- 1Department of Earth Sciences, The University of Hong Kong, Pokfulam, Hong Kong
- 2Swire Institute of Marine Science, The University of Hong Kong, Pokfulam, Hong Kong
Surface waters in the Nordic Seas were colder and fresher throughout the marine isotope stage (MIS) 11 interglacial compared to present day. This has been previously attributed to the continuous delivery of freshwater sourced from large ice structures characteristic of the preceding glacial interval, MIS 12. While it is conventionally believed that high-latitude surface freshening can trigger a reduction of the Atlantic Meridional Overturning Circulation (AMOC), multiple lines of evidence suggest a vigorous AMOC despite elevated freshwater forcing in the Nordic Seas. Here, we review and reanalyze evidence for sea surface properties throughout the Nordic Seas and North Atlantic. We find that surface waters in the Nordic Seas experienced an unusually variable inception of interglacial temperature conditions with multiple high-magnitude cold excursions. While cold events in the North Atlantic were frequently associated with in situ meltwater deposition as reconstructed by ice-rafted debris, this proxy was virtually uncorrelated with cold events in the Nordic Seas. Additionally, stable nitrogen analysis revealed variable levels of nutrient utilization in the Nordic Seas' surface layer throughout MIS 11. This may reflect a dynamic structure of the upper ocean concomitant with an intermittent rate of freshwater delivery. Based on this combination of evidence, we suggest that the colder and fresher surface layer in the Nordic Seas was supplied from higher latitudes, rather than from locally-sourced iceberg meltwater as is characteristic of North Atlantic forcing. Pairing proxy-based evidence with recent numerical simulations further decouples surface freshening in the Nordic Seas and Greenland meltwater input, discrediting Greenland as a source of freshwater to this region during the later phase of MIS 11. Because the origin of freshwater has implications for its rate of delivery, our study might help to explain the active AMOC despite surface freshening during MIS 11 and should be recognized when considering this interglacial as an analog for near-future climate change scenarios.
Introduction
Forecasting future Global changes associated with anthropogenic warming requires a comprehensive understanding of the variables that regulate Earth's climate system. Reconstructing climate variability in the past can help to better characterize impending changes by contextualizing the processes affected by anthropogenic warming. The interglacial period from 424 to 374 ka, marine isotope stage (MIS) 11, has long been considered a powerful analog for modern climate change because of its apparent orbital (Berger and Loutre, 2002; Loutre and Berger, 2003) and atmospheric (Raynaud et al., 2005) similarities to the pre-industrial Holocene. While some records originally suggested comparable internal climate dynamics between MIS 11 and the present interglacial (e.g., Howard, 1997; de Abreu et al., 2005; McManus et al., 2013), later work revealed key differences in cryospheric activity in addition to atmospheric and oceanic circulation patterns (Dickson et al., 2009; Kandiano et al., 2012, 2016; Droxler et al., 2013; Thibodeau et al., 2017a). Here, we review and reanalyze evidence for stage 11's unique sea surface properties with particular attention given to the Nordic Seas, a critical location involved in driving ocean circulation. We investigate ice sheet demise and subsequent freshwater forcing throughout the high-latitude Atlantic Ocean and discuss potential consequences for overturning circulation. Using a combination of published ice-rafted debris (IRD) records and sea surface temperature (SST) reconstructions, we suggest that surface freshening in the Nordic Seas during MIS 11 originated from an external source rather than from in situ meltwater such as is characteristic of North Atlantic forcing. We hypothesize that the observed freshwater was derived from higher latitudes, which may be helpful for informing scenarios of near-future freshening in the region.
The Global temperature optimum of MIS 11 is centered around 405 ka, and occurred late in the stage relative to temperature optima of other quaternary interglacial periods. Most records also indicate that peak interglacial conditions persisted for 25–30 ky, an abnormally long time relative to other quaternary interglacials (McManus et al., 2013; Candy et al., 2014). While evidence from an uninterrupted Lake El'gygytgyn sediment core suggests that stage 11 was characterized by an anomalously warm temperature optimum and uniquely elevated sea levels (Melles et al., 2012), planktic δ18O values suggest it was no warmer than other interglacials during the past 450 ka (McManus et al., 1999; Hodell et al., 2000). The continued uncertainties regarding the climatic structure of MIS 11 highlight problems with using stage 11 for contemporary climate predictions. Additional complications are introduced when comparing the nature of the Atlantic Meridional Overturning Circulation (AMOC) between both periods.
The AMOC broadly describes the movement of waters throughout the Atlantic Ocean. In the North Atlantic and Nordic Seas, waters are cooled intensely by winds, lose buoyancy, and sink to form North Atlantic Deep Water (NADW), which is exported southward. NADW is composed of Labrador Sea Water, formed in the Labrador Sea, and denser overflow water masses such as Denmark Strait Overflow Water and Iceland-Scotland Overflow Water (Buckley and Marshall, 2016). Because the subpolar North Atlantic is a locus of NADW formation, this region is important for facilitating the strength of Global ocean circulation. Drivers of the contemporary AMOC include local wind forcing on the intra- and interannual timescale, and a combination of thermohaline and wind-driven mechanisms on the decadal timescale (Buckley and Marshall, 2016). Thermohaline mechanisms influence AMOC strength via diapycnal mixing, or density-driven heat transfer from the surface to deep water. This occurs as a result of wind and tide action, which produces internal waves. Mixing from these internal waves results in lighter deep ocean waters, which can subsequently rise in low latitudes. On shorter timescales, the AMOC is also influenced via northward movement of waters resulting from westerly circumpolar winds (Kuhlbrodt et al., 2007; Buckley and Marshall, 2016).
Numerical studies utilizing both simple box models and general circulation simulations suggest that NADW formation is sensitive to freshwater forcing at the sea surface. Stommel (1961) developed the first box model to describe the physical properties of overturning circulation and showed that two stable regimes can exist within a theoretical framework of thermohaline flow. This was later validated using a coupled ocean-atmosphere model, which revealed a stable system with and without North Atlantic convection (Manabe and Stouffer, 1988). A direct consequence of Stommel's pioneering work is that there exists a bifurcation point, often referred to as the “Stommel bifurcation point,” whereby sufficient surface freshening may induce hysteresis, destabilize contemporary overturning circulation (Rahmstorf et al., 2005), and result in abrupt changes in Global climate (Delworth et al., 2008). This is increasingly relevant for modern warming scenarios, as multiple modeling studies suggest that the strength of the AMOC may be impacted by meltwater derived from the Greenland Ice Sheet (GIS) (Bakker et al., 2016; Böning et al., 2016; Luo et al., 2016; Yu et al., 2016). While AMOC recovery may be possible under practical melting scenarios (Gregory et al., 2005; Jungclaus et al., 2006; Stouffer et al., 2006), more recent work suggests that these studies may have favored an unrealistically stable AMOC (Liu et al., 2017). A multi-proxy statistical index has illustrated a slowdown of the contemporary AMOC since 1975 (Rahmstorf et al., 2015) coherent with reduced convection in the Labrador Sea (Yang et al., 2016; Thornalley et al., 2018) and SST patterns indicative of a shifting Gulf Stream (Caesar et al., 2018), a major wind-driven surface current in the circulation system. These observations coupled with uncertainties characteristic of modeling studies highlight the need for paleoceanographic investigations of high-latitude freshwater forcing in Earth's geological past to more accurately forecast future changes to ocean circulation.
Throughout the previous glacial cycle, geochemical proxies indicate that the AMOC shut down during Heinrich stadials 11, 2, and 1 coincident with extensive fresh meltwater, but remained active during several other Heinrich events (Böhm et al., 2015). Multiple investigations of AMOC dynamics throughout MIS 11 also suggest that overturning circulation remained strong and active during this time. Paired micropaleontological and stable isotope data suggest that the onset of MIS 11 aligned with strong NADW formation (Vázquez Riveiros et al., 2013; Rodríguez-Tovar et al., 2015). Benthic carbon records throughout the Atlantic Ocean further suggest an abrupt strengthening of the AMOC around 415 ka, which was attributed to obliquity-driven heat redistribution (Dickson et al., 2009). The decay of Global interglacial conditions was also marked by a strengthened leakage of waters from the Indo-Pacific Ocean into the South Atlantic, the “Agulhas leakage,” which was hypothesized to drive another increase in AMOC strength around 400 ka and is consistent with reconstructions of European vegetation patterns (Koutsodendris et al., 2014). Interestingly, strengthened circulation occurred despite heightened freshwater forcing in the Nordic Seas (Kandiano et al., 2016; Thibodeau et al., 2017a), which challenges the conventional belief of meltwater-driven AMOC destabilization.
Various lines of evidence point to the extreme melting of the GIS during MIS 11(Raymo and Mitrovica, 2012; Cronin et al., 2013; Reyes et al., 2014; Hatfield et al., 2016; Schaefer et al., 2016; Robinson et al., 2017), which is commonly invoked as a possible explanation for the cold and fresh surface waters observed in the Nordic Seas relative to the Holocene (Kandiano et al., 2016; Thibodeau et al., 2017a). Evidence for an anomalously cold surface layer is derived from multiple SST records, including the abundance of a polar-indicating foraminiferan, Neogloboquadrina pachyderma sinistral (NPs) (Figure 1; Kandiano et al., 2016; Thibodeau et al., 2017a). Salinity reconstructions derived from the hydrogen composition of alkenones further indicate that these waters were unusually fresh during MIS 11 (Kandiano et al., 2016). The relatively colder sea surface conditions in the Nordic Seas contrast with surface conditions elsewhere throughout the North Atlantic, which have been reconstructed as warmer-than-present (Helmke and Bauch, 2003; Kandiano and Bauch, 2007; Kandiano et al., 2012; Bauch and Erlenkeuser, 2013). This may reflect freshwater release sourced from the extended melting of large ice structures left over from the preceding glacial period, MIS 12 (Kandiano et al., 2016). Such long-term meltwater forcing is also supported by bulk δ15N, a proxy for nutrient utilization and upper-ocean stratification, which suggests a slow thinning of the summer mixed layer relative to MIS 1 (Figure 1; Thibodeau et al., 2017a). These differences in upper-ocean structure reinforce the notion that sea surface conditions in the Nordic Seas during MIS 11 were considerably different from the Holocene.
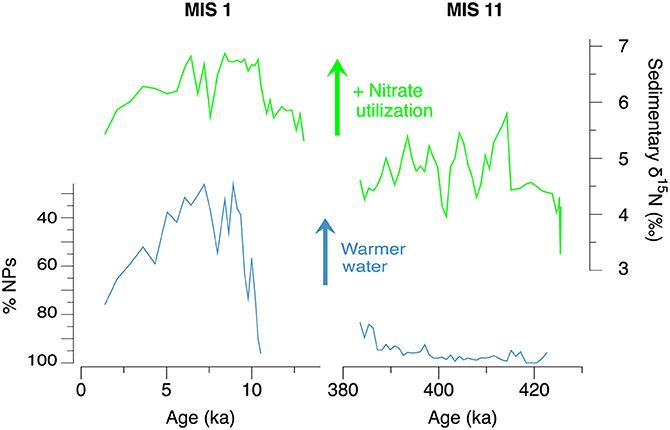
Figure 1. MIS 11 vs. MIS 1 in the Nordic Seas. (Top to bottom) Stable oxygen isotope signature of NPs for MIS 11 (right) and MIS 1 (left) with errors reported as ± 0.08‰. Stable nitrogen signature of host-sediment nitrate for MIS 11 (right) and MIS 1 (left) with errors reported as ± 0.2‰. % NPs as a temperature proxy in MIS 11 (right) and MIS 1 (left). All data were taken from (Thibodeau et al., 2017a).
Further, the Nordic Seas experienced a unique phasing and variability of sea surface properties during interglaciation, including notable high-magnitude cold anomalies (Kandiano et al., 2016) and delayed peak warming conditions relative to more southern locations (Kandiano et al., 2012). Atmospheric circulation has been previously invoked to explain SST patterns throughout the North Atlantic during the development of MIS 11. Kandiano et al. (2012) hypothesized that stage 11 was characterized by a negative North Atlantic Oscillation (NAO) mode, whereby a decreased atmospheric pressure gradient between the Icelandic low and Azores high suppressed the penetration of North Atlantic warm waters into the Nordic Seas and allowed for more extensive seasonal sea ice cover. However, stable nitrogen evidence indicates a thicker and colder surface mixed layer in the Nordic Seas during MIS 11 relative to the Holocene (Thibodeau et al., 2017a), which may be explained by a continuous input of freshwater from ice melt. The relatively thicker mixed layer implies that the dominance of NPs during MIS 11 does not necessitate the absence of North Atlantic waters, which could still feasibly be advected northward at a greater depth. A strong advection of Atlantic waters would be coherent with the intense AMOC supported by benthic δ13C records from Atlantic cores (Vázquez Riveiros et al., 2013).
Nevertheless, it is conceivable that NAO could impact the flow of freshwater from the north, as contemporary observations have shown that negative NAO conditions favor the transport of Arctic freshwater into the Nordic Seas (Morison et al., 2012). Because the source of freshwater would have implications for its rate of delivery into the Nordic Seas, uncertainties surrounding the origin of the fresher sea surface present another challenge with using MIS 11 as an analog for potential near-future freshwater forcing of thermohaline circulation. Below, we consider possible explanations for the source of the colder and fresher sea surface by comparing records from the Nordic Seas to other North Atlantic sites in the context of SST variability and IRD deposition. We find that the freshwater surface layer in the Nordic Seas was likely supplied externally rather than from in situ meltwater input. This has implications for reconsidering the flow of freshwater in the high-latitude North Atlantic, which may enhance our understanding of AMOC dynamics during MIS 11.
Selected Records: Proxies and Positions
In addition to site MD99-2277 in the Nordic Seas, we consider five North Atlantic sites (from higher to lower latitudes, ODP 980, M23414, IODP U1313, MD03-2699, and MD01-2444) for this study (Figure 2). Our analyses are based on independent age models established in previous publications and are not tuned to each other.
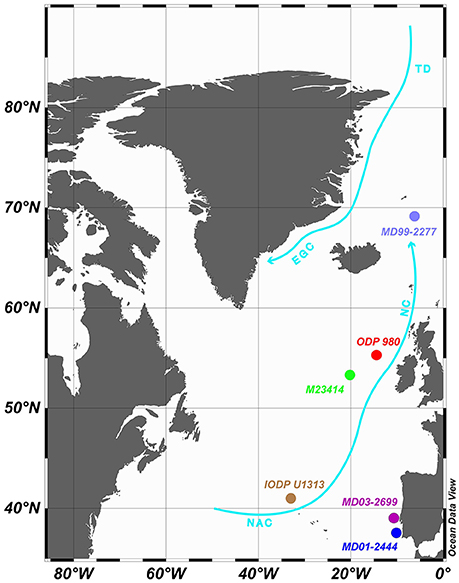
Figure 2. Selected records and relevant currents. North Atlantic Current (NAC), Norwegian Current (NC), East Greenland Current (EGC) and Transpolar Drift Stream (TD) are depicted. This figure was generated using Ocean Data View (Schlitzer, 2017).
Site MD99-2277 (Nordic Seas)
Site MD99-2277 is located at 69° 15′ N, 6° 19′ W in the central Nordic Seas. Here, data from the nearby PS1243 core (69°22′ N, 6°32′ W) are also used to describe conditions at this location. The Nordic Seas are predominately influenced by the Norwegian Current, which carries North Atlantic water northward, and the East Greenland Current, transporting Arctic Water southward. At this site, we consider published proxy data describing freshwater volume, SST (Kandiano et al., 2016), IRD (Helmke et al., 2003; Kandiano et al., 2012), and stable nitrogen evidence of nutrient utilization (Thibodeau et al., 2017a). The chronostratigraphy of MD99-2277 presented here was developed in Kandiano et al. (2012) and the age model for PS1243 was supplied by Bauch et al. (2001).
Salinity was derived from the hydrogen isotopic composition of alkenones retrieved from MD99-2277 (van der Meer et al., 2007; Kandiano et al., 2016). SST was reconstructed from foraminiferal census counts, which record temperature in 0–150 m depth of water (Kandiano et al., 2016). IRD grains larger than 250 μm were picked from PS1243 and nutrient utilization was reconstructed from the δ15N signal of nitrate in PS1243 sediments (Thibodeau et al., 2017a).
Site ODP 980 (High-Latitude North Atlantic)
ODP Site 980 is located at 55° 29′ N, 14° 42′ W, positioned in the Feni Drift of the North Atlantic Ocean. This site is north of the Ruddiman IRD belt, where maximum quaternary ice-rafting occurred (Ruddiman, 1977). Its position is on the northern boundary of the northward flowing North Atlantic Current and the southern boundary of the northward flowing Norwegian Current. Here, we consider SST and IRD. The chronostratigraphy presented with these data was developed in McManus et al. (1999).
SST was reconstructed via benthic and planktic foraminiferal stable oxygen isotopes. The δ18O signal of the benthic foraminiferan Cibicidoides wuellerstorfi was subtracted from NPs-δ18O, which removes the effect of ice volume and allows the result to effectively represent SST (McManus et al., 1999). IRD grains from this core were picked at fractions larger than 150 μm (McManus et al., 1999).
Site M23414 (High-Middle-Latitude North Atlantic)
Site M23414 is located southwest of ODP 980 at 53° 32′ N, 20° 17′ W and is also positioned adjacent to the North Atlantic Current and to the north of the IRD belt. Here, we consider two temperature proxies retrieved from the analog site, GIK23414-8 (54′ N, 20° 29′ W), one recording SST and the other recording mid-depth (0–200 m) temperature. IRD grains are also investigated. The age model presented for this core was taken from Kandiano and Bauch (2007).
While multiple temperature proxies are available at this site, we chose to represent SST by the average of three foraminifera-derived methods (see Kandiano and Bauch, 2007) for two reasons: they are the highest-resolution temperature records available and, because the MD99-2277 SST record also relies on foraminiferal census data, such a record may be more directly comparable to the Nordic Seas. The mid-depth temperature was reconstructed via the relative abundance of isoprenoid glycerol dibiphytanyl glycerol tetraethers (GDGTs) in lipids derived from Thaumarchaeota () (Kandiano et al., 2017). IRD grains were picked at fractions larger than 150 μm (Kandiano and Bauch, 2007).
Site IODP U1313 (Low-Middle-Latitude North Atlantic)
IODP Site U1313 is positioned within the Mid-Atlantic Ridge near the southern boundary of the IRD belt at 41° N, 32° 96′ W. Here, we consider an alkenone-based SST record (the alkenone unsaturation index, ) and an IRD record with grains larger than 315 μm (Stein et al., 2009; Voelker et al., 2010). Ages reported with this core were derived from Stein et al. (2009)
Sites MD03-2699 and MD01-2444 (Low-Latitude North Atlantic)
Sites MD03-2699 (36° 04′ N, 10° 66′ W) and MD01-2444 (37° 34′ N, 10° 09′ W) are both located on the western Iberian margin. SST records for both cores were derived from values (Martrat et al., 2007; Rodrigues et al., 2011). IRD was picked at grains larger than 315 μm at MD03-2699 (Voelker et al., 2010). However, there is no available IRD record at Site MD01-2444. The age model in Voelker et al. (2010) is presented here for MD03-2699, while ages for MD01-2444 were derived from Martrat et al. (2007).
Mechanisms of Climate Change
Orbital Configuration and Atmospheric Greenhouse Gas Composition
Variations in insolation driven by orbital parameters are controlled by the phasing of Earth's orbital eccentricity, operating on a 96,000-year cycle, obliquity of Earth's rotational axis, operating on a 41,000-year cycle, and precession of the Equinoxes, operating on a 23,000-year cycle (Hays et al., 1976). The insolation trend during MIS 11 (Figure 3) was most similar to MIS 1 and MIS 19 compared to other quaternary interglacial periods because of reduced eccentricity forcing (Berger and Loutre, 2002; Loutre and Berger, 2003). This feature in combination with the similar atmospheric CO2 concentrations between stages 11 and the pre-industrial Holocene (Figure 3; Raynaud et al., 2005; Siegenthaler et al., 2005) initially inspired considerations of stage 11 as an analog for Earth's modern climate system.
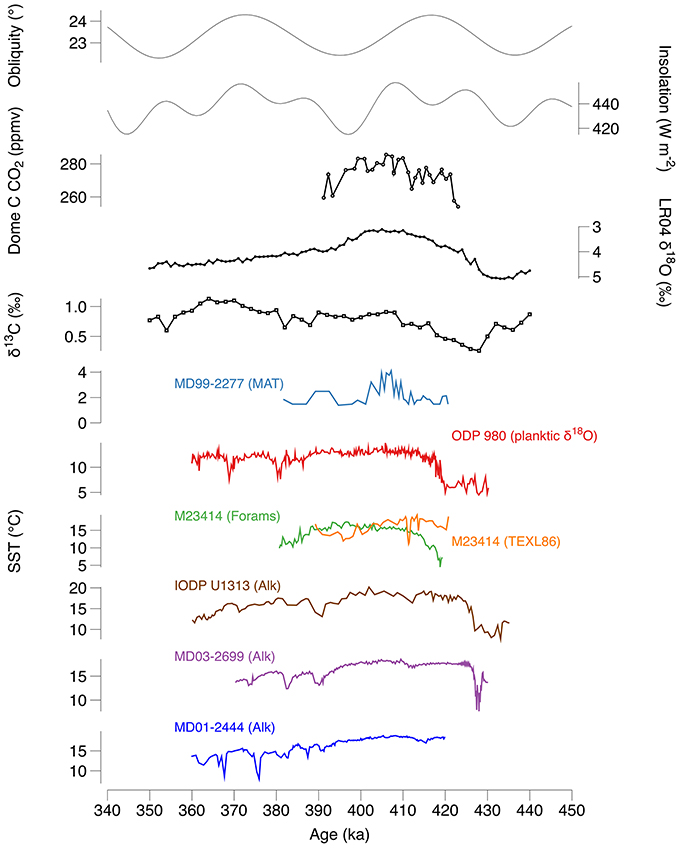
Figure 3. Global signals. (Top to bottom) Orbital configurations: obliquity and mid-July insolation at 65°N (retrieved from pangaea.de; Berger and Loutre, 1991). Atmospheric CO2 concentration derived from the EPICA Dome C ice core record (retrieved from pangaea.de; Siegenthaler et al., 2005). Lisiecki-Raymo (LR04) Global benthic stack of stable oxygen isotopes (retrieved from pangaea.de; Lisiecki and Raymo, 2005). Global stable carbon signal (retrieved from ncdc.noaa.gov; Lisiecki, 2010, 2014). Site MD99-2277 SST data calculated from modern analog technique (retrieved from pangaea.de; Kandiano et al., 2016). Site ODP 980 SST derived from planktic oxygen isotope data (retrieved from ncdc.noaa.gov; McManus et al., 1999). Site M23414 (GIK23414-8) SST data from a combined average of three methods (retrieved from pangaea.de; Kandiano and Bauch, 2007) and 0-200 m -derived temperature (retrieved from pangaea.de; Kandiano et al., 2017). Alkenone-derived SST for sites IODP U1313 (retrieved from pangaea.de; Stein et al., 2009), MD03-2699 (retrieved from pangaea.de; Rodrigues et al., 2011), and MD01-2444 (retrieved from pangaea.de; Martrat et al., 2007).
Ice Volume and AMOC Dynamics
Changes in ice volume, while driven by external forces, also act as a feedback mechanism in the climate system by controlling albedo. Moreover, freshwater forcing of deepwater formation associated with high-latitude ice melt underscores the importance of cryosphere dynamics in the context of Global climate change. Because ice accumulates increased levels of 16O relative to 18O compared to ocean water, ice volume is commonly reconstructed from the stable oxygen signature in benthic foraminifera. The Lisiecki-Raymo stack of globally-distributed benthic oxygen isotope data, “LR04,” is the most comprehensive record of Pleistocene ice volume (Lisiecki and Raymo, 2005), and shows a progressive decline in Global ice cover (lower δ18O) leading up to the inception of MIS 11 (Figure 3).
In addition to oxygen data, stable carbon isotopes in benthic foraminifera can be used to reconstruct water mass properties, where high δ13C values indicate an increased presence of NADW (a strong AMOC) and low values reflect an increase in Antarctic Bottom Water (a weaker AMOC). During MIS 11, a rise in Global benthic δ13C suggests a strong overturning circulation (Figure 3). A cross-latitudinal analysis of stable carbon isotopes suggests an abrupt strengthening of the AMOC approximately 415 ka (Dickson et al., 2009), which is reinforced by empirical orthogonal function analyses of Global sea surface trends (Milker et al., 2013) and may explain the lengthened interglacial temperatures characteristic of MIS 11. This is further supported by subtle SST increases around this time in low-latitude North Atlantic sites IODP U1313 (Stein et al., 2009), MD03-2699 (Rodrigues et al., 2011), and MD01-2444 (Martrat et al., 2007) in addition to the high-latitude North Atlantic site ODP 980 (McManus et al., 1999) and the Nordic Seas record (Kandiano et al., 2016). Taken together, these findings suggest an increase in northward heat transport driven by a stronger circulation (Figure 3).
It is difficult to discern whether this increase also occurs in M23414 as derived from the foraminiferal multi-proxy SST record. The temperature record at this site is further complicated by transient cold excursions recorded in the 0–200 m layer via values (Figure 3). Due to its alignment with a brief decrease in surface salinity and lower ice volume, Kandiano et al. (2017) reasonably attributed the latter cold event to ice melt. However, this trend is not as obviously apparent in the foraminiferal SST record (Figure 4). While the cold excursion may be exaggerated by vertical migration, a separate record at this site also provides evidence of a cold event around this time, though less dramatic than the one reconstructed from values (Kandiano et al., 2017).
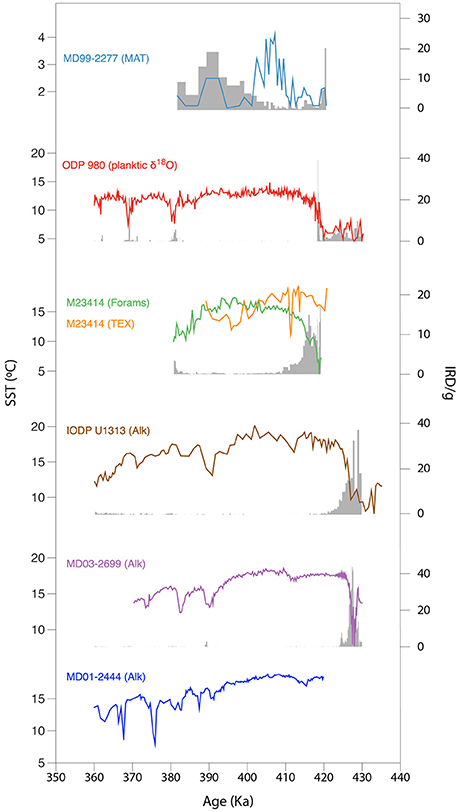
Figure 4. SST and IRD records. (Top to bottom) SST (Kandiano et al., 2016) and IRD >250 μm/g (retrieved from pangaea.de; Helmke et al., 2003; Kandiano et al., 2012) from site MD99-2277. SST and IRD > 150 μm/g from Site ODP 980 (retrieved from ncdc.noaa.gov; McManus et al., 1999). SST and IRD > 150 μm/g from Site GIK23414-8, analog of M23414 (retrieved from pangaea.de; Kandiano and Bauch, 2007). SST (Stein et al., 2009; Rodrigues et al., 2011) and IRD > 315 μm/g from Sites IODP U1313 and MD03-2699 (retrieved from pangaea.de; Voelker et al., 2010). SST from MD01-2444 (Martrat et al., 2007).
The Unique Nordic Seas of MIS 11
Full interglacial SST conditions in the Nordic Seas emerged later relative to all other North Atlantic records (Figure 4). Additionally, SST was more variable during the interglacial inception in the Nordic Seas relative to the North Atlantic, marked by higher-magnitude cold anomalies (Figure 5A). While warm anomalies are to be expected during interglaciation, cold excursions require an additional explanatory mechanism. Cold events in the North Atlantic are highly correlated with IRD (R2 = 0.76 in both M23414 and ODP 980 records), likely reflecting the impact of transient in situ meltwater on SST at these sites (Figures 5B,C). However, this relationship is virtually nonexistent in the Nordic Seas (R2 = 0.03). While the R2-value is identical to two decimal places in both North Atlantic records, methodological differences in SST reconstructions likely make MD99-2277 and M23414 records more directly comparable, rather than comparisons between MD99-2277 and ODP 980 (see section Selected Records: Proxies and Positions).
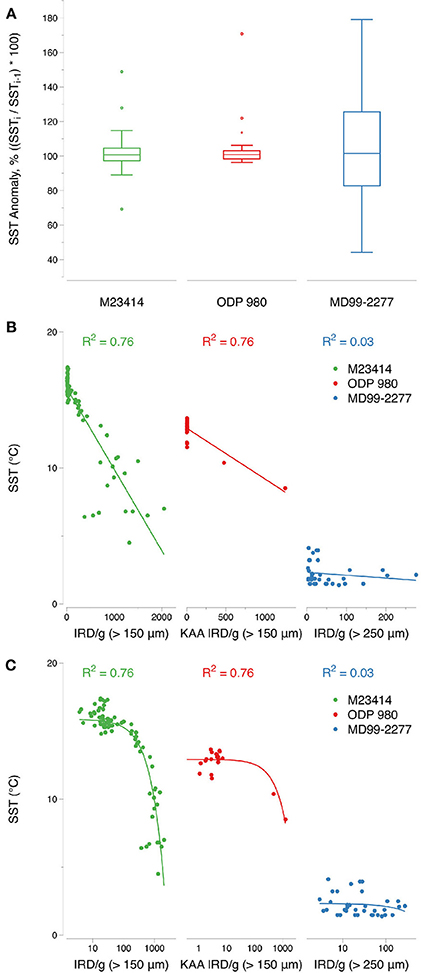
Figure 5. Relationship between SST and IRD (A) SST anomalies expressed as percentage change from previous value from 419 to 390 ka. (B) Relationship between SST (McManus et al., 1999; Kandiano and Bauch, 2007; Kandiano et al., 2016) and IRD (McManus et al., 1999; Helmke et al., 2003; Kandiano and Bauch, 2007; Kandiano et al., 2012) on a linear scale and (C) logarithmic scale. Data from ODP 980 are ka-averaged (“KAA”) to align IRD and SST phasing.
Alkenone-derived hydrogen isotopes suggest a gradual increase in freshwater preceding SST optima in the Nordic Seas and correspond to trivially small IRD values (Figure 6). While the presence of IRD suggests that some minor ice-rafting events must have occurred in the Nordic Seas, such events cannot be realistically invoked as an explanation for the cold and fresh surface conditions reconstructed throughout MIS 11 (Kandiano et al., 2016). Further, because surface waters in the Nordic Seas are always colder than those in the North Atlantic, larger volumes of low-temperature waters would be required to produce the notable cold excursions in the Nordic Seas. This implies that the temperature-forcing mechanisms were different between the Nordic Seas and the North Atlantic during MIS 11. Whereas cold events in the North Atlantic were predominately associated with iceberg discharge, cold events in the Nordic Seas were likely produced by a massive addition of liquid freshwater or sea ice. This is critical to recognize for paleoceanographic reconstructions of MIS 11.
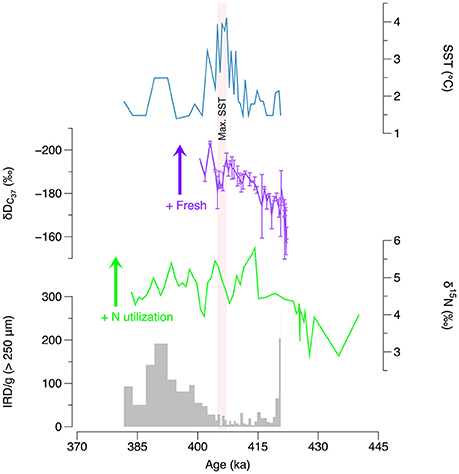
Figure 6. Freshwater input and upper-ocean structure. (Top to bottom) SST, hydrogen composition of C37 alkenones and their standard deviations (Kandiano et al., 2016), stable nitrogen isotope signal of host-sediment nitrate (Thibodeau et al., 2017a, and >250 μm IRD/g (Helmke et al., 2003; Kandiano et al., 2012). Temperature optima are highlighted by the vertical shaded region.
High variability in the bulk δ15N record suggests fluctuating nutrient utilization (Schubert et al., 2001; Ren et al., 2009; Straub et al., 2013; Thibodeau et al., 2017a) characteristic of MIS 11, which may reflect an intermittent rate of freshwater delivery to the Nordic Seas (Figure 6). It has been previously proposed that the prolonged introduction of freshwater to the Nordic Seas during stage 11 resulted in a thicker mixed layer relative to the Holocene, thereby generating lower δ15N values (Figure 1; Thibodeau et al., 2017a). This is caused by an accumulation of buoyant freshwater at the surface that pushes the mixed layer to a greater depth, and is supported by preliminary numerical work using the intermediately complex OSUVic 2.9 climate model (Thibodeau et al., 2017b, 2018). It therefore appears that surface freshening does not always necessitate upper-ocean stratification, and rather that the duration of freshwater forcing is a driving factor when evaluating the impact of surface freshening on the structure of the surface ocean. While bulk isotope data suggest a volatile mixed layer thickness throughout MIS 11, these values may be further complicated by changes in nutrient supply and primary productivity. Accordingly, more robust biogeochemical techniques such as microfossil-bound δ15N (Ren et al., 2009; Straub et al., 2013) are required to unravel nutrient dynamics and upper-ocean structure during this interval.
Freshwater Sources
A natural question arises regarding the origin of the Nordic Seas' fresher surface layer, for which we consider three possibilities: (1) North Atlantic water flowing northward along the North Atlantic Current, (2) meltwater derived from the demise of the GIS, and (3) waters from higher latitudes including both sea ice export from the Arctic and Eurasian runoff.
Maximum quaternary ice rafting occurred in the North Atlantic (Ruddiman, 1977), and waters from the North Atlantic are transported directly to the Nordic Seas via the Norwegian Current. However, North Atlantic surface waters are considerably warmer than those in the Nordic Seas, and so it is unlikely that freshwater derived from the North Atlantic can resolve the high-magnitude cold SST anomalies characteristic of the Nordic Seas during MIS 11 (Figure 5A). SST reconstructed by revised analog method showed that the subpolar North Atlantic was 1.19°C warmer during MIS 11 compared to the present, whereas the Nordic Seas were 1.92°C colder at site MD99-2277 on average (Kandiano et al., 2012). The warmer subpolar North Atlantic during MIS 11 might also reinforce stronger AMOC conditions relative to present day. Because there is no evidence of anomalously cold water in the North Atlantic, this further discredits the region as the origin of the fresh and cold surface layer in the Nordic Seas.
The cold and fresh surface layer of the Nordic Seas during stage 11 may also be attributed to meltwater from the GIS. Extreme melting of the GIS during MIS 11 is supported by terrestrial records that indicate a forested southern Greenland (Willerslev et al., 2007; de Vernal and Hillaire-Marcel, 2008), geochemical and magnetic tracers that suggest minimal southern ice extent (Hatfield et al., 2016), and geomorphological reconstructions that illustrate eustatic sea level changes requiring GIS collapse (Raymo and Mitrovica, 2012; Reyes et al., 2014). Numerical studies also indicate a high sensitivity to warming-induced deglaciation in southern Greenland. However, most of this meltwater would be deposited in the Eirik Drift, which is directly below Greenland's southern tip and distant from the Nordic Seas (Cuffey and Marshall, 2000; Huybrechts, 2002; Tarasov and Peltier, 2003; Lhomme et al., 2005; Reyes et al., 2014). While modeling experiments suggest that early meltwater from the GIS aligns with increased freshwater deposition in the MD99-2277 record (Robinson et al., 2017; Figure 7), this initial simulated meltwater likely predominately reflects the demise of southern Greenland, and could therefore not enter the Nordic Seas (Kandiano et al., 2012).
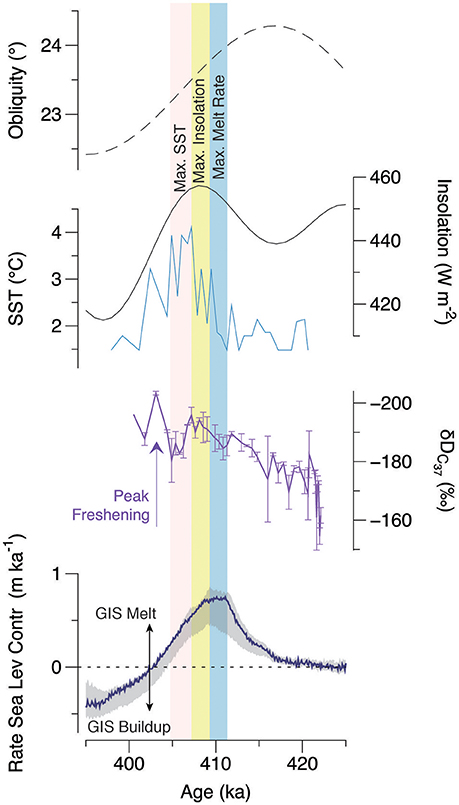
Figure 7. Surface properties of the Nordic Seas and GIS meltwater. (Top to bottom) Insolation and obliquity curves (Berger and Loutre, 1991), SST record from the Nordic Seas (Kandiano et al., 2016), and modeled GIS demise as exemplified by rate of sea level (Robinson et al., 2017).
Landscape reconstructions (Bierman et al., 2014) and numerical simulations (Rachmayani et al., 2017; Robinson et al., 2017) indicate that northern Greenland also experienced considerable melting during MIS 11. This increased melting may have been further enhanced by a stronger AMOC responsible for additional northward heat transport (Rachmayani et al., 2017). The deposition of meltwater masses derived from northern Greenland in the Nordic Seas is conceivable under reconstructed atmospheric circulation patterns (Kandiano et al., 2012), and might help to explain the delayed SST optima relative to the insolation peak (Figure 7). A rapid increase in salinity concomitant with SST optima immediately follows the peak GIS melt rate, which could reflect either a decrease in colder meltwater or an enhanced influence of Atlantic warm waters at the surface. The latter scenario is supported by nutrient utilization and mixed layer dynamics as reconstructed by an increase in bulk δ15N (Figure 6), but more robust evidence is required to understand whether this proxy reflects changes in the mixed layer or other phenomena. Interestingly, maximum freshwater input is observed later in the record and aligns with a regrowth of Greenland's ice (Figure 7), which suggests that an alternative source must be responsible for the late freshening event in the Nordic Seas.
Arctic sea ice is another potential source of the cold freshwater in the Nordic Seas during MIS 11. Because the freezing of ocean water releases salt, waters that freeze also become relatively fresher. Sea ice is exported from the Arctic to the Nordic Seas via the Transpolar Drift Stream, where its melting may hypothetically result in lower temperatures and freshening in the surrounding waters. However, this would require a sufficiently large volume of sea ice export, and micropaleontological evidence indicates a disappearance of perennial Arctic sea ice approximately 400 ka aligning with the mid-Brunhes initiation (Polyak et al., 2013; Cronin et al., 2017). It is conceivable that the mid-Brunhes demise of Arctic sea ice, freshwater release, and subsequent export of meltwater could explain the trends observed in the Nordic Seas. Meltwater derived from higher latitudes might alternatively be sourced from Eurasian runoff coherent with observations of negative-mode NAO conditions during MIS 11 (Kandiano et al., 2012; Morison et al., 2012), although this requires further investigations regarding the volume, phasing, and transportation of waters from the north.
Conclusions
The evidence reviewed here highlights considerable differences in the surface structure of the Nordic Seas between MIS 11 and the Holocene. Because these structural differences may have implications for thermohaline circulation and Global climate, our review suggests exercising caution when making direct comparisons between stage 11 and the current interglacial. Reconciling an active AMOC with continuous and relatively high-volume freshwater forcing in the Nordic Seas remains a challenge in quaternary paleoceanography and may inhibit accurate forecasting of climatic feedbacks associated with GIS demise. Future research is therefore necessary to better assess the location and rate of freshwater input in order to quantify the effect of ice melt on NADW formation and AMOC strength. Our analysis suggests that freshwater in the Nordic Seas during MIS 11 originated from higher latitudes, derived from enhanced Arctic export, elevated Eurasian runoff, and northern Greenland ice melt, rather than from meltwater deposited in situ or from southern Greenland. This should be recognized when considering MIS 11 as an analog for near-future perturbations of Earth's climate system.
Author Contributions
JD prepared this manuscript. JD and BT generated the figures presented here and co-developed the interpretation of this study.
Funding
This work was supported by internal funding via the Department of Earth Sciences at the University of Hong Kong and by the Dr. Stephen S.F. Hui Trust Fund.
Conflict of Interest Statement
The authors declare that the research was conducted in the absence of any commercial or financial relationships that could be construed as a potential conflict of interest.
Acknowledgments
We acknowledge Mandy So for her assistance in formatting Figures 2, 4.
References
Bakker, P., Schmittner, A., Lenaerts, J. T. M., Abe-Ouchi, A., Bi, D., van den Broeke, M. R., et al. (2016). Fate of the Atlantic meridional overturning circulation: strong decline under continued warming and Greenland melting. Geophys. Res. Lett. 43, 12, 252–12,260. doi: 10.1002/2016GL070457
Bauch, H. A., and Erlenkeuser, H. (2013). “Interpreting glacial-interglacial changes in ice volume and climate from subarctic deep water foraminiferal δ18O,” in Geophysical Monograph Series, eds A. W. Droxler, R. Z. Poore and L. H. Burckle (Washington, DC: AGU), 87–102.
Bauch, H. A., Struck, U., and Thiede, J. (2001). “Planktic and benthic foraminifera as indicators for past ocean changes in surface and deep waters of the Nordic Seas,” in The Northern North Atlantic: A Changing Environment, eds P. Schäfer, W. Ritzrau, M. Schlüter, J. Thiede (Berlin: Springer), 411–421.
Berger, A., and Loutre, M. F. (1991). Insolation values for the climate of the last 10 million years. Quat. Sci. Rev. 10, 297–317. doi: 10.1016/0277-3791(91)90033-Q
Berger, A., and Loutre, M. F. (2002). An exceptionally long interglacial ahead? Science 297, 1287–1288. doi: 10.1126/science.1076120
Bierman, P. R., Corbett, L. B., Graly, J. A., Neumann, T. A., Lini, A., Crosby, B. T., et al. (2014). Preservation of a preglacial landscape under the center of the greenland ice sheet. Science 344, 402–405. doi: 10.1126/science.1249047
Böhm, E., Lippold, J., Gutjahr, M., Frank, M., Blaser, P., Antz, B., et al. (2015). Strong and deep Atlantic meridional overturning circulation during the last glacial cycle. Nature 517, 73–76. doi: 10.1038/nature14059
Böning, C. W., Behrens, E., Biastoch, A., Getzlaff, K., and Bamber, J. L. (2016). Emerging impact of Greenland meltwater on deepwater formation in the North Atlantic Ocean. Nat. Geosci. 9, 523–527. doi: 10.1038/ngeo2740
Buckley, M. W., and Marshall, J. (2016). Observations, inferences, and mechanisms of the Atlantic meridional overturning circulation: a review. Rev. Geophys. 54, 5–63. doi: 10.1002/2015RG000493
Caesar, L., Rahmstorf, S., Robinson, A., Feulner, G., and Saba, V. (2018). Observed fingerprint of a weakening Atlantic Ocean overturning circulation. Nature 556, 191–196. doi: 10.1038/s41586-018-0006-5
Candy, I., Schreve, D. C., Sherriff, J., and Tye, G. J. (2014). Marine isotope stage 11: palaeoclimates, palaeoenvironments and its role as an analogue for the current interglacial. Earth Sci. Rev. 128, 18–51. doi: 10.1016/j.earscirev.2013.09.006
Cronin, T. M., Dwyer, G. S., Caverly, E. K., Farmer, J., DeNinno, L. H., Rodriguez-Lazaro, J., et al. (2017). Enhanced Arctic amplification began at the mid-brunhes event ~400,000 years ago. Sci. Rep. 7:14475. doi: 10.1038/s41598-017-13821-2
Cronin, T. M., Polyak, L., Reed, D., Kandiano, E. S., Marzen, R. E., and Council, E. A. (2013). A 600-ka Arctic sea-ice record from Mendeleev Ridge based on ostracodes. Quat. Sci. Rev. 79, 157–167. doi: 10.1016/j.quascirev.2012.12.010
Cuffey, K. M., and Marshall, S. J. (2000). Substantial contribution to sea-level rise during the last interglacial from the Greenland ice sheet. Nature 404, 591–594. doi: 10.1038/35007053
de Abreu, L., Abrantes, F. F., Shackleton, N. J., Tzedakis, P. C., McManus, J. F., Oppo, D. W., et al. (2005). Ocean climate variability in the eastern North Atlantic during interglacial marine isotope stage 11: a partial analogue to the Holocene? Paleoceanography 20, 1–15. doi: 10.1029/2004PA001091
Delworth, T. L., Clark, P. U., Johns, W. E., Lynch-Stieglitz, J., Morrill, C., Seager, R., et al. (2008). Chapter 4, Potential for Abrupt Change in the Atlantic Meridional Overturning Circulation. Final Report, Abrupt Climate Change. Available online at: https://www.gfdl.noaa.gov/bibliography/related_files/td0802.pdf (Accessed March 12, 2017).
de Vernal, A., and Hillaire-Marcel, C. (2008). Natural variability of Greenland climate, vegetation, and ice volume during the past million years. Science 320, 1622–1625. doi: 10.1126/science.1153929
Dickson, A. J., Beer, C. J., Dempsey, C., Maslin, M. A., Bendle, J. A., McClymont, E. L., et al. (2009). Oceanic forcing of the marine isotope stage 11 interglacial. Nat. Geosci. 2, 428–433. doi: 10.1038/ngeo527
Droxler, A. W., Alley, R. B., Howard, W. R., Poore, R. Z., and Burckle, L. H. (2013). “Unique and exceptionally long interglacial marine isotope stage 11: Window into earth warm future climate,” in Geophysical Monograph Series, eds A. W. Droxler, R. Z. Poore and L. H. (Washington, DC: AGU) 1–14.
Gregory, J. M., Dixon, K. W., Stouffer, R. J., Weaver, A. J., Driesschaert, E., Eby, M., et al. (2005). A model intercomparison of changes in the Atlantic thermohaline circulation in response to increasing atmospheric CO2 concentration. Geophys. Res. Lett. 32, 1–5. doi: 10.1029/2005GL023209
Hatfield, R. G., Reyes, A. V., Stoner, J. S., Carlson, A. E., Beard, B. L., Winsor, K., et al. (2016). Interglacial responses of the southern Greenland ice sheet over the last 430,000 years determined using particle-size specific magnetic and isotopic tracers. Earth Planet. Sci. Lett. 454, 225–236. doi: 10.1016/j.epsl.2016.09.014
Hays, J. D., Imbrie, J., and Shackleton, N. J. (1976). Variations in the Earth's orbit: pacemaker of the ice ages. Science 194, 1121–1132. doi: 10.1126/science.194.4270.1121
Helmke, J. P., and Bauch, H. A. (2003). Comparison of glacial and interglacial conditions between the polar and subpolar North Atlantic region over the last five climatic cycles. Paleoceanography 18. doi: 10.1029/2002PA000794
Helmke, J. P., Bauch, H. A., and Mazaud, A. (2003). Evidence for a mid-Pleistocene shift of ice-drift pattern in the Nordic seas. J. Quat. Sci. 18, 183–191. doi: 10.1002/jqs.735
Hodell, D. A., Charles, C. D., and Ninnemann, U. S. (2000). Comparison of interglacial stages in the South Atlantic sector of the southern ocean for the past 450 kyr: implications for marine isotope stage (MIS) 11. Glob. Planet. Change 24, 7–26. doi: 10.1016/S0921-8181(99)00069-7
Huybrechts, P. (2002). Sea-level changes at the LGM from ice-dynamic reconstructions of the Greenland and Antarctic ice sheets during the glacial cycles. Quat. Sci. Rev. 21, 203–231. doi: 10.1016/S0277-3791(01)00082-8
Jungclaus, J. H., Haak, H., Esch, M., Roeckner, E., and Marotzke, J. (2006). Will Greenland melting halt the thermohaline circulation? Geophys. Res. Lett. 33. doi: 10.1029/2006GL026815
Kandiano, E. S., and Bauch, H. A. (2007). Phase relationship and surface water mass change in the northeast Atlantic during marine isotope stage 11 (MIS 11). Quat. Res. 68, 445–455. doi: 10.1016/j.yqres.2007.07.009
Kandiano, E. S., Bauch, H. A., Fahl, K., Helmke, J. P., Röhl, U., Pérez-Folgado, M., et al. (2012). The meridional temperature gradient in the eastern North Atlantic during MIS 11 and its link to the ocean-atmosphere system. Palaeogeogr. Palaeoclimatol. Palaeoecol. 333–334, 24–39. doi: 10.1016/j.palaeo.2012.03.005
Kandiano, E. S., van der Meer, M. T. J., Bauch, H. A., Helmke, J., Damsté, J. S. S., and Schouten, S. (2016). A cold and fresh ocean surface in the Nordic Seas during MIS 11: Significance for the future ocean. Geophys. Res. Lett. 43, 10, 929–10,937. doi: 10.1002/2016GL070294
Kandiano, E. S., van der Meer, M. T., Schouten, S., Fahl, K., Sinninghe Damsté, J. S., and Bauch, H. A. (2017). Response of the North Atlantic surface and intermediate ocean structure to climate warming of MIS 11. Sci. Rep. 7:46192. doi: 10.1038/srep46192
Koutsodendris, A., Pross, J., and Zahn, R. (2014). Exceptional Agulhas leakage prolonged interglacial warmth during MIS 11c in Europe. Paleoceanography 29, 1062–1071. doi: 10.1002/2014PA002665
Kuhlbrodt, T., Griesel, A., Montoya, M., Levermann, A., Hofmann, M., and Rahmstorf, S. (2007). On the driving processes of the Atlantic meridional overturning circulation. Rev. Geophys. 45:RG2001. doi: 10.1029/2004RG000166
Lhomme, N., Clarke, G. K. C., and Marshall, S. J. (2005). Tracer transport in the Greenland Ice Sheet: Constraints on ice cores and glacial history. Quat. Sci. Rev. 24, 173–194. doi: 10.1016/j.quascirev.2004.08.020
Lisiecki, L. E. (2010). A benthic δ13C-based proxy for atmospheric pCO2 over the last 1.5 Myr. Geophys. Res. Lett. 37, 1–5. doi: 10.1029/2010GL045109
Lisiecki, L. E. (2014). Atlantic overturning responses to obliquity and precession over the last 3 Myr. Paleoceanography 29, 71–86. doi: 10.1002/2013PA002505
Lisiecki, L. E., and Raymo, M. E. (2005). A Pliocene-Pleistocene stack of 57 globally distributed benthic δ18O records. Paleoceanography 20. doi: 10.1029/2004PA001071
Liu, W., Xie, S. P., Liu, Z., and Zhu, J. (2017). Overlooked possibility of a collapsed Atlantic Meridional overturning circulation in warming climate. Sci. Adv. 3:e1601666. doi: 10.1126/sciadv.1601666
Loutre, M. F., and Berger, A. (2003). Marine isotope stage 11 as an analogue for the present interglacial. Global Planet. Change 36, 209–217. doi: 10.1016/S0921-8181(02)00186-8
Luo, H., Castelao, R. M., Rennermalm, A. K., Tedesco, M., Bracco, A., Yager, P. L., et al. (2016). Oceanic transport of surface meltwater from the southern Greenland ice sheet. Nat. Geosci. 9, 528–532. doi: 10.1038/ngeo2708
Manabe, S., and Stouffer, R. J. (1988). Two stable equilibria of a coupled ocean-atmosphere model. J. Clim. 1, 841–866.
Martrat, B., Grimalt, J. O., Shackleton, N. J., de Abreu, L., Hutterli, M. A., and Stocker, T. F. (2007). Four climate cycles of recurring deep and surface water destabilizations on the Iberian margin. Science 317, 502–507. doi: 10.1126/science.1139994
McManus, J. F., Oppo, D. W., and Cullen, J. L. (1999). A 0.5-million-year record of millennial-scale climate variability in the North Atlantic. Science 283, 971–975. doi: 10.1126/science.283.5404.971
McManus, J., Oppo, D., Cullen, J., and Healey, S. (2013). “Marine isotope stage 11 (MIS 11): analog for holocene and future climate?” in Geophysical Monograph Series. (Washington, DC: AGU) 69–85.
Melles, M., Brigham-Grette, J., Minyuk, P. S., Nowaczyk, N. R., Wennrich, V., DeConto, R. M., et al. (2012). 2.8 million years of Arctic climate change from Lake El'gygytgyn, NE Russia. Science 337, 315–320. doi: 10.1126/science.1222135
Milker, Y., Rachmayani, R., Weinkauf, M. F. G., Prange, M., Raitzsch, M., Schulz, M., et al. (2013). Global and regional sea surface temperature trends during Marine Isotope Stage 11. Clim. Past 9, 2231–2252. doi: 10.5194/cp-9-2231-2013
Morison, J., Kwok, R., Peralta-Ferriz, C., Alkire, M., Rigor, I., Andersen, R., et al. (2012). Changing Arctic Ocean freshwater pathways. Nature 481, 66–70. doi: 10.1038/nature10705
Polyak, L., Best, K. M., Crawford, K. A., Council, E. A., and St-Onge, G. (2013). Quaternary history of sea ice in the western Arctic Ocean based on foraminifera. Quat. Sci. Rev. 79, 145–156. doi: 10.1016/j.quascirev.2012.12.018
Rachmayani, R., Prange, M., Lunt, D. J., Stone, E. J., and Schulz, M. (2017). Sensitivity of the Greenland Ice Sheet to interglacial climate forcing: MIS 5e versus MIS 11. Paleoceanography 32, 1089–1101. doi: 10.1002/2017PA003149
Rahmstorf, S., Box, J. E., Feulner, G., Mann, M. E., Robinson, A., Rutherford, S., et al. (2015). Exceptional twentieth-century slowdown in Atlantic Ocean overturning circulation. Nat. Clim. Change 5, 475–480. doi: 10.1038/nclimate2554
Rahmstorf, S., Crucifix, M., Ganopolski, A., Goosse, H., Kamenkovich, I., Knutti, R., et al. (2005). Thermohaline circulation hysteresis: a model intercomparison. Geophys. Res. Lett. 32, 1–5. doi: 10.1029/2005GL023655
Raymo, M. E., and Mitrovica, J. X. (2012). Collapse of polar ice sheets during the stage 11 interglacial. Nature 483, 453–456. doi: 10.1038/nature10891
Raynaud, D., Barnola, J. M., Souchez, R., Lorrain, R., Petit, J. R., Duval, P., et al. (2005). Palaeoclimatology: the record for marine isotopic stage 11. Nature 436, 39–40. doi: 10.1038/43639b
Ren, H., Sigman, D. M., Meckler, A. N., Plessen, B., Robinson, R. S., Rosenthal, Y., et al. (2009). Foraminiferal isotope evidence of reduced nitrogen fixation in the ice age Atlantic ocean. Science 323, 244–248. doi: 10.1126/science.1165787
Reyes, A. V., Carlson, A. E., Beard, B. L., Hatfield, R. G., Stoner, J. S., Winsor, K., et al. (2014). South Greenland ice-sheet collapse during Marine Isotope Stage 11. Nature 510, 525–528. doi: 10.1038/nature13456
Robinson, A., Alvarez-Solas, J., Calov, R., Ganopolski, A., and Montoya, M. (2017). MIS-11 duration key to disappearance of the Greenland ice sheet. Nat. Commun. 8:16008. doi: 10.1038/ncomms16008
Rodrigues, T., Voelker, A. H. L., Grimalt, J. O., Abrantes, F., and Naughton, F. (2011). Iberian Margin sea surface temperature during MIS 15 to 9 (580-300 ka): glacial suborbital variability versus interglacial stability. Paleoceanography 26. doi: 10.1029/2010PA001927
Rodríguez-Tovar, F. J., Dorador, J., Martin-Garcia, G. M., Sierro, F. J., Flores, J. A., and Hodell, D. A. (2015). Response of macrobenthic and foraminifer communities to changes in deep-sea environmental conditions from marine isotope stage (MIS) 12 to 11 at the “Shackleton Site.” Glob. Planet. Change 133, 176–187. doi: 10.1016/j.gloplacha.2015.08.012
Ruddiman, W. F. (1977). Late quaternary deposition of ice-rafted sand in the subpolar North Atlantic (lat 40° to 65°N). Bull. Geol. Soc. Am. 88, 1813–1827.
Schaefer, J. M., Finkel, R. C., Balco, G., Alley, R. B., Caffee, M. W., Briner, J. P., et al. (2016). Greenland was nearly ice-free for extended periods during the Pleistocene. Nature 540, 252–255. doi: 10.1038/nature20146
Schubert, C. J., Stein, R., and Calvert, S. E. (2001). Tracking nutrient and productivity variations over the last deglaciation in the Arctic Ocean. Paleoceanography 16, 199–211. doi: 10.1029/2000PA000503
Siegenthaler, U., Stocker, T. F., Monnin, E., Lüthi, D., Schwander, J., Stauffer, B., et al. (2005). Stable carbon cycle–climate relationship during the late Pleistocene. Science 310, 1313–1317. doi: 10.1126/science.1120130
Stein, R., Hefter, J., Grützner, J., Voelker, A., and David A Naafs, B. (2009). Variability of surface water characteristics and Heinrich-like events in the Pleistocene midlatitude North Atlantic Ocean: Biomarker and XRD records from IODP Site U1313 (MIS 16-9). Paleoceanography 24. doi: 10.1029/2008PA001639
Stommel, H. (1961). Thermohaline convection with two stable regimes of flow. Tellus 13, 224–230. doi: 10.3402/tellusa.v13i2.9491
Stouffer, R. J., Yin, J., Gregory, J. M. J. M., Dixon, K. W., Spelman, M. J., Hurlin, W., et al. (2006). Investigating the causes of the response of the thermohaline circulation to past and future climate changes. J. Clim. 19, 1365–1387. doi: 10.1175/JCLI3689.1
Straub, M., Tremblay, M. M., Sigman, D. M., Studer, A. S., Ren, H., Toggweiler, J. R., et al. (2013). Nutrient conditions in the subpolar North Atlantic during the last glacial period reconstructed from foraminifera-bound nitrogen isotopes. Paleoceanography 28, 79–90. doi: 10.1002/palo.20013
Tarasov, L., and Peltier, W. R. (2003). Greenland glacial history, borehole constraints, and Eemian extent. J. Geophys. Res. Solid Earth 108. doi: 10.1029/2001JB001731
Thibodeau, B., Bauch, H. A., Paytan, A., Pedersen, T. F., and Schmittner, A. (2018). Variability in the Nordic Sea stratification during MIS 11: any relevance to AMOC? Ocean Sci. Meet. doi: 10.13140/RG.2.2.34527.15522
Thibodeau, B., Bauch, H. A., and Pedersen, T. F. (2017a). Stratification-induced variations in nutrient utilization in the Polar North Atlantic during past interglacials. Earth Planet. Sci. Lett. 457, 127–135. doi: 10.1016/j.epsl.2016.09.060
Thibodeau, B., Bauch, H., Pedersen, T. F., and Schmittner, A. (2017b). “The sensitivity of Nordic Seas upper-ocean stratification to freshwater input,” in Goldschmidt Conference, (Paris), 13–18 August 2017. In Goldschmidt Abstracts 2017, abstract no. 3921.
Thornalley, D. J. R., Oppo, D. W., Ortega, P., Robson, J. I., Brierley, C. M., Davis, R., et al. (2018). Anomalously weak Labrador Sea convection and Atlantic overturning during the past 150 years. Nature 556, 227–230. doi: 10.1038/s41586-018-0007-4
van der Meer, M. T. J., Baas, M., Rijpstra, W. I. C., Marino, G., Rohling, E. J., Sinninghe Damsté, J. S., et al. (2007). Hydrogen isotopic compositions of long-chain alkenones record freshwater flooding of the Eastern Mediterranean at the onset of sapropel deposition. Earth Planet. Sci. Lett. 262, 594–600. doi: 10.1016/j.epsl.2007.08.014
Vázquez Riveiros, N., Waelbroeck, C., Skinner, L., Duplessy, J.-C., McManus, J. F., Kandiano, E. S., et al. (2013). The “MIS 11 paradox” and ocean circulation: role of millennial scale events. Earth Planet. Sci. Lett. 371–372, 258–268. doi: 10.1016/j.epsl.2013.03.036
Voelker, A. H. L., Rodrigues, T., Billups, K., Oppo, D., McManus, J., Stein, R., et al. (2010). Variations in mid-latitude North Atlantic surface water properties during the mid-Brunhes (MIS 9-14) and their implications for the thermohaline circulation. Clim. Past 6, 531–552. doi: 10.5194/cp-6-531-2010
Willerslev, E., Cappellini, E., Boomsma, W., Nielsen, R., Hebsgaard, M. B., Brand, T. B., et al. (2007). Ancient biomolecules from deep ice cores reveal a forested southern Greenland. Science 317, 111–114. doi: 10.1126/science.1141758
Yang, Q., Dixon, T. H., Myers, P. G., Bonin, J., Chambers, D., and Van Den Broeke, M. R. (2016). Recent increases in Arctic freshwater flux affects Labrador Sea convection and Atlantic overturning circulation. Nat. Commun. 7:10525. doi: 10.1038/ncomms10525
Keywords: MIS 11, AMOC, North Atlantic, Surface waters, Global change, Paleoceaongraphy
Citation: Doherty JM and Thibodeau B (2018) Cold Water in a Warm World: Investigating the Origin of the Nordic Seas' Unique Surface Properties During MIS 11. Front. Mar. Sci. 5:251. doi: 10.3389/fmars.2018.00251
Received: 20 February 2018; Accepted: 29 June 2018;
Published: 02 August 2018.
Edited by:
Juliane Müller, Alfred Wegener Institut Helmholtz Zentrum für Polar und Meeresforschung, Helmholtz-Gemeinschaft Deutscher Forschungszentren (HZ), GermanyReviewed by:
Evgenia Kandiano, GEOMAR Helmholtz-Zentrum für Ozeanforschung Kiel, Helmholtz-Gemeinschaft Deutscher Forschungszentren (HZ), GermanyMatthias Prange, Universität Bremen, Germany
Haojia Ren, National Taiwan University, Taiwan
Copyright © 2018 Doherty and Thibodeau. This is an open-access article distributed under the terms of the Creative Commons Attribution License (CC BY). The use, distribution or reproduction in other forums is permitted, provided the original author(s) and the copyright owner(s) are credited and that the original publication in this journal is cited, in accordance with accepted academic practice. No use, distribution or reproduction is permitted which does not comply with these terms.
*Correspondence: Benoit Thibodeau, YnRoaWJAaGt1Lmhr