
Azam, F., Fenchel, T., Field, J. G., Gray, J. S., Meyer Reil, L. A., and Thingstad, F. (1983). The ecological role of water-column microbes in the sea. Mar. Ecol. Prog. Ser. 10, 257–263. doi: 10.3354/meps010257
Blais, M., Ardyna, M., Gosselin, M., Dumont, D., Belanger, S., and Tremblay, J. E. (2017). Contrasting interannual changes in phytoplankton productivity and community structure in the coastal Canadian Arctic Ocean. Limnol. Oceanogr. 62, 2480–2497. doi: 10.1002/lno.10581
Brown, Z. W., and Arrigo, K. R. (2012). Contrasting trends in sea ice and primary production in the Bering Sea and Arctic Ocean. ICES J. Mar. Sci. 69, 1180–1193 doi: 10.1093/icesjms/fss113
Burkholder, J. M., Glibert, P. M., and Skelton, H. M. (2008). Mixotrophy, a major mode of nutrition for harmful algal species in eutrophic waters. Harm. Algae 8, 77–93. doi: 10.1016/j.hal.2008.08.010
Calbet, A., Bertos, M., Fuentes-Grunewald, C., Alacid, E., Figueroa, R., Renom, B., et al. (2011a). Intraspecific variability in Karlodinium veneficum: growth rates, mixotrophy, and lipid composition. Harm. Algae 10, 654–667. doi: 10.1016/j.hal.2011.05.001
Calbet, A., Riisgaard, K., Saiz, E., Zamora, S., Stedmon, C., and Nielsen, T. G. (2011b). Phytoplankton growth and microzooplankton grazing along a sub-Arctic fjord (Godthåbsfjord, west Greenland). Mar. Ecol. Prog. Ser. 442, 11–22. doi: 10.3354/meps09343
Campbell, R. G., Sherr, E. B., Ashjian, C. J., Plourde, S., Sherr, B. F., Hill, V., et al. (2009). Mesozooplankton prey preference and grazing impact in the western Arctic Ocean. Deep Sea Res. II 56, 1274–1289. doi: 10.1016/j.dsr2.2008.10.027
Carmack, E., and Wassmann, P. (2006). Food webs and physical-biological coupling on pan-Arctic shelves: unifying concepts and comprehensive perspectives. Prog. Oceanogr. 71, 446–477. doi: 10.1016/j.pocean.2006.10.004
Caron, D. A. (2017). Acknowledging and incorporating mixed nutrition into aquatic protistan ecology, finally. Environ. Microbiol. Rep. 9, 41–43. doi: 10.1111/1758-2229.12514
Crawford, D. W. (1989). Mesodinium rubrum: the phytoplankter that wasn't. Mar. Ecol. Prog. Ser. 58, 161–174.
Crawford, D. W., and Lindholm, T. (1997). Some observations on vertical distribution and migration of the phototrophic ciliate Mesodinium rubrum (=Myrionecta rubra) in a stratified brackish inlet. Aquat. Microb. Ecol. 13, 267–274. doi: 10.3354/ame013267
Czypionka, T., Vargas, C. A., Silva, N., Daneti, G., Gonzalez, H. E., and Iriate, J. L. (2011). Importance of mixotrophic nanoplankton in Aysen Fjord (Southern Chile) during austral winter. Cont. Shelf Res. 31, 216–224. doi: 10.1016/j.csr.2010.06.014
Dolan, J. R., and Perez, M. T. (2000). Costs, benefits and characteristics of mixotrophy in marine oligotrichs. Freshw. Biol. 45, 227–238. doi: 10.1046/j.1365-2427.2000.00659.x
Dutz, J., and Peters, J. (2008). Importance and nutritional value of large ciliates for the reproduction of Acartia clausi during the post spring-bloom period in the North Sea. Aquat. Microb. Ecol. 50, 261–277. doi: 10.3354/ame01168
Erga, S. R., Ssebiyonga, N., Hamre, B., Frette, O., Rey, F., and Drinkwater, K. (2014). Nutrients and phytoplankton biomass distribution and activity at the Barents Sea Polar Front during summer near Hopen and Storbanken. J. Mar. Syst. 130, 181–192. doi: 10.1016/j.jmarsys.2012.12.008
Estep, K. W., Davis, P. G., Keller, M. D., Sieburth, J., and Mc, N. (1986). How important are oceanic algal nanoflagellates in bacterivory? Limnol. Oceanogr. 31, 646–650.
Estrada, M., Bayer-Giraldi, M., Felipe, J., Marrase, C., Sala, M. M., and Vidal, M. (2009). Light and nutrient effects on microbial communities collected during spring and summer in the Beaufort Sea. Aquat. Microb. Ecol. 54, 217–231. doi: 10.3354/ame01268
Flynn, K. J., Stoecker, D. K., Mitra, A., Raven, J. A., Glibert, P. M., Hansen, P. J., et al. (2013). Misuse of the phytoplankton zooplankton dichotomy: the need to assign organisms as mixotrophs within plankton functional types. J. Plankton Res. 35, 3–11. doi: 10.1093/plankt/fbs062
Franzè, G., and Lavrentyev, P. J. (2014). Microzooplankton growth rates examined across a temperature gradient in the Barents Sea. PLoS ONE 9:e86429. doi: 10.1371/journal.pone.0086429
Franzè, G., and Lavrentyev, P. J. (2017). Microbial food web structure and dynamics across a natural temperature gradient in a productive polar shelf system. Mar. Ecol. Prog. Ser. 569, 89–102. doi: 10.3354/meps12072
Garcia-Cuetos, L., Moestrup, O., and Hansen, P. J. (2012). Studies on the genus Mesodinium, II. Ultrastructural and molecular investigations of five marine species help clarifying the taxonomy. J. Eukaryt. Microbiol. 59, 374–400. doi: 10.1111/j.1550-7408.2012.00630.x
Gustafson, D. E., Stoecker, D. K., Johnson, M. D., Van Heukelem, W. F., and Sneider, K. (2000). Cryptophyte algae are robbed of their organelles by the marine ciliate Mesodinium rubrum. Nature 405, 1049–1052. doi: 10.1038/35016570
Hansen, B., Christiansen, S., and Pedersen, G. (1996). Plankton dynamics in the marginal ice zone of the central Barents Sea during spring: carbon flow and structure of the grazer food chain. Polar Biol. 16, 115–128. doi: 10.1007/BF02390432
Hansen, P. J. (2011). The role of photosynthesis and food uptake for the growth of marine mixotrophic dinoflagellates. J. Eukaryot. Microbiol. 58, 203–214. doi: 10.1111/j.1550-7408.2011.00537.x
Hansen, P. J., and Hjorth, M. (2002). Growth and grazing responses of Chrysochromulina ericina (Prymnesiophyceae): the role of irradiance, prey concentration and pH. Mar. Biol. 141, 975–983. doi: 10.1007/s00227-002-0879-5
Hartmann, M., Grub, C., Tarran, G. A., Martin, A. P., Burkill, P. H., Scanlan, D. J., et al. (2012). Mixotrophic basis of Atlantic oligotrophic ecosystem. Proc. Natl. Acad. Sci. U.S.A. 109, 5756–5760. doi: 10.1073/pnas.1118179109
Herfort, L., Peterson, T. D., Prahl, F. G., McCue, L. A., Needoba, J. A., Crump, B. C., et al. (2012). Red waters of Myrionecta rubra are biogeochemical hotspots for the Columbia River estuary with impacts on primary/secondary productions and nutrient cycles. Estuar. Coasts 35, 878–891. doi: 10.1007/s12237-012-9485-z
Hunt, G. L., and Megrey, B. (2005). Comparison of the biophysical and trophic characteristics of the Bering and Barents Seas. ICES J. Mar. Sci.62, 1245–1255. doi: 10.1016/j.icesjms.2005.04.008
Iversen, K. R., and Seuthe, L. (2011). Seasonal microbial processes in a high-latitude fjord (Kongsfjorden, Svalbard): I. Heterotrophic bacteria, picoplankton and nanoflagellates. Polar Biol. 34, 731–749. doi: 10.1007/s00300-010-0929-2
Jacobson, D. M., and Anderson, D. M. (1996). Widespread phagocytosis of ciliates and other Protists by marine mixotrophic and heterotrophic thecate dinoflagellates. J. Phycol. 32, 279–285. doi: 10.1111/j.0022-3646.1996.00279.x
Jeong, H. J., Doo, D., Park, J. Y., Song, J. Y., Kim, S. T., Lee, S. H., et al. (2005). Feeding by phototrophic red-tide dinoflagellates: five species newly revealed and six species previously known to be mixotophic. Aquat. Microb. Ecol. 40, 133–150. doi: 10.3354/ame040133
Jeong, H. J., Yoo, Y. D., Kim, J. S., Seong, K. A., Kang, N. S., and Kim, T. H. (2010). Growth, feeding and ecological roles of the mixotrophic and heterotrophic dinoflagellates in marine planktonic food webs. Ocean Sci. J. 45, 65–91. doi: 10.1007/s12601-010-0007-2
Ji, R. B., and Franks, P. J. S. (2007). Vertical migration of dinoflagellates: model analysis of strategies, growth, and vertical distribution patterns. Mar. Ecol. Prog. Ser. 344, 49–61. doi: 10.3354/meps06952
Jiang, Y., Yang, E. J., Min, J. O., Kim, T. W., and Kang, S. H. (2015). Vertical variation of pelagic ciliate communities in the western Arctic Ocean. Deep Sea Res. II 120, 103–113. doi: 10.1016/j.dsr2.2014.09.005
Johnsen, G., Norli, M., Moline, M., Robbins, I., von Quillfeldt, C., Sørensen, K., et al. (2018). The advective origin of an under-ice spring bloom in the Arctic Ocean using multiple observational platforms. Polar Biol. 41, 1197–1216. doi: 10.1007/s00300-018-2278-5
Johnson, M. D. (2015). Inducible mixotrophy in the dinoflagellate Prorocentrum minimum. J Eukaryt. Microbiol. 62, 431–443. doi: 10.1111/jeu.12198
Johnson, M. D., Beaudoin, D. J., Laza-Martinez, A., Dyhrman, S. T., Fensin, E., Lin, S., et al. (2016). The genetic diversity of Mesodinium and associated cryptophytes. Front. Microbiol. 7:2017. doi: 10.3389/fmicb.2016.02017
Johnson, M. D., and Stoecker, D. K. (2005). Role of feeding in growth and photophysiology of Myrionecta rubra. Aquat. Microb. Ecol. 39, 303–312. doi: 10.3354/ame039303
Jones, H. L. J., Leadbeater, B. S. C., and Green, J. C. (1993). Mixotrophy in marine species of Chrysochromulina (Prymnesiophyceae): ingestion and digestion of a small green flagellate. J. Mar. Biol. Assoc. U.K. 73, 283–296. doi: 10.1017/S0025315400032859
Keck, A., Wiktor, J., Hapter, R., and Nilsen, R. (1999). Phytoplankton assemblages related to physical gradients in an arctic, glacier-fed fjord in summer. ICES J. Mar. Sci. 56, 203–214. doi: 10.1006/jmsc.1999.0631
Keller, M. D., Shapiro, L. P., Haugen, E. M., Cucci, T. L., Sherr, E. B., and Sherr, B. F. (1994). Phagotrophy of fluorescently labeled bacteria by an oceanic phytoplankter. Microb. Ecol. 28, 39–52. doi: 10.1007/BF00170246
Kwok, R., and Rothrock, D. A. (2009). Decline in Arctic sea ice thickness from submarine and ICES records: 1958–2008. Geophys. Res. Lett. 36:L15501. doi: 10.1029/2009GL039035
Lavrentyev, P. J. (2012). Microzooplankton studies in the Kara Sea during the Yamal-Arctic 2012 expedition. Russ. Polar Res. 10, 24–26.
Lavrentyev, P. J., and Franzè, G. (2017). CarbonBridge May 2014: Microzooplankton Biomass Distribution in the Waters Northwest of Spitsbergen. University of Akron, PANGAEA.
Legrand, C., Granéli, E., and Carlsson, P. (1998). Induced phagotrophy in the photosynthetic dinoflagellate Heterocapsa triqueta. Aquat. Microb. Ecol. 15, 65–75. doi: 10.3354/ame015065
Leles, S. G., Mitra, A., Flynn, K. J., Stoecker, D. K., Hansen, P. J., Calbet, A., et al. (2017). Oceanic protists with different forms of acquired phototrophy display contrasting biogeographies and abundance. Proc. R. Soc. B. 284:20170664. doi: 10.1098/rspb.2017.0664
Levinsen, H., Nielsen, T. G., and Hansen, B. W. (2000a). Annual succession of marine pelagic protozoans in Disko Bay, West Greenland, with emphasis on winter dynamics. Mar. Ecol. Prog. Ser. 206, 119–134. doi: 10.3354/meps206119
Levinsen, H., Turner, J. T., Nielsen, T. G., and Hansen, B. W. (2000b). On trophic coupling between protists and copepods in arctic marine ecosystems. Mar. Ecol. Prog. Ser. 204, 65–77. doi: 10.3354/meps204065
Li, A., Stoecker, D. K., and Adolf, J. E. (1999). Feeding, pigmentation, photosynthesis and growth of the mixotrophic dinoflagellate Gyrodinium galatheanum. Aquat. Microb. Ecol. 19, 163–176.
Li, A., Stoecker, D. K., and Coats, D. W. (2000). Mixotrophy in Gyrodinium galatheanum (Dinophyceae): grazing responses to light intensity and inorganic nutrients. J. Phycol. 36, 33–45. doi: 10.1046/j.1529-8817.2000.98076.x
Li, W. K., McLaughlin, F. A., Lovejoy, C., and Carmack, E. C. (2009). Smallest algae thrive as the Arctic Ocean freshens. Science 326, 539–539. doi: 10.1126/science.1179798
Lovejoy, C., Legendre, L., Martineau, M. J., Bacle, J., and von Quillfeldt, C. H. (2002). Distribution of phytoplankton and other protists in the North Water. Deep Sea Res. II 49, 5027–5047. doi: 10.1016/S0967-0645(02)00176-5
Lovejoy, C., Vincent, W. F., Bonilla, S., Roy, S., Martineau, M. J., Terrado, R., et al. (2007). Distribution, phylogeny, and growth of cold-adapted picoprasinophytes in Arctic Seas. J. Phycol. 43, 78–89. doi: 10.1111/j.1529-8817.2006.00310.x
Maranger, R., Bird, D. F., and Price, N. M. (1998). Iron acquisition by photosynthetic marine phytoplankton from ingested bacteria. Nature 396, 248–251 doi: 10.1038/24352
McKenzie, C. H., Deibel, D., Paranjape, M. A., and Thompson, R. J. (1995). The marine mixotroph Dinobryon balticum (Chrysophyceae) – phagotrophy and survival in a cold ocean. J. Phycol. 31, 19–24. doi: 10.1111/j.0022-3646.1995.00019.x
McKie-Krisberg, Z. M., Gast, R. J., and Sanders, R. W. (2015). Physiological responses of three species of Antarctic mixotrophic phytoflagellates to changes in light and dissolved nutrients. Microb. Ecol. 70. 21–29. doi: 10.1007/s00248-014-0543-x
McKie-Krisberg, Z. M., and Sanders, R. W. (2014). Phagotrophy by the picoeukaryotic green alga Micromonas: implications for Arctic Oceans. ISME J. 8, 1953–1961. doi: 10.1038/ismej.2014.16
McManus, G. B., Schoener, D. M., and Haberlandt, K. (2012). Chloroplast symbiosis in a marine ciliate: ecophysiology and the risks and rewards of hosting foreign organelles. Front. Microbiol. 3:321. doi: 10.3389/fmicb.2012.00321
McMinn, A., and Martin, A. (2013). Dark survival in a warming world. Proc. R. Soc. B 280:20122909. doi: 10.1098/rspb.2012.2909
Metfies, K., von Appen, W. J., Kilias, E., Nicolaus, A., and Nothig, E. M. (2016). Biogeography and photosynthetic biomass of Arctic marine pico-eukaryotes during summer of the record sea ice minimum 2012. PLoS ONE 11:e0148512. doi: 10.1371/journal.pone.0148512
Millette, N. C., Pierson, J. J., Aceves, A., and Stoecker, D. K. (2017). Mixotrophy in Heterocapsa rotundata: a mechanism for dominating the winter phytoplankton. Limnol. Oceangr. 62, 836–845. doi: 10.1002/lno.10470
Mitra, A., Flynn, K. A., Tillmann, U., Raven, J. A., Caron, D., Stoecker, D. K., et al. (2016).Defining planktonic functional groups on mechanisms for energy and nutrient acquisition: incorporation of diverse mixotrophic strategies. Protist 167, 106–120. doi: 10.1016/j.protis.2016.01.003
Mitra, A., Flynn, K. J., Burkholder, J. M., Berge, T., Calbet, A., Raven, J. A., et al. (2014). The role of mixotrophic protists in the biological carbon pump. Biogeosciences 11, 995–1005. doi: 10.5194/bg-11-995-2014
Möller, E. F., Nielsen, T. G., and Richardson, K. (2006). The zooplankton community in the Greenland Sea: composition and role in carbon turnover. Deep Sea Res. I. 53, 76–93. doi: 10.1016/j.dsr.2005.09.007
Montagnes, D. J. S., Berges, J. A., Harrison, P. J., and Taylor, F. J. R. (1994). Estimating carbon, nitrogen, protein, and chlorophyll-a from volume in marine-phytoplankton. Limnol. Oceangr. 39, 1044–1060.
Moorthi, S., Caron, D. A., Gast, R. J., and Sanders, R. W. (2009). Mixotrophy: a widespread and important ecological strategy for planktonic and sea-ice nanoflagellates in the Ross Sea, Antarctica. Aquat. Microb. Ecol. 54, 269–277. doi: 10.3354/ame01276
Moorthi, S. D., Ptacnik, R., Sanders, R. W., Fischer, R., Busch, M., and Hillebrand, H. (2017). The functional role of planktonic mixotrophs in altering seston stoichiometry. Aquat. Microb. Ecol. 79, 235–245. doi: 10.3354/ame01832
Not, F., Massana, R., Latasa, M., Marie, D., Colson, C., Eikrem, W., et al. (2005). Late summer community composition and abundance of photosynthetic picoeukaryotes in Norwegian and Barents Seas. Limnol. Oceanogr. 50, 1677–1686. doi: 10.4319/lo.2005.50.5.1677
Olli, K., Riser, C. W., Wassmann, P., Rat'kova, T., Arashkevich, E., and Pasternak, A. (2002). Seasonal variation in vertical flux of biogenic matter in the marginal ice zone and the central Barents Sea. J. Mar. Syst. 38, 189–204. doi: 10.1016/S0924-7963(02)00177-X
Olson, M. B., and Strom, S. L. (2002). Phytoplankton growth, microzooplankton herbivory and community structure in the southeast Bering Sea: insight into the formation and temporal persistence of an Emiliania huxleyi bloom. Deep Sea Res. II 49, 5969–5990. doi: 10.1016/S0967-0645(02)00329-6
Oziel, L., Neukermans, G., Ardyna, M., Lancelot, C., Tison, J. L., Wassmann, P., et al. (2017). Role for Atlantic inflows and sea ice loss on shifting phytoplankton blooms in the Barents Sea. J. Geophys. Res. Oceans 122, 5121–5139. doi: 10.1002/2016JC012582
Pabi, S., van Dijken, G. L., and Arrigo, K. R. (2008). Primary production in the Arctic Ocean, 1998-2006. J. Geophys. Res. Oceans 113:C08005. doi: 10.1029/2007JC004578
Park, J. S., Myung, G., Kim, H. S., Cho, B. C., and Yih, W. (2007). Growth responses of the marine photosynthetic ciliate Myrionectra rubra to different cryptomonad strains. Aquat. Microb. Ecol. 48, 83–90. doi: 10.3354/ame048083
Park, M. G., Kim, S., Kim, H. S., Myung, G., Kang, Y. G., and Yih, W. H. (2006). First successful culture of the marine dinoflagellate Dinophysis acuminata. Aquat. Microb. Ecol. 45, 101–106. doi: 10.3354/ame045101
Putt, M. (1990). Abundance, chlorophyll content and photosynthesis rates of ciliates in the Nordic Seas during summer. Deep Sea Res. 37, 1713–1731. doi: 10.1016/0198-0149(90)90073-5
Rat'kova, T. N., and Wassmann, P. (2002). Seasonal variation and spatial distribution of phyto- and protozooplankton in the central Barents Sea. J. Mar. Syst. 38, 47–75. doi: 10.1016/S0924-7963(02)00169-0
Reigstad, M., Carroll, J., Slagstad, D., Ellingsen, I. H., and Wassmann, P. (2011). Intra-regional comparison of productivity, carbon flux and ecosystem composition within the northern Barents Sea. Prog. Oceanogr. 90, 33–46. doi: 10.1016/j.pocean.2011.02.005
Riisgaard, K., and Hansen, P. J. (2009). Role of food uptake for photosynthesis, growth and survival of the mixotrophic dinoflagellate Dinophysis acuminata. Mar. Ecol. Prog. Ser. 381, 51–62. doi: 10.3354/meps07953
Rose, J. M., and Caron, D. A. (2007). Does low temperature constrain the growth rates of heterotrophic protists? Evidence and implications for algal blooms in cold waters. Limnol. Oceanogr. 52, 886–895. doi: 10.4319/lo.2007.52.2.0886
Saiz, E., Calbet, A., Isari, S., and Antó, M. others (2013). Zooplankton distribution and feeding in the Arctic Ocean during a Phaeocystis pouchetii bloom. Deep Sea Res. I 72, 17–33. doi: 10.1016/j.dsr.2012.10.003
Sanders, R. W., and Gast, R. J. (2012). Bacterivory by phototrophic picoplankton and nanoplankton in Arctic waters. FEMS Microbiol. Ecol. 82, 42–253. doi: 10.1111/j.1574-6941.2011.01253.x
Sarmento, H., Montoya, J. M., Vázquez-Domínguez, E., Vaqué, D., and Gasol, J. M. (2010). Warming effects on marine microbial food web processes: how far can we go when it comes to predictions? Philos. Trans. R. Soc. Lond. Ser. B. Biol. Sci. 365, 2137–2149. doi: 10.1098/rstb.2010.0045
Schoener, D. M., and McManus, G. B. (2012). Plastid retention, use, and replacement in a kleptoplastidic ciliate. Aquat. Microb. Ecol. 67, 177–187. doi: 10.3354/ame01601
Selosse, M. A., Charpin, M., and Not, F. (2017). Mixotrophy everywhere on land and in water: the grand ecart hypothesis. Ecol. Lett. 20, 246–263. doi: 10.1111/ele.12714
Seuthe, L., Iversen, K. R., and Narcy, F. (2011a). Microbial processes in a high–latitude fjord (Kongsfjorden, Svalbard): ciliates and dinoflagellates. Polar Biol. 34, 751–766. doi: 10.1007/s00300-010-0930-9
Seuthe, L., Topper, B., Reigstad, M., Thyrhaug, R., and Vaquer-Sunyer, R. (2011b). Microbial communities and processes in ice covered Arctic waters of the northwestern Fram Strait (75 to 80° N) during the vernal pre-bloom phase. Aquat. Microb. Ecol. 64, 253–266. doi: 10.3354/ame01525
Sherr, E. B., Sherr, B. F., and Hartz, A. J. (2009). Microzooplankton grazing impact in the Western Arctic Ocean. Deep Sea Res. II 56, 1264–1273. doi: 10.1016/j.dsr2.2008.10.036
Sherr, E. B., Sherr, B. F., and Ross, C. (2013). Microzooplankton grazing impact in the Bering Sea during spring sea ice conditions. Deep Sea Res II 94, 57–67. doi: 10.1016/j.dsr2.2013.03.019
Slagstad, D., Ellingsen, I. H., and Wassmann, P. (2011). Evaluating primary and secondary production in an Arctic Ocean void of summer sea ice: an experimental simulation approach. Prog. Oceanogr. 90, 117–131. doi: 10.1016/j.pocean.2011.02.009
Smalley, G. W., Coats, D. W., and Stoecker, D. K. (2003). Feeding in the mixotrophic dinoflagellate Ceratium furca is influenced by intracellular nutrient concentrations. Mar. Ecol. Prog. Ser. 262, 137–151. doi: 10.3354/meps262137
Smith, M., and Hansen, P. J. (2007). Interaction between Mesodinium rubrum and its prey: importance of prey concentration, irradiance and pH. Mar. Ecol. Prog. Ser. 338, 61–70. doi: 10.3354/meps338061
Stoecker, D. K., Hansen, P. J., Caron, D. A., and Mitra, A. (2017). Mixotrophy in the marine plankton. Annu. Rev. Mar. Sci. 9, 311–335. doi: 10.1146/annurev-marine-010816-060617
Stoecker, D. K., Johnson, M. D., de Vargas, C., and Not, F. (2009).Acquired phototrophy in aquatic protists. Aquat. Microb. Ecol. 57, 279–310. doi: 10.3354/ame01340
Stoecker, D. K., and Michaels, A. E. (1991). Respiration, photosynthesis and carbon metabolism in planktonic ciliates. Mar. Biol. 108, 441–447. doi: 10.1007/BF01313654
Stoecker, D. K., Silver, M. W., Michaels, A. E., and Davis, L. H. (1988). Obligate mixotrophy in Laboea strobila, a ciliate which retains chloroplasts. Mar. Biol. 99, 415–423.
Stoecker, D. K., Silver, M. W., Michaels, A. E., and Davis, L. H (1988/1989). Enslavement of algal chloroplasts by four Strombidium spp. (Ciliophora, Oligotrichida). Mar. Microb. Fd. Webs 3, 79–100.
Stoecker, D. K., Weigel, A. C., Stockwell, D. A., and Lomas, M. W. (2014). Microzooplankton: abundance, biomass and contribution to chlorophyll in the Eastern Bering Sea in summer. Deep Sea Res. II. 109, 134–144. doi: 10.1016/j.dsr2.2013.09.007
Strom, S. L., and Fredrickson, K. A. (2008). Intense stratification leads to phytoplankton nutrient limitation and reduced microzooplankton grazing in the Southeastern Bering Sea. Deep Sea Res. II 55, 1761–1774. doi: 10.1016/j.dsr2.2008.04.008
Terrado, R., Pasulka, A. L., Lie, A. A., Orphan, V. J., Heidelberg, K. B., and Caron, D. A. (2017). Autotrophic and heterotrophic acquisition of carbon and nitrogen by a mixotrophic chrysophyte established through stable isotope analysis. ISME J. 11, 2022–2034. doi: 10.1038/ismej.2017.68
Unrein, F., Gasol, J. M., Not, F., Forn, I., and Massana, R. (2014). Mixotrophic haptophytes are key bacterial grazers in oligotrophic coastal waters. ISME J. 8, 164–176. doi: 10.1038/ismej.2013.132
Unrein, F., Massana, R., Alonso-Saez, L., and Gasol, J. M. (2007). Significant year-round effect of small mixotrophic flagellates on bacterioplankton in an oligotrophic coastal system. Limnol. Oceanogr. 52, 456–469. doi: 10.4319/lo.2007.52.1.0456
Verity, P. G., Wassmann, P., Frischer, M. E., Howard-Jones, M. H., and Allen, A. E. (2002). Grazing of phytoplankton by microzooplankton in the Barents Sea during early summer. J. Mar. Syst. 38, 109–123. doi: 10.1016/S0924-7963(02)00172-0
Ward, B. A., and Follows, M. J. (2016). Marine mixotrophy increases trophic transfer efficiency, mean organism size and vertical carbon flux. Proc. Nat. Acad. Sci. U.S.A. 113, 2958–2963. doi: 10.1073/pnas.1517118113
Wassmann, P., and Reigstad, M. (2011). Future Arctic Ocean seasonal ice zones and implications for pelagic-benthic coupling. Oceanography 24, 220–231. doi: 10.5670/oceanog.2011.74
Wilken, S., Huisman, J., Naus-Weier, S., and Van Donk, E. (2013). Mixotrophic organisms become more heterotrophic with rising temperature. Ecol. Lett. 16, 225–233. doi: 10.1111/ele.12033
Yokoi, N., Matsuno, K., Ichinomiya, M., Yamaguchi, A., Nishino, S., Onodera, J., et al. (2016). Short-term changes in a microplankton community in the Chukchi Sea during autumn: consequences of a strong wind event. Biogeosciences 13, 913–923. doi: 10.5194/bg-13-913-2016
Yoo, Y. D., Jeong, H. J., Kim, M. S., Kang, N. S., Song, J. Y., Shin, W., et al. (2009). Feeding by phototrophic red-tide dinoflagellates on the ubiquitous marine diatom Skeletonema costatum. J. Eukaryt. Microbiol. 56, 413–420. doi: 10.1111/j.1550-7408.2009.00421.x
Yoo, Y. D., Seong, K. A., Jeong, H. J., Yih, W., Rho, J. R., Nam, S. W., et al. (2017). Mixotrophy in the marine red-tide cryptophyte Teleaulax amphioxeia and ingestion and grazing impact of cryptophytes on natural populations of bacteria in Korean coastal waters. Harm. Algae 68, 105–117. doi: 10.1016/j.hal.2017.07.012
Zhang, Q., Gradinger, R., and Zhou, Q. S. (2003). Competition within the marine microalgae over the polar dark period in the Greenland Sea of high Arctic. Acta Oceanol. Sin. 22, 233–242.
Keywords: mixotrophy, Arctic plankton, mixotrophic flagellates, mixotrophic ciliates, Arctic Ocean
Citation: Stoecker DK and Lavrentyev PJ (2018) Mixotrophic Plankton in the Polar Seas: A Pan-Arctic Review. Front. Mar. Sci. 5:292. doi: 10.3389/fmars.2018.00292
Received: 02 April 2018; Accepted: 30 July 2018;
Published: 22 August 2018.
Edited by:
Holly V. Moeller, University of California, Santa Barbara, United StatesReviewed by:
Rebecca Gast, Woods Hole Oceanographic Institution, United StatesSuzanne Strom, Western Washington University, United States
Copyright © 2018 Stoecker and Lavrentyev. This is an open-access article distributed under the terms of the Creative Commons Attribution License (CC BY). The use, distribution or reproduction in other forums is permitted, provided the original author(s) and the copyright owner(s) are credited and that the original publication in this journal is cited, in accordance with accepted academic practice. No use, distribution or reproduction is permitted which does not comply with these terms.
*Correspondence: Diane K. Stoecker, c3RvZWNrZXJAdW1jZXMuZWR1