- 1Department of Biology, The University of North Carolina at Chapel Hill, Chapel Hill, NC, United States
- 2Department of Biology, Institute for Coastal Science and Policy, East Carolina University, Greenville, NC, United States
Temperature can influence trophic interactions via predictable effects on the metabolism of ecothermic consumers. Under some conditions, warming should increase top–down control, and trophic transfer rates, leading to declines in prey populations. We tested this prediction in the Galápagos Islands, an equatorial upwelling region, where water temperatures are highly variable and nutrient availability is thought to control primary production and standing algal biomass. We used grazing assays, field surveys, and a herbivore exclusion experiment to test the hypothesis that grazing rate and algal biomass are, in part, regulated by temperature via the temperature–dependence of herbivory. Grazing rates were greater during the warm season for urchins and other consumers (including fishes, turtles, and iguanas). Field surveys at 10 sites over 5 years found that temperature was strongly negatively related to macroalgal cover. The results of the exclusion experiment indicate that herbivores had a large effect on macroalgal biomass, even during intense upwelling. Our results suggest that in shallow subtidal habitats across the Galápagos archipelago, grazing pressure increases with temperature, potentially resulting in reduced algal biomass when upwelling is weak and greater algal biomass when upwelling is strong and water is cold; an alternative explanation for widely observed association between upwelling intensity and algal biomass.
Introduction
Due to the temperature dependence of intra–cellular enzyme activity, the biological rates of ectotherms are generally faster at higher temperatures (Huey and Kingsolver, 1989; Pepin, 1991; Gillooly et al., 2001; Clarke and Johnston, 2016). This can affect the strength and outcome of species interactions via predictable effects of temperature on biological processes including primary production, decomposition, digestion, escape velocity, foraging rate, and individual and population growth (Enquist et al., 2003; Brown et al., 2004; Heard et al., 2012; Dell et al., 2014; Bruno et al., 2015). Numerous lab–based experiments (i.e., environmental chambers) and outdoor mesocosm studies have found that sublethal warming can increase per capita grazing rates and the top–down effects of herbivores on plant communities (Largen, 1967; Stickle et al., 1985; Sanford, 2002; O'Connor et al., 2009; Kordas et al., 2011; Kratina et al., 2012; Lemoine and Burkepile, 2012; Carr and Bruno, 2013). Further, in a remarkable large–scale (if unreplicated) field test, Svensson et al. (2017) used the artificially warmed Forsmark biotest basin in the northern Baltic Sea to measure the effects of warming on herbivores and algae in the presence and absence of carnivorous perch. As predicted by theory, they found that warming increased predation on mesopredators and induced a trophic cascade, substantially increasing filamentous algal biomass. Warming appears to have increased the metabolism and predation rate of perch, and also caused perch to begin consuming gammarid amphipods (after they depleted their normal prey presumably due to increased metabolic demand), thereby reducing grazing and indirectly facilitating algae. As predicted and demonstrated in more controlled mesocosm experiments, warming also simplified the food web by effectively removing one trophic level of small fishes (sticklebacks and minnows), that in the cooler control site fed on the amphipods and were preyed on by the perch (Svensson et al., 2017).
Because grazing rate can influence the biomass of primary producers (Paine, 1992; Burkepile and Hay, 2006), the temperature dependence of herbivore–plant interactions could indirectly influence a variety of community properties and ecosystem functions. Moreover, temperature–dependent herbivory is an alternative explanation for the positive association between the biomass of primary producers and the occurrence or intensity of upwelling. This widespread pattern is assumed to be caused only by the increase in nutrient concentration associated with upwelling, enabling higher primary production and greater standing algal biomass (Menge and Branch, 2001; Menge et al., 2002; Vinueza et al., 2014). However, upwelling also causes large, rapid declines in water temperature (Sanford, 1999). Because the metabolism of fish and invertebrate herbivores is temperature–dependent, cooler upwelled water could reduce consumer metabolism and grazing intensity (Bruno et al., 2015). This could in turn lead to increased standing algal biomass. Therefore, upwelling could influence both bottom–up and top–down control of populations and communities of primary producers.
The purpose of this study was to test the hypothesis that grazing rate and algal biomass are, in part, regulated by temperature via the temperature–dependence of herbivory. We combined three types of evidence to measure the effect of temperature on herbivory and algal biomass. First, we performed grazing assays during warm and cold (upwelling) seasons at four sites varying in temperature. Second, we measured herbivore density and algal community structure (cover and composition) across this spatiotemporal temperature gradient to determine how they were related to temperature and also to control for grazer density in the analysis of the grazing assays. Third, we performed a 10–week field exclusion experiment to measure the effect of two herbivore functional groups on macroalgal biomass during the upwelling season, when bottom–up forces were assumed to negate top–down control. Overall, our results suggest that temperature could influence macroalgal biomass, even in an upwelling region where algal community structure is thought to be controlled solely by nutrient availability.
Methods
Study System
This system is ideal for measuring the effects of temperature on species interactions because the archipelago is centered at the convergence of several different oceanographic currents (tropical, subtropical, and upwelled water), resulting in enormous spatiotemporal variation in water temperature (for an equatorial region of the ocean) of ~14–30°C (Houvenaghel, 1978; Schaeffer et al., 2008; Witman et al., 2010). Further, while community composition differs across the Archipelago due to upwelling intensity, there is a suite of organisms that are present at all the sites throughout the year. In the Galápagos shallow subtidal, macroalgae are generally the dominant sessile group and sea urchins are typically the most important invertebrate grazer guild (Irving and Witman, 2009; Brandt et al., 2012). At high densities, sea urchins can convert macroalgal assemblages to urchin barrens or pavements of encrusting algae (Edgar et al., 2010). The two most common sea urchins in rocky subtidal habitats at depths between 1 and 5 m are Lytechinus semituberculatus (green sea urchin) and Eucidaris galapagensis (slate pencil urchin) (Irving and Witman, 2009; Brandt et al., 2012). Other important grazers include fishes (razor surgeonfishes; Prionurus laticlavius, blue chin parrotfish; Scarus ghobban), sea turtles, and marine iguanas.
Grazing Assays
We performed short–term grazing assays in situ at four sites during each of two seasons (warm and cold) in 2013 to measure the effect of temperature on herbivory. We chose the foliose algae Ulva spp. as the prey item because it is by far the most abundant macroalgal species in intertidal and shallow subtidal habitats in the Galápagos (Vinueza et al., 2006; Carr and Bruno, 2013) (Table 1) and also because it is highly palatable (Carpenter, 1986). As a dominant benthic space occupier in the Galápagos shallow subtidal, changes in herbivore–Ulva interactions could influence algal community biomass and food web structure.
The four grazing assay sites (Figure 1) were semi–protected from wave action on four islands that vary in temperature due to variation in upwelling intensity and other oceanographic features such as ocean currents. The temperature range (mean values among the four sites) was 24.5–29.5°C during the warm season (Feb/Mar 2013) and 19.8–24.7°C during the cold season (Sept/Oct 2013). Site selection minimized among–site variation in other factors that could potentially affect grazing rate.
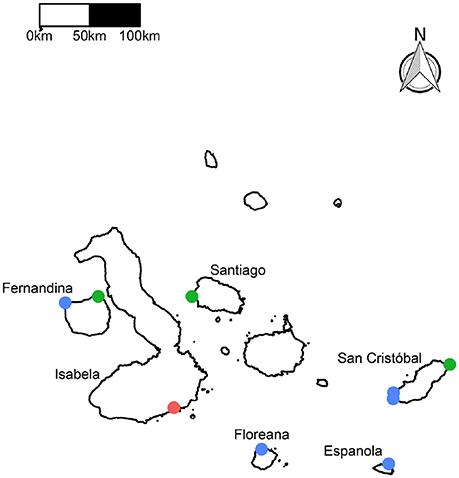
Figure 1. Map of the nine study sites in the Galápagos Islands. Benthic surveys were performed at all nine sites. Survey only sites are blue, grazing assay sites are green, and the experimental site is red.
Fernandina is located in the western region of the Archipelago. The Equatorial Undercurrent (EUC) drives major upwelling as it collides with the western islands, resulting in cold and nutrient–rich waters around Fernandina (Houvenaghel, 1978). The grazing assay site on Fernandina was located at Punta Espinosa, on the northeastern point of the island (Figure 1) and was the coolest site. Isabela is also located in the western region, and assays were conducted on the southern edge of the island at Túnel del Estero. This region is influenced by the EUC and the cool (and nutrient–rich) Humboldt Current from the south, therefore, this region of Isabela is also considered an upwelling region (Houvenaghel, 1978; Schaeffer et al., 2008). Santiago is a northern island, located in the central region of the Archipelago. The Puerto Egas site is located on the northwest side of Santiago. Puerto Egas is influenced by the South Equatorial Current (SEC; a westward flowing current that strongly influences the central region of the Archipelago), the North Equatorial Countercurrent (NECC, a warm and nutrient–poor current) and EUC meanderings, resulting in high productivity, yet warmer waters (Houvenaghel, 1978; Schaeffer et al., 2008; Figure 1). San Cristóbal is the easternmost island in the Archipelago. Grazing assays were conducted at Punta Pitt located on the northeastern point of the island, influenced by the SEC, Humboldt Current, and the NECC (Houvenaghel, 1978; Wellington et al., 2001; Schaeffer et al., 2008). In general, this site has depressed nutrient loads and warmer waters relative to the other sites, as it is north of the upwelling region off San Cristóbal (Houvenaghel, 1978; Schaeffer et al., 2008). Both the NECC and the Humboldt Current contribute to the SEC, and the dominant influence of the SEC changes seasonally (Houvenaghel, 1978; Schaeffer et al., 2008). During the cool season (May–December), the Humboldt Current is the major contributor to the SEC; with the minimum average SST usually occurring in August or September. The maximum average SST usually occurs in February and March.
We simultaneously performed two grazing assays: one with green urchins (L. semituberculatus) and the other with all herbivores present at a given site. The urchin-only grazing assays were performed in cylindrical cages constructed from plastic vexar (30 cm wide, 15 cm tall, mesh size of 2.5 cm), attached to chains on the substrate at 3–5 m depth. Three urchins were placed in each cage along with 5.00 ± 0.06 g (wet mass) of Ulva (n = 10). Urchins were starved for 12 h before Ulva prey was added. Ulva was strung onto bead wire and then was attached to the vexar on cage floors. Urchin densities in the cages were representative of natural urchin densities at these sites (Table 1). Scaled for comparison, we used 21 green urchins per 0.5 m2, and the range of green urchin densities found at the 4 grazing assay sites was 11–39 per 0.5 m2. The average test size for the urchins placed in cages across all sites and both time periods was 3.82 ± 0.09 cm (Table 1), which is representative of the green sea urchin populations across the archipelago. Water temperature was measured and recorded every 5 min with an Onset temperature logger during each assay.
In the second grazing assay, Ulva was attached to round vexar discs attached to the chains (n = 10), so that any herbivore could access the experimental algae. This assay was meant to assess the temperature effect on grazing under more natural conditions (i.e., in which herbivore density and composition were not manipulated) and for other herbivores. Herbivores present at the site with access to the algae included several other urchin species, herbivorous fishes, turtles, and iguanas. Assays were terminated after 24 h, as over ~50% of algal tissue was consumed (Tomas et al., 2011), and final wet algal biomass was measured after removing excess water with a salad spinner (60 revolutions).
Field Surveys
We quantified natural herbivore abundance and benthic community composition to determine how temperature was related to macroalgal cover, and secondarily whether this relationship was confounded by grazer density. Between 2010 and 2014, nine sites were surveyed adjacent to six islands that differ in nutrient availability and temperature due to variation in upwelling intensity and other oceanographic factors (Vinueza et al., 2006, 2014; Schaeffer et al., 2008). All sites were surveyed at least once, and most sites were surveyed multiple times (i.e., during the warm and cool season and/or during a warm and cool year) for a total of 37 surveys. Surveys were performed along five 30–m transects placed parallel to shore at 3–4 m depth. Along each transect, five photoquadrats were placed adjacent to the transect line at fixed intervals, totaling 25 photoquadrats per survey. Each photograph captured an area of 0.42 m2. One hundred points were placed over each photograph in a stratified random design. Image analysis was conducted with ImageJ v1.48. Percent cover of each algal species was classified to genus and filamentous algae was distinguished as red (Centroceras spp., Ceramium spp., Polysiphonia spp.), green (Bryposis spp., Chaetomorpha antennina) or brown (Ectocarpus spp.). Divers conducted visual herbivore censuses along 30 × 2 m belt transects (five per survey) by recording the species–level identity and length of each individual (fishes, iguanas, and turtles). Fish length estimates were converted to fish biomass estimates using published length–biomass relationships (Froese and Pauly, 2013). No iguanas or turtles were encountered in the transects, although they were often observed nearby. Urchin identity and abundance was determined by counting all urchins along five 30 × 1 m belt transects.
Exclusion Experiment
To quantify the effect of herbivores on algal biomass, we conducted a 10–week field exclusion experiment at the Isabela site (Figure 1) during the cold season (September–December 2013). Forty boulders (~35 cm × 25 cm × 15 cm; l × w × h) at a depth of 5 m were collected, brought to the surface, and completely cleared of macroalgae and invertebrates. Each boulder was randomly assigned one of the following treatments (n = 10): (1) open plots that allowed access to all grazers, (2) cages with sides but no top, designed to exclude urchins, but allowed fish, iguana, and sea turtle grazing, i.e., “mobile” herbivores, (3) cages with a top and openings in the sides that prevented grazing by mobile herbivores but allowed access by urchins and other benthic grazers, and (4) full cages that excluded all macroherbivores but not mesograzers such as amphipods or small snails. Cages were 15 × 15 × 15 cm with a mesh size of 2.5 cm. Cages were left in place for 10 weeks, during which a macroalgal community developed, but fouling did not overwhelm the cages. At the conclusion of the experiment, macroalgae was collected, sorted by functional group (or genus), and weighed. Excess water was removed with a salad spinner (60 revolutions) before measuring algal wet mass.
Statistical Analyses
The results of the grazing assays were analyzed with two–factor analysis of variance (ANOVA), using the final algal wet mass as the response variable and season and site as fixed factors.
We used generalized linear mixed–effects models to estimate the relationship between temperature (and other abiotic factors) and herbivore density and the benthic cover of macroalgae. Fixed factors included: herbivorous fish biomass, green and pencil urchin densities, temperature (measured for 30 days prior to sampling date, with either HOBO temperature loggers or AHVRR Pathfinder Version 5.2, PFV5.2, satellite data obtained from the US National Oceanographic Data Center and GHRSST, NOAA 2013), ENSO cycle (obtained from the Multivariate ENSO Index, maintained by NOAA, and based on the Oceanic Niño Index, ONI, or the running 3–month mean SST anomaly), and distance to and area of closest upwelling region to each site measured from Schaeffer et al. (2008). Because of repeated measurements at the same sites over multiple years (2010–2014), we nested sites within years. This random effect of the model structure accounts for the statistical non-independence of these observations. The best fit model was chosen with an Information Theoretic approach (Burnham, 2004). We calculated differences between the Akaike Information Criterion (AICc, for small sample sizes) of each model and the minimum AIC. All numerical explanatory covariates were standardized (divided by two standard deviations) and centered to compare relative effect sizes. We evaluated multi–collinearity between all explanatory covariates with a Spearman rank (rs) correlation matrix and pairs plot based on the mean values. A logistic (logit) transformation was applied to the percent cover data. Normality was determined by plotting the theoretical quantiles vs. the standardized residuals (Q–Q plots). Homogeneity of variance was evaluated by plotting the residuals vs. the fitted values for the final model and for each of the covariates.
For the field exclusion experiment, we compared treatment effects on final algal biomass with ANOVA and Tukey's HSD post-hoc tests. All analyses were performed in the statistical software R v.3.1.2.
Results
Grazing Assays
In the urchin–only grazing assay, grazing rates were more than five times greater during the warm season than the cold season (Figure 2A) at all four sites, and there was a highly statistically significant effect of season (P < 0.0001). The site effect and the season x site interaction were both marginally significant (P = 0.02), both due apparently to the especially low grazing rate at the Isabella site during the cold season. Grazing rates were also lower during the cold season for the all-herbivores grazing assay (Figure 2B), for which the season effect was significant (P < 0.0001) but the site effect and the season x site interaction were not (P = 0.25 and 0.55 respectively).
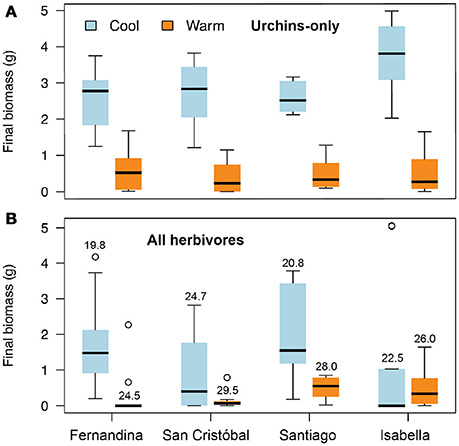
Figure 2. Effects of season (temperature) and site on the grazing rate of Ulva by urchins (A) and all herbivores (B). Solid black midlines are median values, the upper and lower limits of the box indicate the third and first quartiles, and the circles are outliers. Values above the boxes in the lower plot are the mean temperature (°C) during both grazing assays (values also apply to the urchin-only assays) at the four sites during the two seasons.
Field Surveys
Across all sites and time periods, mean Ulva cover was 23%, crustose coralline algae (CCA) was 17%, red filamentous algae was 12%, brown filamentous algae was 11%, coralline macroalgae was 7%, green filamentous algae was 5%, corticated algae was 5%, and bare space was 17%. Spatial and temporal variability in Ulva cover was negatively related to temperature, herbivorous fish biomass, and green urchin density (Table S1, Figures 3A, 4A,B). Non- Ulva macroalgal cover was negatively related to temperature (Table S1, Figure 3B) but not herbivore densities (Figures 4C,D). In other words, at higher temperatures, non- Ulva macroalgal cover decreased, independent of herbivore density.
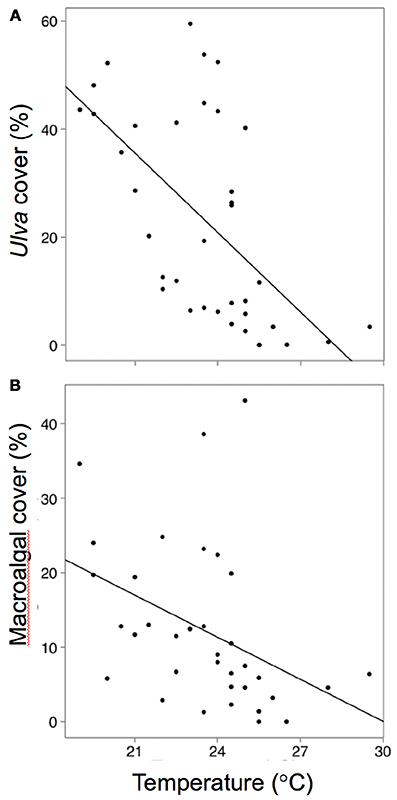
Figure 3. Relationships between (A) ocean temperature and Ulva cover (P < 0.0001, R2 = 21.8), and (B) cover by all other macroalgae (not including Ulva: P = 0.01, R2 = 14.4). Each point represents a single survey at a given site/time period.
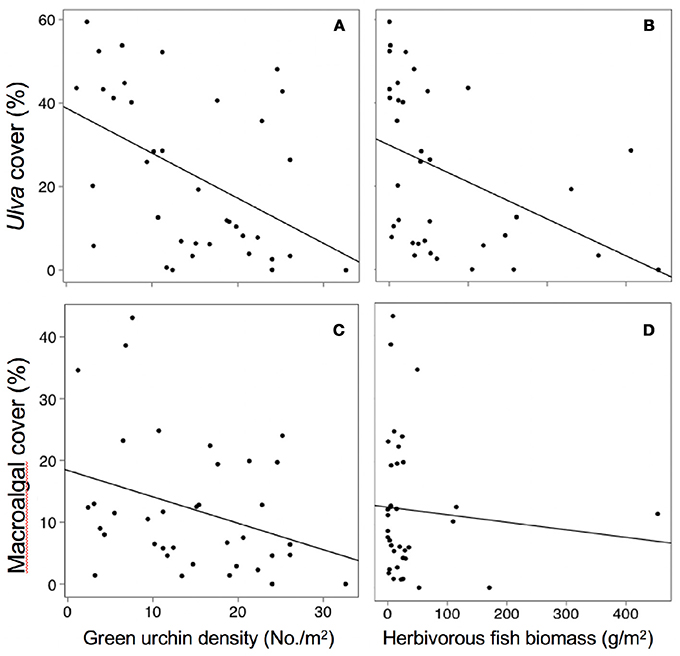
Figure 4. Relationships between herbivore abundance and algal cover. Note in panels C and D “macroalgal cover” does not include Ulva cover. Each point represents a single survey at a given site / time period. (A) P < 0.0001, R2 = 0.22, (B) P < 0.011, R2 = 0.18, (C) P < 0.05, R2 = 0.11, (D) P < 0.45, R2 = 0.02.
We defined “cold” sampling periods as those where mean SST was < 24°C. This delineation is based on the signature of the EUC, adjusted to account for shallow water heating and mixing with the other tropical surface currents. Total non- Ulva macroalgal cover was higher at the cold (48%, n = 21) than the warm (18%, n = 17) sampling periods (Figure 5). The same was true for; foliose (32% ± 3.9% compared to 10% ± 3.0%), coralline algae (10% ± 1.8% compared to 4% ± 2.0%), and filamentous algae (34% ± 4.2% compared to 25% ± 8.0%). Conversely, the cover of bare space was greater during warm (25% ± 2.9%) than cold (11% ± 2.1%) periods, as was the cover of crustose algae (24% ± 0.6% compared to 15% ± 0.8%, Figure 5).
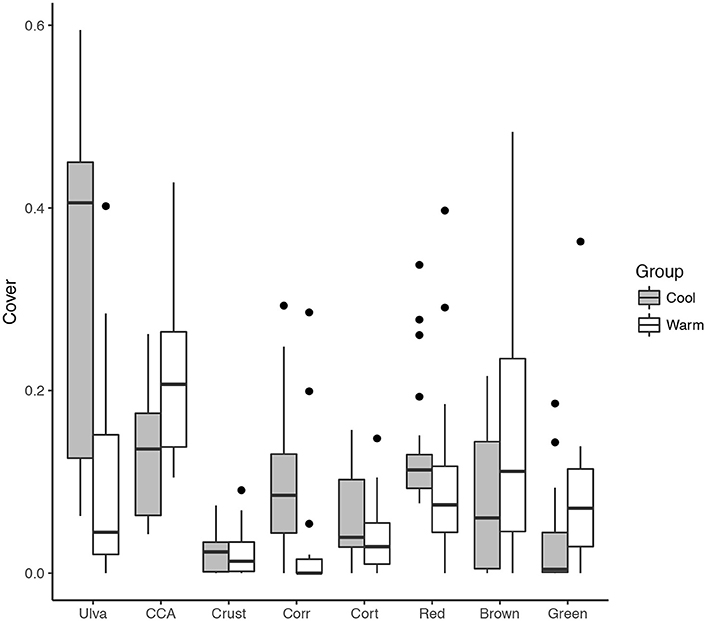
Figure 5. Comparison of the coverage of major benthic groups between “cool” (< 24°C, n = 21) and “warm” (< 24°C, n = 17) sampling periods. Algal groups are shown on the X-axis as Ulva, CCA, crustose, corticated, and filamentous, which are grouped as Red (Centroceras spp., Ceramium spp., Polysiphonia spp.), Green (Bryposis spp., Chaetomorpha antennina), and Brown (Ectocarpus spp.).
Exclusion Experiment
The herbivore presence and composition treatments significantly affected final algal biomass (P < 0.0001), with 90% more Ulva spp. biomass in the full exclusion plots (the “None” plots in Figure 6 which no macro-herbivores were able to access) as compared to the “All” plots, accessible by all herbivores. Algal biomass did not differ between the mobile herbivore and benthic herbivore treatments (post-hoc Tukey HSD tests). Final algal wet biomass of the mobile and benthic treatments differed significantly from both the “All” treatment and the “None” treatment. The dominant algal functional group in all four treatments was green sheet–like algae (Ulva spp.) and relative biomass was similar across treatments: 82.3, 80.7, 76.1, and 78.2%, open plots, benthic herbivores, mobile herbivores, and full exclusions respectively. The other algal functional groups present were red corticated algae (Hypnea spp.) and red filamentous algae (Ceramium spp., Centroceras spp. and Polysiphonia spp.).
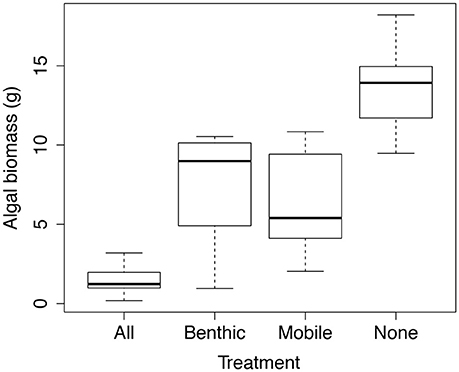
Figure 6. Herbivore effects on algal biomass, plotted as final wet mass at the conclusion of the 10 week field experiment. Treatment labels indicate which herbivores were able to access the plots. “Benthic” = urchins (and potentially other benthic grazers) and “Mobile” = fishes, iguanas, and turtles. n = 10.
Discussion
Grazing Assays: Temperature–Dependent Herbivory
Sublethal warming ranging from 4.7 to 7.2°C (among the four sites) significantly increased the strength of herbivory. These results are consistent with predictions from metabolic theory and numerous empirical studies conducted in environmental chambers (O'Connor, 2009; Kordas et al., 2011; O'Connor et al., 2011), including with Lytechinus urchins (Carr and Bruno, 2013). The open, all-herbivore plots allowed unrestricted grazer access and tested the generality of temperature–dependent herbivory to other grazer guilds (i.e., fishes, sea turtles, and marine iguanas) under more natural conditions. We expected to see higher variance in the open plot grazing due to spatiotemporal variation in grazer composition and density. Nonetheless, more Ulva was consumed during the warm season (Figure 2B), suggesting the observed effects of temperature are general and relatively strong. There was no relationship between green urchin density and open plot grazing rates (urchin density was controlled in the urchin–only cages), suggesting that although green urchin density varied between sites and time periods, this was not a significant factor influencing open plot grazing rates.
Grazing Assays: Hints of Thermal Acclimatization
In the urchin-only grazing assay, grazing rates were generally consistent across sites, despite substantial differences in temperature (Figure 2) suggesting site–specific temperature effects. For example, the warm season grazing rate was nearly identical at the Fernandina and San Cristóbal sites, despite a 5°C difference in temperature. The one exception to this pattern was at the Isabella site during the cool season, when grazing was especially low. The temperature was not lower than for the other three sites, so the cause of this result is not clear. Consistent performance among sites, despite differences in temperature, is consistent with the hypothesis that urchin populations are acclimatized or adapted to local thermal conditions. That thermal history influences temperature sensitivity is well documented (Roberts, 1957; Helmuth et al., 2002; Howells et al., 2013). For example, Marshall and McQuaid (2010) found that the relationship between the metabolic rate of marine snails and temperature depends on the temperature range and variability experienced by the organism. However, there are other explanations for the observed variation in the temperature–dependence of grazing across sites. For example, wave exposure and other physical attributes such as flow characteristics could have influenced herbivory (Dayton, 1985; Irving and Witman, 2009).
Exclusion Experiment: Top-Down Control During Upwelling
Upwelling communities are believed to be strongly influenced by bottom–up effects. A widely–observed pattern is the positive association between the biomass of primary producers and the occurrence and intensity of upwelling (Menge and Branch, 2001; Menge et al., 2002; Vinueza et al., 2014). The generally accepted explanation is that upwelling increases the flux of limiting nutrients to phytoplankton and macroalgae, thereby increasing primary productivity and standing algal biomass (Bustamante et al., 1995; Broitman et al., 2001; Menge and Branch, 2001; Menge et al., 2002; Nielsen, 2003; Nielsen and Navarrete, 2004; Vinueza et al., 2006, 2014). However, a strong role of top–down control is also recognized in marine communities (Menge, 1991; Paine, 1992; Leonard et al., 1998; Trussell et al., 2002; Harley, 2011). Furthermore, in many nearshore systems, the processes that mediate resource supply can also influence consumers and predation intensity, so that bottom–up and top–down forces are mechanistically intertwined and often negatively related. For example, food flux to filter–feeding invertebrates in rocky intertidal sites in Maine is controlled largely by flow velocity, yet high flow also deters predators, thereby releasing prey from top–down control at locations where food and nutrient flux is greatest (Leonard et al., 1998).
The results of the herbivore–exclusion experiment suggest that grazers have a strong effect on macroalgal biomass in shallow subtidal habitats in the Galápagos, even during intense upwelling when nutrient flux was presumably high. At the end of the 10–week experiment, plots open to all grazers (Figure 6) had very little algae and significantly less than the other three treatments. There was no difference in algal biomass between the Mobile and Benthic herbivore treatments, suggesting that both groups had similar effects on macroalgae. Moreover, algal biomass in these treatments was roughly half that in total exclusion treatments (plots which no herbivore could access) and double what was seen in the “all” herbivore plots, suggesting that the effects of mobile and benthic grazers are roughly additive.
The Potential Effect of Temperature-Dependent Herbivory
Our field exclusion experiment clearly demonstrates the large role of herbivores in controlling standing algal biomass during the upwelling season in an upwelling region, indicating strong top–down control. The grazing assays, and previous laboratory experiments with the same species (Carr and Bruno, 2013), indicate that grazing by one of the system's dominant consumers is temperature-dependent. Considered together, these results lead to the inference that grazing pressure is higher when and where upwelling is relaxed across Galápagos archipelago. This interpretation suggests it is plausible that the widely–observed association between higher algal biomass and upwelling intensity could be due in part to reduced grazing pressure during upwelling via the temperature–dependent metabolism of ectothermic herbivores. This interpretation is also concordant with the survey data indicating that macroalgal cover was far greater during cool season than warm season surveys. Thus, our findings suggest that temperature plays an important, but frequently overlooked influence on species interactions and community structure. We did not assess the role of nutrient flux and it is likely that it and other factors influence standing algal biomass. Measuring the relatively and interactive effects of temperature and nutrients on macroalgae would require nutrient manipulations and measurements, both of which were outside the scope of this study.
Vinueza et al. (2006, 2014), working in intertidal habitats in the Galápagos (our study was conducted in the shallow subtidal), attributed among–site variation in algal biomass and productivity to local nutrient concentration via spatiotemporal variation upwelling intensity. However, nutrient concentration was not quantified or manipulated. Moreover, their results were also consistent with temperature–dependent grazing, as temperature was positively related to grazing rate (and generally declined during upwelling). Favoring a bottom–up explanation over the possibility of temperature–mediated top–down control is a nearly universal phenomenon in studies in upwelling systems (Menge and Branch, 2001; Nielsen and Navarrete, 2004). Furthermore, the norm is to not measure or even manipulate nutrient availability, but rather to use upwelling (and chlorophyll a) as a natural (nutrient availability) treatment even though upwelled water is not just nutrient rich, but also much cooler. These mensurative studies have led to the paradigm that algal–biomass in upwelling systems is entirely bottom–up controlled. Nutrient availability and other factors very likely influence macroalgal biomass in this system, however, we propose that top–down and bottom–up processes are coupled via upwelling in the Galápagos islands, as they are in other systems (Leonard et al., 1998). Untangling the relative role of nutrients and temperature is the next step in work on the role of environmental processes like upwelling, in coastal ecosystems.
Author Contributions
LC and JB designed the study with help from RG. All three authors performed the fieldwork. Carr wrote the first draft of the manuscript. JB and RG edited the manuscript and wrote specific sections. LC, RG, and JB performed statistical analysis. All authors made graphics.
Funding
This study was supported by funds from the Lerner–Gray Marine Research Fund, the Department of Biology and the Graduate School at UNC, and the Phycological Society of America, and by the National Science Foundation (OCE #1737071 to JB).
Conflict of Interest Statement
The authors declare that the research was conducted in the absence of any commercial or financial relationships that could be construed as a potential conflict of interest.
Acknowledgments
We thank C. Cox, A. Valdivia, F. J. Fodrie, and J. Witman for providing helpful comments that improved the manuscrpt. We thank the Galápagos National Park, S. Walsh, C. Mena, P. Page, L. Vaca, F. J. Fodrie, D. Amoguimba, and the Galápagos Science Center for providing field and logistical assistance. The crews of the Pirata and the Oberlus II provided reliable access to our study sites; and we especially thank Captain Lenin Cruz Bedon and the Escarabay brothers. We thank P. Mejia and S. Villamar for their help in the field. We thank Maryuri Yepez, Galo Quezada, and Jon Witman for their continued and valuable support and help.
Supplementary Material
The Supplementary Material for this article can be found online at: https://www.frontiersin.org/articles/10.3389/fmars.2018.00279/full#supplementary-material
References
Brandt, M., Witman, J. D., and Chiriboga, A. I. (2012). Influence of a dominant consumer species reverses at increased diversity. Ecology 93, 868–878. doi: 10.1890/10-1785.1
Broitman, B. R., Navarrete, S. A., and Smith, F. (2001). Geographic variation of southeastern Pacific intertidal communities. Mar. Ecol. Prog. Ser. 224, 21–34. doi: 10.3354/meps224021
Brown, J. H., Gillooly, J. F., Allen, A. P., Savage, V. M., and West, G. B. (2004). Toward a metabolic theory of ecology. Ecology 85, 1771–1789. doi: 10.1890/03-9000
Bruno, J. F., Carr, L. A., and O'Connor, M. I. (2015). Exploring the role of temperature in the ocean through metabolic scaling. Ecology 96, 3126–3140. doi: 10.1890/14-1954.1
Burkepile, D. E., and Hay, M. E. (2006). Herbivore vs. nutrient control of marine primary producers: context-dependent effects. Ecology 87, 3128–3139. doi: 10.1890/0012-9658(2006)87[3128:HVNCOM]2.0.CO;2
Burnham, K. P. (2004). Multimodel inference: understanding AIC and BIC in model selection. Sociol. Methods Res. 33, 261–304. doi: 10.1177/0049124104268644
Bustamante, R. H., Branch, G. M., Eekhout, S., Robertson, B., Zoutendyk, P., Schleyer, M., et al. (1995). Gradients of intertidal primary productivity around the coast of South Africa and their relationships with consumer biomass. Oecologia 102, 189–201. doi: 10.1007/BF00333251
Carpenter, R. C. (1986). Partitioning herbivory and its effects on coral-reef algal communities. Ecol. Monogr. 56, 345–363.
Carr, L. A., and Bruno, J. F. (2013). Warming increases the top-down effects and metabolism of a subtidal herbivore. PeerJ 1, 1–15. doi: 10.7717/peerj.109
Clarke, A., and Johnston, N. M. (2016). Scaling of metabolic rate with body mass and temperature in teleost fish. J. Anim. Ecol. 893–905. doi: 10.1046/j.1365-2656.1999.00337.x
Dell, A. I., Pawar, S., and Savage, V. M. (2014). Temperature dependence of trophic interactions are driven by asymmetry of species responses and foraging strategy. J. Anim. Ecol. 83, 70–84. doi: 10.1111/1365-2656.12081
Edgar, G. J., Banks, S. A., Brandt, M., Bustamante, R. H., Chiriboga, A., Earle, S. A., et al. (2010). El Niño, grazers and fisheries interact to greatly elevate extinction risk for Galápagos marine species. Glob. Chang. Biol. 16, 2876–2890. doi: 10.1111/j.1365-2486.2009.02117.x
Enquist, B. J., Economo, E. P., Huxman, T. E., Allen, A. P., Ignace, D. D., and Gillooly, J. F. (2003). Scaling metabolism from organisms to ecosystems. Nature 423, 63–67. doi: 10.1038/nature01671
Froese, R., and Pauly, D. (2013). FishBase. Available online at: http://www.fishbase.org.
Gillooly, J. F., Brown, J. H., Best, G. W., Savage, V., and Charnov, E. L. (2001). Effects of size and temperature on metabolic rate. Science 293, 2248–2251. doi: 10.1126/science.1061967
Harley, C. D. (2011). Climate change, keystone predation, and biodiversity loss. Science 334, 1124–1127. doi: 10.1126/science.1210199
Heard, M. J., Sax, D. F., and Bruno, J. F. (2012). Dominance of non-native species increases over time in a historically invaded strandline community. Divers. Distrib. 18, 1232–1242. doi: 10.1111/j.1472-4642.2012.00918.x
Helmuth, B., Harley, C. D., Halpin, P. M., O'Donnell, M., Hofmann, G. E., and Blanchette, C. A. (2002). Climate change and latitudinal patterns of intertidal thermal stress. Science 298, 1015–1017. doi: 10.1126/science.1076814
Houvenaghel, G. T. (1978). “Oceanographic conditions in the Galapagos Archipelago and their relationship with life on the islands,” in Upwelling Ecosystems, eds R. Boje and M. Tomczak (Berlin; Heidelberg: Springer), 181–200. Available online at: https://link.springer.com/chapter/10.1007/978-3-642-66985-9_15#citeas
Howells, E. J., Berkelmans, R., van, Willis, B. L., and Bay, L. K. (2013). Historical thermal regimes define limits to coral acclimatization. Ecology 94, 1078–1088. doi: 10.1890/12-1257.1
Huey, R. B., and Kingsolver, J. G. (1989). Evolution of thermal sensitivity of ectotherm performance. Trends Ecol. Evol. 4, 131–135.
Irving, A. D., and Witman, J. D. (2009). Positive effects of damselfish override negative effects of urchins to prevent an algal habitat switch. J. Ecol. 97, 337–347. doi: 10.1111/j.1365-2745.2008.01467.x
Kordas, R. L., Harley, C. D. G., and O'Connor, M. I. (2011). Community ecology in a warming world: the influence of temperature on interspecific interactions in marine systems. J. Exp. Mar. Bio. Ecol. 400, 218–226. doi: 10.1016/j.jembe.2011.02.029
Kratina, P., Greig, H. S., Thompson, P. L., Carvalho-Pereira, T. S., and Shurin, J. B. (2012). Warming modifies trophic cascades and eutrophication in experimental freshwater communities. Ecology 93, 1421–1430. doi: 10.1890/11-1595.1
Largen, M. J. (1967). The influence of water temperature upon the life of the Dog-Whelk\emphThais lapillus (Gastropoda: Prosobranchia). J. Anim. Ecol. 36, 207–214.
Lemoine, N. P., and Burkepile, D. E. (2012). Temperature-induced mismatches between consumption and metabolism reduce consumer fitness. Ecology 93, 2483–2489. doi: 10.1890/12-0375.1
Leonard, G. H., Levine, J. M., Schmidt, P. R., and Bertness, M. D. (1998). Flow-driven variation in intertidal community structure in a Maine estuary. Ecology 79, 1395–1411. doi: 10.2307/176751
Marshall, D. J., and McQuaid, C. D. (2010). Warming reduces metabolic rate in marine snails: adaptation to fluctuating high temperatures challenges the metabolic theory of ecology. Proc. R. Soc. B Biol. Sci. 278, 281–288. doi: 10.1098/rspb.2010.1414
Menge, B. A. (1991). Generalizing from experiments: is predation strong or weak in the New England rocky intertidal? Oecologia 88, 1–8.
Menge, B. A., and Branch, G. (2001). “Rocky intertidal communities,” in Marine Community Ecology, eds M. D. Bertness, M. E. Hay, and S. D. Gaines (Sunderland, MA: Sinauer Associates), 221–251.
Menge, B. A., Sanford, E., Daley, B. A., Freidenburg, T. L., Hudson, G., and Lubchenco, J. (2002). Inter-hemispheric comparison of bottom-up effects on community structure: insights revealed using the comparative-experimental approach. Ecol. Res. 17, 1–16. doi: 10.1046/j.1440-1703.2002.00458.x
Nielsen, K. J. (2003). Nutrient loading and consumers: agents of change in open-coast macrophyte assemblages. Proc. Natl. Acad. Sci. U.S.A. 100, 7660–7665. doi: 10.1073/pnas.0932534100
Nielsen, K. J., and Navarrete, S. A. (2004). Mesoscale regulation comes from the bottom-up: Intertidal interactions between consumers and upwelling. Ecol. Lett. 7, 31–41. doi: 10.1046/j.1461-0248.2003.00542.x
O'Connor, M. I. (2009). Warming strengthens an herbivore–plant interaction. Ecology 90, 388–398. doi: 10.1890/08-0034.1
O'Connor, M. I., Gilbert, B., and Brown, C. J. (2011). Theoretical predictions for how temperature affects the dynamics of interacting herbivores and plants. Am. Nat. 178, 626–638. doi: 10.1086/662171
O'Connor, M. I., Piehler, M. F., Leech, D. M., Anton, A., and Bruno, J. F. (2009). Warming and resource availability shift food web structure and metabolism. PLoS Biol. 7:e1000178. doi: 10.1371/journal.pbio.1000178
Paine, R. T. (1992). Food-web analysis through field measurement of per capita interaction strength. Nature 355, 73–75. doi: 10.1038/355073a0
Pepin, P. (1991). Effect of temperature and size on development, mortality, and survival rates of the pelagic early life history stages of marine fish. Can. J. Fish. Aquat. Sci. 48, 503–518.
Roberts, J. L. (1957). Thermal acclimation of metabolism in the crab, Pachygrapsus crassipes Randall. II. mechanisms and the influence of season and latitude. Physiol. Zool. 30, 242–255.
Sanford, E. (1999). Regulation of keystone predation by small changes in ocean temperature. Science 283, 2095–2097.
Sanford, E. (2002). The feeding, growth, and energetics of two rocky intertidal predators (\emphPisaster ochraceus and \emphNucella canaliculata) under water temperatures simulating episodic upwelling. J. Exp. Mar. Bio. Ecol. 273, 199–218. doi: 10.1016/s0022-0981(02)00164-8
Schaeffer, B. A., Morrison, J. M., Kamykowski, D., Feldman, G. C., Xie, L., Liu, Y., et al. (2008). Phytoplankton biomass distribution and identification of productive habitats within the Galapagos Marine Reserve by MODIS, a surface acquisition system, and in-situ measurements. Remote Sens. Environ. 112, 3044–3054. doi: 10.1016/j.rse.2008.03.005
Stickle, W. B., Moore, M. N., and Bayne, B. L. (1985). Effects of temperature, salinity and aerial exposure on predation and lysosomal stability of the dogwhelk Thais (Nucella) lapillus (L.). J. Exp. Mar. Bio. Ecol. 93, 235–258. doi: 10.1016/0022-0981(85)90242-4
Svensson, F., Karlsson, E., Gårdmark, A., Olsson, J., Adill, A., Zie, J., et al. (2017). In situ warming strengthens trophic cascades in a coastal food web. Oikos 126, 1150–1161. doi: 10.1111/oik.03773
Tomas, F., Abbott, J. M., Steinberg, C., Balk, M., Williams, S. L., and Stachowicz, J. J. (2011). Plant genotype and nitrogen loading influence seagrass productivity, biochemistry, and plant-herbivore interactions. Ecology 92, 1807–1817. doi: 10.1890/10-2095.1
Trussell, G. C., Ewanchuk, P. J., and Bertness, M. D. (2002). Field evidence of trait-mediated indirect interactions in a rocky intertidal food web. Ecol. Lett. 5, 241–245. doi: 10.1046/j.1461-0248.2002.00304.x
Vinueza, L. R., Branch, G. M., Branch, M. L., and Bustamante, A. R. H. (2006). Top-down herbivory and bottom-up El Niño effects on Galápagos rocky-shore ccommunities. Ecol. Monogr. 76, 111–131. doi: 10.1890/04-1957
Vinueza, L. R., Menge, B. A., Ruiz, D., and Palacios, D. M. (2014). Oceanographic and climatic variation drive top-down/bottom-up coupling in the Galápagos intertidal meta-ecosystem. Ecol. Monogr. 84, 411–434. doi: 10.1890/13-0169.1
Wellington, G. M., Strong, A. E., and Merlen, G. (2001). Sea surface temperature variation in the Galápagos Archipelago: a comparison between AVHRR nighttime satellite data and in situ instrumentation (1982-1998). Bull. Mar. Sci. 69, 27–42. Available online at: http://www.ingentaconnect.com/contentone/umrsmas/bullmar/2001/00000069/00000001/art00004
Keywords: grazing, temperature acclimation, metabolic ecology, Galápagos Islands, upwelling ecosystems, macroalgae
Citation: Carr LA, Gittman RK and Bruno JF (2018) Temperature Influences Herbivory and Algal Biomass in the Galápagos Islands. Front. Mar. Sci. 5:279. doi: 10.3389/fmars.2018.00279
Received: 08 February 2018; Accepted: 23 July 2018;
Published: 17 August 2018.
Edited by:
Susana Carvalho, King Abdullah University of Science and Technology, Saudi ArabiaReviewed by:
Deron Burkepile, University of California, Santa Barbara, United StatesDavid Andrew Feary, University of Nottingham, United Kingdom
Copyright © 2018 Carr, Gittman and Bruno. This is an open-access article distributed under the terms of the Creative Commons Attribution License (CC BY). The use, distribution or reproduction in other forums is permitted, provided the original author(s) and the copyright owner(s) are credited and that the original publication in this journal is cited, in accordance with accepted academic practice. No use, distribution or reproduction is permitted which does not comply with these terms.
*Correspondence: John F. Bruno, amJydW5vQHVuYy5lZHU=