- 1Department of Biology, California State University Northridge, Northridge, CA, United States
- 2Swire Institute of Marine Science, The University of Hong Kong, Hong Kong, Hong Kong
- 3Institut de Recherche pour le Développment, UMR 9220 ENTROPIE and Laboratoire d'Excellence CORAIL, Université de Perpignan, Perpignan, France
- 4School of Life Sciences, The Chinese University of Hong Kong, Hong Kong, Hong Kong
- 5Department of Geology, Graduate Program in Evolution, Ecology and Behavior, University at Buffalo, Buffalo, NY, United States
- 6Marine Laboratory, University of Guam, Mangilao, GU, United States
- 7Nicholas School of the Environment, Duke University, Beaufort, NC, United States
- 8Department of Ecology, Evolution and Marine Biology, University of California, Santa Barbara, Santa Barbara, CA, United States
- 9Scripps Institution of Oceanography, University of California, San Diego, San Diego, CA, United States
- 10Graduate School of Fisheries Science and Environmental Studies, Nagasaki University, Nagasaki, Japan
- 11Marine Biophysics Unit, Okinawa Institute of Science and Technology Graduate University, Okinawa, Japan
- 12School of Marine Science and Technology, Tokai University, Shizuoka, Japan
- 13Rosenstiel School of Marine and Atmospheric Science, University of Miami, Miami, FL, United States
- 14Marine Biodiversity Research Group, Faculty of Science, Ramkhamhaeng University, Bangkok, Thailand
- 15Hawai'i Institute of Marine Biology, University of Hawai'i at Mānoa, Kāne'ohe, HI, United States
- 16Sesoko Station, Tropical Biosphere Research Center, University of the Ryukyus, Okinawa, Japan
- 17Research Center for Subtropical Fisheries, Seikai National Fisheries Research Institute, Okinawa, Japan
- 18Department of Geography, Department of Geography and Marine Science Institute, University of California, Santa Barbara, Santa Barbara, CA, United States
- 19Department of Chemical Oceanography, Atmosphere and Ocean Research Institute, The University of Tokyo, Tokyo, Japan
Populations of marine organisms on coral reef islands (CRI) are connected in space and time by seawater that transports propagules of plants, animals, and algae. Yet, despite this reality, it is often assumed that routine replenishment of populations of marine organisms on CRI is supported by locally-sourced propagules (hereafter, larvae). Following large disturbances, however, distantly-sourced larvae from less disturbed CRI within a regional meta-population are likely to be important for local population recovery, but evaluating the roles of locally- vs. distantly-sourced larvae remains difficult. While larval sources are relatively well-known for some fishes, they remain virtually unknown for most taxa, particularly those associated with the benthos, as exemplified by scleractinian corals. This review focuses on reef recovery and larval connectivity. Using corals as examples, we argue that CRI can serve as natural laboratories in which studies of these issues can enhance understanding of coral reef community dynamics under future disturbance regimes. Rather than focusing on synthesizing empirical data, we focus on the capacity for CRI to realize their potential in this research area, concluding that progress is impeded by the limited breadth, detail, and spatio-temporal concordance of existing research. Using long-term observational programs of coral reefs in Mo'orea (French Polynesia), Okinawa (Japan), and St. John (US Virgin Islands) as examples of the data currently available, we make the case that new modes of multidisciplinary and collaborative research will be required to exploit the value of CRI in understanding the role of connectivity in mediating ecosystem resilience in a future affected by anthropogenic disturbances.
Marine Connectivity
Most generally, connectivity (Box 1) refers to the transport of fluid media (e.g., air, water), chemicals (e.g., nutrients, dissolved organic matter), and biota (e.g., adult organisms, reproductive propagules, microbes) among spatially distinct population patches (Hanski, 1999, 2002). Interest in demographic connectivity focuses mainly on the passage among populations of individuals and the genetic information they contain (Doherty et al., 1995; Planes and Fauvelot, 2002), thereby modulating the dynamics of local populations as well as the larger networks of populations to which they belong (Cowen et al., 2006; Holstein et al., 2014). Population connectivity is integrally related to metapopulation theory (Paris-Limouzy, 2011; Burgess et al., 2014), because it represents the capacity for exchanges among sub-populations to mediate ecosystem resilience (i.e., the ability to recover following disturbance) by supplying distantly-sourced recruits to augment locally-sourced recruits (Box 1). Given that local population dynamics can be strongly influenced by connectivity, this topic has featured prominently in ecological research for decades (Limouzy-Paris et al., 1997; Hanski, 2002; Cowen et al., 2003), and often has been presented as a dichotomy between connected vs. separated populations (Cowen et al., 2000). Recent work suggests connectivity is better considered as a continuum, along which populations can be intermittently connected by the periodic confluence of temporally constrained events (Kough and Paris, 2015).
Population connectivity is particularly relevant in the oceans, because water provides a dense fluid with buoyant and drag forces (Paris et al., 2013a) supporting the transport of organisms over large distances (Burgess et al., 2014) while differential advection and turbulence increase dispersion. Moreover, the majority of marine taxa has multi-stage life cycles and produce propagules capable of extensive transport by moving seawater (Wood et al., 2014). For many common benthic animals, the production of pelagic larvae supports dispersal among sessile adult populations (Paris-Limouzy, 2011; Burgess et al., 2014), for example, those found on the shores of coral reef islands (CRI). Connected by slow-moving seawater, CRI can create spatially segregated populations, among which the transport of pelagic larvae can mediate ecosystem resilience (Gunderson, 2000), although some oceanic currents can act as barriers to larval migration (Baums et al., 2006; Suzuki et al., 2016; Wood et al., 2016). Adults of highly motile taxa have the potential to move among CRI, although for many typical reef-resident taxa such as reef fishes, lobsters, and sea urchins, such dispersal appears to be infrequent (Saenz-Agudelo et al., 2011), and probably plays a modest role in connectivity relative to that facilitated by pelagic larvae (Botsford et al., 2009). Larval connectivity among CRI can be quantified as the proportional relationship at the population level of distantly-sourced larvae relative to the sum of locally- and distantly-sourced larvae recruiting at a site (Box 2). This ratio varies with time (e.g., seasonally) as a function of the integration time over which larval recruitment is measured.
Box 2. Relationship between connectivity and sources of recruits.
Conceptual relationship between connectivity and sources of recruits in benthic marine populations, defined as originating from the focal CRI RLocal, or another CRI RDistant. For a given site, the connectivity index would be expected to vary from ~ 0 (representing entirely local recruitment) to 1 (representing recruitment sourced entirely from distant sources). As conceived here, this simple ratio is independent of the magnitude or rate of overall recruitment.
CRI provide natural laboratories for studying population connectivity in a changing world (Kendall et al., 2013; Figure 1), which is an objective with broad utility in understanding the conditions favoring a return of community structure to an initial condition following a disturbance (i.e., ecosystem resilience; Gunderson, 2000). While CRI represent unique coral reef habitats that are tractable for the investigations of connectivity in mediating community recovery following disturbance, the principles they represent are applicable to a diversity of coral reef situations, for example, cohesive areas of reef that are being split into segregated communities through mortality, and fragmented habitats in multiple tropical ecosystems including mangroves, seagrasses, and soft sediments.
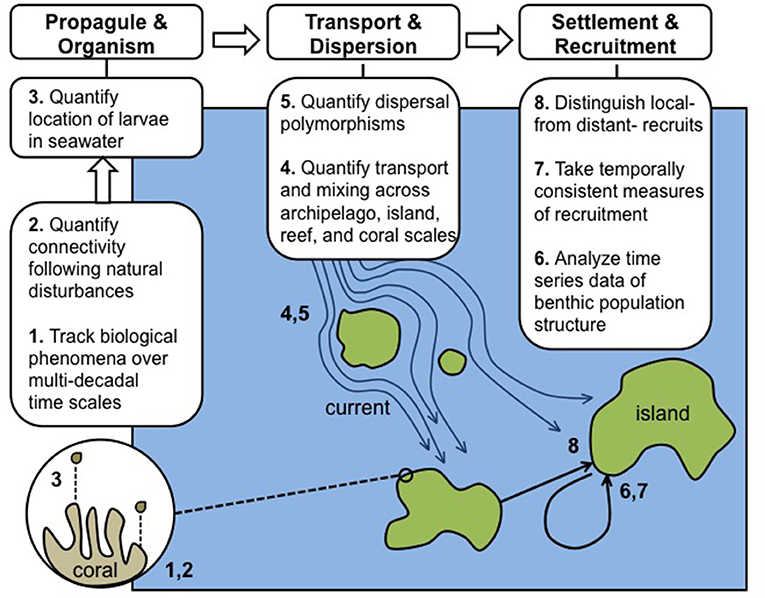
Figure 1. Schematic of eight domains (described in the text with corresponding 1–8) requiring integration, concurrent research, and further quantitative data to allow CRI to achieve their full potential in advancing understanding of the role of connectivity in coral reef resilience. The needs for integration and additional data are divided into three categories underscoring the chronology of biological events beginning with reproduction and ending with recruitment, as exemplified by scleractinian corals: responses at propagule and organism levels, pelagic larval transport processes, and analysis of post-settlement and recruitment patterns.
Previous studies have described the concepts associated with population connectivity in the marine realm (Cowen et al., 2006; Cowen and Sponaugle, 2009; Selkoe and Toonen, 2011), but many of these concepts require nuance in order to translate their meaning to CRI that have unique features associated with their physical oceanography, as well as the biological consequences of small and isolated populations (Paris et al., 2002; Kendall et al., 2013; Vaz et al., 2013). Many of these features differ from those affecting geographically large areas of coral reefs (e.g., continental barrier reefs; Lindo-Atichati et al., 2016), for example, because of the complex ways in which seawater flows among CRI having irregular shapes and deep underwater topography (Wolanski and Hamner, 1988; Paris et al., 2002; Baums et al., 2006). Submerged in the physical seascape surrounding CRI, near-shore reefs contain a diversity of organisms with contrasting life-history strategies that create multiple pathways for connectivity to mediate recruitment by locally- and distantly-sourced larvae (Figure 1). These features are likely to affect the resilience of coral reefs around CRI, and play an important role in determining whether populations of foundation species recover from landscape-scale disturbances, or remain in a depleted state (Munday et al., 2009). Clearly, the factors determining the dynamics of coral reefs around CRI are complex, and will not be fully explained by a single program focused on a single aspect of their ecology (e.g., connectivity). However, complexity does not imply intractability, and we suggest that a cohesive program focused on connectivity around CRI will greatly advance understanding of these issues. Ultimately, such a program will need to be augmented by studies of other processes, such as post-settlement success, the role of disturbances, and the factors controlling adult mortality, to realize its full potential.
Contrasting Population Dynamics Among Coral Reef Islands
Using a framework created by the theoretical and empirical understanding of connectivity in the marine environment (Hanski, 2002; Hastings and Botsford, 2006; Burgess et al., 2014; Thompson et al., 2018), we consider how time-series analyses of the community structure of coral reefs around CRI can be interpreted within a connectivity framework. While such a framework is relevant to understanding the dynamics of a wide variety of organisms having pelagic propagules, including many fishes, algae, and invertebrates, we constrain our review to considering the utility of measures of connectivity among populations of scleractinian corals in order to enhance understanding of coral reef community dynamics. Addressing this topic constitutes a significant research challenge, but providing definitive answers to outstanding questions is beyond the scope of what can be achieved with the available data.
Our objective is to highlight the needs for integration among studies, specifically addressing different disciplines such as physical oceanography, ecology, and genetics, and for specific types of data with the potential to deliver these answers. We underscore the importance of matched sets of complementary data that are collected on commensurate scales and can be used to support synthetic analyses within and among groups of CRI. We focus on scleractinians because they are the ecosystem engineers of coral reefs (Jones et al., 1994), and because they offer the advantage (compared to other taxa such as fishes and algae) that their settlement, recruitment, and growth provide an enduring calcified structure suitable for retrospective analyses of population dynamics. While adopting a restricted taxonomic approach, we recognize that other organisms (e.g., microbes, fish, echinoids, and algae) also support genetic exchange among CRI, and that research addressing connectivity for these groups is underway (e.g., Saenz-Agudelo et al., 2011; D'Aloia et al., 2015).
We illustrate our contribution using the shallow reefs of Mo'orea (French Polynesia), Okinawa (Japan), and St. John (US Virgin Islands), because: (a) they have decadal-scale records of coral reef community dynamics, (b) the records have been collected as part of larger programs co-located with observations of oceanographic conditions, recruitment, and population genetics, and (c) the projects are managed, or conducted, by the authors of the present contribution. Mo'orea, Okinawa, and St. John are surrounded by nearby CRI (i.e., within 10's−100's of kilometers), thereby creating the potential for locally- and distantly-sourced larvae to replenish populations following disturbances. The ecology of the coral reefs around each of these islands operates within different seascapes capturing dissimilar physical oceanography, regional-scale variation in patterns of organism diversity, disturbance histories, and local-scale anthropogenic effects created by human populations. While Mo'orea, Okinawa, and St. John provide examples of CRI within local-scale networks of other CRI, their seascape contexts suggest each CRI might interpret interactions with neighboring CRI in different ways. The coral reefs around these locations illustrate a range of fates similar to those affecting coral reefs around the world (e.g., Bruno and Selig, 2007; Jackson et al., 2014). Some reefs around St. John have undergone a phase transition to macroalgae (Rogers and Miller, 2006; Edmunds, 2015), while the resilient outer reefs of Mo'orea represent a case of rapid recovery from major coral mortality caused by a crown-of-thorns (COTs) outbreak and a cyclone between 2007 and 2010 (Bramanti and Edmunds, 2016; Holbrook et al., 2018). Okinawa represents a resilient coral community supported by exceptionally high recruitment despite frequent major typhoons and historical, yet recurrent, COTs outbreaks (Goto et al., 2011; Edmunds et al., 2015).
Previously, analyses of coral communities from 1992 to 2013 around Mo'orea, Okinawa, and St. John have been used to highlight among-site differences in the benthic community dynamics (Edmunds et al., 2015), particularly in terms of their responses to disturbances and their condition (e.g., coral cover) as of 2013, the most recent sampling included in the previous analysis. In evaluating why these locations have responded differently to disturbances (Edmunds et al., 2015), contrasts in key biological processes among locations emerged, including the delivery of larvae to benthic surfaces (i.e., coral recruitment on terracotta tiles) and the density of juvenile corals (colonies ≤ 5-cm diameter) on natural reef surfaces. For example, coral recruitment varied three orders of magnitude among islands (Okinawa > Mo'orea > St. John), the density of juvenile corals differed an order of magnitude among islands (generally Mo'orea and Okinawa > St. John), and recruitment and density of juvenile corals showed strong variation among years (Edmunds et al., 2015). A proxy for recruitment success—the ratio of the density of juvenile corals to the smaller coral recruits—differed four orders of magnitude among locations (Mo'orea > St. John > Okinawa), suggesting that the “post-settlement gauntlet” (sensu Arnold et al., 2010) was an important factor driving differential resilience of coral reefs around these CRI. This earlier analysis underscored the role of post-settlement mortality and juvenile coral success in mediating differential coral community dynamics among locations. Here we focus on the role of pre-settlement events leading to propagule delivery in determining reef resilience and recovery following disturbances. Survival during propagule delivery and settlement is among the earliest links in the chain of events comprising recruitment success and subsequent reef resilience.
With evidence of dissimilar community dynamics at three CRI, the present study began as an analysis of the role of connectivity in driving temporal differences in coral reef community structure in these locations. We reasoned that factors determining connectivity would differ among locations in ways that could inform our understanding of community dynamics. A fundamental hypothesis has been developed and can be addressed by our review: Connectivity patterns provide insight into how future CRI community dynamics will be influenced by increasing rates of disturbance associated with climate change (Emanuel, 2005; Madin and Connolly, 2006).
Initial inspection of the data suggested this hypothesis might be true. For example, the nearest neighboring CRI, and their sizes, differ among the locations, with St. John as close as ~1 km to CRI ~500 m long, and 2–3 km from CRI ~15 km long. In contrast, Mo'orea is ~16 km from Tahiti which is 60 km long, and Okinawa is as close as ~0.5 km from CRI ~ 3 km long, and within 30 km there are numerous small CRI. Consistent with these observations, the areas of coral reef from which larvae can be sourced vary among locations. For example, a 100 km radius around each CRI encompasses different areas of reef, ranging from 159 km2 for Mo'orea, to 221 km2 for St. John, and 407 km2 for Okinawa (data: http://data.unep-wcmc.org/datasets/1). Likewise, the speeds of net prevailing surface currents also differ among locations, ranging from 11 to 25 cm s−1 for St. John, to 9–12 cm s−1 for Mo'orea, and 16–33 cm s−1 for Okinawa (data: http://bulletin.mercator-ocean.fr). Combining flow speed and distances to available source communities for coral larvae, yields estimates of the areas of reef within multiple days of seawater transport that differ among these CRI. While our observations and estimated potential larval source areas suggest that connectivity among nearby islands is a likely hypothesis to account for differential resilience among our focal CRI due to climate change, specifically quantifying these effects proved beyond the scope the data currently available.
Implications of Connectivity for the Response of Coral Reefs Around CRI to Disturbances
Coral reefs are subject to numerous disturbances causing coral mortality (Nyström et al., 2000; Bellwood et al., 2004; Osborne et al., 2011), and large perturbations to ecosystem structure and function (Hughes et al., 2007; Mumby et al., 2007). Many of these disturbances also modify the genetic structure within populations (Foster et al., 2012), and connectivity to nearby populations (Box 1). Therefore, not only can disturbances reduce local coral cover, they can also impede the capacity for population replenishment through the delivery of larvae from other locations. For example, most corals and their photosynthetic symbionts are negatively affected by temperature elevated beyond normal summer-time values (Hoegh-Guldberg, 1999), with persistence of these conditions causing death through bleaching (Hoegh-Guldberg, 1999; Hughes et al., 2017). Even exposure to sub-lethal temperatures, or warmer-than-normal seasonal low temperatures, can have lingering effects that can depress fecundity for at least a year (Szmant and Gassman, 1990). Elevated seawater temperature also increases larval mortality (Randall and Szmant, 2009), as well as accelerates the development of coral larvae, subsequently reducing their pelagic larval duration (PLD), and the maximum distances over which they are likely to be transported by currents (O'Connor et al., 2007). The multi-faceted effects of seawater temperature on corals therefore have the potential to affect connectivity among CRI, with the implications dependent on whether specific CRI are sources or sinks for coral larvae, and the spatial extent of disturbance to which they are exposed.
Disturbances with serious implications for coral reefs are associated with rising concentrations in atmospheric pCO2, which are driving: (1) elevated seawater temperature (Doney et al., 2012), (2) increasing intensity and frequency of severe tropical storms (Emanuel, 2005; Webster et al., 2005), and (3) declining seawater pH (i.e., ocean acidification; Doney et al., 2009). All three modes of disturbance have implications for connectivity among CRI (e.g., by mediating larval production, survival during dispersal, and settlement success), and the capacity for specific CRI to serve as larval sources or sinks (i.e., through regimes of widespread coral mortality or change in oceanic currents).
While insight into some aspects of the effects of global climate change on coral reefs is provided by decades of research on the thermal biology of corals (e.g., Coles and Jokiel, 1977; Fitt et al., 2001; Brown and Cossins, 2011), many studies lack mechanistic understanding and ecological relevance to the suite of conditions affecting corals in situ. Therefore, current knowledge provides an incomplete basis to evaluate the effects of rising temperature on reef corals around CRI and throughout tropical seas. Even less is known about the possible effects of ocean acidification for coral connectivity. Although some studies demonstrate adverse effects of elevated pCO2 on the development of coral larvae (Nakamura et al., 2011; Fabricius et al., 2017), and their growth and calcification when settled and metamorphosed (Albright et al., 2008; Albright and Langdon, 2011), it is not yet clear whether, but appears likely that, these effects have ecological relevance for connectivity.
The effects of hurricanes and cyclones on coral reefs are well-documented (e.g., Woodley et al., 1981; Massel and Done, 1993; Harmelin-Vivien, 1994), but their projected increased severity in the coming century (Emanuel, 2005; Mann and Emanuel, 2006) is likely to increase the extent, and the rate of formation, of vacant space that is available for coral recruitment. While increased frequency of major storm impacts could increase coral recruitment by creating open space, more frequent scouring of benthic surfaces and high sedimentation associated with coastal run off and physical disturbance of reef sediments could be expected to impede recruitment and frequently reset CRI communities to early post-disturbance states (Grigg, 1983). Large storms can increase ocean mixing (e.g., Bernardo et al., 2017) and result in net decreases in water column temperatures, and some corals may synchronize their reproduction to occur in the period just before wet seasons that are frequently associated with latitudinal shifts in intertropical convergence zones (ITCZ) and storms (Mendes and Woodley, 2002). Thus, coral populations around CRI might receive increased input of larvae associated with connectivity from nearby CRI under future climate scenarios of increased storm frequency and disturbance. However, the combination of physical connectivity among CRI with increasing disturbance frequency, may not favor ecological resilience of coral communities as the spatial scale of reef damage expands.
Storms reflect local weather that is mediated by climatic phenomena, including El Niño, La Niña, Pacific Decadal Oscillation (PDO), the Atlantic Multi-decadal Oscillation (AMO), and latitudinal shifts in the Intertropical Convergence Zones (ITCZ), which may also change in intensity as global temperature rises (Cai et al., 2014). These phenomena bring large-scale changes in local conditions including seawater temperature and oceanic circulation (Cai et al., 2014) that have the potential to alter the transport of pelagic larvae among CRI (Treml et al., 2015; Wood et al., 2016). The effects of these disturbances are likely to be intensified by rising sea level resulting from the melting of polar ice caps and steric thermal expansion of seawater (Stammer et al., 2013), which in turn, will affect physical connectivity among CRI (Veron, 1995). Coral reefs that fringe submarine volcanoes in deep water—for example, Mo'orea and Oahu—are unlikely to experience major changes in absolute reef area, or the ways in which seawater circulates among them, as sea level rises. In contrast, a rise in sea level of only a few decimeters will inundate shallow island platforms in locations such as the Maldives and the Bahamas (Khan et al., 2002; Dasgupta et al., 2009), thereby increasing the area of benthic surfaces for reef growth, and perhaps increasing the net transport of seawater among islands (Veron, 1995). On a smaller scale, increases in sea level of only a few centimeters could enhance the passage of seawater over reef crests separating outer- and back-reef communities (Monismith et al., 2013; Taebi and Pattiaratchi, 2014), and if the corals and algae at the crest are unable to accrete vertically to restrict the flow, this change could enhance connectivity between outer- and back-reef habitats and mediating their ecological resilience.
Finally, major disturbances, as well as numerous more minor and chronic mechanisms, are likely to alter species assemblages on CRI. The near-extirpation of Acropora spp. throughout the Caribbean by white band disease (Aronson and Precht, 2001) provides one example of this possibility. This loss increased the likelihood of local-recruitment for the coral community remaining, because with the rarity of spawning Acropora spp. (Aronson and Precht, 2001; Alvarez-Filip et al., 2009), many shallow Caribbean reefs now have high relative abundances of Porites astreoides (Green et al., 2008; Burman et al., 2012). Although spawning corals generally disperse greater distances than brooding corals (Connolly and Baird, 2010; Figueiredo et al., 2013), which favors strong connectivity among CRI, regional declines in population sizes of these corals are likely to reduce their total larval production. As species assemblages of reef corals and other taxa are modified through ecosystem degradation (Adjeroud et al., 2009; Done et al., 2015), the abundance of taxa producing larvae that can be transported large distances is likely to decrease, and probably will alter both connectivity among CRI and ecosystem resilience.
Achieving the Potential for CRI to Advance Studies of Coral Reef Recovery
The previous section highlights the complex processes that must be understood to evaluate the ecological implications of connectivity among CRI; achieving this understanding presents significant research challenges. There are compelling reasons to address these challenges because CRI can be used to answer long-standing questions regarding the dynamics of coral reef communities, both in CRI and more generally, throughout tropical regions (Table 1). Initially we reasoned that CRI for which time-series analyses of coral communities are well developed would provide opportunities to answer at least some of these questions. Yet despite the large quantities of data provided by the time series for our three focal sites, the data remained incomplete or spatio-temporally incompatible for one or more response variables (Table 2). Where data were more complete, often the sampling frequencies were inconsistent among variables, making it challenging to integrate data streams to achieve emergent properties of value in understanding of connectivity (Figure 2).
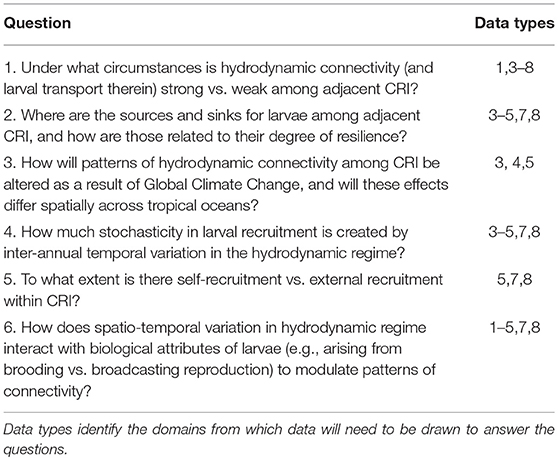
Table 1. Select questions addressing fundamental mechanisms mediating coral reef community dynamics in an age of strong anthropogenic disturbances that could be addressed using revised programs of integrated time-series analyses at CRI that address Domains 1–8 (in the text).
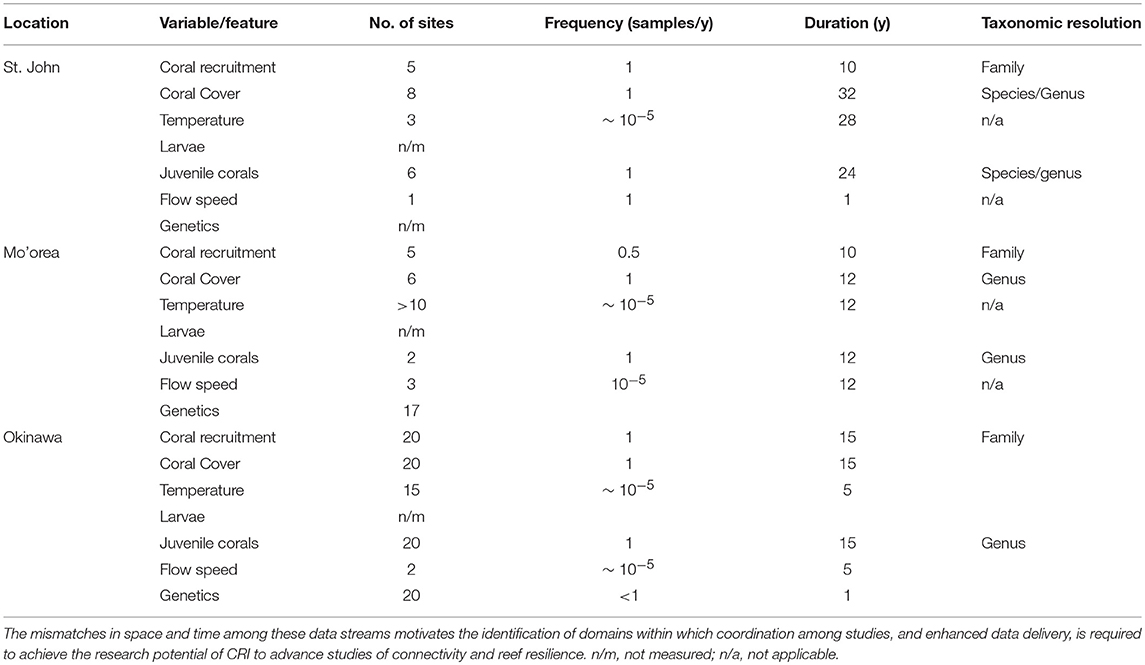
Table 2. Summary of concordance and mismatches among data types originating from time-series analyses of coral reef communities around three CRI, St. John, Mo'orea, and Okinawa.
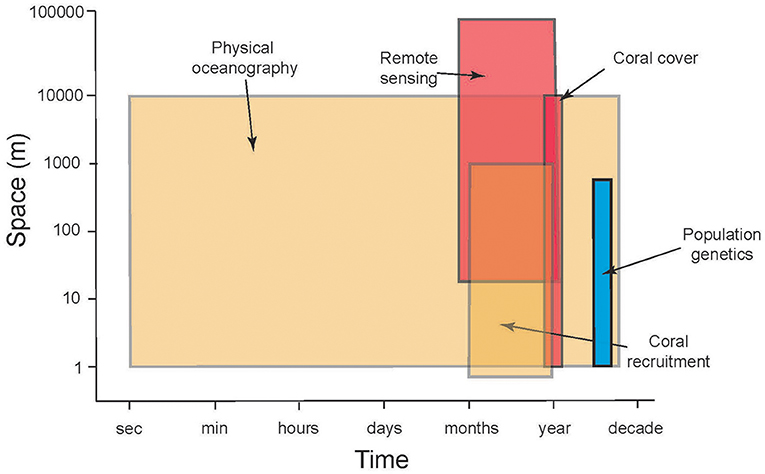
Figure 2. Schematic illustrating the mismatch between scales of space (ordinate) and time (frequency of sampling, abscissa) in time-series analyses of CRI that are relevant to evaluating the roles of connectivity to reef resilience following disturbance. Shading indicates approximate longevity (number of years that sampling is sustained) of repeated sampling: blue = 1 year, brown = ≤ 20 y, red = ≤ 30 y.
Although the biological and physical data available for Mo'orea, Okinawa, and St. John, are extensive by comparison to most CRI (Table 2), substantial limitations were encountered when we attempted to use them to quantify the role of connectivity in mediating coral community dynamics. Quantitative information from eight domains was required for this effort (described below), but in all cases the data available were limited. The temporal mismatch among data from CRI (Figure 2) is exemplified by coral recruitment, which typically is estimated by using settlement tiles. Such tiles rarely are made from the same material among studies; they are not deployed in a standardized orientation and duration; and their deployments are not matched in space or time to local measurements of physical oceanographic processes. Genetic population structure typically was measured for a small number of taxa representing a limited fraction of the local biodiversity. Sampling of biological and physical processes rarely was repeated over multiple years, and empirical data to parameterize changes in connectivity as a result of global climate change were scant. Some of these issues reflect the origins of time-series studies of coral reefs around CRI, which usually began as isolated efforts with modest research budgets, and were situated in locations of convenience such as near existing marine labs, or haphazardly with respect to the ecology and physical oceanography of a specific region. Even the longest of these times-series studies are not yet adequate for understanding climate change effects. These limitations leave investigators with an imperfect, or largely absent, set of tools for studying the role of connectivity in mediating coral reef resilience.
Nonetheless, and despite the aforementioned limitations, CRI provide a unique opportunity to study population connectivity in systems likely to change in coming decades (Kendall et al., 2013; Edmunds et al., 2014). To achieve this potential, there are important information needs for additional data that must be addressed (Botsford et al., 2009). In part, these needs can be met by adding new dependent variables to strengthen ongoing time series, applying emerging technologies (and reducing costs associated with their application), and promoting uniform methods and inter-calibration for physical and ecological observations among studies. It may also be valuable to profoundly revise of the ways that community structure on CRI is measured, notably by adding new locations that are selected in a pro-active manner to test specific hypotheses. Placement of such locations might be motivated by existing data to identify “hotspots” of larval sources (or sinks) or ecosystem resilience (Kough et al., 2013; Wood et al., 2014). Other approaches might use physical oceanographic modeling (Werner et al., 2007; White et al., 2010; Paris et al., 2013a; Treml et al., 2015) to identify locations where community structure might be particularly sensitive to variation in larval connectivity, and differential reliance on local-recruitment vs. recruits from more distant locations (Kough et al., 2013; Snyder et al., 2014).
We propose eight domains in which time-series observations and analyses of CRI can be expanded to enhance understanding of connectivity and its role in modulating ecosystem resilience. The domains we describe are not intended to provide an exhaustive list of research needs, and largely are restricted to pre-settlement and recruitment periods. Clearly, the ecological patterns thus established are modified though post-settlement processes, and while such events are of great importance (Almany and Webster, 2006; Arnold and Steneck, 2011), they are beyond the scope of the current review. We intend here to summarize research needs with the greatest promise in rapidly advancing an understanding of the roles of connectivity in reef resilience. The eight domains are presented (Figure 1) to underscore research applicable to studying the focal taxon of this review (i.e., the Scleractinia), for which virtually all known larvae are pelagic and dispersive to a greater or lesser extent (Gleason and Hofmann, 2011).
Propagules and Organisms
1. Multi-decadal time series are required to identify biological phenomena on CRI that operate over a wide range of time scales.
Ecological interactions often play out over long time scales, and it can be a challenge for ecologists to distinguish secular trends from noise in a time series. For this reason, long time series can be especially valuable for identifying trends in abundance and species composition of communities and detecting state shifts. Yet despite the clear need for such data, and the establishment of programs with the potential to deliver these data, multi-decadal analyses are rarely published or effectively managed to promote their discovery, access, and use. The acute need for access to data collected over ecologically meaningful time scales justifies devoting adequate resources to their management.
Multi-decadal time series on CRI are not abundant (e.g., Graham et al., 2011), but data from such diverse locations as the Caribbean (Graham et al., 2011), the Great Barrier Reef (Connell, 1997; Connell et al., 1997; Sweatman et al., 2011; Johns et al., 2014; Done et al., 2015; Tanner, 2017), northwestern Australia (Gilmour et al., 2013), Okinawa (van Woesik et al., 2011), Palau (Golbuu et al., 2007), and the Central South Pacific (Adjeroud et al., 2009; Holbrook et al., 2018) have revealed the dynamic interplay between physical and natural disturbances and the potential for subsequent community recovery. Lengthy time series provide the opportunity to capture rare events, as well as effects of repeated disturbances of different types. Sampling over long periods has revealed that after severe disturbance the return of the coral assemblage to its pre-disturbance state may take a decade or longer, depending on the life-history characteristics of the component species and the species interactions that set the pace of re-establishment of the community on the reef. For some CRI, repeated or chronic disturbances over decades have resulted in a different community state (Graham et al., 2011, 2015). The issue of shifting baselines is likely to become more critical as future climate change results in more frequent and intense disturbances to coral reefs.
Many time-series programs on coral reefs have focused primarily on assessing patterns in cover of live coral. A priority in the development of future time-series programs on CRI should be to quantify other aspects of the community, including the extent to which the coral taxonomic assemblage as well as other components of the reef community, including fishes, macroalgae, and non-coral invertebrates, re-assemble to their pre-disturbance state following perturbations (Holbrook et al., 2008; Johns et al., 2014; Done et al., 2015; Han et al., 2016; Tanner, 2017). Knowledge of the physical mechanisms and biotic interactions that influence community re-assembly and determine the similarity of pre- and post- disturbance communities is central to our understanding of community resilience in the face of climate change. It will be important to distinguish between recovery of a reef to a pre-disturbance level of coral cover as opposed to re-assembly to its pre-disturbance taxonomic structure and ecological function (i.e., “recovery without resilience” sensu Berumen and Pratchett, 2006).
2. Major natural disturbances including catastrophic storms, outbreaks of predators (e.g., Crown of Thorns Seastars), bleaching, or disease, create opportunities to quantify connectivity independent of varying historical precedence, as these disturbances typically result in large areas being reduced to an early successional state.
Despite the recurrence of large disturbance events in coral reef locations, resources rarely are mobilized in a systematic fashion to realize the potential to understand their effects on coral reefs. Patterns of initial recruitment one-to-several seasons following disturbances are likely to set population trajectories for subsequent multi-year periods (Bramanti and Edmunds, 2016). Adaptive sampling schemes to track disturbances and deploy recruitment tiles could facilitate understanding of patterns of initial recruitment among sites and regions. A large-scale, adaptive sampling approach would be able to capture such effects, but requires collaboration among researchers at multiple locations, and resource flexibility to facilitate rapid response to disturbance events. Targeting of study locations could also be aided by advanced remote sensing and prognostic models to predict, for example, storm tracks and large-scale bleaching events. Advances in remote sampling of sea surface temperature and estimation of bleaching likelihood associated with degree-heating-weeks provide one example of physical environmental observations at large spatial scales that could be used to target the location and deployment times of recruitment studies based on patterns of disturbance. While studies of the ecological effects of disturbances on coral reefs date to the beginning of modern coral reef science (Connell, 1978), such studies have largely been ad hoc and subject to the vagaries of disturbances occurring in regions near existing research stations and ongoing time-series programs. A more coordinated and international effort involving long-term deployment of recruitment sampling substrata and instrumentation for targeted physical sampling (e.g., of sea water temperature, waves, and currents) to capture changes following disturbance events is likely to greatly enhance understanding of connectivity among CRI.
3 Evaluating the location of larvae in the water column during transport among CRI is of central importance in understanding how they are transported by currents among CRI.
Biological connectivity among CRI is ultimately mediated by the behavior of larvae in the water column (Paris et al., 2007; Butler et al., 2011; Treml et al., 2015), and while analyses of seawater flow (Domain 4 below) and PLD (Domain 5 below) can go a long way to evaluating the potential for transport, realized transport will depend on the temporal structuring of the physical location of larvae in the water column (Paris et al., 2007). Larvae that remain near the benthos are unlikely to be transported great distances, whereas those in wind-driven surface currents potentially can be transported over vast distances (Harii and Kayanne, 2003; Paris et al., 2007). Unfortunately, however, there is still little known about the vertical migration of most larvae of coral reefs species in the seawater column in situ, or how long they remain in each location during ontogeny (but see Paris and Cowen, 2004; Irisson et al., 2010; Butler et al., 2011; Suzuki et al., 2011). In situ experiments with newly released larvae in enclosed acrylic tubes offer potential to advance this data need (Raimondi and Morse, 2000; Tay et al., 2011), but more sophisticated free drifting devices offer the potential for analyses of movement and orientation over lengthy periods (Paris et al., 2008, 2013b; Irisson et al., 2009). The observation that full sibs of coral reef animals settled together following several weeks of larval transport points to the need to better understand the role of larval behavior during the planktonic phase (Bernardi et al., 2012).
Transport and Dispersion
4 High resolution, long-term measurements of physical oceanography of CRI and archipelagoes, particularly for near shore locations, are essential for understanding connectivity among CRI.
Quantifying ocean current patterns over a wide range of space and time scales is essential for understanding transport processes leading to connectivity among CRI (Thompson et al., 2018). Knowledge of these patterns can be gained from observations obtained from a variety of stationary platforms established at island research stations, and moving platforms including deployed drifters and buoys, oceanographic cruises, satellites, and ships of opportunity. Direct measurements can be used to parameterize and initialize ocean circulation models, often designed using nested, or flexible grid spacing approaches that can simulate transport processes on large scales, corresponding to archipelagoes, and small scales, corresponding to the size of corals themselves.
The most geographically extensive observations of ocean current patterns come from altimeter satellites that can be used to estimate currents at the sea surface. The observations are coarse in space (~1/4° resolution), but have been used, for example, to explore possible pathways for larval transport over long distances such as among CRI (Kendall et al., 2013). These observations are available for most CRI including Mo'orea, Okinawa, and St. John. Satellite observations have the major advantage of providing long-duration time series of ocean current patterns. The satellites resolve the “mesoscale” (i.e., 10–100 km), which includes important flow structures such as eddies, boundary currents, and large frontal features. These spatial scales are also accessible by global ocean circulation models, which can be validated by the satellite observations, while providing important insights into the dynamics of large-scale current systems. The ARGO network (~ 4000 floats) supplies additional data on large scales about water properties and large-scale flow patterns in addition to critical validation data for ocean circulation models (Werner et al., 2007).
The next step down the “cascade” of processes informing connectivity among CRI are currents transporting the larvae on a spatial scale of 1–10 km, and the variability associated with these currents (D'Aloia et al., 2015). These systems are largely below the resolution limits of mesoscale circulation models and satellite observations (with the exception of along-track satellite observations), but may be observed and estimated with drifting floats, shipboard surveys, and increasingly with autonomous surface and underwater vehicles (together considered as AUVs), and oceanographic radars for measuring surface currents. Current patterns at these scales are arguably the most difficult to quantify because of the difficulty in sustaining time-series observations of sufficient duration, such as from ships, to understand the dominant processes and long-term trends. Oceanographic radars and AUVs are emerging as critical approaches for obtaining these time series.
At smaller scales (i.e., < 1 km) are current patterns linking the inter-island scales with currents on the fore reef and on the inner-shelf closer to shore. At these scales, turbulent surface and bottom boundary layers often merge and cross-shore circulation decreases due to the island boundaries (Wolanski, 2017). To disperse from spawning areas or to reach settlement habitat, coral larvae and those of other taxa typically must cross the inner-shelf region surrounding CRI. Due to the shallower water depths, the fore reef and inner-shelf are generally more easily accessible for the placement of instrumentation to measure currents and water properties, notwithstanding the potential to lose shallow water instrumentation during large storms and swell events. Ease of instrument placement facilitates the acquisition of long time series such as have been obtained at Mo'orea and Okinawa using oceanographic moorings equipped with current meters, wave sensors, and instruments to measure various water properties. Fine-scale bathymetry data are particularly important for analyzing and modeling currents at these scales (Lindo-Atichati et al., 2016).
At the smallest scales (i.e., 1 mm−1 m) of flows around and through coral colonies, and their interactions with coral larvae and micro-scale habitats where larvae ultimately settle or where they initially disperse (Monismith, 2007; Hench and Rosman, 2013). Quantifying these flows requires instruments with high spatial resolution and rapid temporal sampling such as microstructure sensors, acoustic Doppler velocimeters, high-resolution current profilers, and specialized high-resolution water property sensors.
5 Quantification of dispersal patterns of multiple taxa is needed to understand connectivity.
Although the relationship between dispersal distance, pelagic larval duration (PLD), and competency period is complicated by issues of scale and experimental approach (Bradbury et al., 2008; Weersing and Toonen, 2009; Selkoe and Toonen, 2011; Dawson et al., 2014), marine invertebrates, including corals, have varying capacity for dispersal, often associated with PLD and reproductive mode (Connolly and Baird, 2010). For most broadcast-spawning corals, like Acropora spp., PLD or time at first competency is ~5–8 d after spawning, based upon experimental data (Nishikawa et al., 2003; Nishikawa and Sakai, 2005) and field observations (Suzuki et al., 2011). Peaks of larval settlement occur on the seventh day following spawning in Acropora tenuis, and on the first day post-spawning in the brooding coral, Stylophora pistillata. In contrast, the broadcast-spawner Platygyra daedalea, has a short PLD with a settlement peak ~ 2.50–2.75 days following fertilization (Miller and Mundy, 2003). In fine-scale geographic analysis, P. daedalea showed higher genetic differentiation than Goniastrea favulus, which spawns sticky, negatively buoyant eggs (Miller and Ayre, 2008).
In addition to differences among taxa in dispersal capabilities, life history theory predicts that selection should favor dispersal polymorphisms in habitats that exhibit high temporal or spatial variability or for which competition for available space is high, such as most coral reefs (McPeek and Holt, 1992; Dingle, 1996). Such dispersal polymorphisms, in which some individuals ignore suitable habitat to disperse long distances whereas others of the same species or even among progeny of single individuals remain close to their natal site, are well-documented in terrestrial systems, but have rarely been investigated in marine taxa (Dingle, 1996; Toonen and Pawlik, 2001). A handful of examples exist among marine species (e.g., Gibson and Chia, 1989; Raimondi and Keough, 1990; Krug, 2001; Toonen and Pawlik, 2001; Marshall and Keough, 2003), but to date only a single study comparing short and long-distance dispersal within a single species has been published for corals (Torda et al., 2013).
Nevertheless, the few larvae with the greatest dispersal are the ones that maintain connectivity among reefs, and population connectivity of even brooding corals can be maintained by relatively low numbers of migrants (van Oppen et al., 2008). Even in brooding corals like some Pocillopora damicornis sensu lato, PLD is ~100 days based on experimental analyses and competency model predictions (Richmond, 1987). Thus, long PLD is not exclusive to broadcast-spawning corals (Graham et al., 2008), and PLD alone is a poor predictor of mean dispersal ability (Bradbury et al., 2008; Weersing and Toonen, 2009; Selkoe and Toonen, 2011). The success of larval dispersal and recruitment in augmenting coral populations may be influenced by the fecundity of the species and by habitat characteristics (Hughes et al., 2000). Therefore, opportunities for larval dispersal and recruitment may not only vary by species but also within species (e.g., Gibson and Chia, 1989; Krug, 2001) and even among individual families (Toonen and Pawlik, 2001), especially after taking into account differences among CRI in habitat size and distance to nearest neighboring CRI. These observations underscore the importance of more synoptic analyses of dispersal potential and variability in dispersal behaviors for a wide variation of coral reef species.
Settlement and Recruitment
6 Time-series analyses of population structure of a wide diversity of taxa and functional groups of benthic organisms are needed to understand community-wide patterns of connectivity among CRI.
Time-series programs on CRI vary widely in time of initiation, spatial scope, taxa that are quantified, and method and frequency of sampling. Scleractinians are the foundational taxon, and they are often the major focus of study. Percent cover of live coral is the primary state variable reported, but to assess processes of disturbance and recovery, temporal patterns of population structure and colony sizes of key species, as well as overall taxonomic composition of corals, are needed. These data enable observations of recruitment to be better linked to colony survival and growth, both of which are key processes leading to the re-establishment of the coral community following disturbances. Additionally, tropical reefs support a high diversity of non-coral taxa that represent a variety of functional groups and trophic levels, and these components of the community should be incorporated into time-series analyses. Time-series programs that include measurement of population structure of fish, mobile and sessile invertebrates, and algae, matched with population genetic information, could enable comparisons of connectivity among taxa of widely differing life history strategies, trophic status, behavior, and longevity (Selkoe et al., 2016). Such knowledge will be critical for understanding the potential and realized patterns of re-assembly of a CRI community following disturbance, as well as the degree to which the community structure will change in light of future environmental and climate conditions. For example, herbivores play a critical role in suppressing the establishment of fleshy algae following landscape-scale disturbances that kill corals, thus maintaining the reef in a condition suitable for settlement and survival of corals (Adam et al., 2011, 2014; Han et al., 2016; Holbrook et al., 2016). In these cases, connectivity patterns of corals and herbivorous species are both important, and understanding them will enable more informed predictions about the potential for reefs to recover from disturbance.
7 Most measures of recruitment around CRI are inconsistent and restricted temporally to sampling events driven by logistics rather than ecological questions.
Recruitment is a critical process in the sustaining the structure and dynamics of reef communities, particularly for CRI. For example, recovery of coral populations following major disturbances depends on the level of connectivity with adjacent fecund populations, and on the growth and propagation of newly settling larval recruits (e.g., Hughes and Connell, 1999; Arnold and Steneck, 2011; Doropoulos et al., 2015). However, given the long life span of most corals [i.e., ~ 4 y for a recruit of < 1-mm diameter to become a mature adult colony of >5 cm (Penin et al., 2010)], understanding the implication of recruitment in coral recovery requires long-term analysis on population dynamics and demography (Adjeroud et al., 2017). Frequent sampling is necessary to resolve rapidly occurring events such as episodic recruitment, sudden-onset, lethal diseases, or transient population perturbations (Connell et al., 1997; Graham et al., 2011).
Due to the logistic difficulty of quantifying recruitment on time scales allowing cycles of community replenishment to be detected, few studies have examined the interplay among recruitment, dynamics of adult populations, and disturbances, on a relevant time-scale (> 10 y; but see Connell et al., 1997). Moreover, it is difficult to taxonomically resolve recruits, and only limited progress has been made with genetic tools for this purpose (e.g., Shearer and Coffroth, 2006; Suzuki et al., 2012). All of these limitations explain why the majority of surveys of coral recruitment have documented variability in recruitment in a restricted spatio-temporal window, and why they have not achieved their potential to link connectivity, recruitment, and critical processes such as recovery following disturbances (Graham et al., 2011; Adjeroud et al., 2017). Ongoing development of genetic tools to identify the geographic origin of coral larvae and recruits, such as DNA parentage analysis, represent an interesting way to evaluate better the relative contribution of local- vs. distant-recruitment, and to identify potential “sources” and “sinks” among networks of CRI (Adjeroud et al., 2017). In addition, subdivision of sampling intervals could be effective for estimating larval connectivity among CRI. For example, replacing the settlement tiles at 2 or 3 days intervals after a spawning event may reveal settlement peaks of local- and distantly-sourced recruits (Suzuki et al., 2011).
8. Distinguishing local- from distant-recruits for multiple taxa is central to understanding the role of connectivity around CRI, and essential for understanding connectivity of virtually all taxa on coral reefs.
Genetic studies have become an essential component for understanding recruitment and connectivity in marine systems across both ecological and evolutionary timescales (e.g., Almany et al., 2017). These studies are readily integrated into research on the relationship between connectivity among CRI and community resilience provided that sampling design is consistent with the space and time scale required by research questions relying on genetic data. For example, to determine the origin of recruits, the most reliable approach is parentage analysis, which has potential to assign recruits to specific parents at the natal site (Harrison et al., 2013; D'Aloia et al., 2015; Selkoe et al., 2016; but also see Christie et al., 2017), but could also miss some long distance connections outside of the sampling area. In contrast, to determine the relative importance of multiple environmental drivers of population structure, a probabilistic approach (sensu Paris et al., 2013b; Selkoe et al., 2016) may be sufficient to identify the origin of recruits, or to differentiate individuals according to whether they originated from local-recruitment or from more distant locations (Box ).
In each genetic approach, the required sampling regimes will differ in order to address trade-offs in methodologies. For example, in parentage analysis, statistical power is increased by maximizing the proportion of the regional population sampled, whereas for probabilistic assignment of individuals to a natal site, power is increased by maximizing the number of natal sites sampled (Christie et al., 2017). Additionally, to untangle the effects of distant recruitment from the effects of post-settlement success, a different experimental design is required (e.g., Toonen and Grosberg, 2011). While distant sources of larvae may have a low proportional influence on genetic population structure on a healthy reef where settlement space is limited and competition with the preexisting populations is strong, distantly-sourced larvae may drive or modulate recovery following disturbance (Harris et al., 1984; Jennings, 2000; Armsworth, 2002; Baskett and Salomon, 2010). Alternatively, rare survivors of disturbance that are easily missed in ecological surveys may serve as sources of recruits that can drive local community recovery (Edmunds et al., 2016; Tsounis and Edmunds, 2016; Cros et al., 2017). Ultimately distinguishing among these possibilities would require comparing genetic data from adult and recruits to examine multi-generational patterns of structure and/or kinship (Toonen and Grosberg, 2011; Iacchei et al., 2013). Conducting such an analysis on recruitment pulses following disturbance on CRI (Bramanti and Edmunds, 2016), for example, would be particularly relevant.
Conclusions
CRI are abundant and unique reef formations throughout most tropical seas, and characterized by diverse and productive ecosystems on their shores. Over the last few decades, however, multiple assaults of natural and anthropogenic origin have driven coral cover downward on most CRI, and imposed multiple cycles of community destruction and recovery. Some CRI are notable in their long history of ecological and oceanographic research, but much of this effort has been insular and focused on single CRI selected for study based on happenstance or peculiar historical presence. This emphasis has overlooked the potential of CRI to serve as natural laboratories to investigate connectivity as an ecological process shaping community dynamics, and to answer critical questions addressing mechanisms of coral reef resilience (Table 1). Not only has this restricted intellectual advancement in the field of coral reef biology, but also it has deprived resource managers of access to the full spectrum of tools necessary to advance reef conservation. Achieving the potential of CRI to meet this need will require more than integrating the information currently available. As we describe above, there are clear needs for new data (Domains 1–8, above) and more coherence in spatio-temporal scales of sampling of biological and physical variables. Critically, there is much to be gained from the pro-active selection of new CRI for use in time-series analysis designed to address specific hypotheses.
Author Contributions
All authors listed have made a substantial, direct and intellectual contribution to the work, and approved it for publication.
Funding
This project was supported by the Okinawa Institute of Science and Technology Graduate University as a workshop held 16-21 March 2016.
Conflict of Interest Statement
The authors declare that the research was conducted in the absence of any commercial or financial relationships that could be construed as a potential conflict of interest.
Acknowledgments
This paper originated in a workshop funded and hosted by the Okinawa Institute of Science and Technology Graduate University (OIST), and organized by SM, KS, and PE. The ideas expressed herein were developed during the workshop that took place from 16 to 20 March 2016, and later developed through discussions and input from all authors. Much of the empirical research from Mo'orea and St. John was funded by the US National Science Foundation (most recently DEB 13-50146 and OCE 16-37396).
References
Adam, T. C., Brooks, A. J., Holbrook, S. J., Schmitt, R. J., Washburn, L., and Bernardi, G. (2014). How will coral reef fish communities respond to climate-driven disturbances? Insight from landscape-scale perturbations. Oecologia 176, 285–296. doi: 10.1007/s00442-014-3011-x
Adam, T. C., Schmitt, R. J., Holbrook, S. J., Brooks, A. J., Edmunds, P. J., Carpenter, R. C., et al. (2011). Herbivory, connectivity, and ecosystem resilience: response of a coral reef to a large-scale perturbation. PLoS ONE 6:e23717. doi: 10.1371/journal.pone.0023717
Adjeroud, M., Kayal, M., and Penin, L. (2017). “Importance of recruitment processes in the dynamics and resilience of coral reef assemblages,” in Marine Animal Forests: The Ecology of Benthic Biodiversity Hotspots, eds S. Rossi, L. Bramanti, A. Gori and C. Orejas (Cham: Springer), 549–569.
Adjeroud, M., Michonneau, F., Edmunds, P. J., Chancerelle, Y., Lison de Loma, T., Penin, L., et al. (2009). Recurrent disturbances, recovery trajectories, and resilience of coral assemblages on a South Central Pacific reef. Coral Reefs 28, 775–780. doi: 10.1007/s00338-009-0515-7
Albright, R., and Langdon, C. (2011). Ocean acidification impacts multiple early life history processes of the Caribbean coral Porites astreoides. Global Change Biol. 17, 2478–2487. doi: 10.1111/j.1365-2486.2011.02404.x
Albright, R., Mason, B., and Langdon, C. (2008). Effect of aragonite saturation state on settlement and post-settlement growth of Porites astreoides larvae. Coral Reefs 27, 485–490. doi: 10.1007/s00338-008-0392-5
Almany, G. R., Planes, S., Thorrold, S. R., Berumen, M. L., Bode, M., Saenz-Agudelo, P., et al. (2017). Larval fish dispersal in a coral-reef seascape. Nat. Ecol. Evol. 1:0148. doi: 10.1038/s41559-017-0148
Almany, G. R., and Webster, M. S. (2006). The predation gauntlet: early post-settlement mortality in reef fishes. Coral Reefs 25, 19–22. doi: 10.1007/s00338-005-0044-y
Alvarez-Filip, L., Dulvy, N. K., Gill, J. A., Côté, I. M., and Watkinson, A. R. (2009). Flattening of Caribbean coral reefs: region-wide declines in architectural complexity. Proc. Biol. Sci. 276, 3019–3025. doi: 10.1098/rspb.2009.0339
Armsworth, P. R. (2002). Recruitment limitation, population regulation, and larval connectivity in reef fish metapopulations. Ecology 83, 1092–1104. doi: 10.1890/0012-9658(2002)083[1092:RLPRAL]2.0.CO;2
Arnold, S. N., and Steneck, R. S. (2011). Settling into an increasingly hostile world: the rapidly closing “recruitment window” for corals. PLoS ONE 6:e28681. doi: 10.1371/journal.pone.0028681
Arnold, S. N., Steneck, R. S., and Mumby, P. J. (2010). Running the gauntlet: inhibitory effects of algal turfs on the processes of coral recruitment. Mar. Ecol. Prog. Ser. 414, 91–105. doi: 10.3354/meps08724
Aronson, R. B., and Precht, W. F. (2001). White-band disease and the changing face of Caribbean coral reefs. Hydrobiologia 460, 25–38. doi: 10.1023/A:1013103928980
Baskett, M. L., and Salomon, A. K. (2010). Recruitment facilitation can drive alternative states on temperate reefs. Ecology 91, 1763–1773. doi: 10.1890/09-0515.1
Baums, I. B., Paris, C. B., and Chérubin, L. M. (2006). A bio-oceanographic filter to larval dispersal in a reef-building coral. Limnol. Oceanogr. 51, 1969–1981. doi: 10.4319/lo.2006.51.5.1969
Bellwood, D. R., Hughes, T. P., Folke, C., and Nyström, M. (2004). Confronting the coral reef crisis. Nature 429, 827–833. doi: 10.1038/nature02691
Bernardi, G., Beldade, R., Holbrook, S. J., and Schmitt, R. J. (2012). Full-sibs in cohorts of newly settled coral reef fishes. PLoS ONE 7:e44953. doi: 10.1371/journal.pone.0044953
Bernardo, L. P. C., Nadaoka, K., Nakamura, T., and Watanabe, A. (2017). Island-enhanced cooling mechanism in typhoon events revealed by field observations and numerical simulations for a coral reef area, Sekisei Lagoon, Japan. Ocean Dyn. 67, 1369–1384. doi: 10.1007/s10236-017-1096-6
Berumen, M. L., and Pratchett, M. S. (2006). Recovery without resilience: persistent disturbance and long-ter, shifts in the structure of fish and coral communities at Tiahura Reef, Moorea. Coral Reefs 25, 647–653. doi: 10.1007/s00338-006-0145-2
Botsford, L. W., White, J. W., Coffroth, M. A., Paris, C. B., Planes, S., Shearer, T. L., et al. (2009). Connectivity and resilience of coral reef metapopulations in marine protected areas: matching empirical efforts to predictive needs. Coral Reefs 28, 327–337. doi: 10.1007/s00338-009-0466-z
Bradbury, I. R., Laurel, B., Snelgrove, P. V. R., Bentzen, P., and Campana, S. E. (2008). Global patterns in marine dispersal estimates: the influence of geography, taxonomic category and life history. Proc. Biol. Sci. 275, 1803–1809. doi: 10.1098/rspb.2008.0216
Bramanti, L., and Edmunds, P. J. (2016). Density-associated recruitment mediates coral population dynamics on a coral reef. Coral Reefs 35, 543–553. doi: 10.1007/s00338-016-1413-4
Brown, B. E., and Cossins, A. R. (2011). “The potential for temperature acclimatisation of reef corals in the face of climate change,” in Coral Reefs: An Ecosystem in Transition, eds Z. Dubinsky and N. Stambler (Dordrecht: Springer), 421–433. doi: 10.1007/978-94-007-0114-4_24
Bruno, J. F., and Selig, E. R. (2007). Regional decline of coral cover in the Indo-Pacific: timing, extent, and subregional comparisons. PLoS ONE 2:e711. doi: 10.1371/journal.pone.0000711
Burgess, S. C., Nickols, K. J., Griesemer, C. D., Barnett, L. A., Dedrick, A. G., Satterthwaite, E. V., et al. (2014). Beyond connectivity: how empirical methods can quantify population persistence to improve marine protected-area design. Ecol. Appl. 24, 257–270. doi: 10.1890/13-0710.1
Burman, S. G., Aronson, R. B., and van Woesik, R. (2012). Biotic homogenization of coral assemblages along the Florida reef tract. Mar. Ecol. Prog. Ser. 467, 89–96. doi: 10.3354/meps09950
Butler, M. J. IV., Paris, C. B., Goldstein, J. S., Matsuda, H., and Cowen, R. K. (2011). Behavior constrains the dispersal of long-lived spiny lobster larvae. Mar. Ecol. Prog. Ser. 422, 223–237. doi: 10.3354/meps08878
Cai, W., Borlace, S., Lengaigne, M., van Rensch, P., Collins, M., Vecchi, G., et al. (2014). Increasing frequency of extreme El Niño events due to greenhouse warming. Nat. Clim. Change 4, 111–116. doi: 10.1038/NCLIMATE2100
Christie, M. R., Meirmans, P. G., Gaggiotti, O. E., Toonen, R. J., and White, C. (2017). Disentangling the relative merits and disadvantages of parentage analysis and assignment tests for inferring population connectivity. ICES J. Mar. Sci. 74, 1749–1762. doi: 10.1093/icesjms/fsx044
Coles, S. L., and Jokiel, P. L. (1977). Effects of temperature on photosynthesis and respiration in hermatypic corals. Mar. Biol. 43, 209–216. doi: 10.1007/BF00402313
Connell, J. H. (1978). Diversity in tropical rain forests and coral reefs. Science 199, 1302–1310. doi: 10.1126/science.199.4335.1302
Connell, J. H. (1997). Disturbance and recovery of coral assemblages. Coral Reefs 16, S101–S113. doi: 10.1007/s003380050246
Connell, J. H., Hughes, T. P., and Wallace, C. C. (1997). A 30-year study of coral abundance, recruitment, and disturbance at several scales in space and time. Ecol. Monogr. 67, 461–488. doi: 10.1890/0012-9615(1997)067[0461:AYSOCA]2.0.CO;2
Connolly, S. R., and Baird, A. H. (2010). Estimating dispersal potential for marine larvae: dynamic models applied to scleractinian corals. Ecology 91, 3572–3583. doi: 10.1890/10-0143.1
Cowen, R. K., Lwiza, K. M. M., Sponaugle, S., Paris, C. B., and Olson, D. B. (2000). Connectivity of marine populations: open or closed? Science 287, 857–859. doi: 10.1126/science.287.5454.857
Cowen, R. K., Paris, C. B., Olson, D. B., and Fortuna, J. L. (2003). The role of long distance dispersal versus local retention in replenishing marine populations. Gulf Caribb. Res. 14, 129–137. doi: 10.18785/gcr.1402.10
Cowen, R. K., Paris, C. B., and Srinivasan, A. (2006). Scaling of connectivity in marine populations. Science 311, 522–527. doi: 10.1126/science.1122039
Cowen, R. K., and Sponaugle, S. (2009). Larval dispersal and marine population connectivity. Annu. Rev. Mar. Sci. 1, 443–466. doi: 10.1146/annurev.marine.010908.163757
Cros, A., Toonen, R. J., Donahue, M. J., and Karl, S. A. (2017). Connecting Palau's marine protected areas: a population genetic approach to conservation. Coral Reefs 36, 735–748. doi: 10.1007/s00338-017-1565-x
D'Aloia, C. C., Bogdanowicz, S. M., Francis, R. K., Majoris, J. E., Harrison, R. G., and Buston, P. M. (2015). Patterns, causes, and consequences of marine larval dispersal. Proc. Natl. Acad. Sci. U.S.A. 112, 13940–13945. doi: 10.1073/pnas.1513754112
Dasgupta, S., Laplante, B., Meisner, C., Wheeler, D., and Yan, J. (2009). The impact of sea level rise on developing countries: a comparative analysis. Clim. Change 93, 379–388. doi: 10.1007/s10584-008-9499-5
Dawson, M. N., Hays, C. G., Grosberg, R. K., and Raimondi, P. T. (2014). Dispersal potential and population genetic structure in the marine intertidal of the eastern North Pacific. Ecol. Monogr. 84, 435–456. doi: 10.1890/13-0871.1
Dingle, H. (1996). Migration: The Biology of Life on the Move. New York, NY: Oxford University Press.
Doherty, P. J., Planes, S., and Mather, P. (1995). Gene flow and larval duration in seven species of fish from the Great Barrier Reef. Ecology 76, 2373–2391. doi: 10.2307/2265814
Done, T., Gilmour, J., and Fisher, R. (2015). Distance decay among coral assemblages during a cycle of disturbance and recovery. Coral Reefs 34, 727–738. doi: 10.1007/s00338-015-1302-2
Doney, S. C., Fabry, V. J., Feely, R. A., and Kleypas, J. A. (2009). Ocean acidification: the other CO2 problem. Annu. Rev. Mar. Sci. 1, 169–192. doi: 10.1146/annurev.marine.010908.163834
Doney, S. C., Ruckelshaus, M., Duffy, J. E., Barry, J. P., Chan, F., English, C. A., et al. (2012). Climate change impacts on marine ecosystems. Annu. Rev. Mar. Sci. 4, 11–37. doi: 10.1146/annurev-marine-041911-111611
Doropoulos, C., Ward, S., Roff, G., González-Rivero, M., and Mumby, P. J. (2015). Linking demographic processes of juvenile corals to benthic recovery trajectories in two common reef habitats. PLoS ONE 10:e0128535. doi: 10.1371/journal.pone.0128535
Edmunds, P. J., Steneck, R., Albright, R., Carpenter, R. C., Chui, A. P. Y., Fan, T. Y., et al. (2015). Geographic variation in long-term trajectories of change in coral recruitment: a global-to-local perspective. J. Freshw. Mar. Biol. 66, 1–14. doi: 10.1071/MF14139
Edmunds, P. J. (2015). A quarter-century demographic analysis of the Caribbean coral, Orbicella annularis, and projections of population size over the next century. Limnol. Oceanogr. 60, 840–855. doi: 10.1002/lno.10075
Edmunds, P. J., Adjeroud, M., Baskett, M. L., Baums, I. B., Budd, A. F., Carpenter, R. C., et al. (2014). Persistence and change in community composition of reef corals through present, past, and future climates. PLoS ONE 9:e107525. doi: 10.1371/journal.pone.0107525
Edmunds, P. J., Leichter, J. J., Johnson, E. C., Tong, E. J., and Toonen, R. J. (2016). Ecological and genetic variation in reef-building corals on four Society Islands. Limnol. Oceanogr. 61, 543–557. doi: 10.1002/lno.10231
Emanuel, K. (2005). Increasing destructiveness of tropical cyclones over the past 30 years. Nature 436, 686–688. doi: 10.1038/nature03906
Fabricius, K. E., Noonan, S. H. C., Abrego, D., Harrington, L., and De'ath, G. (2017). Low recruitment due to altered settlement substrata as primary constraint for coral communities under ocean acidification. Proc. Biol. Sci. 284:20171536. doi: 10.1098/rspb.2017.1536
Figueiredo, J., Baird, A. H., and Connolly, S. R. (2013). Synthesizing larval competence dynamics and reef-scale retention reveals a high potential for self-recruitment in corals. Ecology 94, 650–659. doi: 10.1890/12-0767.1
Fitt, W. K., Brown, B. E., Warner, M. E., and Dunne, R. P. (2001). Coral bleaching: interpretation of thermal tolerance limits and thermal thresholds in tropical corals. Coral Reefs 20, 51–65. doi: 10.1007/s003380100146
Foster, N. L., Paris, C. B., Kool, J. T., Baums, I. B., Stevens, J. R., Sanchez, J. A., et al. (2012). Connectivity of Caribbean coral populations: complementary insights from empirical and modelled gene flow. Mol. Ecol. 21, 1143–1157. doi: 10.1111/j.1365-294X.2012.05455.x
Gibson, G. D., and Chia, F. -S. (1989). Developmental variability (pelagic and benthic) in Haminoea callidegenita (Opisthobranchia: Cephalaspidea) is influenced by egg mass jelly. Biol. Bull. 176, 103–110. doi: 10.2307/1541577
Gilmour, J. P., Smith, L. D., Heyward, A. J., Baird, A. H., and Pratchett, M. S. (2013). Recovery of an isolated coral reef system following severe disturbance. Science 340, 69–71. doi: 10.1126/science.1232310
Gleason, D. F., and Hofmann, D. K. (2011). Coral larvae: from gametes to recruits. J. Exp. Mar Biol. Ecol. 408, 42–57. doi: 10.1016/j.jembe.2011.07.025
Golbuu, Y., Victor, S., Penland, L., Idip, J.r,., Emaurois, C., Okaji, K., et al. (2007). Palau's coral reefs show differential habitat recovery following the 1998-bleaching event. Coral Reefs 26, 319–332. doi: 10.1007/s00338-007-0200-7
Goto, K., Miyagi, K., Kawana, T., Takahashi, J., and Imamura, F. (2011). Emplacement and movement of boulders by known storm waves — field evidence from the Okinawa Islands, Japan. Mar. Geol. 283, 66–78. doi: 10.1016/j.margeo.2010.09.007
Graham, E. M., Baird, A. H., and Connolly, S. R. (2008). Survival dynamics of scleractinian coral larvae and implications for dispersal. Coral Reefs 27, 529–539. doi: 10.1007/s00338-008-0361-z
Graham, N. A., Jennings, S., MacNeil, M. A., Mouillot, D., and Wilson, S. K. (2015). Predicting climate-driven regime shifts versus rebound potential in coral reefs. Nature 518, 94–97. doi: 10.1038/nature14140
Graham, N. A., Nash, K. L., and Kool, J. T. (2011). Coral reef recovery dynamics in a changing world. Coral Reefs 30, 283–294. doi: 10.1007/s00338-010-0717-z
Green, D. H., Edmunds, P. J., and Carpenter, R. C. (2008). Increasing relative abundance of Porites astreoides on Caribbean reefs mediated by an overall decline in coral cover. Mar. Ecol. Prog. Ser. 359, 1–10. doi: 10.3354/meps07454
Grigg, R. W. (1983). Community structure, succession and development of coral reefs in Hawaii. Mar. Ecol. Prog. Ser. 11, 1–14.
Gunderson, L. H. (2000). Ecological resilience—in theory and application. Annu. Rev. Ecol. Syst. 31, 425–439. doi: 10.1146/annurev.ecolsys.31.1.425
Han, X., Adam, T. C., Schmitt, R. J., Brooks, A. J., and Holbrook, S. J. (2016). Response of herbivore functional groups to sequential perturbations in Moorea, French Polynesia. Coral Reefs 35, 999–1009. doi: 10.1007/s00338-016-1423-2
Hanski, I. (1999). Habitat connectivity, habitat continuity, and metapopulations in dynamic landscapes. Oikos 87, 209–219. doi: 10.2307/3546736
Harii, S., and Kayanne, H. (2003). Larval dispersal, recruitment, and adult distribution of the brooding stony octocoral Heliopora coerulea on Ishigaki Island, southwest Japan. Coral Reefs 22, 188–196. doi: 10.1007/s00338-003-0302-9
Harmelin-Vivien, M. L. (1994). The effects of storms and cyclones on coral reefs: a review. J. Coastal Res. 12, 211–231.
Harris, L. G., Ebeling, A. W., Laur, D. R., and Rowley, R. J. (1984). Community recovery after storm damage: a case of facilitation in primary succession. Science 224, 1336–1338. doi: 10.1126/science.224.4655.1336
Harrison, H. B., Saenz-Agudelo, P., Planes, S., Jones, G. P., and Berumen, M. L. (2013). Relative accuracy of three common methods of parentage analysis in natural populations. Mol. Ecol. 22, 1158–1170. doi: 10.1111/mec.12138
Hastings, A., and Botsford, L. W. (2006). Persistence of spatial populations depends on returning home. Proc. Natl. Acad. Sci. U.S.A. 103, 6067–6072. doi: 10.1073/pnas.0506651103
Hench, J. L., and Rosman, J. H. (2013). Observations of spatial flow patterns at the coral colony scale on a shallow reef flat. J. Geophys. Res. 118, 1142–1156. doi: 10.1002/jgrc.20105
Hoegh-Guldberg, O. (1999). Climate change, coral bleaching and the future of the world's coral reefs. Mar. Freshw. Res. 50, 839–866. doi: 10.1071/MF99078
Holbrook, S. J., Adam, T. C., Edmunds, P. J., Schmitt, R. J., Carpenter, R. C., Brooks, A. J., et al. (2018). Recruitment drives spatial variation in recovery rates of resilient coral reefs. Sci. Rep. 8:7338. doi: 10.1038/s41598-018-25414-8
Holbrook, S. J., Schmitt, R. J., Adam, T. C., and Brooks, A. J. (2016). Coral reef resilience, tipping points and the strength of herbivory. Sci. Rep. 6:35817. doi: 10.1038/srep35817
Holbrook, S. J., Schmitt, R. J., and Brooks, A. J. (2008). Resistance and resilience of a coral reef fish community to changes in coral cover. Mar. Ecol. Prog. Ser. 371, 263–271. doi: 10.3354/meps07690
Holstein, D. M., Paris, C. B., and Mumby, P. J. (2014). Consistency and inconsistency in multispecies population network dynamics of coral reef ecosystems. Mar. Ecol. Prog. Ser. 499, 1–18. doi: 10.3354/meps10647
Hughes, T. P., Baird, A. H., Dinsdale, E. A., Moltschaniwskyj, N. A., Pratchett, M. S., Tanner, J. E., et al. (2000). Supply-side ecology works both ways: the link between benthic adults, fecundity, and larval recruits. Ecology 81, 2241–2249. doi: 10.2307/177111
Hughes, T. P., Barnes, M. L., Bellwood, D. R., Cinner, J. E., Cumming, G. S., Jackson, J. B. C., et al. (2017). Coral reefs in the Anthropocene. Nature 546, 82–90. doi: 10.1038/nature22901
Hughes, T. P., and Connell, J. H. (1999). Multiple stressors on coral reefs: a long-term perspective. Limnol. Oceanogr. 44, 932–940. doi: 10.4319/lo.1999.44.3_part_2.0932
Hughes, T. P., Rodrigues, M. J., Bellwood, D. R., Ceccarelli, D., Hoegh-Guldberg, O., McCook, L., et al. (2007). Phase shifts, herbivory, and the resilience of coral reefs to climate change. Curr. Biol. 17, 360–365. doi: 10.1016/j.cub.2006.12.049
Iacchei, M., Ben-Horin, T., Selkoe, K. A., Bird, C. E., García-Rodríguez, F. J., and Toonen, R. J. (2013). Combined analyses of kinship and FST suggest potential drivers of chaotic genetic patchiness in high gene-flow populations. Mol. Ecol. 22, 3476–3494. doi: 10.1111/mec.12341
Irisson, J.-O., Guigand, C., and Paris, C. B. (2009). Detection and quantification of marine larvae orientation in the pelagic environment. Limnol. Oceanogr. Methods 7, 664–672. doi: 10.4319/lom.2009.7.664
Irisson, J.-O., Paris, C. B., Guigand, C., and Planes, S. (2010). Vertical distribution and ontogenetic “migration” in coral reef fish larvae. Limnol. Oceanogr. 55, 909–919. doi: 10.4319/lo.2010.55.2.0909
Jackson, J., Donovan, M., Cramer, K., and Lam, V. (2014). Status and Trends of Caribbean Coral Reefs: 1970–2012. Gland: Global Coral Reef Monitoring Network, IUCN.
Jennings, S. (2000). Patterns and prediction of population recovery in marine reserves. Rev. Fish Biol. Fish. 10, 209–231. doi: 10.1023/A:1016619102955
Johns, K. A., Osborne, K. O., and Logan, M. (2014). Contrasting rates of coral recovery and reassembly in coral communities on the Great Barrier Reef. Coral Reefs 33, 553–563. doi: 10.1007/s00338-014-1148-z
Jones, C. G., Lawton, J. H., and Shachak, M. (1994). Organisms as ecosystem engineers. Oikos 69, 373–386. doi: 10.2307/3545850
Kendall, M. S., Poti, M., Wynne, T. T., Kinlan, B. P., and Bauer, L. B. (2013). Consequences of the life history traits of pelagic larvae on interisland connectivity during a changing climate. Mar. Ecol. Prog. Ser. 489, 43–59. doi: 10.3354/meps10432
Khan, T. M. A., Quadir, D. A., Murty, T. S., Kabir, A., Aktar, F., and Sarker, M. A. (2002). Relative sea level changes in Maldives and vulnerability of land due to abnormal coastal inundation. Mar. Geod. 25, 133–143. doi: 10.1080/014904102753516787
Kough, A. S., and Paris, C. B. (2015). The influence of spawning periodicity on population connectivity. Coral Reefs 34, 753–757. doi: 10.1007/s00338-015-1311-1
Kough, A. S., Paris, C. B., and Butler, M. J. IV. (2013). Larval connectivity and the international management of fisheries. PLoS ONE 8:e64970. doi: 10.1371/journal.pone.0064970
Krug, P. J. (2001). Bet-hedging dispersal strategy of a specialist marine herbivore: a settlement dimorphism among sibling larvae of Alderia modesta. Mar. Ecol. Prog. Ser. 213, 177–192. doi: 10.3354/meps213177
Limouzy-Paris, C. B., Graber, H. C., Jones, D. L., Röpke, A. W., and Richards, W. J. (1997). Translocation of larval coral reef fishes via sub-mesoscale spin-off eddies from the Florida Current. Bull. Mar. Sci. 60, 966–983.
Lindo-Atichati, D., Curcic, M., Paris, C. B., and Buston, P. M. (2016). Description of surface transport in the region of the Belizean Barrier Reef based on observations and alternative high-resolution models. Ocean Model. 106, 74–89. doi: 10.1016/j.ocemod.2016.09.010
Madin, J. S., and Connolly, S. R. (2006). Ecological consequences of major hydrodynamic disturbances on coral reefs. Nature 444, 477–480. doi: 10.1038/nature05328
Mann, M. E., and Emanuel, K. A. (2006). Atlantic hurricane trends linked to climate change. Earth Space Sci. News 87, 233–241. doi: 10.1029/2006EO240001
Marshall, D. J., and Keough, M. J. (2003). Variation in the dispersal potential of non-feeding invertebrate larvae: the desperate larva hypothesis and larval size. Mar. Ecol. Prog. Ser. 255, 145–153. doi: 10.3354/meps255145
Massel, S. R., and Done, T. J. (1993). Effects of cyclone waves on massive coral assemblages on the Great Barrier Reef: meteorology, hydrodynamics and demography. Coral Reefs 12, 153–166. doi: 10.1007/BF00334475
McPeek, M. A., and Holt, R. D. (1992). The evolution of dispersal in spatially and temporally varying environments. Am. Nat. 140, 1010–1027. doi: 10.1086/285453
Mendes, J. M., and Woodley, J. D. (2002). Timing of reproduction in Montastraea annularis: relationship to environmental variables. Mar. Ecol. Prog. Ser. 227, 241–251. doi: 10.3354/meps227241
Miller, K., and Mundy, C. (2003). Rapid settlement in broadcast spawning corals: implications for larval dispersal. Coral Reefs 22, 99–106. doi: 10.1007/s00338-003-0290-9
Miller, K. J., and Ayre, D. J. (2008). Population structure is not a simple function of reproductive mode and larval type: insights from tropical corals. J. Anim. Ecol. 77, 713–724. doi: 10.1111/j.1365-2656.2008.01387.x
Monismith, S. G. (2007). Hydrodynamics of coral reefs. Annu. Rev. Fluid Mech. 39, 37–55. doi: 10.1146/annurev.fluid.38.050304.092125
Monismith, S. G., Herdman, L. M. M., Ahmerkamp, S., and Hench, J. L. (2013). Wave transformation and wave-driven flow across a steep coral reef. J. Phys. Oceanogr. 43, 1356–1379. doi: 10.1175/JPO-D-12-0164.1
Mumby, P. J., Hastings, A., and Edwards, H. J. (2007). Thresholds and the resilience of Caribbean coral reefs. Nature 450, 98–101. doi: 10.1038/nature06252
Munday, P. L., Leis, J. M., Lough, J. M., Paris, C. B., Kingsford, M. J., Berumen, M. L., et al. (2009). Climate change and coral reef connectivity. Coral Reefs 28, 379–395. doi: 10.1007/s00338-008-0461-9
Nakamura, M., Ohki, S., Suzuki, A., and Sakai, K. (2011). Coral larvae under ocean acidification: survival, metabolism, and metamorphosis. PLoS ONE 6:e14521. doi: 10.1371/journal.pone.0014521
Nishikawa, A., Katoh, M., and Sakai, K. (2003). Larval settlement rates and gene flow of broadcast-spawning (Acropora tenuis) and planula-brooding (Stylophora pistillata) corals. Mar. Ecol. Prog. Ser. 256, 87–97. doi: 10.3354/meps256087
Nishikawa, A., and Sakai, K. (2005). Settlement-competency period of planulae and genetic differentiation of the scleractinian coral Acropora digitifera. Zool. Sci. 22, 391–399. doi: 10.2108/zsj.22.391
Nyström, M., Folke, C., and Moberg, F. (2000). Coral reef disturbance and resilience in a human-dominated environment. Trends Ecol. Evol. 15, 413–417. doi: 10.1016/S0169-5347(00)01948-0
O'Connor, M. I., Bruno, J. F., Gaines, S. D., Halpern, B. S., Lester, S. E., Kinlan, B. P., et al. (2007). Temperature control of larval dispersal and the implications for marine ecology, evolution, and conservation. Proc. Natl. Acad. Sci. U.S.A. 104, 1266–1271. doi: 10.1073/pnas.0603422104
Osborne, K., Dolman, A. M., Burgess, S. C., and Johns, K. A. (2011). Disturbance and the dynamics of coral cover on the Great Barrier Reef (1995–2009). PLoS ONE 6:e17516. doi: 10.1371/journal.pone.0017516
Paris, C. B., Atema, J., Irisson, J. O., Kingsford, M., Gerlach, G., and Guigand, C. M. (2013a). Reef odor: a wake up call for navigation in reef fish larvae. PLoS ONE 8:e72808. doi: 10.1371/journal.pone.0072808
Paris, C. B., Chérubin, L. M., and Cowen, R. K. (2007). Surfing, spinning, or diving from reef to reef: effects on population connectivity. Mar. Ecol. Prog. Ser. 347, 285–300. doi: 10.3354/meps06985
Paris, C. B., and Cowen, R. K. (2004). Direct evidence of a biophysical retention mechanism for coral reef fish larvae. Limnol. Oceanogr. 49, 1964–1979. doi: 10.4319/lo.2004.49.6.1964
Paris, C. B., Cowen, R. K., Lwizab, K. M. M., Wang, D.-P., and Olson, D. B. (2002). Multivariate objective analysis of the coastal circulation of Barbados, West Indies: implication for larval transport. Deep Sea Res. I 49, 1363–1386. doi: 10.1016/S0967-0637(02)00033-X
Paris, C. B., Guigand, C. M., Irisson, J. -O., Fisher, R., and D'Alessandro, E. (2008). “Orientation with no frame of reference (OWNFOR): a novel system to observe and quantify orientation in reef fish larvae,” in Caribbean Connectivity: Implications for Marine Protected Area Management, Proceedings of a Special Symposium, 59th Annual Meeting of the Gulf and Caribbean Fisheries Institute, eds R. Grober-Dunsmore and B. D. Keller (Silver Spring, MD: Department of Commerce, National Oceanic and Atmospheric Administration, National Marine Sanctuary Program), 54–64.
Paris, C. B., Helgers, J., Van Sebille, E., and Srinivasan, A. (2013b). Connectivity modeling system: a probabilistic modeling tool for the multi-scale tracking of biotic and abiotic variability in the ocean. Environ. Modell. Softw. 42, 47–54. doi: 10.1016/j.envsoft.2012.12.006
Paris-Limouzy, C. B. (2011). “Reef interconnectivity/larval dispersal,” in Encyclopedia of Modern Coral Reefs: Structure, Form and Process: Encyclopedia of Earth Sciences Series, ed D. Hopley (Dordrecht: Springer), 881–889.
Penin, L., Michonneau, F., Baird, A. H., Connolly, S. R., Pratchett, M. S., Kayal, M., et al. (2010). Early post-settlement mortality and the structure of coral assemblages. Mar. Ecol. Prog. Ser. 408, 55–64. doi: 10.3354/meps08554
Planes, S., and Fauvelot, C. (2002). Isolation by distance and vicariance drive genetic structure of a coral reef fish in the Pacific Ocean. Evolution 56, 378–399. doi: 10.1111/j.0014-3820.2002.tb01348.x
Raimondi, P. T., and Keough, M. J. (1990). Behavioural variability in marine larvae. Austral. J. Ecol. 15, 427–437. doi: 10.1111/j.1442-9993.1990.tb01468.x
Raimondi, P. T., and Morse, A. N. C. (2000). The consequences of complex larval behavior in a coral. Ecology 81, 3193–3211. doi: 10.1890/0012-9658(2000)081[3193:TCOCLB]2.0.CO;2
Randall, C. J., and Szmant, A. M. (2009). Elevated temperature reduces survivorship and settlement of the larvae of the Caribbean scleractinian coral, Favia fragum (Esper). Coral Reefs 28, 537–545. doi: 10.1007/s00338-009-0482-z
Richmond, R. H. (1987). Energetics, competency, and long-distance dispersal of planula larvae of the coral Pocillopora damicornis. Mar. Biol. 93, 527–533. doi: 10.1007/BF00392790
Rogers, C. S., and Miller, J. (2006). Permanent ′phase shifts' or reversible declines in coral cover? Lack of recovery of two coral reefs in St. John, US Virgin Islands. Mar. Ecol. Prog. Ser. 306, 103–114. doi: 10.3354/meps306103
Saenz-Agudelo, P., Jones, G. P., Thorrold, S. R., and Planes, S. (2011). Connectivity dominates larval replenishment in a coastal reef fish metapopulation. Proc. Biol. Sci. 278, 2954–2961. doi: 10.1098/rspb.2010.2780
Selkoe, K. A., D'Aloia, C. C., Crandall, E. D., Iacchei, M., Liggins, L., Puritz, J. B., et al. (2016). A decade of seascape genetics: contributions to basic and applied marine connectivity. Mar. Ecol. Prog. Ser. 554, 1–19. doi: 10.3354/meps11792
Selkoe, K. A., and Toonen, R. J. (2011). Marine connectivity: a new look at pelagic larval duration and genetic metrics of dispersal. Mar. Ecol. Prog. Ser. 436, 291–305. doi: 10.3354/meps09238
Shearer, T. L., and Coffroth, M. A. (2006). Genetic identification of Caribbean scleractinian coral recruits at the Flower Garden Banks and the Florida Keys. Mar. Ecol. Prog. Ser. 306, 133–142. doi: 10.3354/meps306133
Snyder, R. E., Paris, C. B., and Vaz, A. C. (2014). How much do marine connectivity fluctuations matter? Am. Nat. 184, 523–530. doi: 10.1086/677925
Stammer, D., Cazenave, A., Ponte, R. M., and Tamisiea, M. E. (2013). Causes for contemporary regional sea level changes. Annu. Rev. Mar. Sci. 5, 21–46. doi: 10.1146/annurev-marine-121211-172406
Suzuki, G., Arakaki, S., and Hayashibara, T. (2011). Rapid in situ settlement following spawning by Acropora corals at Ishigaki, southern Japan. Mar. Ecol. Prog. Ser. 421, 131–138. doi: 10.3354/meps08896
Suzuki, G., Arakaki, S., Kai, S., and Hayashibara, T. (2012). Habitat differentiation in the early life stages of simultaneously mass-spawning corals. Coral Reefs 31, 535–545. doi: 10.1007/s00338-011-0865-9
Suzuki, G., Keshavmurthy, S., Hayashibara, T., Wallace, C. C., Shirayama, Y., Chen, C. A., et al. (2016). Genetic evidence of peripheral isolation and low diversity in marginal populations of the Acropora hyacinthus complex. Coral Reefs 35, 1419–1432. doi: 10.1007/s00338-016-1484-2
Sweatman, H., Delean, S., and Syms, C. (2011). Assessing loss of coral cover on Australia's Great Barrier Reef over two decades, with implications for longer-term trends. Coral Reefs 30, 521–531. doi: 10.1007/s00338-010-0715-1
Szmant, A. M., and Gassman, N. J. (1990). The effects of prolonged “bleaching” on the tissue biomass and reproduction of the reef coral Montastrea annularis. Coral Reefs 8, 217–224. doi: 10.1007/BF00265014
Taebi, S., and Pattiaratchi, C. (2014). Hydrodynamic response of a fringing coral reef to a rise in mean sea level. Ocean Dyn. 64, 975–987. doi: 10.1007/s10236-014-0734-5
Tanner, J. E. (2017). Multi-decadal analysis reveals contrasting patterns of resilience and decline in coral assemblages. Coral Reefs 36, 1225–1233. doi: 10.1007/s00338-017-1614-5
Tay, Y. C., Guest, J. R., Chou, L. M., and Todd, P. A. (2011). Vertical distribution and settlement competencies in broadcast spawning coral larvae: implications for dispersal models. J. Exp. Mar. Biol. Ecol. 409, 324–330. doi: 10.1016/j.jembe.2011.09.013
Thompson, D. M., Kleypas, J., Castruccio, F., Curchitser, E. N., Pinsky, M. L., Jonsson, B., et al. (2018). Variability in oceanographic barriers to coral larval dispersal: Do currents shape biodiversity? Prog. Oceanogr. 165, 110–122. doi: 10.1016/j.pocean.2018.05.007
Toonen, R. J., and Grosberg, R. K. (2011). “Causes of chaos: spatial and temporal genetic heterogeneity in the intertidal anomuran crab Petrolisthes cinctipes,” in Phylogeography and Population Genetics in Crustacea. eds C. Held, S. Koenemann and C. D. Schubart (Boca Raton, FL: CRC press), 73–106.
Toonen, R. J., and Pawlik, J. R. (2001). Foundations of gregariousness: a dispersal polymorphism among the planktonic larvae of a marine invertebrate. Evolution 55, 2439–2454. doi: 10.1111/j.0014-3820.2001.tb00759.x
Torda, G., Lundgren, P., Willis, B. L., and van Oppen, M. J. (2013). Genetic assignment of recruits reveals short- and long-distance larval dispersal in Pocillopora damicornis on the Great Barrier Reef. Mol. Ecol. 22, 5821–5834. doi: 10.1111/mec.12539
Treml, E. A., Roberts, J., Halpin, P. N., Possingham, H. P., and Riginos, C. (2015). The emergent geography of biophysical dispersal barriers across the Indo-West Pacific. Divers. Distributions 21, 465–476. doi: 10.1111/ddi.12307
Tsounis, G., and Edmunds, P. J. (2016). The potential for self-seeding by the coral Pocillopora spp. in Moorea, French Polynesia. PeerJ 4:e2544. doi: 10.7717/peerj.2544
van Oppen, M. J., Lutz, A., De'ath, G., Peplow, L., and Kininmonth, S. (2008). Genetic traces of recent long-distance dispersal in a predominantly self-recruiting coral. PLoS ONE 3:e3401. doi: 10.1371/journal.pone.0003401
van Woesik, R., Sakai, K., Ganase, A., and Loya, Y. (2011). Revisiting the winners and the losers a decade after coral bleaching. Mar. Ecol. Prog. Ser. 434, 67–76. doi: 10.3354/meps09203
Vaz, A. C., Richards, K. J., Jia, Y., and Paris, C. B. (2013). Mesoscale flow variability and its impact on connectivity for the island of Hawai'i. J. Geophys. Res. 40, 332–337. doi: 10.1029/2012GL054519
Veron, J. E. N. (1995). Corals in Space and Time: The Biogeography and Evolution of the Scleractinia. Ithaca, NY: Cornell University Press.
Webster, P. J., Holland, G. J., Curry, J. A., and Chang, H. R. (2005). Changes in tropical cyclone number, duration, and intensity in a warming environment. Science 309, 1844–1846. doi: 10.1126/science.1116448
Weersing, K., and Toonen, R. J. (2009). Population genetics, larval dispersal, and connectivity in marine systems. Mar. Ecol. Prog. Ser. 393, 1–12. doi: 10.3354/meps08287
Werner, F. E., Cowen, R. K., and Paris, C. B. (2007). Coupled biological and physical models: present capabilities and necessary developments for future studies of population connectivity. Oceanography 20, 54–69. doi: 10.5670/oceanog.2007.29
White, C., Selkoe, K. A., Watson, J., Siegel, D. A., Zacherl, D. C., and Toonen, R. J. (2010). Ocean currents help explain population genetic structure. Proc. Biol. Sci. 277, 1685–1694. doi: 10.1098/rspb.2009.2214
Wolanski, E. (2017). Bounded and unbounded boundaries – untangling mechanisms for estuarine-marine ecological connectivity: scales of m to 10,000 km – a review. Estuar. Coast. Shelf Sci. 198, 378–392. doi: 10.1016/j.ecss.2016.06.022
Wolanski, E., and Hamner, W. M. (1988). Topographically controlled fronts in the ocean and their biological influence. Science 241, 177–181 doi: 10.1126/science.241.4862.177
Wood, S., Baums, I. B., Paris, C. B., Ridgwell, A., Kessler, W. S., and Hendy, E. J. (2016). El Niño and coral larval dispersal across the eastern Pacific marine barrier. Nat. Commun. 7:12571. doi: 10.1038/ncomms12571
Wood, S., Paris, C. B., Ridgwell, A., and Hendy, E. J. (2014). Modelling dispersal and connectivity of broadcast spawning corals at the global scale. Global Ecol. Biogeogr. 23, 1–11. doi: 10.1111/geb.12101
Keywords: marine larvae, coral reef, scleractinia, connectivity, resilience, ecology
Citation: Edmunds PJ, McIlroy SE, Adjeroud M, Ang P, Bergman JL, Carpenter RC, Coffroth MA, Fujimura AG, Hench JL, Holbrook SJ, Leichter JJ, Muko S, Nakajima Y, Nakamura M, Paris CB, Schmitt RJ, Sutthacheep M, Toonen RJ, Sakai K, Suzuki G, Washburn L, Wyatt ASJ and Mitarai S (2018) Critical Information Gaps Impeding Understanding of the Role of Larval Connectivity Among Coral Reef Islands in an Era of Global Change. Front. Mar. Sci. 5:290. doi: 10.3389/fmars.2018.00290
Received: 16 February 2018; Accepted: 30 July 2018;
Published: 27 August 2018.
Edited by:
Jesús Ernesto Arias González, Centro de Investigación y de Estudios Avanzados del Instituto Politécnico Nacional (CINVESTAV-IPN), MexicoReviewed by:
Aldo Cróquer, Simón Bolívar University, VenezuelaCarolyn J. Lundquist, National Institute of Water and Atmospheric Research (NIWA), New Zealand
Copyright © 2018 Edmunds, McIlroy, Adjeroud, Ang, Bergman, Carpenter, Coffroth, Fujimura, Hench, Holbrook, Leichter, Muko, Nakajima, Nakamura, Paris, Schmitt, Sutthacheep, Toonen, Sakai, Suzuki, Washburn, Wyatt and Mitarai. This is an open-access article distributed under the terms of the Creative Commons Attribution License (CC BY). The use, distribution or reproduction in other forums is permitted, provided the original author(s) and the copyright owner(s) are credited and that the original publication in this journal is cited, in accordance with accepted academic practice. No use, distribution or reproduction is permitted which does not comply with these terms.
*Correspondence: Peter J. Edmunds, cGV0ZXIuZWRtdW5kc0Bjc3VuLmVkdQ==