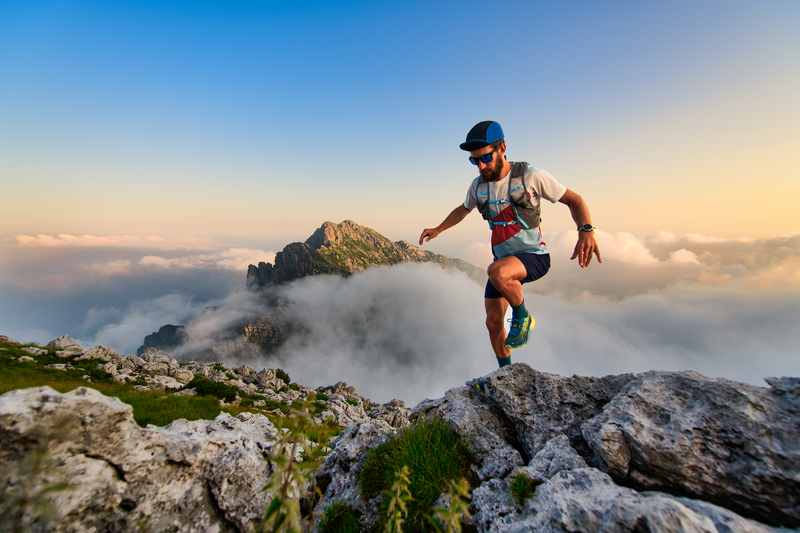
95% of researchers rate our articles as excellent or good
Learn more about the work of our research integrity team to safeguard the quality of each article we publish.
Find out more
ORIGINAL RESEARCH article
Front. Mar. Sci. , 04 June 2018
Sec. Coral Reef Research
Volume 5 - 2018 | https://doi.org/10.3389/fmars.2018.00187
This article is part of the Research Topic EU Project TASCMAR - A Springboard for Accelerating the Valorisation of Mesophotic Coral Ecosystems View all 7 articles
Accompanying the recent technological innovations in remotely operated vehicles (ROVs), submersibles, technical SCUBA, and closed-circuit rebreather diving gear, new discoveries are being made on mesophotic coral ecosystems around the world. However, collecting live fishes from mesophotic depths (60–150 m) is challenging, given the difficulty of accessing the habitat, catching the fishes, and the barotrauma that can result from rapid decompression during their transport to the surface. Here, we designed and tested the performance of a portable, submersible hyperbaric chamber, the SubCAS, which we used to safely surface reef fishes from mesophotic depths. During six expeditions between 2014 and 2017 to the Philippines, Vanuatu, Palau and Pohnpei, we assessed the survival of 174 fishes caught between 60 and 150 m depth and decompressed using this chamber. A total of 155 (89.1%) fishes survived decompression, and 143 of 148 specimens shipped (96.6%) survived air cargo transport from remote field sites to the Steinhart Aquarium at the California Academy of Sciences. Survival was significantly related to taxonomic family, with Pomacentridae and Apogonidae showing the highest mortality. Collection depth, fish body size, and length of decompression had no relation to survivorship. Significant interactions between individual decompression events and both fish body size and taxonomic family indicate that low survival was associated with specific SubCAS trials. The SubCAS has allowed us to reliably surface charismatic fishes previously unknown to science and maintain them in aquaria for research and public engagement purposes. This opportunity facilitates a direct connection between our more than one million annual visitors and the wonders of exploration and the science of mesophotic coral ecosystems.
The deeper portions of coral reefs, known as mesophotic coral ecosystems (MCEs) are currently the subject of intense research and are adding significant advances to our knowledge of coral reef systems (Bongaerts et al., 2010; Slattery et al., 2011; van Oppen et al., 2011; Loya et al., 2016; Pinheiro et al., 2016). This explosion in deep coral reef biology and ecology has been fueled by technological advances in remotely operated vehicles (ROV), autonomous underwater vehicles (AUVs), manned submersibles and submarines, and technical SCUBA diving gear, especially mixed-gas, closed circuit rebreathers (CCR). Scientific teams have begun investigating MCEs (60–150 m) around the globe, with major implications to the understanding of coral reef biology and the emerging threats to coral reef ecosystems (Bongaerts et al., 2010; Slattery et al., 2011; van Oppen et al., 2011; Loya et al., 2016; Pinheiro et al., 2016). New records and new species discoveries are slowly filling our knowledge gap of the diversity and distribution of fishes inhabiting MCEs (Kane et al., 2014; Wagner et al., 2014; Pinheiro et al., 2015; Pyle and Kosaki, 2016; Simon et al., 2016; Rocha et al., 2017). However, despite all of this work, there are relatively few instances where live specimens collected from MCEs are successfully being studied ex situ or utilized to directly engage and inform the public about the existence of and threats to these remarkable ecosystems.
Collecting live fishes from mesophotic depths is challenging due to the limited access to the environment and the difficulty of catching fishes using ROVs or submarines, or while diving in extreme exposure situations (Pyle, 2000). The logistical considerations for this type of work are daunting, given the pressure changes between even the shallowest mesophotic ecosystems (30–60 m) and the surface. These deep environments were formed by coral reefs during the last glacial period, which drowned as sea level rose to present levels roughly 14,000 years ago, and many remain complex, three-dimensional habitats (Sanborn et al., 2017). The complexity of deep reefs allows fishes to easily escape the bright illumination and loud noises associated with ROVs and AUVs and remain undiscovered. Although it is very difficult to catch a fish with a robot, maneuverable arms, chemical injection systems, hydraulic traps, suction devices, and even laser-aimed sedative spear injections have been used to collect fishes from the deep ocean (Gilmore, 2016). A particularly innovative design developed in Japan, the pressure-stat aquarium, allowed collection of deepwater fishes via submersibles directly into a stainless-steel chamber, where they were maintained under pressure in a laboratory for up to 64 days (Koyama et al., 2002).
Divers employing mixed-gas, closed-circuit rebreathers have less time to work in these deep reefs compared to ROVs or submarines, but they are quieter, less disruptive, and more agile, and are thus more successful at finding and catching small, cryptic reef fishes (Pyle, 2000). However, mixed-gas divers must exit the bottom rapidly, ascending as much as 50 m before beginning their own decompression, and then pausing every 3 m or so for progressively longer times. This requirement complicates safe handling of the fishes, which are sensitive to changes in temperature, light levels, oxygen concentrations, and pressure, with a safe decompression timetable that often differs from that of the divers (Wilson and Smith, 1985). Rapid ascent barotrauma causes a variety of physical and physiological effects to fishes, including exophthalmia, swim-bladder overexpansion, oral eversion, cloacal protrusion, subcutaneous gas bubbles, emboli, hematoma, swim-bladder rupture, and other organ damage (Rogers et al., 1986; Rummer and Bennett, 2005; Jarvis and Lowe, 2008; Sato et al., 2010; Pribyl et al., 2011). Thus, in order for fishes to survive a trip to the surface from mesophotic depths, intervention is required.
Ascending fishes from mesophotic depths usually employs one of three methods: (1) venting (2) staged decompression or (3) hyperbaric treatment. Venting, also known as “fizzing” or “needling,” consists of puncturing the swim bladder with a hypodermic needle to release the expanded gas before it can cause damage to internal organs. Although this technique can be employed successfully, there is risk of unintentional damage to neighboring organs, necrosis at the puncture site, and potential bacterial infection from repeated punctures and/or reused needles (Nguyen et al., 2009; Drumhiller et al., 2014; Munday et al., 2015). Divers or manned submersibles will occasionally stage fishes for decompression stops at intermediate depths in baskets, buckets or other enclosures, retrieving them later (Munday et al., 2015). However, this complicates remote operations where multiple field sites are being surveyed across sequential days. This approach can also expose the fishes to temperature stress from being staged above the thermocline(s) as well as stress or damage from being contained an unnatural environment with other, perhaps incompatible, fishes. Finally, hyperbaric treatment involves using a pressurized chamber to slowly decompress fishes at a rate where they do not suffer the ill effects of barotrauma (Lobel and Culp, 1976; Smiley and Drawbridge, 2007; Welsh, 2012; Munday et al., 2015).
Portable hyperbaric chambers have been successfully employed to collect deepwater fishes, however, most of these are employed after the fish has been surfaced, and are actually recompression chambers (Smiley and Drawbridge, 2007; Ballard, 2008; Sato et al., 2010; Welsh, 2012). Submersible hyperbaric chambers, true decompression chambers, have been used to reduce stress and mortality in live fish collections (Lobel and Culp, 1976; Wilson and Smith, 1985). However the prior designs were too large, heavy and cumbersome to be deployed by mesophotic divers, who already are carrying a significant amount of equipment during extreme exposure, mixed-gas diving. Additionally, most prior designs were closed-systems pressurized by air or oxygen, and thus did not allow the user to perform water changes or observe, feed or otherwise manage the fishes during the lengthy decompression (Lobel and Culp, 1976; Ballard, 2008). A hyperbaric-trap aquarium was constructed and deployed at 1,000–1,400 m depth in order to capture and study grenadiers, Coryphaenoides acrolepis, however no fish were successfully decompressed to ambient pressure (Wilson and Smith, 1985). At the Monterey Bay Aquarium, California (USA), a recompression chamber design facilitated care and management of deepwater fishes throughout extended decompression by allowing for water exchange, temperature control, feeding, and observation of the specimens (Welsh, 2012). These fishes were caught on rod and reel and placed in the chamber at the surface, and thus were subjected to barotrauma (Welsh, 2012). This approach is similar to that taken by recreational anglers, and numerous studies have been conducted on how recompression increases survival of deep-sea catch and release fishing (reviewed in Brown et al., 2010).
As part of an initiative to increase scientific knowledge and public engagement around coral reefs, we developed an exhibit about MCEs at the California Academy of Sciences (San Francisco, USA). To facilitate the acquisition of living fishes for our exhibit, we designed and constructed a portable, submersible hyperbaric chamber, the SubCAS (Submersible Chamber for Ascending Specimens). This tool was successfully employed to decompress 174 fishes from up to 150 m depth during six expeditions at remote field sites from 2014 through 2017. Here, we describe the design and use of this device, and the testing of its performance, along with how taxonomic family, size class, capture depth, capture location, length of decompression, and various external factors impacted our success.
Several constraints guided our design process. First, the SubCAS had to be robust, and easily transportable to remote field sites. Second, it must be made of readily-available, standard-size parts and fittings so that it could be repaired or modified in the field, as necessary. It needed to be submersible, compact enough to carry and easy enough to operate by mesophotic divers, who are already burdened by nearly 100 kg of other equipment: the rebreather, several SCUBA cylinders of emergency bail-out gases, various safety and collecting gear, and diver propulsion vehicles. Ideally it would be transparent or have a large window to monitor the fishes during decompression. Finally, it must be able to handle enough pressure to enable safe transport and decompression of fishes from depths of up to 150 m.
In order to meet these constraints, we designed the SubCAS around an injection molded 50.8 cm polypropylene water filter housing (PureT C908-BK1-PR, PureT Water Treatment Technologies, USA). This filter housing has a working pressure rating of 6.9 bar, which allows pressurization at intermediate depths where mesophotic divers can briefly pause during ascent. The filter housing consists of two main parts: a clear cylinder and an opaque black cap (Figure 1). The housing is sealed by screwing the cylinder into the cap and tightening it with a plastic filter housing wrench. Two O-rings, one in the cap and one set into a groove in the top rim of the cylinder, seal the unit and maintain the internal pressure. The cap has two integrated 2.5 cm female threaded ports and a pressure-relief button. We reversed the intended flow direction through these ports, using the inlet as the outlet, because the outlet port was more suited to channel water flow directly into the inner collecting jar. In order to prevent inadvertent depressurization of the unit, we disabled the pressure-relief button by removing the internal spring and locking it in the closed position with a stainless steel screw. One 2.5 cm threaded port was reduced with a PVC bushing to connect 6 mm diameter plastic tubing and a small valve to control water flow into the housing. A modified PVC plastic pipe cap or a custom 3D-printed plastic shield was used to cover and protect these fittings and the 6 mm water supply tubing connection. A 2.5 cm true-union valve was plumbed into the second threaded port. Water leaves the chamber through the true-union valve, which also serves as a convenient handle for divers to use when closing and pressurizing the chamber. The true-union fitting on the downstream side of the valve handle can easily be removed and swapped with other fittings while keeping the valve closed and the chamber pressurized. This design creates a convenient connection point for divers to attach a low-pressure hose from a small SCUBA cylinder to re-pressurize the unit in the event that they encounter a slow leak upon ascent, as well as for the fittings to connect the 12-mm water discharge tubing once the SubCAS is on the surface. In order to prevent divers from accidentally depressurizing the SubCAS during ascent, the handle on the true-union valve was removed or cut into a circular shape (Figure 2).
Figure 1. The portable, submersible hyperbaric chamber (SubCAS) described in the text, showing: (A) hyperbaric chamber, (B) inner collecting jar, (C) submersible depth gauge, (D) removable chamber cap, (E) filter housing wrench, (F) 6 mm tubing connection and isolation valve for water supply, and (G) true-union valve, and connection for 12 mm water discharge tubing, which also serves as a second handle to securely close the unit.
Figure 2. The SubCAS chamber cap, showing: (A) 2.5 cm threaded port, reduced to connect 6 mm diameter water supply tubing and an isolation valve, (B) 2.5 cm threaded port with true-union valve and connection for 12 mm water discharge tubing (C) the pressure-relief button, which has been disabled, and (D) the custom 3-D printed plastic shield that protects the tubing and fittings. Note the reversed flow direction through the cap inlet and outlet, and the modified true-union valve handle, as described in the text.
A custom-designed acrylic inner collecting jar (My Reef Creations, USA) serves as primary containment for the fishes, and was designed to fit snugly within the water filter housing (Figure 3). This inner collecting jar has a series of 6 mm perforations in both ends, allowing for water to flow from top to bottom through the entire length of the SubCAS. It also has a hinged access door secured with a Velcro lock. Divers add fishes to the collecting jar through this door. One end can be completely removed by unscrewing 3 plastic thumb screws. The various components of the hyperbaric chamber are shown in Figures 1–3, and component part numbers and manufacturers are listed in Supplementary Table 1.
Figure 3. The custom-designed inner collecting jar, showing: (A) the hinged access door that divers use when collecting fishes, (B) the Velcro “lock” for the access door, (C) the submersible depth gauge and (D) the small holes for channeling water flow through the jar during decompression.
The chambers are connected to a filtered and temperature-controlled reservoir, essentially a temporary aquarium holding system (Figure 4). Water circulation to the chambers is provided by a high-pressure pump (SHURflo model 9325-043-101, Pentair Filtration, Inc., USA). Water supply can be routed to multiple chambers in parallel through a control manifold assembly and 12 mm diameter plastic tubing and fittings (John Guest Super Speedfit, John Guest USA Inc., USA). It is critical that all fittings and tubing have a pressure-rating of at least 6.9 bar, and preferably 10.3 bar. An adjustable pressure control valve (Plastomatic RVDM In-line Pressure Relief Valve, PVC/EPDM Thd, Indelco Plastics Corporation, USA) allows the chamber operator to control the working pressure of the system. The working pressure, which equates to the virtual depth of the fishes within the chamber, is monitored by an in-line pressure gauge located adjacent to the pressure control valve, as well as submersible depth gauges located in each inner collecting jar.
Figure 4. Schematic of the hyperbaric chamber in field use, showing: (A) pair of hyperbaric chambers containing mesophotic fishes, (B) seawater reservoir, (C) high-pressure pump (D) control manifold with in-line pressure gauge and adjustable pressure control valve, (E) aquarium life support equipment for filtration and temperature control. Arrows indicate water flow direction. Black lines denote 6 mm water supply tubing, gray lines represent 12 mm water discharge tubing, and the dashed line signifies the 6 mm bypass tubing connection. Circles marked with diagonal lines represent the valves used for flow-control and to isolate the chambers.
Divers collect mesophotic fishes using hand nets, occasionally with the assistance of chemical anesthetics (clove oil or quinaldine sulfate). Fishes are selected for collection based on several criteria: taxonomic status (new species and new records are priorities), suitability for aquarium care (appropriate size and demeanor), ability to engage members of the public (appearance and behavior), and the divers' ability to capture the fishes. Following capture, fishes are placed in the inner collecting jar for the duration of the working part of the dive. After leaving the bottom, divers ascend to a deep stop at ~55–60 m. Here, the inner collecting jar is inserted into the hyperbaric chamber, and an air bubble (~300 cm3) is blown into the chamber lid. The air bubble is critical, as it expands during ascent and maintains the internal pressure in the chamber. Care must be taken to ensure that the O-rings are free from debris or sediment, and then the unit is closed and sealed (Figure 5). At least one of the control valves needs to be closed after the unit is sealed; if these valves are closed before sealing, it is nearly impossible to remove or replace the lid due to the incompressibility of water. Once sealed properly, the SubCAS maintains a pressure of ~6–7 bar for the duration of the ascent.
Figure 5. A rebreather diver places the inner collection jar into the SubCAS, and then seals the chamber at an intermediate depth of ~60 m. The hinged access door, Velcro lock, submersible depth gauge and thermometer are visible on the inner collection jar, which is in the diver's right hand. The chamber cap and body are in the diver's left hand. In this version of the SubCAS, the valves and fittings on the chamber cap were protected by a white shroud constructed from an 18 cm diameter schedule 40 PVC cap.
A support dive team meets the mesophotic team at ~30 m, and the SubCAS is handed off and immediately brought to the surface. The support team connects a 12 V battery powered high-pressure pump to the SubCAS via the tubing connections. This pump maintains pressure and provides clean, oxygenated water to the fishes inside. The team uses bags of ice to control water temperature during the boat ride back to the field station. At the field station, the SubCAS is switched over to an AC-powered high-pressure pump and connected to a larger water system where temperature, salinity and metabolic wastes are managed and kept within tolerance limits. Here, the fishes are given professional care for the duration of their decompression and during the period between release from the chamber and shipping back to our museum.
Decompression was performed by opening the adjustable pressure control valve at set time intervals and targeting a specific depth reduction, as measured by the submersible depth gauge. Decompression algorithms were initially based on planned decompression used by our mesophotic dive team and prior work with fish recompression chambers (Smiley and Drawbridge, 2007; Welsh, 2012). The clear walls of the SubCAS allow observation of the fishes, and monitoring and management of stress during decompression. Working pressures were continuously monitored by either a submersible depth gauge (dive computer) located within the chamber(s) and/or the in-line pressure gauge on the system plumbing. Depths and times of decompression were adjusted based on the response of the fishes. If signs of stress, such as a high respiratory rate, listing, or excessive buoyancy were observed, fishes were compressed back down in 3 m increments until they recovered, and then decompression was resumed. Decompression algorithms often also took into account the field schedule: allowing for sleep, meals, and subsequent dives by the support and deep diving team. Water exchanges to dilute nitrogenous wastes were performed by simply removing water from the larger recirculating system and replenishing it with fresh seawater matching the system temperature and salinity. The adjustable pressure control valve creates some back pressure on the system and prevents the SubCAS from ascending fish all the way to the surface. In order to overcome this limitation, on the final decompression stops chamber operators open the bypass valve, which enables a reduction of pressure down to near-ambient conditions. Fishes were considered to have survived decompression if they were alive at the time of their release from the SubCAS.
Following decompression, fishes were released into the recirculating water system, where they were able to swim freely until they were packed for transport back to our museum. Sections of plastic pipe and/or small rocks were placed in the bottom of the aquariums to provide shelter. On shipping day, fishes were packed in plastic bags filled with fresh natural seawater and oxygen in a 1:1 ratio, securely packed in Styrofoam coolers with cardboard outer liners and shipped to the Steinhart Aquarium in San Francisco, CA, USA, via air cargo (~24–36 h total transit time, depending on field site). Ice packs were used to maintain low temperatures (~20°C) during shipping. When they reached our facility, fishes were acclimated to aquarium water by slowly equalizing temperature, pH, and salinity, and then placed into quarantine tanks for 30 days of observation and professional care by our veterinarian and his team. Fishes were considered to have survived decompression and shipping if they were alive upon arrival at our facility.
Specific data for each of the 174 fishes in this study included taxonomic family, genus, and species of the fishes, the location of the expedition, the specific dive site where collection occurred, the collection date, collection depth, decompression event, and the total time for decompression. In order to minimize stress and damage associated with physical handling, the total length of all fishes was visually estimated, and all fishes were placed into three size classes: small (0–5 cm TL), medium (5–10 cm TL) and large (10–15 cm TL). Fishes larger than 15 cm TL were not collected. Collection depth was organized in four zones: 30–60, 60–90, 90–120, and 120–150 m. Percent survival was calculated for all expeditions, for the 27 independent decompression events, for fish families, and for specific genera.
We had detailed time records for 18 of the 27 decompression events included in this study, which were converted to elapsed time (minutes) and depth (meters). In order to visualize if decompression rate impacted survival, total decompression time was organized in three classes for statistical analysis: short (1,000–2,000 min), medium (2,000–4,000 min), and long (4,000–6,500 min). We performed Kruskall–Wallis tests to assess the existence of significant differences in the survivorship among decompression events, taxonomic family, size classes, collection depth and the total time of decompression. We also performed two independent Two-Way Factorial ANOVAs to investigate the interaction between decompression events with both taxonomic family and body size. As the survivorship data does not have a normal distribution (Shapiro–Wilk normality test: W = 0.34854, p < 0.0001), we applied a logarithmic transformation to it to approximate the test assumptions of normality. Analyses were performed in R version 3.4.2 (R Core Team, 2017).
Our portable, submersible decompression chamber, the SubCAS, worked as designed and allowed transport of fishes from mesophotic depths to the surface. Of the 18 decompression events where we have detailed data, the average starting depth was 59.4 m (sd 8.8 m), and the maximum starting depth was 78.8 m. By placing specimens into the SubCAS at these intermediate depths, we were able to decompress fishes collected from up to 150 m deep. During decompression, water is continuously circulated through the SubCAS by the high-pressure pump, providing oxygen and diluting metabolites. By adjusting the high-pressure control valve, we progressively reduce the pressure within the chamber and surface the fishes in a highly controlled manner. The clear plastic body of the SubCAS allows the chamber operators to easily monitor the fishes during decompression and adjust the profile accordingly. The units themselves are robust, compact for transport to remote field sites, easy for the mesophotic divers to operate, and have proven very reliable.
During six mesophotic dive expeditions from 2014 through 2017, we collected 174 fishes representing a minimum of 80 species, 38 or more genera, and 19 families (Table 1; Supplementary Table 2). The most represented families were Serranidae, Labridae, and Pomacentridae, primarily due to our selection criteria and the high abundance of these small, planktivorous fishes on mesophotic reefs (Pinheiro et al., 2016). Decompression profiles varied widely between collecting dives and across all expeditions. In total, the chamber was used for 27 independent decompression events. The detailed data points (depth and elapsed time) taken for 18 of these 27 events are presented in Figure 6. Fishes were decompressed for a minimum of 1,050 min and a maximum of 6,330 min, with an average of 2,597 min (sd 1,272 min). In seven of these 18 events, fish were observed responding negatively to the decrease in pressure (e.g., visibly struggling with buoyancy, or floating at the top of the chamber), so they were brought back down a single 3 m stop and given time to recover. In one instance, there was a power outage that subjected the fishes to rapid pressure loss, as the depth went from 36 to 14 m in 5 min. The system was stabilized within 10 min, the fish were recompressed to 42 m, and then given 400 min to recover before resuming decompression. In the end, 11 of 15 fishes survived the barotrauma associated with this rapid decompression event.
Table 1. Families of mesophotic fishes collected and decompressed using the SubCAS submersible hyperbaric chamber during six research expeditions to remote field sites from 2014 through 2017.
Figure 6. Decompression profiles of 18 independent events on field expeditions to the Philippines, Vanuatu, Palau, and Pohnpei from 2014 through 2017. Black solid lines are decompression events with 100% survival (n = 13). Gray dashed lines indicate decompression events where there was at least one mortality (n = 5). The bar graph (inset) summarizes all 27 independent decompression events in this study. Stacked columns include all 174 fishes decompressed in our study. Black bars indicate success (100% survival, 20 of 27 events) and gray bars illustrate the 7 events where mortalities occurred during decompression.
Upon release from the decompression chamber, some fishes still appeared to be positively buoyant, constantly swimming with their heads down toward the bottom of the tank or resting against the top of plastic pipes or other hiding structures placed within their holding tanks. This behavior occurred with 17 out of 174 specimens (~10%). Such situations required medical intervention in only two cases. A Brotula multibarbata (Ophidiidae) was vented immediately after release from the chamber due to being excessively buoyant. On one dive, a SubCAS was improperly sealed and decompressed upon ascent, resulting in a ruptured swim bladder in a Sacura speciosa (Serranidae). After this fish had been shipped back to our museum, it was anesthetized and the gas was aspirated with a hypodermic syringe. This fish is currently still alive in our aquarium, 3 years after collection. In all other cases, fishes that were slightly buoyant were placed in plastic boxes or plastic pipe sections and submerged in the holding tanks where they recovered on their own over a period of several days to weeks. In total, 148 specimens were shipped from remote field sites in Vanuatu, the Philippines, Palau and Pohnpei to our museum in San Francisco, with transport times as long as 36 h.
Overall, 155 specimens (89%) survived collection and decompression. Survival differs significantly among decompression events (H-test, X2 = 56.277, df = 26, p < 0.001), and taxonomic family (H-test, X2 = 22.045, df = 6, p = 0.001), where Pomacentridae and Apogonidae presented the lowest survival rates (Figure 7). Survival was not significantly different among depth of collection, fish body size, or time of decompression; all variables exhibit high survival rates (Figures 8A–C). The Two-Way Factorial ANOVA test identified significant interactions between decompression events and both fish body size and taxonomic family (Table 2), indicating the lower survivorship was specific to certain chamber trials. From the 148 shipped specimens, 143 survived the packing and air cargo transport, representing 96.6% survivorship.
Figure 7. Survival rates of the main families of reef fishes decompressed through the chamber. Only families with sample sizes of more than three specimens are presented.
Figure 8. Survival rates of reef fishes decompressed with the SubCAS chamber. (A) Depth range of collection; (B) Fish size classes: small (0–5 cm TL), medium (5–10 cm TL) and large (10–15 cm TL); (C) Decompression time: short (1,000–2,000 min), medium (2,000–4,000 min) and long (4,000–6,500 min).
Table 2. Summary of Two-Way Factorial ANOVA, testing interaction between decompression events and (A) taxonomic family and (B) body size (visually estimated in 3 size classes).
The SubCAS is a proven method to collect a broad taxonomic range of reef fishes from mesophotic depths and safely bring them to ambient pressure at the surface. This approach has worked across several remote field locations, facilitating the acquisition of specimens for scientific research and education in our aquarium. Specimens from 16 of the 19 families represented in our study had 90% survival or greater through collection, decompression and transport. A total of 155 of the 174 specimens collected survived decompression in the SubCAS.
Sixteen of the 19 mortalities in our study can be explained by various external factors, including injury during collection, predation, or aggression while undergoing decompression, unexpected power outages or poor water quality. The single mortality within the Labridae, Cirrhilabrus roseafascia, became tangled in a monofilament net during collection and was damaged; this fish likely died from factors unrelated to decompression. A slopefish, Symphysanodon sp., also died after being released from the chamber; likely a result of physical damage during collection. Two specimens of Pseudanthias flavoguttatus were eaten by a Cephalopholis igarashiensis inside the chamber, and a Centropyge multicolor died from intraspecific aggression while in the chamber: two examples of specimen incompatibility. In addition, eight specimens perished from the first collection in Vanuatu, probably due to the unexpected low salinity and high temperature of the source water. This single episode impacted overall survival within the Pomacentridae and Apogonidae, as these fishes were disproportionately represented in this event. Also in Vanuatu, three fish died in a chamber that rapidly decompressed due to a sudden power outage. The single lizardfish (Synodontidae) in our study jumped out of the holding tank during the packing and shipping process and was lost. If we remove these incidents, all of which are not actually the direct result of barotrauma, but rather the varying conditions and often unpredictable circumstances of remote field operations, our total overall survival through decompression increases to 98.7%. Our statistical analysis supports this conclusion. We found significant differences among individual decompression events, and significant interactions between decompression event and both size class and taxonomic family. Therefore, the lower survivorship in taxonomic family and size class is associated with the various external factors described above.
Incompatible fishes should not be mixed within the SubCAS, as there is no way for them to hide or escape aggression. Opening the chamber to separate them is not an option as it would result in rapid, extreme barotrauma and (likely) death. Three specimens died from either predation or intraspecific aggression while decompressing in the SubCAS. A potential solution to these losses is to segregate the fishes within the inner collecting jar with partitions, perhaps with a series of slightly smaller openings, or by creating a segmented inner collecting jar with individual compartments. One potential drawback to this approach is increased complexity of operation by a mesophotic diver already taxed by multiple tasks.
Fishes of the families Pomacentridae and Apogonidae did not fare well with our methodology. Our statistical analysis revealed an interaction between the lower survivorship in these families and the individual decompression events, but there may be other contributing factors. It is possible that this is related to specific aspects of the physiology or anatomy of these families, or varying sensitivity to being contained in a tight environment through decompression. When attempts to decompress deep-sea grenadiers (C. acrolepis) failed, the authors suspected that increases in temperature or the relative concentration of oxygen may have played a role (Wilson and Smith, 1985). We also observed mortality that may have been associated with poor source water quality, where we suspect that low salinity and high temperature caused eight fishes, all within the Pomacentridae or Apogonidae, to perish. Nevertheless, relatively higher incidences of barotrauma have been observed in these two families following international shipping for the marine aquarium trade (Tom Bowling, personal communication). Additional trials need to be conducted to determine the root causes of our lack of success with these two families, which may be related to acclimation responses independent of swim bladder dynamics.
The methods described in this paper enable divers to safely decompress reef fishes from remote mesophotic coral ecosystems so that live specimens are available for research and education. This important innovation allows for detailed ex situ studies of deep reef fishes, including physiology, behavior, reproductive biology, and longevity. Our fish decompression chamber has high survival across a diversity of fish families, size classes, collection depths, and decompression profiles. The lack of significant impact of varying decompression profiles on survival of fishes indicates that the chambers can be used with the decompression schedule that is most convenient to the daily operations of the field team.
One of the great advantages of our design is the clear chamber housing and collection jar, which allows the operator to view the fishes during their lengthy decompression. Fish should be regularly monitored during decompression. If they show any signs of stress or excessive buoyancy in the chamber, the profile should be adjusted by dropping them by ~3 m depth and giving them time to recover. This approach contrasts with prior attempts, where a stepwise decompression was conducted by reducing pressure until the fish became positively buoyant and started listing. The fish was then maintained at this pressure until it righted itself and once again attained neutral buoyancy, after which pressure was further reduced in the same manner (Wilson and Smith, 1985). We suspect that repeated episodes of excessive buoyancy may damage the blood vessels in the swim bladder, reducing the fish's ability to remove the gases through physiological processes. We have also seen at least one instance where rapid, accidental decompression led to a ruptured swim bladder, with gas escaping into the coelomic cavity where it cannot be physiologically absorbed.
Because the study of MCEs is a recent area of focus for coral reef science, the potential to study living fishes from these depths, exhibit them in public museums, and use them as a tool to promote educational outreach is an important innovation supporting public awareness during a time when coral reefs are in a state of global crisis. The need for increased awareness is especially the case with MCEs, which are rarely included in marine protected areas or sanctuaries, and are often subject to the same natural and anthropogenic stressors that are facing the better-known shallow coral reef ecosystems. The SubCAS, when deployed properly, is a highly successful and relatively easy approach to decompressing mesophotic fishes for research, outreach and education, and other activities where working with living specimens is required.
The fishes in this study have been engaging more than one million members of the public each year in our Twilight Zone: Deep Reefs Revealed exhibit at the California Academy of Sciences. They are maintained at ambient pressure under carefully controlled environmental conditions in a series of display aquariums ranging from 28 to 5,300 L. At the time of publication, several specimens described in this study have been in human care for 3 years, and have acclimated well to public aquarium conditions: feeding primarily on frozen foods, and living under increased light levels with busy crowds visiting the gallery. Our exhibit is well attended and has high guest satisfaction. Surveys completed by 964 visitors during July-September 2016 report a 77% overall satisfaction rating: the highest of any new exhibit since our new museum opened in 2008. These surveys also indicate that the Twilight Zone exhibit effectively communicates the importance of coral reefs (89% of guests reporting “very well” to “somewhat” effective), the causes of the problems facing coral reefs (83%) and actions to sustain coral reefs (79%). Interestingly, 38% of guests stated that the fish decompression chamber was their favorite part of the exhibit. Twilight Zone: Deep Reefs Revealed won an innovation award from the Association of Zoos and Aquariums in September 2017. In addition, the SubCAS was used as an example of a real-life application of physics in an online video, Bringing Fish Up From The Deep (https://ww2.kqed.org/quest/2015/03/05/bringing-fish-up-from-the-deep/), produced in partnership with KQED Quest.
The raw data supporting the conclusions of the manuscript will be made available by the authors, without undue reservation, to any qualified researcher.
This study was carried out with the approval of the Steinhart Aquarium Research Committee. Fishes were collected according to protocols approved by the Institutional Animal Care and Use Committee of the California Academy of Sciences.
MW designed several iterations of the SubCAS chamber and developed the operational protocols. BS, HP and LR conducted the diving and collected the mesophotic fishes. BS and HP analyzed the data. BS, MW, HP, and LR wrote and edited the manuscript and created the figures and tables.
The authors declare that the research was conducted in the absence of any commercial or financial relationships that could be construed as a potential conflict of interest.
We are grateful for the generous support of donors to the California Academy of Sciences' Hope for Reefs Initiative who helped make this study possible. This research was also supported by grant DEB 12576304 from the National Science Foundation to Terry Gosliner, Rich Mooi, LR, and Gary Williams. All activities in the Philippines were conducted under Gratuitous Permits GP-0077-14, GP-0085-15, GP-0112-16, GP-0124-17, under the supervision by partners from BFAR FRQD and NFRDI. We sincerely thank several colleagues in the Philippines who have contributed to our research over the years, including November Romena and Luvi Labe. We also thank our colleagues who served as chamber technicians and provided expert care to the fishes collected on the six field expeditions: Cristina Castillo, J. Charles Delbeek, Allan Jan, Pam Montbach, Richard Ross, Margarita Upton, and Nick Yim. Joe Welsh advised and supported us during the early stages of this project, and Toshiro Chang and the California Academy of Sciences' electronics engineering team assisted with the development of several crucial aspects of the chamber design. The Academy Scientific Diving department, especially Mauritius V. Bell, Elliott Jessup, Mark Lane, Narineh Nazarian and Will Love supported safe field operations during our continual quest to go deeper in more and more remote locations. Tom Bowling, Grant Norton, Michael Carpenter, Allois Malfitani, Simon Ellis and Mark Figueras graciously hosted us during field operations. Brian Greene and Rich Pyle taught us much of what we know about catching fishes while diving at mesophotic depths. Freeland Dunker, DVM and the Steinhart Aquarium Animal Health Department, especially Carissa Mendoza, provided excellent care for these fishes during their quarantine period. We thank the three reviewers for greatly improving the manuscript, especially concerning our statistical model used for data analysis.
The Supplementary Material for this article can be found online at: https://www.frontiersin.org/articles/10.3389/fmars.2018.00187/full#supplementary-material
Ballard, J. (2008). Therapeutic pressure chambers for fish. Drum and Croaker. 39, 40–46. Available online at: http://drumandcroaker.org/pdf/2008.pdf
Bongaerts, P., Ridgway, T., Sampayo, E. M., and Hoegh-Guldberg, O. (2010). Assessing the ‘deep reef refugia' hypothesis: focus on Caribbean reefs. Coral Reefs 29, 309–327. doi: 10.1007/s00338-009-0581-x
Brown, I. W., Sumpton, W., McLennan, M. F., Mayer, D. G., Campbell, M., Kirkwood, J., et al. (2010). An improved technique for estimating short-term survival of released line-caught fish, and an application comparing barotrauma-relief methods in red emperor (Lutjanus sebae Cuvier 1816). J. Exp. Mar. Biol. Ecol. 385, 1–7. doi: 10.1016/j.jembe.2010.01.007
Drumhiller, K. L., Johnson, M. W., Diamond, S. L., Reese Robillard, M. M., and Stunz, G. W. (2014). Venting or rapid recompression increase survival and improve recovery of red snapper with barotrauma. Mar. Coast. Fish. 6, 190–199. doi: 10.1080/19425120.2014.920746
Gilmore, R. G. Jr. (2016). You can't catch a fish with a robot. Gulf Caribbean Res. 27:1. doi: 10.18785/gcr.2701.11
Jarvis, E. T., and Lowe, C. G. (2008). The effects of barotrauma on the catch and release survival of southern California nearshore and shelf rockfish (Scorpaenidae, Sebastes spp.) Can. J. Fish. Aquat. Sci. 65, 1286–1296. doi: 10.1139/F08-071
Kane, C., Kosaki, R. K., and Wagner, D. (2014). High levels of mesophotic reef fish endemism in the Northwestern Hawaiian Islands. Bull. Mar. Sci. 90, 693–703. doi: 10.5343/bms.2013.1053
Koyama, S., Miwa, T., Horii, M., Ishikawa, Y., Horikoshi, K., and Aizawa, M. (2002). Pressure-stat aquarium system designed for capturing and maintaining deep-sea organisms. Deep Sea Res. I. 49, 2095–2102. doi: 10.1016/S0967-0637(02)00098-5
Loya, Y., Eyal, G., Treibitz, T., Lesser, M. P., and Appeldoorn, R. (2016). Theme section on mesophotic coral ecosystems: advances in knowledge and future perspectives. Coral Reefs 35, 1–9. doi: 10.1007/s00338-016-1410-7
Munday, E. S., Tissot, B. N., Heidel, J. R., and Miller-Morgan, T. (2015). The effects of venting and decompression on Yellow Tang (Zebrasoma flavescens) in the marine ornamental aquarium fish trade. Peer J. 3:e756. doi: 10.7717/peerj.756
Nguyen, V., Gravel, M., Mapleston, M., Hanson, K. C., and Cooke, S. J. (2009). The post-release behavior and fate of tournament-caught smallmouth bass after ‘fizzing' to alleviate distended swim bladders. Fish. Res. 96, 313–318. doi: 10.1016/j.fishres.2008.12.003
Pinheiro, H. T., Goodbody-Gringley, G., Jessup, M. E., Shepherd, B., Chequer, A. D., and Rocha, L. A. (2016). Upper and lower mesophotic coral reef fish communities evaluated by underwater visual censuses in two Caribbean locations. Coral Reefs 35, 139–151. doi: 10.1007/s00338-015-1381-0
Pinheiro, H. T., Mazzei, E., Moura, R. L., Amado-Filho, G. M., Carvalho-Filho, A., Braga, A. C., et al. (2015). Fish biodiversity of the Vitória-Trindade Seamount Chain, southwestern Atlantic: an updated database. PLoS ONE 10:e0118180. doi: 10.1371/journal.pone.0118180
Pribyl, A., Kent, M., Parker, S., and Shreck, C. (2011). The response to forced decompression in six species of Pacific rockfish. Trans. Am. Fish. Soc. 140, 374–383. doi: 10.1080/00028487.2011.567858
Pyle, R. L. (2000). Assessing undiscovered fish biodiversity on deep coral reefs using advanced self-contained diving technology. Mar. Technol. Soc. J. 34, 82–91. doi: 10.4031/MTSJ.34.4.11
Pyle, R. L., and Kosaki, R. K. (2016). Prognathodes basabei, a new species of butterflyfish (Perciformes, Chaetodontidae) from the Hawaiian archipelago. Zookeys 614, 137–152. doi: 10.3897/zookeys.614.10200
R Core Team (2017). R: A Language and Environment for Statistical Computing. Vienna: R Foundation for Statistical Computing. Available online at: http://www.R-project.org/
Rocha, L. A., Pinheiro, H. T., Wandell, M., Rocha, C. R., and Shepherd, B. (2017). Roa rumsfeldi, a new butterflyfish (Teleostei, Chaetodontidae) from mesophotic coral ecosystems of the Philippines. ZooKeys 709, 127–134. doi: 10.3897/zookeys.709.20404
Rogers, S. G., Langston, H. T., and Targett, T. E. (1986). Anatomical trauma to sponge- coral reef fishes captured by trawling and angling. U.S. Natl. Mar. Fish. Serv. Fish. Bull. 84, 697–704.
Rummer, J. L., and Bennett, W. A. (2005). Physiological effects of swim bladder overexpansion and catastrophic decompression on red snapper. Trans. Am. Fish. Soc. 134, 1457–1470. doi: 10.1577/T04-235.1
Sanborn, K. L., Webster, J. M., Yokoyama, Y., Dutton, A., Braga, J. C., Clague, D. A., et al. (2017). New evidence of Hawaiian coral reef drowning in response to meltwater pulse-1A, Q. Sci. Rev. 175, 60–72. doi: 10.1016/j.quascirev.2017.08.022
Sato, K., Kaneko, A., and Toda, M. (2010). “Using the SGPT chamber for field sampling and long-term captivity of deep demersal fishes from tropical waters,” in Proceedings of an International Symposium Into the Unknown, Researching Mysterious Deep-Sea Animals, ed S. Uchida (Okinawa), 137–143.
Simon, T., Pinheiro, H. T., Moura, R. L., Carvalho-Filho, A., Rocha, L. A., Martins, A. S., et al. (2016). Mesophotic fishes of the Abrolhos Shelf, the largest reef ecosystem in the South Atlantic. J. Fish Biol. 89, 990–1001. doi: 10.1111/jfb.12967
Slattery, M., Lesser, M. P., Brazeau, D., Stokes, M. D., and Leichter, J. J. (2011). Connectivity and stability of mesophotic coral reefs. J. Exp. Mar. Bio. Ecol. 408, 32–41. doi: 10.1016/j.jembe.2011.07.024
Smiley, J., and Drawbridge, M. (2007). Techniques for live capture of deepwater fishes with special emphasis on the design and application of a low-cost hyperbaric chamber. J. Fish Biol. 70, 867–878. doi: 10.1111/j.1095-8649.2007.01347.x
van Oppen, M. J., Bongaerts, P., Underwood, J. N., Peplow, L. M., and Cooper, T. F. (2011). The role of deep reefs in shallow reef recovery: an assessment of vertical connectivity in a brooding coral from west and east Australia. Mol. Ecol. 20, 1647–1660. doi: 10.1111/j.1365-294X.2011.05050.x
Wagner, D., Kosaki, R. K., Spalding, H. L., Whitton, R. K., Pyle, R. L., Sherwood, A. R., et al. (2014). Mesophotic surveys of the flora and fauna at Johnston Atoll, Central Pacific Ocean. Mar. Biodiv. Records 7:e68. doi: 10.1017/S1755267214000785
Welsh, J. (2012). “Hyperbaric chambers for rockfish (Northeast Pacific Sebastes),” in Diving for Science 2012: Proceedings of the American Academy of Underwater Sciences 31st Symposium, eds D. Steller and L. Lobel (Dauphin Island, AL: AAUS), 129–134.
Keywords: mesophotic coral ecosystems, coral reefs, barotrauma, aquarium, decompression, reef fishes
Citation: Shepherd B, Wandell M, Pinheiro HT and Rocha LA (2018) SubCAS: A Portable, Submersible Hyperbaric Chamber to Collect Living Mesophotic Fishes. Front. Mar. Sci. 5:187. doi: 10.3389/fmars.2018.00187
Received: 26 January 2018; Accepted: 09 May 2018;
Published: 04 June 2018.
Edited by:
Yehuda Benayahu, Tel Aviv University, IsraelReviewed by:
Aldo Cróquer, Simón Bolívar University, VenezuelaCopyright © 2018 Shepherd, Wandell, Pinheiro and Rocha. This is an open-access article distributed under the terms of the Creative Commons Attribution License (CC BY). The use, distribution or reproduction in other forums is permitted, provided the original author(s) and the copyright owner are credited and that the original publication in this journal is cited, in accordance with accepted academic practice. No use, distribution or reproduction is permitted which does not comply with these terms.
*Correspondence: Bart Shepherd, YnNoZXBoZXJkQGNhbGFjYWRlbXkub3Jn
†Present Address: Matt Wandell, Monterey Bay Aquarium, Monterey, CA, United States.
Disclaimer: All claims expressed in this article are solely those of the authors and do not necessarily represent those of their affiliated organizations, or those of the publisher, the editors and the reviewers. Any product that may be evaluated in this article or claim that may be made by its manufacturer is not guaranteed or endorsed by the publisher.
Research integrity at Frontiers
Learn more about the work of our research integrity team to safeguard the quality of each article we publish.