- IRCM, Institut de Recherche en Cancérologie de Montpellier, INSERM U1194, Université de Montpellier, Institut Régional du Cancer de Montpellier, Montpellier, France
The tumor immune microenvironment contributes to tumor initiation, progression and response to therapy. Among the immune cell subsets that play a role in the tumor microenvironment, innate-like T cells that express T cell receptors composed of γ and δ chains (γδ T cells) are of particular interest. Indeed, γδ T cells contribute to the immune response against many cancers, notably through their powerful effector functions that lead to the elimination of tumor cells and the recruitment of other immune cells. However, their presence in the tumor microenvironment has been associated with poor prognosis in various solid cancers (breast, colon and pancreatic cancer), suggesting that γδ T cells also display pro-tumor activities. In this review, we outline the current evidences of γδ T cell pro-tumor functions in human cancer. We also discuss the factors that favor γδ T cell polarization toward a pro-tumoral phenotype, the characteristics and functions of such cells, and the impact of pro-tumor subsets on γδ T cell-based therapies.
Introduction
Within a tumor, the malignant features of cancer cells are tightly regulated by their local environment and the reciprocal network they form with host cells (e.g., immune cells, angiogenic vascular cells, endothelial cells, and cancer-associated fibroblasts) and that define the cancer ecosystem. The tumor immune microenvironment is a critical determinant of cancer evolution and outcome. In this context, the nature and frequency of tumor-infiltrating immune cells are considered to be prognostic factors in many cancers. A better knowledge of this dynamic immune environment is required to improve prognosis, choose therapies, and evaluate the response to treatments.
Among the tumor-infiltrating immune cells, T cell sub-populations, especially CD8+ T lymphocytes, are a key anti-tumor immune component. γδ T cells, a subgroup of T cells that belong to the non-conventional or innate lymphocyte family, also are found in the tumor microenvironment and are involved in tumor surveillance. Although they share many properties with αβ T cells, such as cytotoxic activity and pro-inflammatory cytokine production, the structure of their T cell receptor (TCR; composed of γ and δ chains) is different as well as their activation mechanisms that are independent of major histocompatibility complex (MHC) molecules. Human γδ T cells can be divided in three main populations, based on their TCR δ chain (δ1, δ2, δ3) (1, 2). Vδ2 T cells, also known as Vγ9Vδ2 T cells, are the main γδ T subtype (90%) in peripheral blood. The Vδ1 and Vδ3 subsets are mostly found in tissues and mucosa, respectively.
Vγ9Vδ2 T cells display specific properties, such as the TCR-dependent recognition of non-peptidic phosphorylated antigens, called phosphoantigens. Phosphoantigens are molecules produced by the isoprenoid synthesis pathways of prokaryotic pathogens and by infected or transformed eukaryotic cells. Although phosphoantigen recognition does not require MHC molecule presentation, several studies brought evidences of the involvement of the cell surface butyrophilin 3A (BTN3A) (3) and the requirement of butyrophilin 2A1 (BTN2A1) (4). Phosphoantigen-induced TCR activation of Vγ9Vδ2 T cells triggers their proliferation, cytokine production, and cytotoxic activity (5). Vγ9Vδ2 T cells also express natural killer (NK) receptors, such as NKG2A and NKG2D, and their activation is modulated by the presence of their ligands in the environment (6, 7).
Vδ1 T cells recognize the stress-inducible MHC class I-related chain A and B (MICA and MICB) proteins that are expressed by some tumor and virus-infected cells (8), as well as glycolipid antigens presented by the CD1c (9) and CD1d proteins (10, 11), and the algal protein phycoerythrin (12). Additionally, Vδ1 T cells can be activated independently of their TCR, via ligation of stimulatory receptors, including NKG2C, NKG2D, NKp30, toll-like receptors, and the β-glucan receptor dectin 1 (13–17). To date, little is known on the activation mechanisms of the Vδ3 T cell subset.
Although the human Vδ1, Vδ2 and Vδ3 T cell subsets display a strong reactivity against tumor cells, γδ T cell-based immunotherapies primarily target the Vδ2 subset because they are easily expanded and activated by synthetic clinical-grade phosphoantigens (e.g., bromohydrin pyrophosphate) or by pharmacological inhibitors (e.g., zoledronate) of the isoprenoid synthesis pathway that produces these metabolites (18, 19).
Many clinical trials using Vγ9Vδ2 T cells have been carried out. Although their safety have been proven, response rate was moderate and only in 10–33% of patients with hematologic and solid malignancies benefit from Vγ9Vδ2 T cell-based immunotherapies (20–25). This suggests the presence in the tumor microenvironment (TME) of suppressive mechanisms that inhibit/divert Vγ9Vδ2 T cell functions and/or their ability to infiltrate tumors. New tools to target and boost Vγ9Vδ2 T cell anti-tumor functions are currently under study (26), while other γδ T cell subtypes (e.g., Vδ1 T cells) are now tested as new therapeutic candidates (27). Although therapies using γδ T cells received a new burst of interest due to these new research axes, the existence of γδ T cell subsets with pro-tumor functions has also been suggested.
In this review, we will discuss the evidences concerning γδ T cell pro-tumor functions in human cancer, and the factors that could favor γδ T cell polarization toward a pro-tumoral phenotype, the characteristics and functions of these cells, and also the possible consequences for γδ T cell-based therapies.
Evidence of Pro-tumoral γδ T Cells in Human Cancer (Table 1)
In line with the potent anti-tumor properties of γδ T cells, a large study of publicly available gene expression data from bulk tumors showed that the γδ T cell signature is associated with the most significant favorable prognosis in 25 malignancies (37). However, it was later demonstrated that the sorting algorithm used in this study could not accurately differentiate γδ T cells from CD8+ and NK cells due to the transcriptome overlaps in these three cell types (38). Using a refined signature for the Vγ9Vδ2 T cells subset based on sorted cells, the authors found that a high-level infiltration of γδ T cells in tumors was not always associated with a positive outcome (38). In line with these results, recent studies suggested that these cells may also have a pro-tumor role in some cancers.
In breast cancer, high Vδ1 T cell prevalence has been associated with immunosuppressive functions, such as inhibition of naive T cell proliferation and the impairment of dendritic cell (DC) maturation and function (28). Moreover, γδ T cell infiltration level in breast cancer was the most significant independent prognostic factor of disease severity, in terms of survival and relapse (29).
In colorectal cancer, CD39+ Vδ1 T cell infiltration establishes an immunosuppressive microenvironment through the adenosine pathway and the recruitment of myeloid-derived suppressive cells (MDSCs). The presence of these cells has been associated with the disease severity (31). Another study demonstrated the pro-tumor functions of IL-17-producing γδ T cells in colon cancer through their capacity to recruit MDSCs (33). Moreover, pro-inflammatory Vδ2 T cells might participate in colorectal cancer pathogenesis by supporting chronic inflammation (39). Besides breast and colon cancer, several studies have shown a potentially deleterious role of γδ T cell subsets in pancreatic, ovarian, gallbladder and renal cancer (32, 34–36).
Polarization of γδ T Cells Toward a Pro-tumor Functional Phenotype (Figure 1)
Although γδ T cells have been originally described as pro-inflammatory cells with a Th1-like phenotype, they display high plasticity and can be polarized toward different functional phenotypes, depending on their environment (40). Understanding precisely the influence of different environmental factors, such as cytokines, on γδ T cells and the limits of their plasticity is crucial to determine how the TME can skew γδ T cells toward a pro-tumor function that will directly or indirectly impair the anti-tumor immune response and support tumor growth. Although studying T cell functional plasticity within tumors is a complex endeavor, several ex vivo studies involving the activation of naive γδ T cells in the presence of various cytokines have brought some insights into how γδ T cells can be skewed toward a pro-tumoral activity. Specifically, it has been shown that TGF-β, IL-4 and more recently IL-21 favor the acquisition of pro-tumoral properties by human and mouse γδ T cells. Moreover, various cytokine combinations can polarize γδ T cells into Th17-like cells with pro-tumor effects.
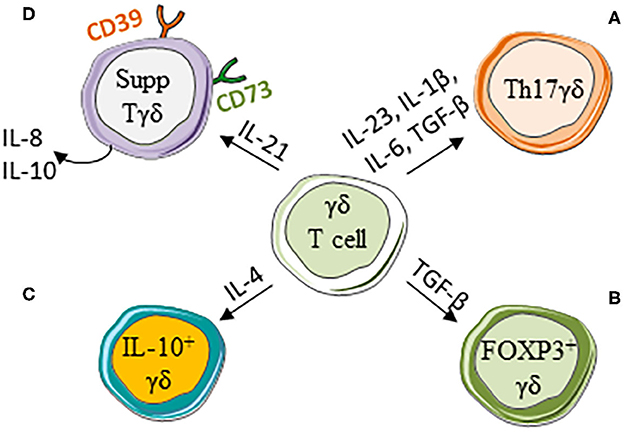
Figure 1. γδ T cell polarization into pro-tumor cells. Cytokines present in the tumor microenvironment induce the differentiation of γδ T cells into pro-tumor cells: (A) Th17-like γδ T cells (Th17 γδ), (B) FOXP3+γδ T cells (FOXP3+γδ), (C) IL-10-producing γδ T cells (IL-10+ γδ), and (D) regulatory γδ T cells that express CD39 and/or CD73 (Supp γδ).
TGF-β
TGF-β is a pleiotropic cytokine that is produced by most cells in a latent form. TGF-β1 (subsequently referred to as TGF-β), the most studied isoform, is a potent suppressor of the immune system. It can be secreted in a complex with latent TGF-beta binding proteins (LTBP) and deposited in the extracellular matrix, or tethered to the surface of cells when bound in a covalent manner to glycoprotein A repetitions predominant (GARP) or leucine-rich-repeat-containing protein 33 (LRRC33). Active TGF-β needs to be released from the latent complex through the interaction with other partners, such as integrins, to act on its target cells through binding to TGF-β receptors (41, 42). TGF-β can induce the differentiation of naive CD4+ T cells into regulatory T cells (Tregs) or Th17 cells, depending on the context, and is often enriched in tumors. Therefore, TGF-β could play a crucial role in γδ T cell polarization toward pro-tumoral cells in the TME (43, 44). In vitro, human peripheral blood mononuclear cells (PBMCs) can be stimulated with phosphoantigens and cultured with IL-2 to selectively expand Vγ9Vδ2 T cells. Addition of TGF-β to the culture increases FOXP3 expression in these cells. FOXP3 expression remains stable for at least 10 days. Sorted FOXP3+ Vγ9Vδ2 T cells inhibit the proliferation of TCR-stimulated PBMCs (45). Another study confirmed TGF-β role in the development of FOXP3+ Vγ9Vδ2 T cells and demonstrated that decitabin, a DNA hypomethylating agent, promotes the generation and the immunosuppressive activity of FOXP3+ Vγ9Vδ2 T cells induced by TGF-β (46). Importantly, the relevance of FOXP3 as a regulatory marker depends on the type of stimulation. Indeed, Vδ2 cell activation using anti-CD3 and anti-CD28 antibodies instead of phosphoantigens leads to transient FOXP3 expression that does not correlate with the regulatory phenotype (47, 48). Interestingly, vitamin C increases the stability of TGF-β-induced FOXP3 expression in Vδ2 cells through an epigenetic modification of the FOXP3 gene, and enhances their suppressive capacities (49). Li et al. demonstrated that upon TCR stimulation Vδ1 T cells can be polarized toward a suppressive phenotype in the presence of IL-2 and TGF-β. These Vδ1 cells express FOXP3 and suppress the proliferation of activated CD4+ T cells (50). In human colorectal cancer, tumor-infiltrating CD39+ γδ T cells were described as regulatory γδ T cells that express FOXP3 and act mainly through the adenosine pathway (31). The authors found that TGF-B1 mRNA level is higher in the tumor than in the associated normal tissue. Moreover, CD39+ γδ T cells from normal tissue incubated with tumor supernatant acquire a potent suppressive capacity through increased adenosine production. This effect can be abrogated by incubation with an anti-TGF-β antibody, and can be reproduced by stimulating cells with recombinant TGF-β. TGF-β-induced polarization of γδ T cells toward FOXP3+ suppressive cells was also demonstrated in the mouse (51). Additionally, TGF-β is required for the polarization of Vγ9Vδ2 into IL-17-producing γδ T cells, together with IL-1β, IL-6 and IL-23, as described below (52). Overall, these results suggest that TGF-β could be one of the key factors responsible for conversion of γδ T cells into suppressive and/or IL-17-producing cells.
IL-4
IL-4 is a potent regulator of the humoral response and more generally of the adaptive immunity, particularly through the differentiation of naive T cells into Th2 cells. In cancer, IL-4 has been associated with tumor aggressiveness, and IL-4 pathway blockade is currently investigated as anti-cancer strategy (53). IL-4 is often enriched in the microenvironment of human solid tumors, notably in cancers with high γδ T cell infiltration, such as breast cancer (54). In vitro, human Vδ2 cells isolated from peripheral blood and activated by phosphoantigens in the presence of IL-4 produce low levels of interferon γ (IFN-γ) and high levels of IL-4, although this production is not stable over time (55). In a more recent study, Mao et al. showed that IL-4 inhibits in vitro the activation of blood γδ T cells induced by TCR stimulation (54). Nevertheless, IL-4 promotes the growth of activated γδ T cells and increases the levels of Vδ1 T cells, which in turn inhibit Vδ2 T-cell growth via significant IL-10 secretion (54). IL-4 inhibits γδ T cell activation when present at the moment of the stimulation, but enhances their proliferation when added later. Moreover, concanavalin A-stimulated Vδ1 T cells cultured with IL-4 retain their cytotoxic properties against tumor cells. This suggests a complex and context-dependent role of IL-4 in γδ T cell polarization (56).
IL-21
IL-21 is a potent immunomodulatory cytokine, mainly produced by activated CD4+ T cells and NKT cells. IL-21 enhances the effector functions of NK cells, helper CD4+ T cells and cytotoxic T cells (CTL), but also inhibits Tregs (57). Therefore, it is often defined as a pro-inflammatory cytokine. In colorectal cancer, IL-21 is strongly associated with chronic inflammatory colitis that precedes the malignant disease (57–59). A similar pro-inflammatory effect of IL-21 on γδ T cells was initially described. Upon in vitro expansion with IL-21, human Vγ9Vδ2 cells display increased levels of granzyme B and increased production of IFN-γ after activation, resulting in enhanced cytotoxic activity toward tumor cells (60). However, IL-21 modulatory role may depend on the cell type and the duration of the exposure. For example, IL-21 enhances IL-10 production by regulatory B cells and their proliferation. Similarly, our group recently found that IL-21 is implicated in the polarization of human Vγ9Vδ2 T cells and Vδ1 T cells toward a regulatory phenotype (30, 61). We isolated a subpopulation of CD73+ regulatory Vγ9Vδ2 T cells following their expansion in the presence of IL-21. We demonstrated that this subset can synthetize adenosine through CD73 enzymatic activity, and produces the suppressive cytokine IL-10 and the chemokine IL-8 (also known as CXCL8) that is involved in the recruitment of polymorphonuclear leukocytes (PMN)-MDSCs. This CD73+ cell subpopulation can suppress the T cell immune response directly in an adenosine- and IL-10-dependent manner, and indirectly by impairing DC antigen presentation (61). We then extended these observations to Vδ1 T cells. We identified in the blood of healthy donors a Vδ1 T cell subpopulation that expresses CD73 and displays immunosuppressive phenotype and functions (i.e., production of immunosuppressive molecules, such as IL-10, adenosine and IL-8). As shown for Vγ9Vδ2 T cells, incubation with IL-21 favors the development and amplification of this Vδ1 subset. Importantly, we detected CD73+ γδ T cells in breast cancer biopsies, suggesting that they could interfere with the anti-tumor response (30). Moreover, in mouse γδ T cells, CD73 expression is increased after exposure to IL-21, suggesting that this polarization could be a common mechanism among different species (61). Interestingly, after infection with Mycobacterium bovis Bacillus Calmette-Guerin (BCG), the number of IL-17-producing γδ T cells was higher in IL-21 receptor knockout mice than wild type animals. IL-21 induces the apoptosis of these cells, suggesting the existence of a balance between IL-21-induced regulatory γδ T cells and IL-17-producing γδ T cells, at least in some contexts (62).
Polarization Into Th17-Like Cells
IL-17 production was first described in helper CD4+ cells, called Th17 cells. Th17 cell cytokine secretion, transcription regulation and effects on the immune system are now well-characterized. Their development is controlled by the transcription factors RORγt (63) and STAT3, and also by IRF4 in some cases when the differentiation is induced by cytokines (64). In mice, TGF-β, IL-6, IL-21 and IL-23 play a critical role in the differentiation or polarization of CD4+ cells into Th17 cells. In humans, IL-1 and IL-23 seem to have the most important role in Th17 cell differentiation, followed by TGF-β and IL-6 (65–67). IL-17 is produced by murine γδ T cells (68) and also by human γδ T cells (69). In both species, IL-7 strongly promotes the expansion of IL-17-producing γδ T cells (Th17 γδ T cells) (70). Moreover, several studies have shown that when cultured in the presence of various cytokine combinations, naive Vγ9Vδ2 T cells acquire an IL-17-secreting Th17-like phenotype or a mixed Th1/Th17 phenotype, and produce both IFN-γ and IL-17 (52, 71, 72). Human cord blood-derived Vγ9Vδ2 T cells stimulated with the phosphoantigen (E)-4-hydroxy-3-methyl-but-2-enyl pyrophosphate (HMBPP) require IL-6, IL-1β and TGF-β to differentiate into Th17 γδ cells, and also IL-23 for differentiation into γδ Th1/Th17 cells (71, 72). In adults, differentiation of naive γδ T cells into memory γδ Th1/Th17 T cells and Th17 γδ T cells requires IL-23, IL-1β and TGF-β, but not IL-6. γδ Th17 cells can also produce IL-22 (especially cells in the cord blood) (71, 72). The pro-tumor role of IL-17 has been well established in some contexts, and the pro-tumor role of Th17 γδ T cells will be developed in the next part.
Pro-tumoral Functions of γδ T Cells (Figure 2)
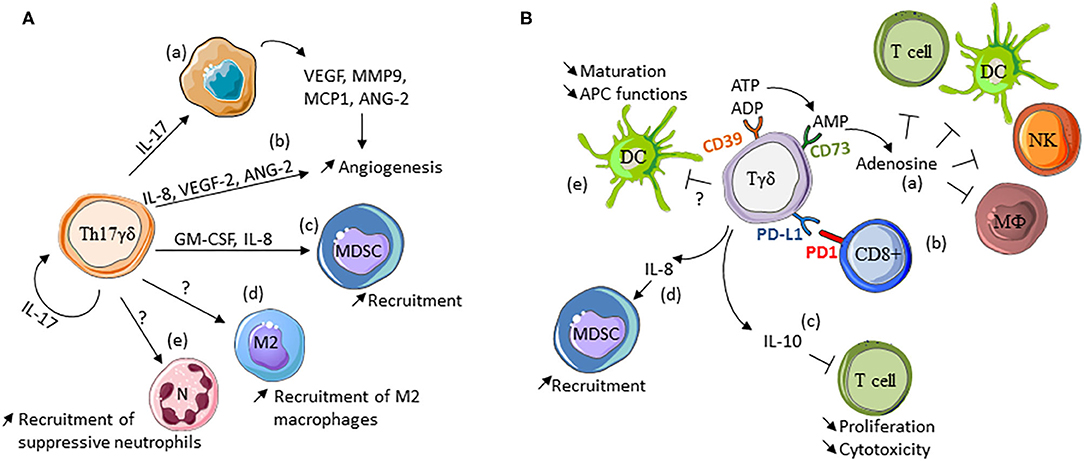
Figure 2. Pro-tumor functions of γδ T cells. (A) Th17-like γδ T cells. Th17γδ cells promote angiogenesis indirectly by inducing the production of angiogenic factors by cancer cells (a), or directly by producing angiogenic factors (b). They also induce myeloid-derived suppressor cell (MDSC) recruitment through GM-CSF and IL-8 production (c), and recruit or polarize M2 macrophages (d) and suppressive neutrophils (e). (B) Pro-tumor γδ T cells. CD39+/CD73+ γδ T cells produce adenosine that inhibits αβ T cell proliferation and anti-tumor functions (a). γδ T cells can express PD-L1 and inhibit the function of PD1-expressing cells, such as CD8 + cells (b). IL-10 produced by γδ T cells inhibits CD8 + cell proliferation and cytotoxicity (c). They also favor MDSC recruitment via IL-8 (d) and inhibit dendritic cell (DC) maturation and functions through not identified mechanisms (e). NK, natural killer cells; Mϕ, macrophages, APC, antigen-presenting cell.
Th17 γδ T Cells
IL-17 is detected in mice and human tumor (73–75), and αβ Th17 cells are not the only source of IL-17. Indeed, NK cells, neutrophils and γδ T cells also produce IL-17. Notably, Th17 γδ T cells are the first and major source of IL-17 at sites of inflammation or infection, and also in tumors.
Although Ma et al. showed that IL-17-producing γδ T cells (Vγ4 and Vγ6) contribute to the chemotherapy-induced anti-cancer immune response (76), many studies found that Th17 γδ cells display pro-tumor functions in mouse models and human solid cancers.
In mouse models of fibrosarcoma (77), ovarian (78) and breast cancers (79), γδ T cells are the main IL-17 producers at the tumor site, and promote tumor growth. Th17 γδ T cells increase the expression of the angiogenic factors VEGF-2 and ANG-2 at the tumor sites, suggesting that tumor-infiltrating IL-17-producing γδ T cells promote tumor development by enhancing angiogenesis (77). They also participate in the establishment of an immunosuppressive TME through the recruitment, expansion and polarization of neutrophils that can suppress cytotoxic T lymphocyte (CTL) activities (79), and the recruitment of MDSCs or small peritoneal macrophages in ovarian cancer. All these cells also induce the expression of pro-tumor and pro-angiogenic factors that promote tumor growth.
In human solid cancers, Wu et al. were the first to demonstrate the pro-tumor role of IL-17-producing γδ T cells in human colorectal cancer (33). They showed that the main IL-17 producers in colon cancer are γδ T cells (up to 83% of Vδ1 T cells). In this cancer, Th17 γδ T cell differentiation and activation are triggered by IL-23 produced by activated DCs present at the tumor site. Colon cancer-infiltrating Th17 γδ T cells produce also IL-8 that participates in tumor progression through its role in angiogenesis and in MDSC recruitment. These MDSCs contribute to establishing an immunosuppressive microenvironment that favors tumor development. Interestingly, the strong and positive correlation between tumor-infiltrating Th17 γδ T cells and TNM stage (tumor size, lymphatic invasion, and metastases) strengthens the pro-tumor activities of Th17 γδ T cells in human colorectal cancer (33). Studies in patients with gallbladder cancer showed an increase of Th17 γδ T cells in the blood (compared with healthy individuals), and also of tumor-infiltrating lymphocytes in patients who did not receive any treatment. They confirmed the implication of Th17 γδ T cells in angiogenesis promotion (induction of VEGF production by gallbladder cancer cells) and tumor progression. Moreover, the presence of Th17 γδ T cells in the blood of patients is associated with poor survival compared with patients with few or without Th17 γδ T cells (34). Lo Presti et al. showed that γδ T cells are increased in the blood and at the tumor site in patients with squamous cell carcinoma. Interestingly, tumor-infiltrating γδ T cells are functionally different depending on the tumor stage (80). At early stages, γδ T cells produce mainly IFN-γ, while at late stages, they produce IL-17. Indeed, higher numbers of IL-17-producing cells (both Vδ1 and Vδ2 γδ T cell subsets) are found in advanced-stage squamous cell carcinoma compared with early stage tumors. They also showed that both Vδ1 and Vδ2 cell subsets produce high levels of IL-17 at the tumor site. Moreover, Vδ2 T cells produce IFN-γ in the blood, suggesting that Th17 γδ T polarizing factors are present in the TME (80).
Overall, many reports demonstrated the pro-tumor functions of γδ T cells with a Th17 γδ T phenotype. To date, it is not possible to say whether this Th17 γδ T cell sub-population is recruited at the tumor site or is polarized in situ toward IL-17-producing cells due to the presence of Th17-polarizing cytokines in the TME (e.g., IL-1β, IL-23, TGF- β, IL-6). Nevertheless, it is now well-established that Th17 γδ T cells favor tumor growth by promoting angiogenesis, metastasis development, and the recruitment of other immunosuppressive cells, such as suppressive neutrophils and MDSCs.
Production of Suppressive Cytokines
As discussed in the polarization section, upon exposure to specific stimuli γδ T cells can acquire potent regulatory functions, particularly through the production of IL-10 and TGF-β, two strongly suppressive cytokines.
IL-10 is a key anti-inflammatory cytokine that inhibits the production of pro-inflammatory cytokines and the expression of co-stimulatory molecules by Th1 and antigen-presenting cells (81). In vitro, IL-4-polarized Vδ1 T cells produce IL-10 and inhibit the growth of Vδ2 T cells in an IL-10-dependent manner. Similarly, Vδ1 T cells activated with anti-TCR antibodies strongly secrete IL-10 (54, 82). In the presence of IL-21, the CD73+ Vδ2 and Vδ1 T cell subsets secrete high levels of IL-10 upon activation (30, 61). In human colorectal cancer, infiltrating CD39+ γδ T cells, which are mainly Vδ1+ cells, produce more IL-10 than CD39- γδ T cells and CD39+ γδ T cells from the tumor-adjacent normal tissue. However, after several days of culture ex vivo, these cells do not maintain IL-10 production and lose their ability to suppress the proliferation of activated T cells (31). In mice, IL-10-producing γδ T cells have been identified in tumors. In a breast cancer model, supernatant from infiltrating γδ T cells suppresses the proliferation of anti-tumor CTLs in an IL-10-dependent manner (83). In a syngeneic model of OVA-expressing EL4 tumors (lymphoma), IL-10-producing γδ T cells suppress the CD8-dependent anti-tumor response, and their depletion significantly reduces tumor growth (84). Similarly, IL-10+ γδ T cells are observed in the spleen and tumors of mice grafted with TC1 cells (transformed lung epithelial cells) (61). IL-10-producing γδ T cells are also observed in other conditions, for instance during pregnancy (both human and mouse), and in oral tolerance and infection in the mouse (85–87). Collectively, these results suggest that Vδ1 and Vδ2 T cells can produce IL-10; however, the amount and the impact of this production in human tumors has not been clearly established yet.
TGF-β is a potent immunosuppressive factor that is tightly regulated, particularly at the post-translational level. To be active, the mature part of the protein needs to be released from the latent peptide (LAP) through interaction with the integrin αvβ6 or αvβ8, the main activating partners of TGF-β. In vitro, TGFβ mRNA level and LAP surface expression are increased in Vδ1 T cells sorted from PBMCs and activated with anti-CD3 and anti-CD28 antibodies (88). High TGF-β level has also been detected in the supernatant of PBMCs stimulated with an anti-TCR Vδ1 antibody (82), and in the supernatant of Vδ2 T cells stimulated with the ligand isopentenyl pyrophosphate and expanded with TGF-β and IL-15 (45). In colorectal cancer, TGF-β surface expression is higher in γδ T cells isolated from tumors than from normal tissue (31). Interestingly, in the mouse tumor model MM2, infiltrating γδ T cells suppress the anti-MM2 CTLs through TGF-β in addition to IL-10 (83). However, it is unclear whether total or active TGF-β was measured in these studies. While total TGF-β is a measure of the whole TGF-β production by the cells, only active TGF-β quantification indicates the actual suppressive potential of such cells through TGF-β. Indeed, in these studies, γδ T cell suppressive properties were not affected by a neutralizing anti-TGF-β antibody, despite their supposed high level of TGF-β production, or the impact of TGF-β neutralization was not explored. A possible explanation for this discrepancy is that only total TGF-β was measured and not active TGF-β. This argument is supported by the reported high concentration that is more consistent with the measurement of total TGF-β. These results suggest that human γδ T cells, particularly Vδ1 T cells, can produce and present latent TGF-β at their surface in some contexts. However, because of the lack of αvβ6 or αvβ8 integrin expression, γδ T cells might not be able to produce active TGF-β on their own, unlike conventional Tregs (89, 90). Nonetheless, the presence of latent TGF-β at the γδ T cell surface is highly relevant because they represent a new source of latent TGF- β that may be activated by integrin-expressing partners within the tumor.
Besides the production of directly suppressive cytokines, γδ T cells also support the establishment of a suppressive TME through the production of other cytokines, such as IL-8 and granulocyte macrophage-colony stimulating factor (GM-CSF) that favor PMN-MDSC accumulation and expansion in colorectal cancer (33). Interestingly, IL-21, which is highly expressed in this cancer type, increases the production of IL-8 by CD73+ Vδ2 T cells and Vδ1 T cells in vitro (30, 61).
Involvement of the Adenosine Pathway
Extracellular ATP and adenosine are considered potent modulators of the anti-tumor immune response. Extracellular ATP, released by apoptotic cells for example, induces inflammation and promotes strong anti-tumor responses because it increases the immunogenicity of dying cancer cells (91, 92). It favors the recruitment of phagocytes, the recruitment and maturation of DC, inhibits the proliferation of tumor cells but not of healthy cells, and promotes cancer cell death (91, 93, 94). Conversely, extracellular adenosine inhibits the anti-tumor immune response and induces the establishment of an immunosuppressive microenvironment (95). The adenosine pathway involves the ectonucleoside triphosphate diphosphohydrolase 1 (ENTPD1 or CD39) that catalyzes the phosphohydrolysis of extracellular ATP into ADP and of ADP into AMP, and the ecto-5′-nucleotidase CD73 that completes AMP conversion into adenosine (92, 96, 97). It has been shown that γδ T cells express CD39 and/or CD73 during inflammation and in the TME. Their expression is associated with suppression or inhibition of the immune response (98–100). In murine pancreatic cancer, Daley et al. found that tumor-infiltrating γδ T cells upregulate CD39 expression (among other immunosuppressive molecules) and promote tumor progression by restricting αβ T cell activation (36). Hu and colleagues described in human colorectal cancer a subpopulation of regulatory γδ T cells that express CD39 (31). CD39+ γδ T cells are enriched at the tumor site and produce high levels of adenosine in the TME, compared with other regulatory cells such as conventional Tregs. Furthermore, they showed that infiltration of CD39+ γδ T cells is positively correlated with the TNM stage, suggesting that these cells participate in the establishment of an immunosuppressive TME, thus promoting tumor growth (31). In vitro, our group identified subpopulations of regulatory γδ T cells isolated from peripheral blood that express CD73 and can produce adenosine. These CD73+ populations (Vγ9Vδ2 or Vδ1) also express CD39 and catalyze the transformation of ATP into adenosine, thus displaying immunosuppressive functions, as revealed by their capacity to inhibit αβ T cell proliferation (30, 61). These regulatory CD73+ γδ T cells are found in human breast cancer samples, suggesting that they could interfere with the anti-tumor immune response and favor tumor progression (30). Altogether, these studies indicate that the CD39/CD73/adenosine pathway is a major component of γδ T cell regulatory/immunosuppressive functions in the TME.
Other Suppressive Mechanisms of γδ T Cells
The previously described regulatory γδ T cells can contribute to the establishment of an immunosuppressive microenvironment and to the inhibition of the anti-tumor response in different manners, for instance by producing inhibitory factors (e.g., IL-10, IL-8, TGF-β and adenosine) or by recruiting immunosuppressive cells (e.g., MDSCs and neutrophils). γδ T cells can also exert their regulatory functions by providing negative co-stimulatory signals to T cells in the TME through expression of immune checkpoint proteins. Programmed cell death 1 (PD1) and its ligand programmed cell death 1 ligand 1 (PD-L1) play a major role in the negative regulation of cell-mediated immune responses. Indeed, PD1 is expressed by T cells, and upon binding to its ligand (expressed by B cells, macrophages and cancer cells), it inhibits T cell activation, thus impairing the anti-tumor T cell response. Peters et al. showed that Vδ2 T cells obtained from the blood of healthy donors can express PD-L1 following activation (47). These cells inhibit αβ T cell proliferation in co-culture experiments, and this effect can be abrogated by PD-L1 blockade (47). This could be another mechanism by which regulatory γδ T cells exert their immunosuppressive activities and promote tumor growth. In agreement, Daley et al. showed in a pancreatic cancer mouse model that PD-L1 expression is higher in tumor-infiltrating γδ T cells than in splenic γδ T cells (36). In co-culture experiments, they found that tumor-infiltrating γδ T cells prevent αβ T cell activation and that this inhibition is reversed by an anti-PD-L1 antibody (36). Interestingly, the same regulatory phenotype is observed in pancreatic ductal adenocarcinoma (PDAC). Indeed, PD-L1 is strongly expressed in γδ T cells from the blood of patients with pancreatic cancer compared with healthy donors. Tumor-infiltrating γδ T cells also express PD-L1 in human PDAC (50% of infiltrating γδ T cells), suggesting that γδ T cells can promote tumor progression through the PD1/PD-L1 axis (36).
T-cell immunoglobulin mucin receptor 3 (TIM-3) and its ligand galectin-9 (GAL-9) are other immune checkpoint molecules that participate in T cell response inhibition. TIM-3 interaction with GAL-9 limits T cell expansion and effector function in the TME (101, 102). GAL-9 expression is upregulated on tumor-infiltrating γδ T cells in human and mouse PDAC, and γδ T cell-mediated suppression is dependent on GAL-9 (36).
Little is known about the expression of other immune checkpoint molecules, such as PD-L2, CD80/86 and CTLA-4, by γδ T cells in cancer. More studies are needed to investigate the expression of these and other suppressive molecules to fully understand the mechanisms of action of regulatory γδ T cells.
Implications for γδ T Cell-Based Tumor Immunotherapy
The discovery of γδ T cell-mediated tumor immune surveillance has led to much research to understand the underlying mechanisms and to harness their potent anti-tumor properties. It is now firmly established that γδ T cells are well-equipped to recognize and eliminate malignant cells (20, 103). Thus, much effort has focused on the development of therapeutics using γδ T cells, especially the Vγ9Vδ2 subset because they can be easily obtained and expanded from the blood (104, 105). Two main strategies were first investigated: (i) in vivo expansion of Vγ9Vδ2 T cells by injection of phosphoantigens and low-dose IL-2 in the patient, and (ii) adoptive transfer of ex vivo expanded Vγ9Vδ2 T cells. Clinical trials using both strategies in patients with hematological or solid cancers confirmed the safety of this immunotherapy (well-tolerated and no toxicity), but showed moderate clinical success (106–109). Indeed, the results were not as good as expected because only few patients showed complete response to the therapy. Among the reasons of these relatively modest clinical results were the skewing of γδ T cells toward a non-reactive or even a pro-tumor phenotype. For example, Hoeres et al. showed that incubation of PBMCs from patients with leukemia with IL-2 and/or zoledronic acid, which are used to activate γδ T cells, induces PD-1 expression by γδ T cells and impairs their anti-tumor functions (110). Similarly, Castella et al. reported PD-1 expression by γδ T cells in patients with myeloma after phosphoantigen activation (111). Several in vitro and in vivo studies, summarized here, have demonstrated that γδ T cell polarization toward suppressive and/or IL-17-producing cells is a real possibility and that anti- and pro-tumor γδ T cells might co-exist in the tumor.
After these first clinical trials, new refined approaches based on recent discoveries are currently being developed. Aminobisphosphonate activation of γδ T cells in combination with chemotherapy or with FDA-approved antibodies is one of these axes. Hoeres et al. and Castella et al. showed that incubation with an anti-PD-1 antibody restores the proliferative and anti-tumor properties of Vγ9Vδ2 T cells from patients with leukemia or lymphoma (110, 111). However, Castella et al. then found that phosphoantigen stimulation of anergic PD-1+ Vγ9Vδ2 combined with PD-1 blockade increases the expression of PD-1 and of two other immune checkpoint molecules (TIM-3 and LAG-3), leading to a “super-anergic” state (112). Thus, although the combination of γδ T cell stimulation and immune checkpoint blockade is an interesting and easily feasible therapeutic alternative, it still needs to be improved, by combining for example two or more antibodies against immune checkpoint molecules. The use of bi-specific T-cell engagers (BITEs), tribodies, and engineered T cells harboring a chimeric antigen receptor (CAR) are other interesting options. For instance, the redirection of Vγ9Vδ2 T cells against tumor cells using bispecific antibodies or tribodies is efficient in HER-2-positive PDAC and ovarian cancer (113). TEGs are αβ T cells engineered to express tumor-specific Vγ9Vδ2 TCRs. In in vitro models and in humanized mouse cancer models, TEGs reduce colony formation of progenitor cells of primary acute myeloid leukemia blasts and inhibit leukemia growth (114). TEGs engineered from patients with myeloma can recognize and efficiently kill myeloma cells in a 3D bone marrow niche model. Phase 1 clinical trials are currently in development to test TEGs, CAR γδ T cells, and antibodies (bispecific antibodies or anti-BTN3A antibodies) to specifically “engage” γδ T cells in the anti-tumor immune response (26).
Another strategy would be to focus on Vδ1 T cells, the main subpopulation that infiltrates the TME of solid tumors. Despite their potent anti-tumor properties, Vδ1 T cells had never been tested in the clinic due to lack of suitable expansion/differentiation protocols. Recently, Silva-Santos' team developed a new and robust clinical-grade method for selective and large-scale expansion and differentiation of cytotoxic Vδ1 T cells, and showed that these cells can inhibit tumor growth and dissemination in preclinical models of chronic lymphocytic leukemia (27).
On the basis of reports demonstrating γδ T cell pro-tumor functions, regulatory γδ T cell subsets could be a thorn in the side of these newly developed therapies and need to be taken into account. Unfortunately, no clear phenotypic marker of such cells has emerged yet. Vδ1 cells have been associated with pro-tumor T cells, but when cultured in proper conditions they show very high potential for anti-tumor therapies due to their strong reactivity and cytotoxicity toward tumor cells. Adenosine pathway markers (e.g., CD39 and CD73) are interesting, but do not characterize pro-tumor γδ T cells on their own. Indeed, CD39 can be considered as an activation marker for T cells (115, 116), and CD73 is also expressed by naive γδ T cells (117, 118). More studies are needed to better characterize γδ T cell pro-tumor phenotypes and to identify markers or marker combinations that will allow the depletion of pro-tumor subsets in the whole γδ T cell population.
In the absence of such specific phenotypic markers to deplete or sort out the pro-tumor γδ T cells before cell therapy, targeting polarizing cytokines or pro-tumor cytokines produced by pro-tumor γδ T cells could be of interest. While IL-21 expression might favor the emergence of a regulatory γδ T cell population, its positive role on the cytotoxicity of other cell types, such as CTL and NK cells, might be important for the anti-tumor response. Alternatively, targeting TGF-β as a pro-tumor cytokine and a polarizing factor for γδ T cells toward both suppressive and IL-17-producing cells might be of interest. Newly developed highly selective approaches targeting the TGF-β-anchoring protein GARP or the latent TGF-β peptide LAP could be employed in pro-tumor γδ T cell-rich tumors, such as colorectal cancer, or with γδ T cell-based therapies to avoid their polarization (119, 120). While no anti-human IL-10 antibody has been approved for cancer treatment, the production of IL-17A and adenosine could be targeted in tumors that are highly infiltrated by pro-tumor γδ T cells, such as breast and colorectal cancer.
Concluding Remarks
Although γδ T cells offer interesting perspectives for clinical applications in cell-based immunotherapy, their pro-tumor functions have to be taken into account. Indeed, environmental factors can polarize or repolarize γδ T cells, leading to loss of the anti-tumor function. Moreover, important advances in γδ T cell immunobiology have revealed a large diversity in functionality and activation modes of these cells. The new challenge is to better characterize and understand the role of the various γδ T cell subsets in function of the specific context.
Author Contributions
GC, CB, and VL wrote the initial draft. GC prepared the figures and CB the table. GC, CB, VL, and NB reviewed the manuscript. All authors contributed to the article and approved the submitted version.
Funding
This work was supported by the Institute National de la Santé et de la Recherche Médicale (INSERM); Université de Montpellier; the Institut Régional du Cancer de Montpellier (ICM), the SIRIC Montpellier Cancer (Grant INCa_Inserm_DGOS_12553), and the Ligue contre le Cancer. This work was also supported by the Fondation pour la Recherche Médicale (FRM), grant number ECO201806006863, to GC.
Conflict of Interest
The authors declare that the research was conducted in the absence of any commercial or financial relationships that could be construed as a potential conflict of interest.
Acknowledgments
We acknowledge all the members of NB's team for their stimulating comments.
References
1. Kabelitz D, Marischen L, Oberg HH, Holtmeier W, Wesch D. Epithelial defence by gamma delta T cells. Int Arch Allergy Immunol. (2005) 137:73–81. doi: 10.1159/000085107
2. Willcox CR, Pitard V, Netzer S, Couzi L, Salim M, Silberzahn T, et al. Cytomegalovirus and tumor stress surveillance by binding of a human gammadelta T cell antigen receptor to endothelial protein C receptor. Nat Immunol. (2012) 13:872–9. doi: 10.1038/ni.2394
3. Yang Y, Li L, Yuan L, Zhou X, Duan J, Xiao H, et al. A structural change in butyrophilin upon phosphoantigen binding underlies phosphoantigen-mediated Vgamma9Vdelta2 T cell activation. Immunity. (2019) 50:1043–53 e1045. doi: 10.1016/j.immuni.2019.02.016
4. Rigau M, Ostrouska S, Fulford TS, Johnson DN, Woods K, Ruan Z, et al. Butyrophilin 2A1 is essential for phosphoantigen reactivity by gammadelta T cells. Science. (2020) 367:eaay5516. doi: 10.1126/science.aay5516
5. Fournie JJ, Bonneville M. Stimulation of gamma delta T cells by phosphoantigens. Res Immunol. (1996) 147:338–47. doi: 10.1016/0923-2494(96)89648-9
6. Battistini L, Borsellino G, Sawicki G, Poccia F, Salvetti M, Ristori G, et al. Phenotypic and cytokine analysis of human peripheral blood gamma delta T cells expressing NK cell receptors. J Immunol. (1997) 159:3723–30.
7. De Libero G. Control of gammadelta T cells by NK receptors. Microbes Infect. (1999) 1:263–7. doi: 10.1016/S1286-4579(99)80043-4
8. Groh V, Steinle A, Bauer S, Spies T. Recognition of stress-induced MHC molecules by intestinal epithelial gammadelta T cells. Science. (1998) 279:1737–40. doi: 10.1126/science.279.5357.1737
9. Spada FM, Grant EP, Peters PJ, Sugita M, Melian A, Leslie DS, et al. Self-recognition of CD1 by gamma/delta T cells: implications for innate immunity. J Exp Med. (2000) 191:937–48. doi: 10.1084/jem.191.6.937
10. Bai L, Picard D, Anderson B, Chaudhary V, Luoma A, Jabri B, et al. The majority of CD1d-sulfatide-specific T cells in human blood use a semiinvariant Vdelta1 TCR. Eur J Immunol. (2012) 42:2505–10. doi: 10.1002/eji.201242531
11. Uldrich AP, Le Nours J, Pellicci DG, Gherardin NA, Mcpherson KG, Lim RT, et al. CD1d-lipid antigen recognition by the gammadelta TCR. Nat Immunol. (2013) 14:1137–45. doi: 10.1038/ni.2713
12. Zeng X, Wei YL, Huang J, Newell EW, Yu H, Kidd BA, et al. gammadelta T cells recognize a microbial encoded B cell antigen to initiate a rapid antigen-specific interleukin-17 response. Immunity. (2012) 37:524–34. doi: 10.1016/j.immuni.2012.06.011
13. Fausther-Bovendo H, Wauquier N, Cherfils-Vicini J, Cremer I, Debre P, Vieillard V. NKG2C is a major triggering receptor involved in the V[delta]1 T cell-mediated cytotoxicity against HIV-infected CD4 T cells. AIDS. (2008) 22:217–26. doi: 10.1097/QAD.0b013e3282f46e7c
14. Wesch D, Peters C, Oberg HH, Pietschmann K, Kabelitz D. Modulation of gammadelta T cell responses by TLR ligands. Cell Mol Life Sci. (2011) 68:2357–70. doi: 10.1007/s00018-011-0699-1
15. Hudspeth K, Fogli M, Correia DV, Mikulak J, Roberto A, Della Bella S, et al. Engagement of NKp30 on Vdelta1 T cells induces the production of CCL3, CCL4, and CCL5 and suppresses HIV-1 replication. Blood. (2012) 119:4013–6. doi: 10.1182/blood-2011-11-390153
16. Kuroda H, Saito H, Ikeguchi M. Decreased number and reduced NKG2D expression of Vdelta1 gammadelta T cells are involved in the impaired function of Vdelta1 gammadelta T cells in the tissue of gastric cancer. Gastric Cancer. (2012) 15:433–9. doi: 10.1007/s10120-011-0138-x
17. Maher CO, Dunne K, Comerford R, O'dea S, Loy A, Woo J, et al. Candida albicans stimulates IL-23 release by human dendritic cells and downstream IL-17 secretion by Vdelta1 T cells. J Immunol. (2015) 194:5953–60. doi: 10.4049/jimmunol.1403066
18. Bonneville M, O'brien RL, Born WK. Gammadelta T cell effector functions: a blend of innate programming and acquired plasticity. Nat Rev Immunol. (2010) 10:467–78. doi: 10.1038/nri2781
19. Adams EJ, Gu S, Luoma AM. Human gamma delta T cells: evolution and ligand recognition. Cell Immunol. (2015) 296:31–40. doi: 10.1016/j.cellimm.2015.04.008
20. Gomes AQ, Martins DS, Silva-Santos B. Targeting gammadelta T lymphocytes for cancer immunotherapy: from novel mechanistic insight to clinical application. Cancer Res. (2010) 70:10024–7. doi: 10.1158/0008-5472.CAN-10-3236
21. Marquez-Medina D, Salla-Fortuny J, Salud-Salvia A. Role of gamma-delta T-cells in cancer: another opening door to immunotherapy. Clin Transl Oncol. (2012) 14:891–5. doi: 10.1007/s12094-012-0935-7
22. Buccheri S, Guggino G, Caccamo N, Li Donni P, Dieli F. Efficacy and safety of gammadeltaT cell-based tumor immunotherapy: a meta-analysis. J Biol Regul Homeost Agents. (2014) 28:81–90.
23. Pressey JG, Adams J, Harkins L, Kelly D, You Z, Lamb LSJr. In vivo expansion and activation of gammadelta T cells as immunotherapy for refractory neuroblastoma: a phase 1 study. Medicine. (2016) 95:e4909. doi: 10.1097/MD.0000000000004909
24. Lo Presti E, Pizzolato G, Gulotta E, Cocorullo G, Gulotta G, Dieli F, et al. Current advances in gammadelta T cell-based tumor immunotherapy. Front Immunol. (2017) 8:1401. doi: 10.3389/fimmu.2017.01401
25. Pauza CD, Liou ML, Lahusen T, Xiao L, Lapidus RG, Cairo C, et al. Gamma delta T cell therapy for cancer: it is good to be local. Front Immunol. (2018) 9:1305. doi: 10.3389/fimmu.2018.01305
26. Garber K. gammadelta T cells bring unconventional cancer-targeting to the clinic - again. Nat Biotechnol. (2020) 38:389–91. doi: 10.1038/s41587-020-0487-2
27. Almeida AR, Correia DV, Fernandes-Platzgummer A, Da Silva CL, Da Silva MG, Anjos DR, et al. Delta one T cells for immunotherapy of chronic lymphocytic leukemia: clinical-grade expansion/differentiation and preclinical proof of concept. Clin Cancer Res. (2016) 22:5795–804. doi: 10.1158/1078-0432.CCR-16-0597
28. Peng G, Wang HY, Peng W, Kiniwa Y, Seo KH, Wang RF. Tumor-infiltrating gammadelta T cells suppress T and dendritic cell function via mechanisms controlled by a unique toll-like receptor signaling pathway. Immunity. (2007) 27:334–48. doi: 10.1016/j.immuni.2007.05.020
29. Ma C, Zhang Q, Ye J, Wang F, Zhang Y, Wevers E, et al. Tumor-infiltrating gammadelta T lymphocytes predict clinical outcome in human breast cancer. J Immunol. (2012) 189:5029–36. doi: 10.4049/jimmunol.1201892
30. Chabab G, Barjon C, Abdellaoui N, Salvador-Prince L, Dejou C, Michaud HA, et al. Identification of a regulatory Vdelta1 gamma delta T cell subpopulation expressing CD73 in human breast cancer. J Leukoc Biol. (2020) 107:1057–67. doi: 10.1002/JLB.3MA0420-278RR
31. Hu G, Wu P, Cheng P, Zhang Z, Wang Z, Yu X, et al. Tumor-infiltrating CD39(+)gammadeltaTregs are novel immunosuppressive T cells in human colorectal cancer. Oncoimmunology. (2017) 6:e1277305. doi: 10.1080/2162402X.2016.1277305
32. Rong L, Li K, Li R, Liu HM, Sun R, Liu XY. Analysis of tumor-infiltrating gamma delta T cells in rectal cancer. World J Gastroenterol. (2016) 22:3573–80. doi: 10.3748/wjg.v22.i13.3573
33. Wu P, Wu D, Ni C, Ye J, Chen W, Hu G, et al. gammadeltaT17 cells promote the accumulation and expansion of myeloid-derived suppressor cells in human colorectal cancer. Immunity. (2014) 40:785–800. doi: 10.1016/j.immuni.2014.03.013
34. Patil RS, Shah SU, Shrikhande SV, Goel M, Dikshit RP, Chiplunkar SV. IL17 producing gammadeltaT cells induce angiogenesis and are associated with poor survival in gallbladder cancer patients. Int J Cancer. (2016) 139:869–81. doi: 10.1002/ijc.30134
35. Chen X, Shang W, Xu R, Wu M, Zhang X, Huang P, et al. Distribution and functions of gammadelta T cells infiltrated in the ovarian cancer microenvironment. J Transl Med. (2019) 17:144. doi: 10.1186/s12967-019-1897-0
36. Daley D, Zambirinis CP, Seifert L, Akkad N, Mohan N, Werba G, et al. gammadelta T cells support pancreatic oncogenesis by restraining alphabeta T cell activation. Cell. (2016) 166:1485–99 e1415. doi: 10.1016/j.cell.2016.07.046
37. Gentles AJ, Newman AM, Liu CL, Bratman SV, Feng W, Kim D, et al. The prognostic landscape of genes and infiltrating immune cells across human cancers. Nat Med. (2015) 21:938–45. doi: 10.1038/nm.3909
38. Tosolini M, Pont F, Poupot M, Vergez F, Nicolau-Travers ML, Vermijlen D, et al. Assessment of tumor-infiltrating TCRVgamma9Vdelta2 gammadelta lymphocyte abundance by deconvolution of human cancers microarrays. Oncoimmunology. (2017) 6:e1284723. doi: 10.1080/2162402X.2017.1284723
39. Lo Presti E, Mocciaro F, Mitri RD, Corsale AM, Di Simone M, Vieni S, et al. Analysis of colon-infiltrating gammadelta T cells in chronic inflammatory bowel disease and in colitis-associated cancer. J Leukoc Biol. (2020) 108:749–60. doi: 10.1002/JLB.5MA0320-201RR
40. Lafont V, Sanchez F, Laprevotte E, Michaud HA, Gros L, Eliaou JF, et al. Plasticity of gammadelta T cells: impact on the anti-tumor response. Front Immunol. (2014) 5:622. doi: 10.3389/fimmu.2014.00622
41. Travis MA, Sheppard D. TGF-beta activation and function in immunity. Annu Rev Immunol. (2014) 32:51–82. doi: 10.1146/annurev-immunol-032713-120257
42. Qin Y, Garrison BS, Ma W, Wang R, Jiang A, Li J, et al. A milieu molecule for TGF-beta required for microglia function in the nervous system. Cell. (2018) 174:156–71. e116. doi: 10.1016/j.cell.2018.05.027
43. Chen W, Jin W, Hardegen N, Lei KJ, Li L, Marinos N, et al. Conversion of peripheral CD4+CD25- naive T cells to CD4+CD25+ regulatory T cells by TGF-beta induction of transcription factor Foxp3. J Exp Med. (2003) 198:1875–86. doi: 10.1084/jem.20030152
44. Manel N, Unutmaz D, Littman DR. The differentiation of human T(H)-17 cells requires transforming growth factor-beta and induction of the nuclear receptor RORgammat. Nat Immunol. (2008) 9:641–9. doi: 10.1038/ni.1610
45. Casetti R, Agrati C, Wallace M, Sacchi A, Martini F, Martino A, et al. Cutting edge: TGF-beta1 and IL-15 Induce FOXP3+ gammadelta regulatory T cells in the presence of antigen stimulation. J Immunol. (2009) 183:3574–7. doi: 10.4049/jimmunol.0901334
46. Hu Y, Cui Q, Gu Y, Sheng L, Wu K, Shi J, et al. Decitabine facilitates the generation and immunosuppressive function of regulatory gammadeltaT cells derived from human peripheral blood mononuclear cells. Leukemia. (2013) 27:1580–5. doi: 10.1038/leu.2012.345
47. Peters C, Oberg HH, Kabelitz D, Wesch D. Phenotype and regulation of immunosuppressive Vdelta2-expressing gammadelta T cells. Cell Mol Life Sci. (2014) 71:1943–60. doi: 10.1007/s00018-013-1467-1
48. Peters C, Hasler R, Wesch D, Kabelitz D. Human Vdelta2 T cells are a major source of interleukin-9. Proc Natl Acad Sci USA. (2016) 113:12520–5. doi: 10.1073/pnas.1607136113
49. Kouakanou L, Peters C, Sun Q, Floess S, Bhat J, Huehn J, et al. Vitamin C supports conversion of human gammadelta T cells into FOXP3-expressing regulatory cells by epigenetic regulation. Sci Rep. (2020) 10:6550. doi: 10.1038/s41598-020-63572-w
50. Li X, Kang N, Zhang X, Dong X, Wei W, Cui L, et al. Generation of human regulatory gammadelta T cells by TCRgammadelta stimulation in the presence of TGF-beta and their involvement in the pathogenesis of systemic lupus erythematosus. J Immunol. (2011) 186:6693–700. doi: 10.4049/jimmunol.1002776
51. Kang N, Tang L, Li X, Wu D, Li W, Chen X, et al. Identification and characterization of Foxp3(+) gammadelta T cells in mouse and human. Immunol Lett. (2009) 125:105–13. doi: 10.1016/j.imlet.2009.06.005
52. Caccamo N, La Mendola C, Orlando V, Meraviglia S, Todaro M, Stassi G, et al. Differentiation, phenotype, and function of interleukin-17-producing human Vgamma9Vdelta2 T cells. Blood. (2011) 118:129–38. doi: 10.1182/blood-2011-01-331298
53. Setrerrahmane S, Xu H. Tumor-related interleukins: old validated targets for new anti-cancer drug development. Mol Cancer. (2017) 16:153. doi: 10.1186/s12943-017-0721-9
54. Mao Y, Yin S, Zhang J, Hu Y, Huang B, Cui L, et al. A new effect of IL-4 on human gammadelta T cells: promoting regulatory Vdelta1 T cells via IL-10 production and inhibiting function of Vdelta2 T cells. Cell Mol Immunol. (2016) 13:217–28. doi: 10.1038/cmi.2015.07
55. Wesch D, Glatzel A, Kabelitz D. Differentiation of resting human peripheral blood gamma delta T cells toward Th1- or Th2-phenotype. Cell Immunol. (2001) 212:110–7. doi: 10.1006/cimm.2001.1850
56. Siegers GM, Ribot EJ, Keating A, Foster PJ. Extensive expansion of primary human gamma delta T cells generates cytotoxic effector memory cells that can be labeled with Feraheme for cellular MRI. Cancer Immunol Immunother. (2013) 62:571–83. doi: 10.1007/s00262-012-1353-y
57. Chabab G, Bonnefoy N, Lafont V. IL-21 Signaling in the Tumor Microenvironment. Adv Exp Med Biol. (2020) 1240:73–82. doi: 10.1007/978-3-030-38315-2_6
58. Stolfi C, Rizzo A, Franze E, Rotondi A, Fantini MC, Sarra M, et al. Involvement of interleukin-21 in the regulation of colitis-associated colon cancer. J Exp Med. (2011) 208:2279–90. doi: 10.1084/jem.20111106
59. De Simone V, Ronchetti G, Franze E, Colantoni A, Ortenzi A, Fantini MC, et al. Interleukin-21 sustains inflammatory signals that contribute to sporadic colon tumorigenesis. Oncotarget. (2015) 6:9908–23. doi: 10.18632/oncotarget.3532
60. Thedrez A, Sabourin C, Gertner J, Devilder MC, Allain-Maillet S, Fournie JJ, et al. Self/non-self discrimination by human gammadelta T cells: simple solutions for a complex issue? Immunol Rev. (2007) 215:123–35. doi: 10.1111/j.1600-065X.2006.00468.x
61. Barjon C, Michaud HA, Fages A, Dejou C, Zampieri A, They L, et al. IL-21 promotes the development of a CD73-positive Vgamma9Vdelta2 T cell regulatory population. Oncoimmunology. (2017) 7:e1379642. doi: 10.1080/2162402X.2017.1379642
62. Huang Y, Matsumura Y, Hatano S, Noguchi N, Murakami T, Iwakura Y, et al. IL-21 inhibits IL-17A-producing gammadelta T-cell response after infection with Bacillus Calmette-Guerin via induction of apoptosis. Innate Immun. (2016) 22:588–97. doi: 10.1177/1753425916664125
63. Yang XO, Pappu BP, Nurieva R, Akimzhanov A, Kang HS, Chung Y, et al. T helper 17 lineage differentiation is programmed by orphan nuclear receptors ROR alpha and ROR gamma. Immunity. (2008) 28:29–39. doi: 10.1016/j.immuni.2007.11.016
64. Stockinger B, Veldhoen M. Differentiation and function of Th17 T cells. Curr Opin Immunol. (2007) 19:281–6. doi: 10.1016/j.coi.2007.04.005
65. Cua DJ, Sherlock J, Chen Y, Murphy CA, Joyce B, Seymour B, et al. Interleukin-23 rather than interleukin-12 is the critical cytokine for autoimmune inflammation of the brain. Nature. (2003) 421:744–8. doi: 10.1038/nature01355
66. Wilson NJ, Boniface K, Chan JR, Mckenzie BS, Blumenschein WM, Mattson JD, et al. Development, cytokine profile and function of human interleukin 17-producing helper T cells. Nat Immunol. (2007) 8:950–7. doi: 10.1038/ni1497
67. Zhou L, Ivanov Ii, Spolski R, Min R, Shenderov K, Egawa T, et al. IL-6 programs T(H)-17 cell differentiation by promoting sequential engagement of the IL-21 and IL-23 pathways. Nat Immunol. (2007) 8:967–74. doi: 10.1038/ni1488
68. Sutton CE, Lalor SJ, Sweeney CM, Brereton CF, Lavelle EC, Mills KH. Interleukin-1 and IL-23 induce innate IL-17 production from gammadelta T cells, amplifying Th17 responses and autoimmunity. Immunity. (2009) 31:331–41. doi: 10.1016/j.immuni.2009.08.001
69. Deknuydt F, Scotet E, Bonneville M. Modulation of inflammation through IL-17 production by gammadelta T cells: mandatory in the mouse, dispensable in humans? Immunol Lett. (2009) 127:8–12. doi: 10.1016/j.imlet.2009.08.003
70. Michel ML, Pang DJ, Haque SF, Potocnik AJ, Pennington DJ, Hayday AC. Interleukin 7 (IL-7) selectively promotes mouse and human IL-17-producing gammadelta cells. Proc Natl Acad Sci USA. (2012) 109:17549–54. doi: 10.1073/pnas.1204327109
71. Ness-Schwickerath KJ, Jin C, Morita CT. Cytokine requirements for the differentiation and expansion of IL-17A- and IL-22-producing human Vgamma2Vdelta2 T cells. J Immunol. (2010) 184:7268–80. doi: 10.4049/jimmunol.1000600
72. Ness-Schwickerath KJ, Morita CT. Regulation and function of IL-17A- and IL-22-producing gammadelta T cells. Cell Mol Life Sci. (2011) 68:2371–90. doi: 10.1007/s00018-011-0700-z
73. Kato Y, Tanaka Y, Miyagawa F, Yamashita S, Minato N. Targeting of tumor cells for human gammadelta T cells by nonpeptide antigens. J Immunol. (2001) 167:5092–8. doi: 10.4049/jimmunol.167.9.5092
74. Numasaki M, Watanabe M, Suzuki T, Takahashi H, Nakamura A, Mcallister F, et al. IL-17 enhances the net angiogenic activity and in vivo growth of human non-small cell lung cancer in SCID mice through promoting CXCR-2-dependent angiogenesis. J Immunol. (2005) 175:6177–89. doi: 10.4049/jimmunol.175.9.6177
75. Kryczek I, Wei S, Zou L, Altuwaijri S, Szeliga W, Kolls J, et al. Cutting edge: Th17 and regulatory T cell dynamics and the regulation by IL-2 in the tumor microenvironment. J Immunol. (2007) 178:6730–3. doi: 10.4049/jimmunol.178.11.6730
76. Ma Y, Aymeric L, Locher C, Mattarollo SR, Delahaye NF, Pereira P, et al. Contribution of IL-17-producing gamma delta T cells to the efficacy of anticancer chemotherapy. J Exp Med. (2011) 208:491–503. doi: 10.1084/jem.20100269
77. Wakita D, Sumida K, Iwakura Y, Nishikawa H, Ohkuri T, Chamoto K, et al. Tumor-infiltrating IL-17-producing gammadelta T cells support the progression of tumor by promoting angiogenesis. Eur J Immunol. (2010) 40:1927–37. doi: 10.1002/eji.200940157
78. Rei M, Goncalves-Sousa N, Lanca T, Thompson RG, Mensurado S, Balkwill FR, et al. Murine CD27(−) Vgamma6(+) gammadelta T cells producing IL-17A promote ovarian cancer growth via mobilization of protumor small peritoneal macrophages. Proc Natl Acad Sci USA. (2014) 111:E3562–70. doi: 10.1073/pnas.1403424111
79. Coffelt SB, Kersten K, Doornebal CW, Weiden J, Vrijland K, Hau CS, et al. IL-17-producing gammadelta T cells and neutrophils conspire to promote breast cancer metastasis. Nature. (2015) 522:345–8. doi: 10.1038/nature14282
80. Lo Presti E, Toia F, Oieni S, Buccheri S, Turdo A, Mangiapane LR, et al. Squamous cell tumors recruit gammadelta T cells producing either IL17 or IFNgamma depending on the tumor stage. Cancer Immunol Res. (2017) 5:397–407. doi: 10.1158/2326-6066.CIR-16-0348
81. Wei H, Li B, Sun A, Guo F. Interleukin-10 family cytokines immunobiology and structure. Adv Exp Med Biol. (2019) 1172:79–96. doi: 10.1007/978-981-13-9367-9_4
82. Hua F, Kang N, Gao YA, Cui LX, Ba DN, He W. Potential regulatory role of in vitro-expanded Vdelta1 T cells from human peripheral blood. Immunol Res. (2013) 56:172–80. doi: 10.1007/s12026-013-8390-2
83. Seo N, Tokura Y, Takigawa M, Egawa K. Depletion of IL-10- and TGF-beta-producing regulatory gamma delta T cells by administering a daunomycin-conjugated specific monoclonal antibody in early tumor lesions augments the activity of CTLs and NK cells. J Immunol. (1999) 163:242–9.
84. Ke Y, Kapp LM, Kapp JA. Inhibition of tumor rejection by gammadelta T cells and IL-10. Cell Immunol. (2003) 221:107–14. doi: 10.1016/S0008-8749(03)00066-2
85. Arck PC, Ferrick DA, Steele-Norwood D, Croitoru K, Clark DA. Murine T cell determination of pregnancy outcome: I. Effects of strain, alphabeta T cell receptor, gammadelta T cell receptor, and gammadelta T cell subsets. Am J Reprod Immunol. (1997) 37:492–502. doi: 10.1111/j.1600-0897.1997.tb00265.x
86. Rhodes KA, Andrew EM, Newton DJ, Tramonti D, Carding SR. A subset of IL-10-producing gammadelta T cells protect the liver from Listeria-elicited, CD8(+) T cell-mediated injury. Eur J Immunol. (2008) 38:2274–83. doi: 10.1002/eji.200838354
87. Fan DX, Duan J, Li MQ, Xu B, Li DJ, Jin LP. The decidual gamma-delta T cells up-regulate the biological functions of trophoblasts via IL-10 secretion in early human pregnancy. Clin Immunol. (2011) 141:284–92. doi: 10.1016/j.clim.2011.07.008
88. Kuhl AA, Pawlowski NN, Grollich K, Blessenohl M, Westermann J, Zeitz M, et al. Human peripheral gammadelta T cells possess regulatory potential. Immunology. (2009) 128:580–8. doi: 10.1111/j.1365-2567.2009.03162.x
89. Stockis J, Lienart S, Colau D, Collignon A, Nishimura SL, Sheppard D, et al. Blocking immunosuppression by human Tregs in vivo with antibodies targeting integrin alphaVbeta8. Proc Natl Acad Sci USA. (2017) 114:E10161–8. doi: 10.1073/pnas.1710680114
90. Siegers GM. Integral Roles for Integrins in gammadelta T Cell Function. Front Immunol. (2018) 9:521. doi: 10.3389/fimmu.2018.00521
91. Aymeric L, Apetoh L, Ghiringhelli F, Tesniere A, Martins I, Kroemer G, et al. Tumor cell death and ATP release prime dendritic cells and efficient anticancer immunity. Cancer Res. (2010) 70:855–8. doi: 10.1158/0008-5472.CAN-09-3566
92. Allard B, Longhi MS, Robson SC, Stagg J. The ectonucleotidases CD39 and CD73: novel checkpoint inhibitor targets. Immunol Rev. (2017) 276:121–44. doi: 10.1111/imr.12528
93. Elliott MR, Chekeni FB, Trampont PC, Lazarowski ER, Kadl A, Walk SF, et al. Nucleotides released by apoptotic cells act as a find-me signal to promote phagocytic clearance. Nature. (2009) 461:282–6. doi: 10.1038/nature08296
94. Silva-Vilches C, Ring S, Mahnke K. ATP and its metabolite adenosine as regulators of dendritic cell activity. Front Immunol. (2018) 9:2581. doi: 10.3389/fimmu.2018.02581
95. La Sala A, Ferrari D, Corinti S, Cavani A, Di Virgilio F, Girolomoni G. Extracellular ATP induces a distorted maturation of dendritic cells and inhibits their capacity to initiate Th1 responses. J Immunol. (2001) 166:1611–7. doi: 10.4049/jimmunol.166.3.1611
96. Antonioli L, Pacher P, Vizi ES, Hasko G. CD39 and CD73 in immunity and inflammation. Trends Mol Med. (2013) 19:355–67. doi: 10.1016/j.molmed.2013.03.005
97. Bastid J, Cottalorda-Regairaz A, Alberici G, Bonnefoy N, Eliaou JF, Bensussan A. ENTPD1/CD39 is a promising therapeutic target in oncology. Oncogene. (2013) 32:1743–51. doi: 10.1038/onc.2012.269
98. Otsuka A, Hanakawa S, Miyachi Y, Kabashima K. CD39: a new surface marker of mouse regulatory gammadelta T cells. J Allergy Clin Immunol. (2013) 132:1448–51. doi: 10.1016/j.jaci.2013.05.037
99. Bastid J, Regairaz A, Bonnefoy N, Dejou C, Giustiniani J, Laheurte C, et al. Inhibition of CD39 enzymatic function at the surface of tumor cells alleviates their immunosuppressive activity. Cancer Immunol Res. (2015) 3:254–65. doi: 10.1158/2326-6066.CIR-14-0018
100. Liang D, Zuo A, Zhao R, Shao H, Born WK, O'brien RL, et al. CD73 Expressed on gammadelta T cells shapes their regulatory effect in experimental autoimmune uveitis. PLoS ONE. (2016) 11:e0150078. doi: 10.1371/journal.pone.0164502
101. Sakuishi K, Apetoh L, Sullivan JM, Blazar BR, Kuchroo VK, Anderson AC. Targeting Tim-3 and PD-1 pathways to reverse T cell exhaustion and restore anti-tumor immunity. J Exp Med. (2010) 207:2187–94. doi: 10.1084/jem.20100643
103. Hannani D, Ma Y, Yamazaki T, Dechanet-Merville J, Kroemer G, Zitvogel L. Harnessing gammadelta T cells in anticancer immunotherapy. Trends Immunol. (2012) 33:199–206. doi: 10.1016/j.it.2012.01.006
104. Bonneville M, Scotet E. Human Vgamma9Vdelta2 T cells: promising new leads for immunotherapy of infections and tumors. Curr Opin Immunol. (2006) 18:539–46. doi: 10.1016/j.coi.2006.07.002
105. Sugie T, Murata-Hirai K, Iwasaki M, Morita CT, Li W, Okamura H, et al. Zoledronic acid-induced expansion of gammadelta T cells from early-stage breast cancer patients: effect of IL-18 on helper NK cells. Cancer Immunol Immunother. (2013) 62:677–87. doi: 10.1007/s00262-012-1368-4
106. Bennouna J, Bompas E, Neidhardt EM, Rolland F, Philip I, Galea C, et al. Phase-I study of Innacell gammadelta, an autologous cell-therapy product highly enriched in gamma9delta2 T lymphocytes, in combination with IL-2, in patients with metastatic renal cell carcinoma. Cancer Immunol Immunother. (2008) 57:1599–609. doi: 10.1007/s00262-008-0491-8
107. Nakajima J, Murakawa T, Fukami T, Goto S, Kaneko T, Yoshida Y, et al. A phase I study of adoptive immunotherapy for recurrent non-small-cell lung cancer patients with autologous gammadelta T cells. Eur J Cardiothorac Surg. (2010) 37:1191–7. doi: 10.1016/j.ejcts.2009.11.051
108. Kobayashi H, Tanaka Y, Yagi J, Minato N, Tanabe K. Phase I/II study of adoptive transfer of gammadelta T cells in combination with zoledronic acid and IL-2 to patients with advanced renal cell carcinoma. Cancer Immunol Immunother. (2011) 60:1075–84. doi: 10.1007/s00262-011-1021-7
109. Sakamoto M, Nakajima J, Murakawa T, Fukami T, Yoshida Y, Murayama T, et al. Adoptive immunotherapy for advanced non-small cell lung cancer using zoledronate-expanded gammadeltaTcells: a phase I clinical study. J Immunother. (2011) 34:202–11. doi: 10.1097/CJI.0b013e318207ecfb
110. Hoeres T, Holzmann E, Smetak M, Birkmann J, Wilhelm M. PD-1 signaling modulates interferon-gamma production by Gamma Delta (gammadelta) T-Cells in response to leukemia. Oncoimmunology. (2019) 8:1550618. doi: 10.1080/2162402X.2018.1550618
111. Castella B, Foglietta M, Sciancalepore P, Rigoni M, Coscia M, Griggio V, et al. Anergic bone marrow Vgamma9Vdelta2 T cells as early and long-lasting markers of PD-1-targetable microenvironment-induced immune suppression in human myeloma. Oncoimmunology. (2015) 4:e1047580. doi: 10.1080/2162402X.2015.1047580
112. Castella B, Melaccio A, Foglietta M, Riganti C, Massaia M. Vgamma9Vdelta2 T cells as strategic weapons to improve the potency of immune checkpoint blockade and immune interventions in human myeloma. Front Oncol. (2018) 8:508. doi: 10.3389/fonc.2018.00508
113. Oberg HH, Kellner C, Gonnermann D, Sebens S, Bauerschlag D, Gramatzki M, et al. Tribody [(HER2)2xCD16] is more effective than trastuzumab in enhancing gammadelta T cell and natural killer cell cytotoxicity against HER2-expressing cancer cells. Front Immunol. (2018) 9:814. doi: 10.3389/fimmu.2018.00814
114. Marcu-Malina V, Heijhuurs S, Van Buuren M, Hartkamp L, Strand S, Sebestyen Z, et al. Redirecting alphabeta T cells against cancer cells by transfer of a broadly tumor-reactive gammadeltaT-cell receptor. Blood. (2011) 118:50–9. doi: 10.1182/blood-2010-12-325993
115. Dombrowski KE, Ke Y, Brewer KA, Kapp JA. Ecto-ATPase: an activation marker necessary for effector cell function. Immunol Rev. (1998) 161:111–8. doi: 10.1111/j.1600-065X.1998.tb01575.x
116. Dwyer KM, Deaglio S, Gao W, Friedman D, Strom TB, Robson SC. CD39 and control of cellular immune responses. Purinergic Signal. (2007) 3:171–80. doi: 10.1007/s11302-006-9050-y
117. Regateiro FS, Cobbold SP, Waldmann H. CD73 and adenosine generation in the creation of regulatory microenvironments. Clin Exp Immunol. (2013) 171:1–7. doi: 10.1111/j.1365-2249.2012.04623.x
118. Bono MR, Fernandez D, Flores-Santibanez F, Rosemblatt M, Sauma D. CD73 and CD39 ectonucleotidases in T cell differentiation: beyond immunosuppression. FEBS Lett. (2015) 589:3454–60. doi: 10.1016/j.febslet.2015.07.027
119. Cuende J, Lienart S, Dedobbeleer O, Van Der Woning B, De Boeck G, Stockis J, et al. Monoclonal antibodies against GARP/TGF-beta1 complexes inhibit the immunosuppressive activity of human regulatory T cells in vivo. Sci Transl Med. (2015) 7:284ra256. doi: 10.1126/scitranslmed.aaa1983
Keywords: γδ T cells, cancer, pro-tumor functions, immunosuppression, therapy
Citation: Chabab G, Barjon C, Bonnefoy N and Lafont V (2020) Pro-tumor γδ T Cells in Human Cancer: Polarization, Mechanisms of Action, and Implications for Therapy. Front. Immunol. 11:2186. doi: 10.3389/fimmu.2020.02186
Received: 22 June 2020; Accepted: 11 August 2020;
Published: 16 September 2020.
Edited by:
Khashayarsha Khazaie, Mayo Clinic College of Medicine & Science, United StatesReviewed by:
Serena Meraviglia, University of Palermo, ItalyXinhui Wang, Massachusetts General Hospital and Harvard Medical School, United States
Copyright © 2020 Chabab, Barjon, Bonnefoy and Lafont. This is an open-access article distributed under the terms of the Creative Commons Attribution License (CC BY). The use, distribution or reproduction in other forums is permitted, provided the original author(s) and the copyright owner(s) are credited and that the original publication in this journal is cited, in accordance with accepted academic practice. No use, distribution or reproduction is permitted which does not comply with these terms.
*Correspondence: Virginie Lafont, dmlyZ2luaWUubGFmb250QGluc2VybS5mcg==
†These authors share first authorship
‡Present address: Clément Barjon, Duve Institute, UCLouvain, Brussels, Belgium
§These authors share last authorship