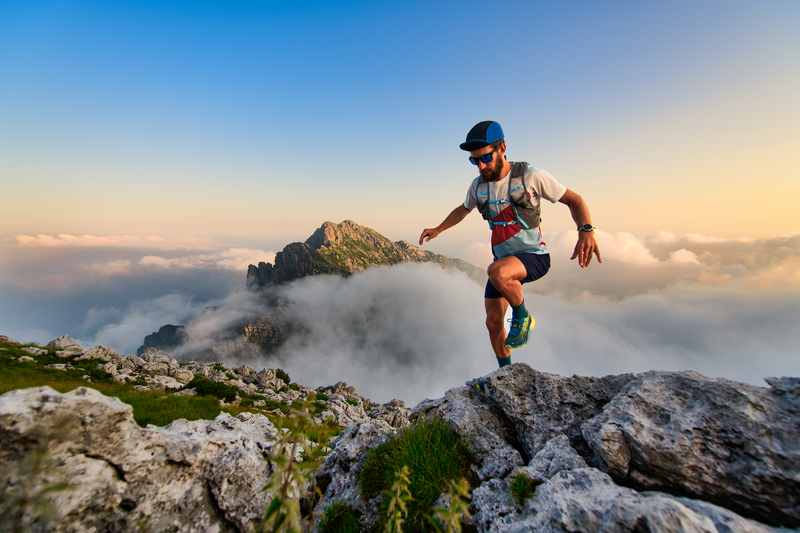
95% of researchers rate our articles as excellent or good
Learn more about the work of our research integrity team to safeguard the quality of each article we publish.
Find out more
ORIGINAL RESEARCH article
Front. Mar. Sci. , 25 July 2018
Sec. Marine Ecosystem Ecology
Volume 5 - 2018 | https://doi.org/10.3389/fmars.2018.00241
This article is part of the Research Topic Mixotrophy in Protists: from Model Systems to Mathematical Models View all 12 articles
Cryptophyte algae are globally distributed photosynthetic flagellates found in freshwater, estuarine, and neritic ecosystems. While cryptophytes can be highly abundant and are consumed by a wide variety of protistan predators, few studies have sought to quantify in situ grazing rates on their populations. Here we show that autumnal grazing rates on in situ communities of cryptophyte algae in Chesapeake Bay are high throughout the system, while growth rates, particularly in the lower bay, were low. Analysis of the genetic diversity of cryptophyte populations within dilution experiments suggests that microzooplankton may be selectively grazing the fastest-growing members of the population, which were generally Teleaulax spp. We also demonstrate that potential grazing rates of ciliates and dinoflagellates on fluorescently labeled (FL) Rhodomonas salina, Storeatula major, and Teleaulax amphioxeia can be high (up to 149 prey predator−1 d−1), and that a Gyrodinium sp. and Mesodinium rubrum could be selective grazers. Potential grazing was highest for heterotrophic dinoflagellates, but due to its abundance, M. rubrum also had a high overall impact. This study reveals that cryptophyte algae in Chesapeake Bay can experience extremely high grazing pressure from phagotrophic protists, and that this grazing likely shapes their community diversity.
Cryptophyte algae are predominantly a photosynthetic lineage of flagellated protists in aquatic ecosystems (Mallin et al., 1991; Gervais, 1997; Marshall et al., 2005), capable of thriving in turbid and low light environments due to their highly efficient green light harvesting phycobiliproteins (Spear-Bernstein and Miller, 1989). Several species have been shown to use dissolved organic carbon (DOC) to supplement their growth requirements (Lewitus et al., 1991; Lewitus and Kana, 1995; Gervais, 1997), while others, particularly in freshwater and polar habitats, ingest bacterial prey (Marshall and Laybourn-Parry, 2002; Yoo et al., 2017). Collectively, these traits allow cryptophytes to thrive in diverse environmental conditions.
Cryptophyte algae are either preferred or optimal prey for numerous protist and mesozooplankton grazers. Most non-constitutive mixotrophic (i.e., acquired phototrophic or kleptoplastidic) dinoflagellates selectively graze on cryptophytes for their plastids, in both marine (Larsen, 1988; Skovgaard, 1998) and freshwater (Fields and Rhodes, 1991; Onuma and Horiguchi, 2016) environments. Many constitutively mixotrophic (i.e., phagotrophic phototrophs) and heterotrophic dinoflagellates have also been shown to selectively graze cryptophyte algae (Li et al., 1999; Jeong et al., 2007; Johnson, 2015). Numerous studies have also demonstrated that heterotrophic and mixotrophic ciliates are important grazers of cryptophyte algae (Stoecker and Silver, 1990; Jakobsen and Hansen, 1997; Weisse and Kirchhoff, 1997). Several studies have also shown that certain mesozooplankton grazers may also selectively graze cryptophyte algae (Liu et al., 2010; Tõnno et al., 2016).
In Chesapeake Bay the cryptophyte pigment alloxanthin peaks within the southern Bay during autumn, but is present throughout the Bay year-round (Adolf et al., 2006). High concentrations of cryptophyte algae are commonly found in many of the tidal regions of Chesapeake Bay tributaries (Marshall et al., 2005), and are sometimes associated with blooms of the organelle stealing ciliate Mesodinium rubrum (Johnson et al., 2013) or the mixotrophic dinoflagellate Karlodinium veneficum (Li et al., 2000; Adolf et al., 2008). While cryptophytes are known to be ecologically important in estuarine ecosystems, few studies have directly measured their in situ growth rates or grazing pressure on their populations. Here we provide estimates of growth and grazing rates of in situ cryptophyte communities, the effects of grazing on community diversity, as well as potential loss rates on fluorescently labeled cryptophyte prey added to natural samples.
All research occurred within the main stem of Chesapeake Bay and three of its tributaries (Table 1, Figure 1). In Chesapeake Bay and the Potomac River, all sampling was conducted during October 2011 on the R/V Sharp. Otherwise tributary sampling was conducted from small boats and occurred in the Choptank River in April 2012 and in the Pocomoke River during May, June, and October 2001–2002 (Table 1). Station sampling within the main stem of Chesapeake Bay and the Potomac River was conducted during a cruise that surveyed much of the Bay system, and is part of a series of long-term monitoring locations visited in previous studies (Johnson et al., 2003).
Figure 1. Map of Chesapeake Bay Ecosystem with approximate location of all sampling stations. Stations where dilution experiments were performed are marked with a red circle and experiments where fluorescently labeled cryptophytes were used are marked with a green circle. Map edited from original source: Saxby, T. and Boicourt, K., Integration and Application Network, University of Maryland Center for Environmental Science (ian.umces.edu/imagelibrary/).
Water was collected from Chesapeake Bay and Potomac River stations at 1 m depth from R/V Sharp using Niskin bottles attached to a rosette equipped with a CTD probe (Seabird 911 plus, conductivity, temperature and density). Water was kept in carboys within a flow-through incubator until used for “on-deck” experiments. From the Choptank River, water was obtained from 1 m depth, using a small boat and a single Niskin bottle affixed to a CTD probe cage. Surface water was collected from the Pocomoke River using small boats and a bucket. Water collected using small boats was stored in carboys on deck or in bottles in coolers until returned to the lab for experiments.
Dilution experiments were used to measure in situ cryptophyte community growth (μ) and mortality (g) rates due to grazing by microzooplankton. Pre-screened (<200 μm) whole seawater (WSW), containing phytoplankton and microzooplankton, was prepared by gently passing water through a 200 μm mesh filter and particle free filtered seawater (FSW) was prepared by filtering water through Pall 0.2 μm vented sterile filter capsule. A three-point dilution method was used to create 100, 20, and 5% WSW, diluted with FSW, and all treatments were measured in triplicate bottles (Landry, 1993). All dilution bottles were incubated on deck at in situ surface temperature and at ~50% surface irradiance. Sampling for enumeration of cryptophytes and assessment of cryptophyte diversity was conducted at time 0 and 24 h. Cryptophyte populations from dilution experiments were counted using a BD Accuri C6 flow cytometer equipped with a 488 nm laser. Cryptophyte cells were differentiated based on their autofluorescence properties using bivariate scatter plots of orange (585/40 nm emission filter) for high phycoerythrin and red fluorescence (>670 nm emission filter) for chlorophyll against side scatter. Cultures of the cryptophytes Teleaulax amphioxeia (strain GCEP01), Storeatula major (strain SM), and Rhodomonas salina (strain Q) were used as standards for establishing “ballpark” settings for assessing field populations. However, the acquisition gates used on the flow cytometer did not exactly correspond to these species. The T. amphioxeia culture was almost exclusively in gate G3 (97%), while R. salina and S. major cultures were in gates G1 (19 and 26%) and G2 (81 and 74%).
DNA was extracted from filter-collected samples using the DNeasy Plant Mini Kit (Qiagen) following the manufacturer's recommendations. Ribulose-1,5-bisphosphate carboxylase oxygenase (RuBisCO) gene fragments were PCR amplified from DNA extracts using the cryptophyte plastid-targeting primers L2F (Hoef-Emden, 2005) and crypt_rbcLR2 (Johnson et al., 2016). PCR conditions were: 95°C for 5 min followed by 40 cycles of 95°C for 60 s, 55°C for 60 s, and 72°C for 90 s followed by 72°C for 7 min. PCR products were visualized by agarose gel electrophoresis and later excised and purified from the gels using the Zymoclean Gel DNA Recovery Kit (Zymo Research). Clone libraries were constructed from gel purified fragments using the pGEM-T Easy Vector in the pGEM-T Easy Vector System II cloning kit (Promega Corporation) according to the manufacturer's protocol. A clone library was constructed for each sample and time point used in this study. For each library, ~45 clones were submitted for Sanger sequencing with a single primer to the W.M. Keck Ecological and Evolutionary Genetics Facility at the MBL as directed. Sequences were edited and assembled into contigs using Sequencher (Gene Codes Corporation). All sequence data were submitted to Genbank (NCBI) under accession numbers MH488130-MH488710.
Cultures of the cryptophytes R. salina, Stoeratula major, and T. amphioxeia were grown in F/2-Si media at 15, 10, and 5 PSU salinity, in order to have a range of options for field conditions in Chesapeake Bay. All cultures were grown at 18–20°C and in 14:10 L:D and maintained in log growth phase during field experiments. In order to stain cryptophytes for grazing experiments, a final concentration of 2 μg ml−1 proflavine was added to 10–20 ml of cryptophyte culture and cells were allowed to take up the dye for 30 min in darkness. Proflavine is a protein stain that is typically not used for labeling living cells, however, it has been used previously on Isochrysis galbana (Dupuy et al., 1999) and marine ciliates (Vincent and Hartmann, 2001) with no short-term mortality. Proflavine was used to stain cryptophyte algae after failed attempts to stain them with Cell-Tracker Green CMFDA (5-chloromethylfluorescein diacetate), a more commonly employed stain (Li et al., 1996). Stained cells were then added to a 15 ml 25 mm diameter glass tower with a 2.0 μm polycarbonate (PC) filter membrane and attached to a side arm flask. Using gentle pressure applied to a hand pump, culture media was slowly removed over 20–30 min, until cells were concentrated down to 2-3 ml. After concentrating the cells, they were washed with F/2-Si media by returning cells to their original volume, and the concentrating and wash step was repeated. When finished, cells were observed live under a dissecting microscope to verify that they were still motile. A small aliquot was then preserved with 1% glutaraldehyde and after 15 min filtered onto a 2 μm PC membrane filter and observed with a Zeiss Axio Scope A1 fluorescence microscope using an excitation BP filter of 450–490 nm in order to verify they were sufficiently stained with proflavine, as well as to count the stock culture. Success of this method depended greatly on how carefully the cells could be concentrated and washed, and the failure rate of the procedure was close to 20%. However, the protocol was considered successful when cryptophyte prey remained motile and all cells were apparently fluorescently stained.
Stained cryptophyte cells were added to Chesapeake Bay or tributary water samples at a final concentration of between ~1,000 and 10,000 cells ml−1. In most cases these concentrations were substantially higher than in situ levels of cryptophytes and likely resulted in estimates of saturated grazing rates. Therefore, these estimates of potential grazing rates are probably akin to maximum rates (but see discussion). Samples were first screened with a 200 μm mesh in order to remove copepods and other mesozooplankton. Samples (10 ml) were taken and preserved with glutaraldehyde, at time 0, 10, 20, 40, and 120 min, as well as 24 h and stored at 4°C in darkness until filtered (5 ml) onto a PC membrane as described above, and mounted on a glass microscope slide as described previously (Johnson et al., 2013). All species of protist that were observed to have ingested fluorescently labeled cryptophytes (FLC) were counted across all time points and instantaneous ingestion rates (IIR) were determined by taking the slope of ingested FLC cell−1 vs. time in hours, and multiply by 24 for prey cells ingested grazer−1 day−1. Clearance rates were calculated using the formula C = IIR/Nprey, where Nprey is the concentration of FLC (Rublee and Gallegos, 1989).
Differences in growth and grazing rates across different experiments were determined using a one-way ANOVA, and comparisons between treatments were clarified using a Tukey HSD test. All statistics were calculated using the R statistical function “AOV” (R Core Team, 2013).
During a cruise in October 2011 we ran dilution experiments in Chesapeake Bay, and measured dynamics of cryptophyte populations within the experiments using flow cytometry. At each station 2–3 subpopulations of cryptophytes could be discerned using flow cytometry and analyzing forward scatter and orange fluorescence (i.e., phycoerythrin) as well as orange vs. red fluorescence. Growth and grazing rates on these cryptophyte subpopulations varied greatly both within and between stations. Growth rates of in situ populations of cryptophytes were higher in the upper Bay (mean: 0.47 d−1) than in the lower Bay and the mouth of the James River Estuary (mean: 0.13 d−1). Grazing rates on cryptophytes were generally high, exceeding combined population growth rates at all stations (Table 2). Only 2 of the 12 subpopulations analyzed in these experiments had positive net population growth, G1 at station 845 and G3 at 834. In the lower Bay and James River station, grazing was 3.2–10.3x (mean: 6.5x) that of growth rates, while in the upper Bay it was 0.3–3.6x (mean: 1.9) greater (Table 2).
Table 2. Population-specific apparent growth and grazing rates for cryptophyte algae during dilution experiments in Chesapeake Bay.
Except for M. rubrum-like ciliates, potential grazers were not counted at every station where dilution experiments were performed. The concentration of M. rubrum at stations 845, 834, and 724 were 7.7, 23.1, and 3.8 cells ml−1, respectively. Cell counts for microzooplankton groups that may have been potential grazers of cryptophytes were only made for one lower and one upper Chesapeake Bay station, which was similar to the location of stations 724 and 845/834, respectively. In lower Chesapeake Bay, potential grazers included oligotrich ciliates at 3.1 cells ml−1, tintinnid ciliates at 6.6 cells ml−1, and naked heterotrophic dinoflagellates (NHD) at 1.2 cells ml−1. The upper Chesapeake Bay station had 1.9 tintinnids ml−1, 8.1 oligotrichs ml−1, and 26 NHDs ml−1. Counts of tintinnid and oligotrich ciliates only included cells >30 μm.
The impact of grazing on cryptophyte community diversity was assessed from dilution experiments at 4 stations during a cruise in October, by clone library sequencing of cryptophyte 18S rDNA amplicons. The cryptophyte clone libraries were dominated by Teleaulax spp. (72%), followed by Rhodomonas spp. (17%) and Hemiselmis spp. (11%). A Teleaulax gracilis phylotype was one of the largest constituents (34.5% of clones) of the cryptophyte communities measured in this study and increased with dilution at all stations (Figure 2). A phylotype of T. amphioxeia was also a dominant species within the clone libraries (32% of all clones), but only revealed increases in the 20% whole seawater dilution at 3 of the stations, and not in 5% dilution treatments (Figure 2). In the upper and mid Bay stations (845, 834), Rhodomonas spp. were a major component of clone libraries, but were nearly absent from lower Bay samples. At both the upper and mid-bay stations, a Rhodomonas sp. phylotype also revealed increases with dilution, consistent with net growth following the dilution of grazing pressure. No data are available for cryptophyte diversity for the 5% dilution at station 845 because no samples were taken for DNA.
Figure 2. Genetic diversity of cryptophytes in dilution experiments during October 2011 in Chesapeake Bay. Dilution experiment treatment is shown on the x-axis, % clone library composition on the y-axis. (A) Results for Stations 845 and 834; (B) Results for stations 724 and James River station 6. Rhodo: Rhodomonas sp., Hemi: Hemiselmis sp., Tamp: Teleaulax amphioxeia; Tgrac: T. gracilis; Tmin: T. minuta.
In order to estimate potential grazing rates of protistan predators on specific cryptophyte algae, we tracked the ingestion of fluorescently labeled prey added to natural samples in three Chesapeake tributaries (Tables 3–5). The mixotrophic ciliate M. rubrum was the most persistent grazer of fluorescently labeled (FL) cryptophytes (Figure 3), being present in all samples, and sometimes at high concentrations (Table 3). Within the Pocomoke River experiments (n = 14), only grazing by M. rubrum was enumerated, and only R. salina was used as prey. The highest ingestion rates for M. rubrum were observed for T. amphioxeia prey (5.4–18.3 prey pred−1 d−1), with grazing on S. major and R. salina being lower (0.17–6.53 prey pred−1 d−1). Grazing by M. rubrum on FL R. salina and S. major was statistically lower than on T. amphioxeia [ANOVA, F(2, 19) = 7.85, p = 0.003; Figure 4A]. Potential grazing impact on cryptophyte populations by M. rubrum alone, a function of both ingestion rate and abundance, varied across all experiments from consumption of 0.14–25.8% of the population d−1.
Table 3. Grazing rates of Mesodinium rubrum (MR)-like ciliates in Chesapeake Bay Tributaries on fluorescently labeled (FL) cryptophyte algae.
Figure 3. Ingestion of fluorescently labeled cryptophytes (FLC) by Mesodinium rubrum in the Choptank River. (A) Examples of ingested FL Teleaulax amphioxeia over time. Regression equations for each experiment are as follows: 4/24/12 station 13, y = 0.225x + 0.072, R2 = 0.999; 4/24/12 station 11, y = 0.567x + 0.160, R2 = 0.908; 4/25/12 station 13, y = 0.748x + 0.156, R2 = 0.888; (B) An M. rubrum cell with an ingested FL Rhodomonas salina under green emission (BP 515–565 nm); (C) Same M. rubrum cell as in (B) with an ingested FL R. salina under a dichromatic long pass emission (>515 nm).
Figure 4. Grazing by phagotrophic protists on three species of cryptophytes. (A) Ingestion rates (IR) of Mesodinium rubrum (Mrub), a Karlodinium veneficum-like dinoflagellate (“Kvene”), a Gyrodinium sp. (Gyro), Oxyrrhis marina (Omar), and an unidentified naked heterotrophic dinoflagellate (NHD) on Rhodomonas salina, Storeatula major and Teleaulax amphioxeia. (B) Grazing potential (GP) on cryptophyte populations. Same information as in (A). Significant differences between IR and GP on different prey indicated by letters.
Grazing on cryptophytes was also documented for various heterotrophic and mixotrophic dinoflagellates. In an experiment within the Potomac River grazing was assessed on all three cryptophyte species, while only T. amphioxeia was used in two experiments in the Choptank River. Ingestion rates by dinoflagellates were high, averaging 28 prey pred−1 d−1 across all dinoflagellate and prey types. Highest grazing rates on cryptophytes were by heterotrophic species (Table 4, Figure 4A), however, many dinoflagellate species did not ingest cryptophytes during experiments. Generally only small or medium (20–40 μm) naked heterotrophic species and small plastid-containing mixotrophic dinoflagellates were observed to graze cryptophytes. High ingestion rates were measured for both Prorocentrum cordatum ( = P. minimum) on T. amphioxeia prey and for a Karlodinum veneficum-like mixotrophic dinoflagellate (referred to hereafter as “K. veneficum”) on all three species (Table 4). Grazing by these mixotrophic dinoflagellates was high in the Choptank River at station S11, which was downriver from a small P. cordatum bloom (6,900 cells ml−1) at station S13. No grazing was observed by P. cordatum on labeled cryptophytes from within the bloom at S13. For one location we measured grazing on multiple cryptophyte cultures, and therefore could determine if certain prey species are grazed more than others. At station P8 in the Potomac River, no differences were observed for grazing rates on three cryptophyte prey species by the mixotroph “K. veneficum” or the heterotrophs O. marina and an unidentified naked heterotrophic dinoflagellate, while the heterotrophic Gyrodinium sp. had significantly lower grazing on T. amphioxeia compared to the other two species [ANOVA, F(2, 3) = 39.68, p = 0.007; HSD P < 0.05; Figure 4A]. The overall potential grazing impact on cryptophyte populations by dinoflagellates varied between 0.7 and 15.5% of any one species of labeled cryptophytes d−1.
Table 4. Grazing rates of dinoflagellates (DINO) in Chesapeake Bay Tributaries on the fluorescently labeled (FL) cryptophytes Storeatula major, Rhodomonas salina, and Teleaulax amphioxeia.
Since we evaluated relatively small volumes of water for estimating potential ingestion rates, only species that were present at high abundances could be measured. Thus, grazing rates were obtained for only two heterotrophic ciliate species. Both a Eutintinnus sp. and an unidentified heterotrophic oligotrich ciliate were found to ingest T. amphioxeia at two stations in the Choptank River, and ingestion rates for both ciliate species were high. The heterotrophic oligotrich ciliate had the highest grazing rate of any species observed, at 149 ± 20 prey pred−1 d−1. Potential impact on T. amphioxeia populations for these ciliates varied from 1.3 to 4.2% of the population d−1.
While numerous laboratory studies have demonstrated that cryptophyte algae are consumed at high rates by a variety of heterotrophic and mixotrophic protists (Jeong et al., 2005; Lewitus et al., 2006; Adolf et al., 2008), fewer studies have sought to document their grazing losses in situ. Cryptophytes are abundant in coastal marine and estuarine ecosystems (Mallin et al., 1991; Jeong et al., 2013; Johnson et al., 2013), and while they may form blooms (Laza-Martínez, 2012; Šupraha et al., 2014), such events are rare and generally short lived. More typically, cryptophytes form multiple seasonal peaks of sustained abundance, but remain in the planktonic community year round (Mallin et al., 1991; Adolf et al., 2006). Here we provide evidence that in situ grazing pressure on cryptophyte algae is high in Chesapeake Bay, and that variability among cryptophyte species in growth rates and susceptibility to grazing may help explain their persistence within the plankton.
Several previous studies using the dilution technique coupled with either flow cytometry (Paterson et al., 2008) or quantitative pigment analyses (Burkill et al., 1987; McManus and Ederington-Cantrell, 1992; Suzuki et al., 1998; Lie and Wong, 2010) have also measured growth and grazing rates on in situ cryptophyte populations. The use of quantitative pigment analysis to monitor in situ population dynamics can be problematic due to regulatory changes in cellular pigment levels during experiments (e.g., photoacclimation) as well as incorrect assignment of pigments to taxonomic groups. In the case of the carotenoid alloxanthin, one cannot differentiate between cryptophyte algae per se and protists that steal their plastids (e.g., M. rubrum), which can be abundant in estuarine and coastal ecosystems (Stoecker et al., 2009). Despite these caveats, such studies are valuable for their potential to estimate group-specific in situ population dynamics. Previous studies that have traced the concentration of alloxanthin within dilution experiments suggest that cryptophyte algal populations are dynamic, with high growth and grazing rates. In one such study using mesocosm enclosures within Saanich Inlet, Canada, alloxanthin-based estimates of cryptophyte growth were >1.0 (max 1.7) d−1 and grazing >0.5 (max 0.9) d−1 during the first 4 days of the experiment (Suzuki et al., 2002). In contrast, another study found that microzooplankton within the coastal seas of Eastern Hong Kong selectively grazed alloxanthin-containing populations, with grazing rates 1–45 times that of growth rates (Lie and Wong, 2010). In a Western Australia study that also measured growth and grazing rates using flow cytometry with dilution experiments, the percent of cryptophyte production grazed varied seasonally and over 3 sites between ~30 and 120% (Paterson et al., 2008).
In Chesapeake Bay, one study found high growth (1.22–2.23 d−1) and grazing (1.08–1.22 d−1) rates based on alloxanthin during spring, becoming lower in August (with net population loss), with both rates becoming negligible during fall (McManus and Ederington-Cantrell, 1992). In our study growth rates of cryptophyte populations measured using flow cytometry during October revealed moderate rates in the upper Bay with net population loss due to grazing, and very low growth rates in the Southern Bay and James River that were greatly exceeded by grazing rates. The James River population had the highest concentrations of M. rubrum-like ciliates encountered during the October Chesapeake Bay cruise (Supplemental Table 1). Such high differences between measurements of growth and grazing rates of a phytoplankton population is not uncommon in dilution experiments, and suggests a decoupling of these processes. In an estuarine or productive coastal environment grazing responses may be greater at times, due to higher standing stocks of microzooplankton from greater overall ecosystem productivity and biomass (Calbet and Landry, 2004). Previous studies have shown that cryptophyte populations in Chesapeake Bay peak in fall (McManus and Ederington-Cantrell, 1992), and that blooms of diatoms or cryptophytes appear to alternate within the southern regions (Adolf et al., 2006). Our measurements appear to capture a period of demise for rich cryptophyte populations in these regions, with growth rates being low or negative and decoupled from intense grazing pressure.
Relatively little is known regarding the genetic diversity of cryptophyte algae in marine ecosystems. Several studies using microscopy or molecular approaches have found that Teleaulax/Plagioselmis/Geminigera (TPG) and Hemiselmis cryptophytes are abundant in marine ecosystems (Hill et al., 1992; Cerino and Zingone, 2007; Metfies et al., 2010; Johnson et al., 2016; Luo et al., 2016). However, these studies are somewhat limited in scope due to their regional specificity. While the study of Johnson et al. (2016) included a broad geographic sampling for cryptophyte genetic diversity, many of the samples were from red tides of the ciliate M. rubrum and therefore were likely skewed toward Teleaulax-dominated communities. While the present study doesn't provide a comprehensive assessment of cryptophyte diversity in Chesapeake Bay, it reveals relative changes in genetic diversity of their communities when grazers are diluted. In this study Teleaulax cryptophytes were dominant in the Southern Bay and James River, comprised about 50% of the population in the middle Bay (834), and were between 35 and 40% in the upper Bay (845). During every experiment, a T. gracilis phylotype increased in response to dilution, suggesting that grazing pressure on this species is high. However, interpreting the observed dynamics of other taxa in these experiments was more complicated. In experiments at stations 834 and JR6, we observed increases in T. amphioxeia only at 20% dilution, but a decline in the proportion of this phylotype within the clone library at 5%. This result suggests that as grazing pressure is diluted, species with higher growth rates are better able to capitalize and grow (e.g., T. gracilis). At station 845 a phylotype of Rhodomonas sp. increased with dilution, suggesting that it too had high grazing pressure and in situ growth rates. Rhodomonas tends to be larger than many other marine cryptophyte species (Johnson, 2015) and station 845 was the only sample where the largest population of cryptophytes measured by flow cytometry had the highest growth rate (Table 2). Our observations of changes in cryptophyte diversity in response to protistan predation is not surprising, as selection or preference for certain species has been observed among various mixotrophs. Acquired phototrophic predators such as M. rubrum (Park et al., 2007; Hansen et al., 2012; Peltomaa and Johnson, 2017), M. chamaeleon (Moeller and Johnson, 2017), and the dinoflagellate Nusuttodinium aeruginosum (Onuma and Horiguchi, 2016) have all been shown to select certain cryptophyte species as prey and/or selectively retain plastids from certain species. Selective grazing on cryptophyte species by constitutive mixotrophic protists, i.e., that possess their own plastids (Mitra et al., 2016), is more rare, but has been demonstrated in the dinoflagellate P. cordatum (Johnson, 2015). Other mixotrophic dinoflagellates, such as K. veneficum, are known to graze on a variety of cryptophytes (Adolf et al., 2008). Less is known regarding selective grazing by heterotrophic protists on cryptophytes.
The majority of our grazing experiments using fluorescently labeled prey were conducted using 10,000 cells ml−1, which is at or near saturating levels of cryptophyte prey for most protist grazers. Saturation of ingestion rate varies with predator type and concentration, but in general dinoflagellates have lower saturation levels then ciliates, occurring in the mixotrophic Prorocentrum donghaiense around 5,000 cells ml−1 (Jeong et al., 2005) and in a small heterotrophic Gyrodinium sp. at around 2,000 cells ml−1 (Jakobsen and Hansen, 1997) when feeding on cryptophytes. For the ciliate M. rubrum saturation of ingestion rate when feeding on T. amphioxeia occurred in a Danish strain (variant F) between 3,000 and 7,000 cells ml−1 (Smith and Hansen, 2007), while in a Chesapeake Bay strain (Variant G) it occurred between 5,000 and 7,000 cells ml−1 (Peltomaa and Johnson, 2017). In contrast ingestion rate of the medium sized mixotrophic oligotrich ciliate, Strombidium rassoulzadegani, saturated around 15,000 cells ml−1 when fed Rhodomonas lens (Schoener and McManus, 2012). While many of our experiments using R. salina prey to measure grazing by M. rubrum were probably below concentrations (i.e., 730–4,223 cells ml−1) that would have saturated grazing using T. amphioxeia as prey, its functional response to Rhodomonas spp. has not been determined.
Another important consideration when adding labeled prey to natural assemblages is the concentration of similar species preexisting within the community. Unfortunately we do not have cell count data for cryptophytes within these samples prior to adding labeled prey, but the mean and range concentrations of cryptophytes observed in Chesapeake Bay are 1,432 and 15,720 cells ml−1, respectively (Johnson et al., 2013).
Previous studies have measured high potential grazing rates by mixotrophic dinoflagellates on cryptophyte algae added to natural samples in Chesapeake Bay (Li et al., 1996), and many cultured mixotrophic dinoflagellates have also been shown to have high grazing rates for cryptophytes (Jeong et al., 2005; Adolf et al., 2008). In Chesapeake Bay, both K. veneficum and P. cordatum have been observed to possess phycoerythrin-containing food vacuoles (Li et al., 1996, 2000, 2001; Stoecker et al., 1997). Predation on cryptophyte populations in Chesapeake Bay is thought to play an important role in bloom initiation of K. veneficum due to the stimulation of its growth rate by mixotrophy (Adolf et al., 2008). Mixotrophic grazing by a P. cordatum culture isolated from the James River region of Chesapeake Bay was induced under N and P limitation and was almost exclusively limited to ingestion of the cryptophyte T. amphioxeia (Johnson, 2015). Consistent with these previous studies, here we found that both “K. veneficum” and P. cordatum ingested T. amphioxeia prey at high rates while “K. veneficum” also readily ingested R. salina and S. major.
In our samples, heterotrophic dinoflagellates were the greatest consumers of labeled cryptophyte prey, due to both their high abundance and ingestion rates (Figure 4B). Small (~20–25 μm) naked heterotrophic dinoflagellates (e.g., Amphidinium sp., Gyrodinium, Oxyrrhis, Pfiesteria) are known to graze voraciously upon cryptophyte algae (Jakobsen and Hansen, 1997; Strom et al., 1998; Eriksen et al., 2002; Jeong et al., 2010), in addition to other prey. In this study, small naked heterotrophic dinoflagellates, consistent in size and shape with Gyrodinium sp., were observed to consume large quantities of R. salina and S. major, but not T. amphioxeia. A microcosm study of natural communities from the Southern Ocean rich in cryptophyte algae also found that cryptophytes are consumed at high rates by heterotrophic Gyrodinium-like dinoflagellates (Bjørnsen and Kuparinen, 1991). While we found O. marina-like cells consumed fluorescently labeled cryptophytes, their rates were lower than co-occurring mixotrophic and other heterotrophic dinoflagellates. Previous studies on cultures of O. marina suggest that it prefers to ingest slightly larger prey than cryptophytes, such as raphidophytes and dinoflagellates (Jeong et al., 2003, 2010).
The highest cell-specific grazing rates that we observed were by large heterotrophic ciliates, including two tintinnid species and an oligotrich. While the grazing rate of the oligotrich ciliate was extremely high, it was comparable to previous rates measured for other large species grazing on cryptophytes (Müller and Schlegel, 1999). In contrast, maximum ingestion rates of S. rassoulzadegani on R. lens were ~10 cells ciliate−1 d−1, (Schoener and McManus, 2012). While this was an order of magnitude lower than our observed rate for an unidentified oligotrich species (Table 5), the species that we observed was larger than S. rassoulzadegani (not shown). The mixotrophic oligotrich ciliate Laboea strobila is known to ingest and steal plastids from cryptophyte prey (Stoecker et al., 1988), and natural populations of the ciliate have been reported to have predominantly cryptophyte plastids (McManus and Fuhrman, 1986).
Table 5. Grazing rates of heterotrophic ciliates in the Choptank River on fluorescently labeled (FL) Teleaulax amphioxeia.
Acquired phototrophs, or non-constitutive mixotrophs (NCMs), which steal plastids from algal prey, are one of the main consumers of cryptophyte algae in marine ecosystems (Stoecker et al., 2009). In particular M. rubrum and M. major are known to selectively graze cryptophytes from the Teleaulax/Plagioselmis/Geminigera (TPG) group (Park et al., 2007; Myung et al., 2011; Hansen et al., 2012; Peltomaa and Johnson, 2017). In the Columbian River Estuary, M. rubrum/major have been observed to rapidly ingest large quantities of cryptophytes by “gathering” prey cells in their feeding tentacles and possibly their cirri as well as cytoplasmic protrusions from their oral region (Peterson et al., 2013). This novel observation needs to be validated under laboratory conditions in order to determine the mechanism of this unusual feeding behavior. Garcia-Cuetos et al. (2012) observed a “medusa” form of M. major that had cytoplasmic projections from its oral regions, and a similar phenotype has been observed in blooms from the Southampton estuary (Crawford, 1993), however feeding events were not observed. We observed the highest grazing rates by Mesodinium spp. on T. amphioxeia, however, moderate grazing rates were also observed on R. salina and S. major in several experiments (Table 3). While previous studies have demonstrated that M. rubrum will ingest non-Teleaulax/Geminigera/Plagioselmis (TGP) cryptophytes (Myung et al., 2011; Hansen et al., 2012), these species have not been shown to support sustained growth. One recent study that used an M. rubrum isolate from the James River in Chesapeake Bay, found that the ciliate ingested and sequestered plastids from T. acuta and T. amphioxeia equally, but found no evidence for ingesting S. major (Peltomaa and Johnson, 2017). This result contrasts with our observations of in situ populations of M. rubrum ingesting fluorescently labeled S. major. Our average grazing rates measured for M. rubrum on T. amphioxeia in this study (9.3 prey MR−1 d−1) were consistent with rates measured previously for a culture from the James River (10–13 prey MR−1 d−1) (Peltomaa and Johnson, 2017). In comparison, the average grazing rates on R. salina and S. major for M. rubrum in Chesapeake Bay were about an order of magnitude lower, both only 1.65 prey MR−1 d−1. While M. rubrum had lower ingestion rates on non-Teleaulax cryptophytes, our experiments demonstrated that it could still have a profound effect on cryptophyte populations (Table 3, Figure 4B). In two cases, the potential impact on cryptophyte populations was >20% of the population d−1, caused in one case by elevated M. rubrum concentrations and ingestion rates, and in another case by low ingestion rates but very high ciliate concentrations (Table 3). These data suggest that when M. rubrum is abundant, they may clear cryptophyte populations, even if they are not their preferred TGP prey. Causes of variation observed here in grazing rates by M. rubrum on fluorescently labeled cryptophytes obviously depend upon the prey species offered, however other factors such as feeding history, the variant(s) of M. rubrum present, and the makeup and abundance of in situ cryptophyte populations are other possible sources.
Our findings demonstrate that cryptophyte populations in Chesapeake Bay are heterogeneous in their species composition, cell size, and growth rates, and experience high in situ grazing pressure. During October 2011 in Chesapeake Bay, cryptophyte populations had high grazing pressure due to protistan predators throughout the system, but the impact was particularly severe in the southern regions where growth rates were very low. Our experiments using fluorescently labeled cryptophyte prey, which were conducted primarily during spring, demonstrated high species-specific grazing rates for various dinoflagellates and ciliates that sometimes varied with prey species. The combined potential impact of various grazers on fluorescently labeled cryptophytes ranged between 19 and 50% of the population d−1, which was lower than our community loss rates estimated from dilution experiments for cryptophyte populations (44–313% d−1). However, direct comparisons between methods are problematic since the experiments were run in different seasons and locations and conducted over different time scales. Further, not all grazers of fluorescently labeled cryptophytes were enumerated, since many predators were at concentrations that were too low to include. Future experiments with fluorescently labeled cryptophytes could be improved by processing larger fixed sample volumes for cell counts. In summary, our findings are the first to demonstrate species-specific selection of cryptophyte prey by natural communities of protist grazers. Observed changes in cryptophyte diversity in dilution experiments combined with high ingestion rates on labeled T. amphioxeia, suggest that Teleaulax cryptophytes generally dominate this ecosystem, possess high growth rates, and are heavily grazed. These results help to explain why certain mixotrophs that select Teleaulax as prey, such as M. rubrum and P. cordatum, are able to maintain high abundance within coastal ecosystems. Further, since heterotrophic protists in our labeled cryptophyte experiments showed either no preference for T. amphioxeia or grazed them at lower rates, competition with these mixotrophic predators for cryptophyte prey may be somewhat alleviated, thus facilitating overlapping niches for phagotrophic protists.
MJ designed and performed experiments, analyzed data, and wrote the manuscript. DB and MF helped with experiments, sample analysis, and in preparing the manuscript. EB helped with experiments and in preparing the manuscript. DS helped in designing and performing experiments, analyzing data, and in preparing the manuscript.
The authors thank the National Science Foundation (OCE 1031718 and 1436169) for providing support for this research.
The authors declare that the research was conducted in the absence of any commercial or financial relationships that could be construed as a potential conflict of interest.
The reviewer GM declared a past co-authorship with several of the authors DS and MJ to the handling Editor.
The authors would like to thank the captain and crew of the R/V Hugh R. Sharp for their assistance and support during our cruises in May and October of 2011. We also thank Drs. Don Anderson and Mengmeng Tong for kindly providing T. amphioxeia culture GCEP01.
The Supplementary Material for this article can be found online at: https://www.frontiersin.org/articles/10.3389/fmars.2018.00241/full#supplementary-material
Adolf, J., Yeager, C. L., Miller, W. D., Mallonee, M., and Harding, L. Jr. (2006). Environmental forcing of phytoplankton floral composition, biomass, and primary productivity in Chesapeake Bay, USA. Estuar. Coast. Shelf Sci. 67, 108–122. doi: 10.1016/j.ecss.2005.11.030
Adolf, J. E., Bachvaroff, T., and Place, A. R. (2008). Can cryptophyte abundance trigger toxic Karlodinium veneficum blooms in eutrophic estuaries? Harmful Algae 8, 119–128. doi: 10.1016/j.hal.2008.08.003
Bjørnsen, P., and Kuparinen, J. (1991). Growth and herbivory by heterotrophic dinoflagellates in the Southern Ocean, studied by microcosm experiments. Mar. Biol. 109, 397–405. doi: 10.1007/BF01313505
Burkill, P., Mantoura, R., Llewellyn, C., and Owens, N. (1987). Microzooplankton grazing and selectivity of phytoplankton in coastal waters. Mar. Biol. 93, 581–590. doi: 10.1007/BF00392796
Calbet, A., and Landry, M. R. (2004). Phytoplankton growth, microzooplankton grazing, and carbon cycling in marine systems. Limnol. Oceanogr. 49, 51–57. doi: 10.4319/lo.2004.49.1.0051
Cerino, F., and Zingone, A. (2007). “Decrypting cryptomonads: a challenge for molecular taxonomy,” in Unravelling the Algae: the Past, Present, and Future of Algal Systematics, eds J. Brodie and J. Lewis (Boca Raton, FL: CRC Press), 197–214. doi: 10.1201/9780849379901.ch11
Crawford, D. W. (1993). Some observations on morphological variation in the red-water ciliate Mesodinium rubrum. J. Mar. Biol. Assoc. U.K. 73, 975–978. doi: 10.1017/S002531540003486X
Dupuy, C., Le Gall, S., Hartmann, H. J., and Bréret, M. (1999). Retention of ciliates and flagellates by the oyster Crassostrea gigas in French Atlantic coastal ponds: protists as a trophic link between bacterioplankton and benthic suspension-feeders. Mar. Ecol. Prog. Ser. 177, 165–175. doi: 10.3354/meps177165
Eriksen, N. T., Hayes, K. C., and Lewitus, A. J. (2002). Growth responses of the mixotrophic dinoflagellates, Cryptoperidiniopsis sp. and Pfiesteria piscicida, to light under prey-saturated conditions. Harmful Algae 1, 191–203. doi: 10.1016/S1568-9883(02)00011-2
Fields, S. D., and Rhodes, R. G. (1991). Ingestion and retention of Chroomonas spp. (Cryptophyceae) by Gymnodinium acidotum (Dinophyceae). J. Phycol. 27, 525–529. doi: 10.1111/j.0022-3646.1991.00525.x
Garcia-Cuetos, L., Moestrup, Ø., and Hansen, P. J. (2012). Studies on the genus Mesodinium II. Ultrastructural and molecular investigations of five marine species help clarifying the taxonomy. J. Eukaryot. Microbiol. 59, 374–400. doi: 10.1111/j.1550-7408.2012.00630.x
Gervais, F. (1997). Light-dependent growth, dark survival, and glucose uptake by cryptophytes isolated from a freshwater chemocline. J. Phycol. 33, 18–25. doi: 10.1111/j.0022-3646.1997.00018.x
Hansen, P. J., Moldrup, M., Tarangkoon, W., Garcia-Cuetos, L., and Moestrup, Ø. (2012). Direct evidence for symbiont sequestration in the marine red tide ciliate Mesodinium rubrum. Aquat. Microb. Ecol. 66, 63–75. doi: 10.3354/ame01559
Hill, D. R., Moestrup, Ø., and Vørs, N. (1992). “Rekylalger (Cryptophyceae),” in Plankton i de Indre Danske Farvande, ed H. A. Thompson (Havforskning fra Miljøstyrelsen), 251–265.
Hoef-Emden, K. (2005). Multiple independent losses of photosynthesis and differing evolutionaryrates in the genus Cryptomonas (Cryptophyceae): combined phylogenetic analyses of DNA sequences of the nuclear and the nucleomorph ribosomal operons. J. Mol. Evol. 60, 183–195. doi: 10.1007/s00239-004-0089-5
Jakobsen, H. H., and Hansen, P. J. (1997). Prey size selection, grazing and growth response of the small heterotrophic dinoflagellate Gymnodinium sp. and the ciliate Balanion comatum—a comparative study. Mar. Ecol. Prog. Ser. 158, 75–86.
Jeong, H., Yoo, Y., Kim, J., Seong, K., Kang, N., and Kim, T. (2010). Growth, feeding and ecological roles of the mixotrophic and heterotrophic dinoflagellates in marine planktonic food webs. Ocean Sci. J. 45, 65–91. doi: 10.1007/s12601-010-0007-2
Jeong, H. J., Du Yoo, Y., Lee, K. H., Kim, T. H., Seong, K. A., Kang, N. S., et al. (2013). Red tides in Masan Bay, Korea in 2004–2005: I. Daily variations in the abundance of red-tide organisms and environmental factors. Harmful Algae 30, S75–S88. doi: 10.1016/j.hal.2013.10.008
Jeong, H. J., Ha, J. H., Yoo, Y. D., Park, J. Y., Kim, J. H., Kang, N. S., et al. (2007). Feeding by the Pfiesteria-like heterotrophic dinoflagellate Luciella masanensis. J. Eukaryot. Microbiol. 54, 231–241. doi: 10.1111/j.1550-7408.2007.00259.x
Jeong, H. J., Kim, J. S., Yoo, Y. D., Kim, S. T., Kim, T. H., Park, M. G., et al. (2003). Feeding by the heterotrophic dinoflagellate Oxyrrhis marina on the red-tide raphidophyte Heterosigma akashiwo: a potential biological method to control red tides using mass-cultured grazers. J. Eukaryot. Microbiol. 50, 274–282. doi: 10.1111/j.1550-7408.2003.tb00134.x
Jeong, H. J., Yoo, Y. D., Park, J. Y., Song, J. Y., Kim, S. T., Lee, S. H., et al. (2005). Feeding by phototrophic red-tide dinoflagellates: five species newly revealed and six species previously known to be mixotrophic. Aquat. Microb. Ecol. 40, 133–150. doi: 10.3354/ame040133
Johnson, M., Rome, M., and Stoecker, D. (2003). Microzooplankton grazing on Prorocentrum minimum and Karlodinium micrum in Chesapeake Bay. Limnol. Oceanogr. 48, 238–248. doi: 10.4319/lo.2003.48.1.0238
Johnson, M. D. (2015). Inducible mixotrophy in the dinoflagellate Prorocentrum minimum. J. Eukaryot. Microbiol. 62, 431–443. doi: 10.1111/jeu.12198
Johnson, M. D., Beaudoin, D. J., Laza-Martinez, A., Dyhrman, S. T., Fensin, E., Lin, S., et al. (2016). The genetic diversity of Mesodinium and associated cryptophytes. Front. Microbiol. 7:2017. doi: 10.3389/fmicb.2016.02017
Johnson, M. D., Stoecker, D. K., and Marshall, H. G. (2013). Seasonal dynamics of Mesodinium rubrum in Chesapeake Bay. J. Plankton Res. 35, 877–893. doi: 10.1093/plankt/fbt028
Landry, M. (1993). Estimating Rates of Growth and Grazing Mortality of Phytoplankton by the Dilution Method. Boca Raton, FL: Lewis Publishers.
Larsen, J. (1988). An ultrastructural-study of Amphidinium poecilochroum (Dinophyceae), a phagotrophic dinoflagellate feeding on small species of cryptophytes. Phycologia 27, 366–377. doi: 10.2216/i0031-8884-27-3-366.1
Laza-Martínez, A. (2012). Urgorri complanatus gen. et sp. nov.(cryptophyceae), a red-tide-forming species in brackish waters. J. Phycol. 48, 423–435. doi: 10.1111/j.1529-8817.2012.01130.x
Lewitus, A. J., Caron, D. A., and Miller, K. R. (1991). Effects of light and glycerol on the organization of the photsyntehtic apparatus in the faculatative heterotroph Pyrenomonas salina (cryptophyceae).1. J. Phycol. 27, 578–587. doi: 10.1111/j.0022-3646.1991.00578.x
Lewitus, A. J., and Kana, T. M. (1995). Light respiration in six estuarine phytoplankton species: contrasts under photoautotrophic and mixotrophic growth conditions. J. Phycol. 31, 754–761. doi: 10.1111/j.0022-3646.1995.00754.x
Lewitus, A. J., Wetz, M. S., Willis, B. M., Burkholder, J. M., Parrow, M. W., and Glasgow, H. B. (2006). Grazing activity of Pfiesteria piscicida (Dinophyceae) and susceptibility to ciliate predation vary with toxicity status. Harmful Algae 5, 427–434. doi: 10.1016/j.hal.2006.04.012
Li, A., Stoecker, D. K., and Adolf, J. E. (1999). Feeding, pigmentation, photosynthesis and growth of the mixotrophic dinoflagellate Gyrodinium galatheanum. Aquat. Microb. Ecol. 19, 163–176. doi: 10.3354/ame019163
Li, A., Stoecker, D. K., and Coats, D. W. (2000). Spatial and temporal aspects of Gyrodinium galatheanum in Chesapeake Bay: distribution and mixotrophy. J. Plankton Res. 22, 2105–2124. doi: 10.1093/plankt/22.11.2105
Li, A., Stoecker, D. K., and Coats, D. W. (2001). Use of the 'food vacuole content' method to estimate grazing by the mixotrophic dinoflagellate Gyrodinium galatheanum on cryptophytes. J. Plankton Res. 23, 303–318. doi: 10.1093/plankt/23.3.303
Li, A., Stoecker, D. K., Coats, D. W., and Adam, E. J. (1996). Ingestion of fluorescently labeled and phycoerythrin-containing prey by mixotrophic dinoflagellates. Aquat. Microb. Ecol. 10, 139–147. doi: 10.3354/ame010139
Lie, A. A., and Wong, C. K. (2010). Selectivity and grazing impact of microzooplankton on phytoplankton in two subtropical semi-enclosed bays with different chlorophyll concentrations. J. Exp. Mar. Biol. Ecol. 390, 149–159. doi: 10.1016/j.jembe.2010.05.001
Liu, H., Chen, M., Suzuki, K., Wong, C. K., and Chen, B. (2010). Mesozooplankton selective feeding in subtropical coastal waters as revealed by HPLC pigment analysis. Mar. Ecol. Prog. Ser. 407, 111–123. doi: 10.3354/meps08550
Luo, W., Li, H., Gao, S., Yu, Y., Lin, L., and Zeng, Y. (2016). Molecular diversity of microbial eukaryotes in sea water from Fildes Peninsula, King George Island, Antarctica. Polar Biol. 39, 605–616. doi: 10.1007/s00300-015-1815-8
Mallin, M. A., Paerl, H. W., and Rudek, J. (1991). Seasonal phytoplankton composition, productivity and biomass in the Neuse River estuary, North Carolina. Estuar. Coast. Shelf Sci. 32, 609–623. doi: 10.1016/0272-7714(91)90078-P
Marshall, H. G., Burchardt, L., and Lacouture, R. (2005). A review of phytoplankton composition within Chesapeake Bay and its tidal estuaries. J. Plankton Res. 27, 1083–1102. doi: 10.1093/plankt/fbi079
Marshall, W., and Laybourn-Parry, J. (2002). The balance between photosynthesis and grazing in Antarctic mixotrophic cryptophytes during summer. Freshw. Biol. 47, 2060–2070. doi: 10.1046/j.1365-2427.2002.00950.x
McManus, G. B., and Ederington-Cantrell, M. C. (1992). Phytoplankton pigments and growth rates, and microzooplankton grazing in a large temperate estuary. Mar. Ecol. Prog. Ser. 87, 77–85. doi: 10.3354/meps087077
McManus, G. B., and Fuhrman, J. A. (1986). Photosynthetic pigments in the ciliate Laboea strobila from Long Island Sound, USA. J. Plankton Res. 8, 317–327. doi: 10.1093/plankt/8.2.317
Metfies, K., Gescher, C., Frickenhaus, S., Niestroy, R., Wichels, A., Gerdts, G., et al. (2010). Contribution of the class cryptophyceae to phytoplankton structure in the German Bight. J. Phycol. 46, 1152–1160. doi: 10.1111/j.1529-8817.2010.00902.x
Mitra, A., Flynn, K. J., Tillmann, U., Raven, J. A., Caron, D., Stoecker, D. K., et al. (2016). Defining planktonic protist functional groups on mechanisms for energy and nutrient acquisition: incorporation of diverse mixotrophic strategies. Protist 167, 106–120. doi: 10.1016/j.protis.2016.01.003
Moeller, H. V., and Johnson, M. D. (2017). Preferential plastid retention by the acquired phototroph Mesodinium chamaeleon. J. Eukaryot. Microbiol. 65, 148–158. doi: 10.1111/jeu.12446
Müller, H., and Schlegel, A. (1999). Responses of three freshwater planktonic ciliates with different feeding modes to cryptophyte and diatom prey. Aquat. Microb. Ecol. 17, 49–60. doi: 10.3354/ame017049
Myung, G., Kim, H. S., Park, J. S., Park, M. G., and Yih, W. (2011). Population growth and plastid type of Myrionecta rubra depend on the kinds of available cryptomonad prey. Harmful Algae 10, 536–541. doi: 10.1016/j.hal.2011.04.005
Onuma, R., and Horiguchi, T. (2016). Specificity of Chroomonas (Cryptophyceae) as a source of kleptochloroplast for Nusuttodinium aeruginosum (Dinophyceae). Phycol. Res. 64, 35–43. doi: 10.1111/pre.12117
Park, J. S., Myung, G., Kim, H. S., Cho, B. C., and Yih, W. (2007). Growth responses of the marine photosynthetic ciliate Myrionecta rubra to different cryptomonad strains. Aquat. Microb. Ecol. 48, 83–90. doi: 10.3354/ame048083
Paterson, H., Knott, B., Koslow, A., and Waite, A. (2008). The grazing impact of microzooplankton off south west Western Australia: as measured by the dilution technique. J. Plankton Res. 30, 379–392. doi: 10.1093/plankt/fbn004
Peltomaa, E., and Johnson, M. D. (2017). Mesodinium rubrum exhibits genus-level but not species-level cryptophyte prey selection. Aquat. Microb. Ecol. 78, 147–159. doi: 10.3354/ame01809
Peterson, T. D., Golda, R. L., Garcia, M. L., Li, B., Maier, M. A., Needoba, J. A., et al. (2013). Associations between Mesodinium rubrum and cryptophyte algae in the Columbia River estuary. Aquat. Microb. Ecol. 68, 117–130. doi: 10.3354/ame01598
R Core Team (2013). R: A Language and Environment for Statistical Computing. Vienna: R Foundation for Statistical Computing. Available online at: http://www.R-project.org/
Rublee, P. A., and Gallegos, C. L. (1989). Use of fluorescently labelled algae (FLA) to estimate microzooplankton grazing. Mar. Ecol. Prog. Ser. 51, 221–227. doi: 10.3354/meps051221
Schoener, D., and McManus, G. (2012). Plastid retention, use, and replacement in a kleptoplastidic ciliate. Aquat. Microb. Ecol. 67:177. doi: 10.3354/ame01601
Skovgaard, A. (1998). Role of chloroplast retention in a marine dinoflagellate. Aquat. Microb. Ecol. 15, 293–301. doi: 10.3354/ame015293
Smith, M., and Hansen, P. J. (2007). Interaction between Mesodinium rubrum and its prey: importance of prey concentration, irradiance and pH. Mar. Ecol. Progr. Ser. 338, 61–70. doi: 10.3354/meps338061
Spear-Bernstein, L., and Miller, K. R. (1989). Unique location of the phycobiliprotein light-harvesting pigment in the Cryptophyceae. J. Phycol. 25, 412–419. doi: 10.1111/j.1529-8817.1989.tb00245.x
Stoecker, D., Li, A., Coats, D., Gustafson, D., and Nannenl, M. (1997). Mixotrophy in the dinoflagellate Prorocentrum minimum. Mar. Ecol. Prog. Ser. 152, 1–12. doi: 10.3354/meps152001
Stoecker, D. K., Johnson, M. D., de Vargas, C., and Not, F. (2009). Acquired phototrophy in aquatic protists. Aquat. Microb. Ecol. 57, 279–310. doi: 10.3354/ame01340
Stoecker, D. K., and Silver, M. W. (1990). Replacement and aging of chloroplasts in Strombidium capitatum (Ciliophora, Oligotrichida). Mar. Biol. 107, 491–502. doi: 10.1007/BF01313434
Stoecker, D. K., Silver, M. W., Michaels, A. E., and Davis, L. H. (1988). Obligate mixotrophy inLaboea strobila, a ciliate which retains chloroplasts. Mar. Biol. 99, 415–423.
Strom, S. L., Morello, T. A., and Bright, K. J. (1998). Protozoan size influences algal pigment degradation during grazing. Mar. Ecol. Prog. Ser. 164, 189–197. doi: 10.3354/meps164189
Šupraha, L., Bosak, S., Ljubešić, Z., Mihanović, H., Olujić, G., Mikac, I., et al. (2014). Cryptophyte bloom in a Mediterranean estuary: high abundance of Plagioselmis cf. prolonga in the Krka River estuary (eastern Adriatic Sea). Sci. Mar. 78, 329–338. doi: 10.3989/scimar.03998.28C
Suzuki, K., Tsuda, A., Kiyosawa, H., Takeda, S., Nishioka, J., Saino, T., et al. (2002). Grazing impact of microzooplankton on a diatom bloom in a mesocosm as estimated by pigment-specific dilution technique. J. Exp. Mar. Biol. Ecol. 271, 99–120. doi: 10.1016/S0022-0981(02)00038-2
Suzuki, T., Yamada, N., and Taniguchi, A. (1998). Standing crops of planktonic ciliates and nanoplankton in oceanic waters of the western Pacific. Aquat. Microb. Ecol. 14, 49–58. doi: 10.3354/ame014049
Tõnno, I., Agasild, H., Kõiv, T., Freiberg, R., Nõges, P., and Nõges, T. (2016). Algal diet of small-bodied crustacean zooplankton in a cyanobacteria-dominated Eutrophic Lake. PLoS ONE 11:e0154526. doi: 10.1371/journal.pone.0154526
Vincent, D., and Hartmann, H. J. (2001). Contribution of ciliated microprotozoans and dinoflagellates to the diet of three copepod species in the Bay of Biscay. Hydrobiologia 443, 193–204. doi: 10.1023/A:1017502813154
Weisse, T., and Kirchhoff, B. (1997). Feeding of the heterotrophic freshwater dinoflagellate Peridiniopsis berolinense on cryptophytes: analysis by flow cytometry and electronic particle counting. Aquat. Microb. Ecol. 12, 153–164. doi: 10.3354/ame012153
Yoo, Y. D., Seong, K. A., Jeong, H. J., Yih, W., Rho, J.-R., Nam, S. W., et al. (2017). Mixotrophy in the marine red-tide cryptophyte Teleaulax amphioxeia and ingestion and grazing impact of cryptophytes on natural populations of bacteria in Korean coastal waters. Harmful Algae 68, 105–117. doi: 10.1016/j.hal.2017.07.012
Keywords: cryptophytes, mixotrophy, grazing, Chesapeake Bay, dinoflagellates, Mesodinium rubrum
Citation: Johnson MD, Beaudoin DJ, Frada MJ, Brownlee EF and Stoecker DK (2018) High Grazing Rates on Cryptophyte Algae in Chesapeake Bay. Front. Mar. Sci. 5:241. doi: 10.3389/fmars.2018.00241
Received: 27 March 2018; Accepted: 25 June 2018;
Published: 25 July 2018.
Edited by:
Stelios Katsanevakis, University of the Aegean, GreeceReviewed by:
Kalle Olli, University of Tartu, EstoniaCopyright © 2018 Johnson, Beaudoin, Frada, Brownlee and Stoecker. This is an open-access article distributed under the terms of the Creative Commons Attribution License (CC BY). The use, distribution or reproduction in other forums is permitted, provided the original author(s) and the copyright owner(s) are credited and that the original publication in this journal is cited, in accordance with accepted academic practice. No use, distribution or reproduction is permitted which does not comply with these terms.
*Correspondence: Matthew D. Johnson, bWF0dGpvaG5zb25Ad2hvaS5lZHU=
Disclaimer: All claims expressed in this article are solely those of the authors and do not necessarily represent those of their affiliated organizations, or those of the publisher, the editors and the reviewers. Any product that may be evaluated in this article or claim that may be made by its manufacturer is not guaranteed or endorsed by the publisher.
Research integrity at Frontiers
Learn more about the work of our research integrity team to safeguard the quality of each article we publish.