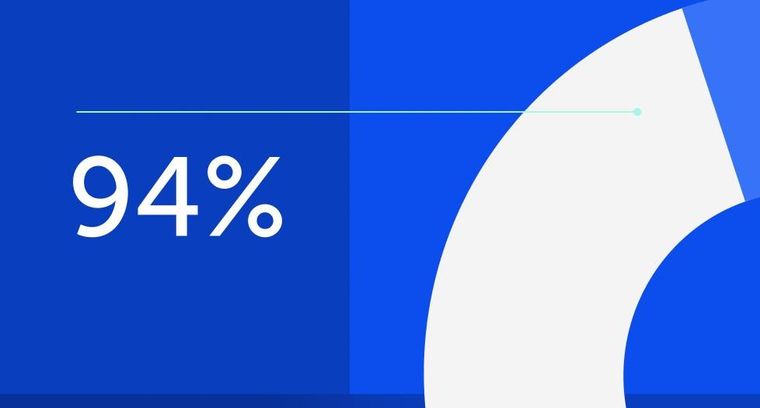
94% of researchers rate our articles as excellent or good
Learn more about the work of our research integrity team to safeguard the quality of each article we publish.
Find out more
ORIGINAL RESEARCH article
Front. Immunol., 28 July 2020
Sec. Autoimmune and Autoinflammatory Disorders
Volume 11 - 2020 | https://doi.org/10.3389/fimmu.2020.01569
This article is part of the Research TopicFocusing on T-cells for Novel Treatments of Systemic Lupus ErythematosusView all 12 articles
CD138 (syndecan 1), a member of the heparan-sulfate proteoglycan family, regulates diverse biological responses by interacting with chemokines, cytokines, growth factors, and adhesion molecules. Expression of CD138 has been detected on T cells from both healthy and sick mice mimicking systemic lupus erythematosus (SLE) disease. However, the characteristics and the role of CD138+ T cells in SLE pathogenesis remain largely unknown. We analyzed the lupus-prone MRL/Lpr mice and the control MRL/MpJ strain as well as the common laboratory strains Balb/c, and C57BL/6 for CD138-expression and found that only the MRL/Lpr strain harbored TCRβ+CD138+ cells in various organs. The frequency of TCRβ+CD138+ cells progressively expanded in MRL/Lpr mice with age and correlated with disease severity. Majority of the TCRβ+CD138+ cells were CD4 and CD8 double-negative and 20% were CD4. At least a portion of TCRβ+CD138+ cells originated from CD4+ cells because substantial number of CD4+TCRβ+CD138- cells expressed CD138 after in vitro cultivation. Compared to TCRβ+CD138- cells, TCRβ+CD138+ cells exhibited central memory (Tcm) phenotype with reduced ability to proliferate and produce the cytokines IFNγ and IL-17. When co-cultured with B cells, the ability of TCRβ+CD138+ cells to promote plasma cell formation and autoreactive antibody production was dependent on the presence of autoantigen, CD4 co-receptor expression and cell-to-cell contact. Surprisingly, adoptively transferred TCRβ+CD138+ T cells slowed down disease progression in young recipient MRL/Lpr mice but had the opposite effect when DNA was co-administered with TCRβ+CD138+ T cells or when TCRβ+CD138+ cells were transferred to older MRL/Lpr mice with established disease. Thus, CD138-expressing T cells with Tcm phenotype enhance disease progression in SLE by rapidly activating autoreactive B cells when self-antigens are exposed to the immune system.
Systemic lupus erythematosus (SLE) is an autoimmune disease characterized by the production of autoantibodies and inflammatory cell infiltration in multiple tissues (1). T cells are critical for SLE pathogenesis as they, not only provide help to autoreactive B cells, but also infiltrate and damage the target organs, such as the skin, joints, brain, lung, heart, and kidneys (2). T cells from SLE patients and lupus-prone mice have expanded T helper 1 (Th1), Th17, follicular, and extrafollicular helper T (Tfh and eTfh) cell subsets, which contribute to the inflammation and autoreactive antibody production through increased IFNγ, TNFα, IL-6, IL-17, and IL-21 secretion (3–7). Diminished populations of T regulatory cells (Treg), T follicular regulatory (Tfr) cells, and cytotoxic CD8+ T cells were also thought to be contributing to the pathogenesis of SLE (8–11). In addition, increased circulating TCRαβ+CD4-CD8- double negative T (dnT) cells and renal accumulation of the minor γδT population have been associated with autoantibody production and lupus nephritis (12). Besides the phenotypic and functional alterations in effector T cells, terminally differentiated memory T cells may also be contributing to the tissue damage as these cells accumulate in SLE patients with high disease activity (13–15).
Syndecans, type I transmembrane heparan sulfate proteoglycans (HSPG), regulate diverse biological processes, such as tissue wound repair, angiogenesis, epithelial-mesenchymal transformation, and inflammation, by modifying the local concentration, stability, and accessibility of extracellular matrix components, cytokines, chemokines, and growth factors (16). Syndecan family consists of four distinct members that are mostly expressed on epithelial, endothelial, neural, or fibroblastic cells, but they are also detected on haemopoietic cells (17, 18). Syndecan 2 and syndecan 4 are up-regulated upon CD4+ T cell activation and act as inhibitors by promoting T cell receptor (TCR) clearance or by activating tyrosine phosphatase CD148 (19, 20). Syndecan 1 (CD138) is commonly used as a marker to identify plasmablasts and plasma cells (21). Recently, CD138 has been suggested to be a marker to distinguish IL-17 producing natural killer T17 (NKT17) cells from other invariant NKT cells based on its selective expression on NKT17 cells but not on NKT1 and NKT2 cells (22, 23). Moreover, CD138+ T cells were observed accumulated in gut epithelia of aged C3H wild type as well as in the spleen and lymph nodes of FasL loss-of-function C3H gld mice (24). Similarly, CD138-expressing T cells were detected in spleen and lymph nodes of lupus prone μMT/lpr mouse but these cells were only present in the lymph nodes, and not in the spleen, of another lupus-prone strain, B6/lpr mouse (25). Thus, accumulating evidence indicate the existence of CD138+ T cells in both healthy and diseased mice. However, the characteristics and pathologic roles of CD138+ T cells in lupus disease remain to be elucidated.
Here, we detected the presence of TCRβ+CD138+ cells in various organs of the lupus-prone MRL/Lpr mice. The numbers of TCRβ+CD138+ cells increased as the disease progressed. We also identified CD4+ T cells among the TCRβ+CD138- population as an important source of TCRβ+CD138+ cells. These accumulating TCRβ+CD138+ cells manifested mostly central memory phenotype (Tcm) and promoted lupus disease progression only when autoantigens were present, despite exhibiting slower activation kinetics, reduced proliferation, and diminished cytokine production after stimulation with anti-CD3/CD28 antibodies.
MRL/MpJ-Faslpr/J (referred to as MRL/Lpr throughout the manuscript), MRL/MpJ (referred to as MRL throughout the manuscript) mice, and C57BL/6 mice were purchased from The Jackson Laboratory (Bar Harbor, ME). Balb/c mice were purchased from Charles River Laboratories (Wilmington, MA). Only age-matched female mice were used for experiments. All mice were bred and maintained under specific pathogen-free conditions in the animal facility of US Food and Drug Administration (FDA), Center for Biologics Evaluation and Research (CBER) Veterinary Services. The breeding and use of animals were approved by the US FDA, CBER Institutional Animal Care and Use Committee (permit numbers 2002-37 and 2017-47).
Serum anti-dsDNA and anti-Smith antigen (SM) antibodies were measured by ELISA as described previously (26). Briefly, calf thymic DNA (Sigma-Aldrich, St. Louis, MO) or SM (Immunovision, Springdale, AR) were coated on 96-well microtiter plates (Dynatech Immulon 4 HBX; Dynatech Labs., Chantilly, VA) at 1 μg/ml with 0.1 M of carbonate-bicarbonate buffer (pH 9.6) overnight at 4°C. Plates were blocked for 30 min at room temperature in 5% BSA in PBS, then washed with 0.05% Tween-20 in PBS. Diluted serum samples were added to wells in triplicates and incubated at 37°C for 2 h. Plates were washed with 0.05% Tween-20 in PBS and further incubated with HRP-conjugated goat antibodies directed against mouse IgG (Southern Biotech, Birmingham, AL) for 1 h at room temperature. Finally, plates were washed with 0.05% Tween-20 in PBS and measured at 405 nm absorbance after developing with ABTS solution (Invitrogen, Carlsbad, CA). Antibody titers were recorded as the last titration corresponding to the OD that is twice the mean OD of blank wells.
Single cell suspensions of spleen, bone marrow, lymph nodes, and thymus were obtained by mechanic dissociation of tissue through a 40 μm cell strainer. The dissociated cells were filtered through a 100 μm cell strainer. Red blood cells were then lysed using ACK lysing buffer (Lonza, Wallersville, MD). In addition, mouse blood leukocytes were collected by lysing red blood cells with ACK lysing buffer and centrifugation at 300 × g for 5 min. Cells were stained with fluorescent-conjugated anti-mouse antibodies after blocking CD16/CD32 with Fc Block (BD Biosciences, San Jose, CA). For intracellular staining, Brefeldin A (BD Biosciences)-treated cells were stained with the surface markers and LIVE/DEAD™ Fixable Near-IR Dead cell kit (IR-Red) (Thermo Fisher, Waltham, MA) before fixation, permeabilization, and intracellular staining as per manufactures instructions (BD Biosciences). The following antibodies were used in flow cytometry analysis: Pacific blue anti-CD19, BV421 anti-CD19, BV421 anti-TCRβ, APC anti-CD138, APC anti-TCRβ, BV605 anti-CD3, FITC anti-CD3, Percp Cy5.5 anti-CD44, FITC anti-62L, PE-Cy7 anti-PD-1, APC anti-CXCR5, Percp Cy5.5 anti-B220, PE-Cy7 anti-CD8, PE anti-CD21, PE anti-CD22, BV421anti-CD23, Alexa647 anti-CD40, FITC anti-CD80, FITC anti-CD86, Percp Cy5.5 anti-CD25, FITC anti-CD69, APC anti-CD95, Percp Cy5.5 anti-IL17, Percp Cy5.5 anti-CCR7, FITC anti-Foxp3 (all purchased from BioLegend, San Diego, CA). PE-anti-CD138 was purchased from BD Biosciences. In addition, FITC anti-BCMA, PE anti-TACI, FITC anti-IFNγ, Annexin V, (R&D system, Minneapolis, MN), ATTO 488 anti-BAFFR (Enzo life Science Inc., Farmingdale, NY), CellTrace™ CFSE Cell Proliferation Kit and Qdot605 anti-CD4 antibody (Thermo Fisher). Stained cells were acquired using LSR II flow cytometer (BD Biosciences) and data were analyzed using FlowJo (Tree Star, Ashland, OR) version 10.1 for PC.
Total RNA was extracted from flow cytometry-sorted cells using the RNeasy Mini kit (Qiagen, Germantown, MD). Two hundred nanograms of total RNA were reverse-transcribed into cDNA using random hexamers with the Taqman Reverse transcription kit (Invitrogen). The expression of targeted genes and GAPDH were determined using Taqman Gene Expression assays and CFX96 Touch Real-Time System (BioRad, Hercules, CA). Relative expression values were determined by the 2-ΔCt method where samples were normalized to GAPDH gene expression.
Splenic T cells from MRL/Lpr mice were purified with Dynabeads™ FlowComp™ Mouse Pan T (CD90.2) Kit and dissocated from beads as per manufacture's instructions (Thermo Fisher). Purified T cells were staind with PE-conjugated anti-CD138 antibody, and TCRβ+CD138+ and TCRβ+CD138- cells were further separated with anti-PE magnetic MicroBeads (Miltenyi Biotec, Auburn, CA). After three washes with PBS, the purity of isolated TCRβ+CD138+ cells was >95% in all experiments as determined by flow cytometry. For in vivo transfer, purified TCRβ+CD138+ and TCRβ+CD138- cells were suspended in PBS and 1 × 107 cells in 100 μl were i.v. injected into recipient mice. For in vitro culture, CD4+TCRβ+CD138- cells were further isolated from purified TCRβ+CD138- cells using the CD4 (L3T4) MicroBeads (Miltenyi Biotec), and unbound cells were identified as CD8+TCRβ+CD138- cells (over 94% purity). To block mTOR, isolated CD4+TCRβ+CD138- cells were cultured in the presence of 100 nM rapamycin (Tocris Biosciences, Minneapolis, MN). After 3 days of incubation cell viability as well as CD138 and CD4 expression levels were assessed in flow cytometry.
Splenic B cells were isolated from 5 or 12 weeks old MRL/Lpr mice using B Cell Isolation Kit (Miltenyi Biotec). The purity of isolated B cells was over 97%. B cells were stained with CSFE before co-culturing with purified TCRβ+CD138+ or TCRβ+CD138- cells in the presence of anti-CD3/CD28 antibodies (BD Biosciences), phorbol 12-myristate 13-acetate (PMA)/ionomycin, or autoantigens [1 μg/ml of DNA or SM (Immunovision)]. DNA was isolated from MRL/Lpr splenocytes by hyperthemo treatment at 42°C for 4 h. After 3 to 4 days of incubation, cells were analyzed for CFSE dilution by flow cytometry. In other assays, after 10 days of culture, culture supernatants were analyzed for antibody production as well as IL-2 and IFNγ secretion by ELISA (R&D Systems). In CD4 blocking expriments, Ultra-LEAF™ purified CD4 antibody (clone GK1.5, Biolegend), or control rat IgG (Sigma-Aldrich) were added to T and B cell co-cultures. In some co-culture experiments, T and B cells were incubated either as mixed or separated with 0.4 μm pore sized polyester Corning Transwell® membrane insert (Sigma-Aldrich).
Nine weeks old female Balb/c or C57BL/6 mice received a single i.p. injection of 0.5 ml pristane (Sigma-Aldrich) or 0.5 ml of sterile PBS. Fourteen weeks later, sera were collected and spleens were harvested. Sera were analyzed for auto-antibodies by ELISA, and splenocytes were subjected to flow cytometry for the presence of TCRβ+CD138+ cells.
Proteinuria was measured using Fisherbrand™ Urine Reagent Strips (Fisher scientific, Hampton, NH) and scored on a scale of 0–5 (0, none; 1, trace; 2, 30 mg/dl; 3, 100 mg/dl; 4, 300 mg/dl; and 5, ≥2,000 mg/dl).
Mouse kidneys were fixed in 10% buffered formalin overnight, processed, and embedded in paraffin. Sections were processed as previously described (26). Hematoxylin and eosin (H&E) and Masson trichrome stainings were performed. Stained slides were scanned by Nanozoomer XR (Hamamatsu corporation, Japan) and data was store as ndpi files for analysis. Overall severity, glomerular sclerosis, inflammatory cell accumulation, and interstitial fibrosis were evaluated and scored semiquantatively between 0 and 3 (0 = within normal limits, 1 = mild pathology, 2 = moderate pathology, and 3 = severe pathology). Average scores were analyzed by GraphPad Prism software (GraphPad, San Diego, CA).
Data from groups were compared using GraphPad Prism software and non-parametric testing was performed by the Mann-Whitney rank sum two-tailed test for two groups and by two-way ANOVA on ranks for three or more groups. Corresponding p-values for the test data points were listed on the figure.
The presence of CD138-expressing αβ T cells has been reported in Fas and FasL mutant mouse strains (C3H gld, μMT/lpr, and B6/lpr mice) manifesting lupus-like disease, but the physiological significance of CD138-expressing T cells in lupus pathogenesis remains unexplored (24, 25). Here, we investigated the immunopathological role of CD138-expressing T cells in MRL/Lpr mouse, a widely used lupus-prone strain (27). First, we found a large fraction of CD138-expressing cells among CD19-TCRβ+ gated splenic population in MRL/Lpr mice (6 weeks old), even before the onset of autoimmune manifestations (Figure 1A, Supplemental Figure 1A). Measurement of CD138 mRNA in qRT-PCR assay on sorted TCRβ+CD138+ cells confirmed the expression of CD138 (Supplemental Figure 1B). However, the frequency of TCRβ+CD138+ cells in the spleens of age-matched Balb/c, C57BL/6 as well as the parental MRL mice remained negligible (Figure 1A). Thus, the expression of CD138 on T cells appears to be uniquely associated with Fas signaling deficiency (24, 25). Second, we investigated a possible correlation between TCRβ+CD138+ cells and the progression of disease in MRL/Lpr mice and found that the frequency of TCRβ+CD138+ cells gradually increased with the age of mice (Figure 1B). Moreover, the increase in TCRβ+CD138+ cell population was also detected in pristine-injected Balb/c and C57BL/6 mice (Supplemental Figures 1C,D), which also develop lupus-like autoimmune symptoms (27). It is important to note that although TCRβ+CD138+ cells were previously reported to be confined to the lymph nodes in B6/lpr mice (25), we found high percentage of these cells also in the thymus, spleen, lymph nodes, and blood of MRL/Lpr mice (Figure 1C). As in MRL/Lpr mice, higher frequencies of TCRβ+CD138+ cells were also found in the thymus, lymph node and blood of pristane-treated Balb/c mice (Supplemental Figure 1E). The increase in the frequency of blood TCRβ+CD138+ cells in 14-week old MRL/Lpr and pristane-treated mice suggest extensive circulation of TCRβ+CD138+ cells in lupus mice when they develop severe lupus disease.
Figure 1. TCRβ+CD138+ cells populate the spleen, lymph nodes, thymus, and blood of MRL/Lpr mice, and their numbers increase with age. In each flow cytometry experiment TCRβ+CD138+ cells were quantified after gating out dead cells and CD19+ B cells. (A) Splenic TCRβ+CD138+ cells from 6 weeks old Balb/c, C57BL/6, MRL, and MRL/Lpr mice were quantified. Representative pseudocolor plots from each mouse strain are shown. Mean percentages ± SD of five mice from two independent experiments are plotted. Two-way ANOVA test was used to calculate statistical significance. The means of the four groups were statistically significantly different (p < 0.0001) in 2-way ANOVA test. Mann Whitney test was used to calculate the exact p-value for the comparisons between MRL/Lpr and other groups. (B) Splenic TCRβ+CD138+ cells from 4, 6, 14, to 26 weeks old MRL/Lpr mice were quantified. Representative pseudocolor plot from each time point is shown. Mean percentages ± SD of five to eight mice from three separate experiments are plotted. Two tailed Mann-Whitney rank sum test was used to calculate statistical significance. (C) TCRβ+CD138+ cells in bone marrow, thymus, spleen, lymph nodes, and blood of 14 weeks old MRL/Lpr mice were quantified. Representative pseudocolor plots from each organ are shown. Mean percentages ± SD of five mice from two independent experiments are plotted.
Next, we further characterized the MRL/Lpr mice TCRβ+CD138+ cells by assessing the expression of surface markers and the genes associated with B and T cell lineages. As shown previously in μMT/lpr and B6/lpr mice (24, 25), most of the TCRβ+CD138+ cells and only about 5% of TCRβ+CD138- cells in MRL/Lpr mice expressed B220 (Supplemental Figure 2A). There was no apparent difference in the expression of other B cell-related surface proteins BAFFR, BCMA, IgM, CD21, CD23, CD40, CD80, or CD86 as well as mRNA for CD19, IGHM, Myc, tnfrsf13c (BAFFR), and tnfrsf13b (TACI) between TCRβ+CD138+ and TCRβ+CD138- cells (Supplemental Figures 2B–D). In addition, the expression levels of transcription factors (Pax5, PU1, Irf4, and Xbp1) associated with B and plasma cell-differentiation were comparable between TCRβ+CD138+ and TCRβ+CD138- cells, although Bcl-6 was higher and Prdm1 was lower in CD138-expressing cells (Supplemental Figure 2E). CD138 expression did not affect the expression of the T cell marker CD3 on TCRβ+ cells as both TCRβ+CD138+ and TCRβ+CD138- were CD3+ (Figures 2A,B). In μMT/lpr and B6/lpr mice, all TCRβ+CD138+ cells were reported to be negative for CD4 and CD8 (24, 25). Although the majority of TCRβ+CD138+ cells were negative for CD4 and CD8 in MRL/Lpr mice also, ~20% of TCRβ+CD138+ were CD4+, while only ~2% were CD8+ (Figures 2A,B). Further confirming the association of TCRβ+CD138+ cells with T-cell but not with B-cell lineage, we measured comparable levels of GATA3 and Tbet expression in TCRβ+CD138+ and TCRβ+CD138- cells, the transcription factors associated with Th2 and Th1 subsets (28), respectively (Figure 2C). Interestingly, CD138- cells expressed the Treg cell transcription factor Foxp3 but not CD138-expressing cells (Figure 2C). To assess whether CD138 expression changed over time in incubated TCRβ+ T cells, we assessed the changes in the percentage of CD138+ cells in cultured TCRβ+CD138+ and TCRβ+CD138- cells in vitro. As shown in Figure 2D, CD138 levels remained high on TCRβ+CD138+ cells throughout the 7-days culture period. By contrast, there was a gradual increase in the frequency of CD138+ cells among the cultured TCRβ+CD138- cells from day 1 to 3. We next sought to determine whether CD138+ cells emerged from CD4+TCRβ+CD138- or CD8+TCRβ+CD138- subsets. Incubation of highly purified CD4+TCRβ+CD138- and CD8+TCRβ+CD138- for 5 days indicated that a substantial number of CD138+ cells derived from CD4+ cells. As shown in Figure 2E and paralleling the kinetics of CD138 expression among the TCRβ+CD138- population in Figure 2D, a significant increase in CD138 expression was observed among the CD4+ cells on day 1 which plateaued on day 3, while the increase in CD138+ population among the CD8+ cells remained limited. These results suggested that a portion of CD4+TCRβ+CD138- cells expresses CD138 after culture.
Figure 2. Most of the TCRβ+CD138+ cells are CD4 and CD8 negative while some derive from CD4+ T cells. (A) Splenocytes were harvested from 14 weeks old MRL/Lpr mice. After pre-gating live and single cells, TCRβ+CD138+ and TCRβ+138- cells were further analyzed for CD3, CD4, and CD8 expression by flow cytometry. Representative pseudocolor plots from out of five mice are shown. (B,C) Splenocytes were collected from 14 weeks old MRL/Lpr mice. CD19+ B cells, CD19+CD138- plasmablasts, CD19-CD138+ plasma cells, CD19-TCRβ+CD138- cells, and CD19-TCRβ+CD138+ cells were sorted by flow cytometry. The mRNA expression levels of cell surface molecules TCRβ, CD4, CD8 (B) and transcription factors GATA3, Tbet, and Foxp3 (C) were quantified in Q-PCR. Mean ± SD of five to six mice from three independent experiments are plotted. (D) Splenocytes were collected from 14 weeks old MRL/Lpr mice. After removing CD19+ B cells, TCRβ+CD138-, and TCRβ+CD138+ cells were sorted with magnetic beads and then cultured separately for 5 days. Frequencies of CD138-expressing TCRβ+ cells were quantified by flow cytometry. Representative pseudocolor plots are shown. Mean percentages ± SD of six mice from three independent experiments are plotted. (E) Splenocytes were collected from 14 weeks old MRL/Lpr mice. After removing CD19+ B cells, CD4+TCRβ+CD138-, and CD8+TCRβ+CD138- cells were sorted with magnetic beads and then cultured separately for 5 days. Frequencies of CD138-expressing CD4+ and CD8+ T cells were measured by flow cytometry. Mean percentages ± SD of six mice from three separate experiments are plotted. Two tailed Mann-Whitney rank sum test was used to calculate statistical significance.
Multiple transduction pathways, including (mammalian target of rapamycin) (mTOR), phosphatidylinositol-3-kinase (PI3K), and mitogen-activated protein kinase (MAPK), control T cell survival, metabolism, and differentiation (29). Besides, elevated mTOR activity was found to control the expansion of dnT cells (30–32). Moreover, mTOR promotes the differentiation of CD138-expressing plasma cells (33). To test whether these pathways were also critical for CD138 expression on lupus T cells, we treated CD4+TCRβ+CD138- cells with rapamycin, a specific mTOR inhibitor, and assessed the increase in CD138 expression. As reported previously (34), rapamycin significantly reduced cell survival compared to media or DMSO treated cells after 3 days of incubation (Supplemental Figure 2F). As observed in Figure 2E, around 30% of the CD4+TCRβ+CD138- cells incubated in media expressed CD138 after 3 days of incubation (Supplemental Figure 2G). A similar increase in CD138 expression was observed in cells incubated in DMSO. Although, rapamycin treatment did not reduce the CD4 expression on the cell surface, as suspected, the inhibition of mTOR pathway significantly decreased both the CD138 expression level and the frequency of CD138+ cells (Supplemental Figure 2G). Thus, mTOR signaling pathway may be involved in the expression of CD138 on CD4+TCRβ+CD138- cells.
After TCR activation, T cells undergo a series of events, including up-regulation of cell surface markers, proliferation, apoptosis, and cytokine secretion (35). We assessed the differences in proliferation of TCRβ+CD138+ and TCRβ+CD138- cells following stimulation with anti-CD3/CD28 antibodies. Compared to TCRβ+CD138- cells, TCRβ+CD138+ cells proliferated significantly less at 48- and 72-h time points (Figure 3A). Stimulated cells were also assessed for apoptosis by flow cytometry. Compared to TCRβ+CD138- cells, TCRβ+CD138+ cells had higher number of live cells accompanied by lower number of early apoptotic and necrotic cells (Figure 3B). The early and late activation state of TCR-stimulated T cells were assessed by CD69 and CD25 expression, respectively (36). Overall, anti-CD3/CD28 antibody-induced activation of TCRβ+CD138+ cells were delayed compared to TCRβ+CD138- cells. At 24-h time point, CD69 expression was higher on TCRβ+CD138- cells than on TCRβ+CD138+ cells, but by 48 h its expression decreased compared to TCRβ+CD138+ cells (Figure 3C). Conversely, the increase in CD69 expression on TCRβ+CD138+ cells peaked with a delay at 48 h. A similar delay in the activation markers were observed in PMA/ionomycin-stimulated TCRβ+CD138+ cells (Supplemental Figure 3A). In SLE, activated T cells participate in the inflammatory process through the production of cytokines such as IFNγ, TNFα, and IL-17 (2–4, 7, 37, 38). We found that after TCR engagement and PMA/ionomycin stimulation more than 90% of TCRβ+CD138- cells were positive for IFNγ, while <30% of TCRβ+CD138+ cells produced IFNγ (Figure 3D, Supplemental Figures 3B,F). Similarly, TCRβ+CD138+ cells expressed less TNFα and IL-21 compared to TCRβ+CD138- cells (Supplemental Figures 3C,D). Unlike IFNγ and TNFα, IL-17 production was not different between the two subsets after TCR stimulation (Figure 3D, Supplemental Figure 3E). However, TCRβ+CD138+ cells produced less IL-17 than TCRβ+CD138- cells after PMA/ionomycin stimulation (Supplemental Figure 3F). These results indicate that the phenotype of TCRβ+CD138+ cells activated with either PMA/ionomycin or through TCR are markedly different than their CD138 deficient counterparts.
Figure 3. TCRβ+CD138+ cells proliferate less and resist early apoptosis after activation. (A) Splenic TCRβ+CD138+ and TCRβ+CD138- were sorted with magnetic beads and pre-stained with CSFE prior to stimulation with anti-CD3/CD28 antibodies for 3 days. The proliferation was assessed by flow cytometry. Representative histogram images are shown. Mean percentages ± SD of seven mice from three separate experiments are plotted. (B) Sorted splenic TCRβ+CD138+ and TCRβ+CD138- were stimulated for 4 days with anti-CD3/CD28 antibodies. Live, early apoptotic, apoptotic, and necrotic cells were measured by flow cytometry. Representative pseudocolor plots are shown. Mean percentages ± SD of six mice from three independent experiments are plotted. (C) Sorted splenic TCRβ+CD138+ and TCRβ+CD138- cells were activated with anti-CD3/CD28 antibodies for 24 or 48 h. Cells were stained with CD69 and CD25 antibodies to assess activation kinetics in flow cytometry. Representative histogram images indicating the frequency of positive cells are shown. Mean percentages ± SD of seven mice from three independent experiments are plotted. (D) Sorted splenic TCRβ+CD138+ and TCRβ+CD138- cells were activated with anti-CD3/CD28 antibodies for 16 h. Representative pseudocolor plots show intracellular IFNγ, and IL-17 staining. For each cytokine, mean ± SD of five mice are plotted. Two tailed Mann-Whitney rank sum test was used to calculate statistical significance.
An important function of T cells is to enhance antibody-mediated immunity by driving B cell proliferation and development into long lived memory B cells or antibody-secreting plasma cells (39). To investigate the capacity of TCRβ+CD138+ in helping B cells, we co-incubated splenic B cells purified from 6 weeks old (disease free) MRL/Lpr mice with splenic TCRβ+CD138+ or TCRβ+CD138- cells from 10 to 12 weeks old MRL/Lpr mice in the presence of anti-CD3/CD28 antibodies and determined the proliferation of B cells (Supplemental Figure 4A). We found significantly less proliferation of B cells co-cultured with TCRβ+CD138+ cells than those co-cultured with TCRβ+CD138- cells (Figure 4A). Although TCRβ+CD138+ cells harbor higher Bcl-6 (Supplemental Figure 2E), a transcription factor for CXCR5+PD-1+ T follicular helper (Tfh) cells, than TCRβ+CD138- cells, in B cell co-culture experiments the increase in Tfh cell numbers among TCRβ+CD138+ cells was modest and remained less than the increase in TCRβ+CD138- cells (Supplemental Figures 4A,B). Moreover, TCRβ+CD138+ cells were less efficient in inducing the generation of plasma cells (Figure 4B) and the production of IgM and IgG than TCRβ+CD138- cells (Figure 4C).
Figure 4. TCRβ+CD138+ cells are unable to promote lupus diseases development in young MRL/Lpr mice. (A–C) Sorted splenic TCRβ+CD138+ and TCRβ+CD138- cells from 10 to 12 weeks old MRL/Lpr mice were co-cultured with purified splenic B cells from 6 weeks old mice in the presence of anti-CD3/CD28 antibodies for 5 days. After gating-out TCRβ+ T cells, the proliferation of B cells (A) and the frequency of plasma cells (CD19intCD138+) (B) were measured by flow cytometry. Mean ± SD of 10 mice (A) or six mice (B) from three independent experiments are plotted. (C) Culture supernatants from the above co-culture experiment were analyzed for total IgM and IgG concentrations by ELISA. Mean ± SD of 10 mice from three independent experiments are plotted. (D,E) Splenic TCRβ+CD138+ and TCRβ+CD138- cells were sorted from 10 to 12 weeks old MRL/Lpr mice and then adoptively transferred into 7 to 8 weeks old MRL/Lpr mice without disease symptoms. (D) Autoreactive IgG antibody (dsDNA) and proteinuria levels were measured on indicated days. Mean ± SEM of 15 mice from three independent experiments are plotted. (E) Kidneys were collected 2 weeks after the transfer of cells and histopathological evaluations were performed on H&E and Masson stained specimens. Average pathology scores of 10 mice from two separate experiments are plotted. (F) Splenic TCRβ+CD138+ and TCRβ+CD138- cells were sorted from 10 to 12 weeks old MRL/Lpr mice and then adoptively transferred into 11 to 12 weeks old MRL/Lpr mice with existing disease symptoms. Autoreactive IgG antibody (dsDNA) and proteinuria levels were measured on indicated days. Mean ± SEM of 10 mice from two independent experiments are plotted. Two tailed Mann-Whitney rank sum test was used to calculate statistical significance.
To further characterize TCRβ+CD138+ cells, we tested the immunomodulatory effect of TCRβ+CD138+ cells in SLE by adoptively transferring TCRβ+CD138+ or TCRβ+CD138- cells into MRL/Lpr mice and evaluating the disease progression. We conducted the adoptive transfer experiments in two different recipient mice age groups, 7 to 8 weeks old mice with minimal lupus symptoms and 11 to 12 weeks old mice with established lupus symptoms. We chose these two age groups to assess whether the CD138-expressing cells impact the lupus progression differently in MRL/Lpr mice from different stages of disease. To our surprise, TCRβ+CD138+ cells had completely opposite effect in the recipient mice at different ages. The 7 to 8 weeks old recipient mice without lupus symptoms manifested slower progression of disease when they were transferred with TCRβ+CD138+ cells from 10 to 12 weeks old MRL/Lpr (sick) mice, compared to those that were injected with PBS. The increase in anti-dsDNA antibody and proteinuria levels were slower compared to those that were injected with PBS (Figure 4D, Supplemental Figure 4C). Kidney histopathological findings showed the most severe changes in recipients of TCRβ+CD138- group, including end stage glomeruloscrerosis with severe inflammation and interstitial fibrosis. The glomerular histopathological changes of TCRβ+CD138+ cell-recipient mice were reduced as compared to PBS-injected mice 2 weeks after the transfer of cells, but the difference in histopathological scores did not reach statistical significance (Figure 4E). However, histopathological changes were significantly less in TCRβ+CD138+ cell-injected mice than those injected with TCRβ+CD138- cells. In sharp contrast to its effect in young recipient mice, TCRβ+CD138+ cells significantly increased the development of anti-dsDNA antibodies and proteinuria in older MRL/Lpr mice with existing disease symptoms (Figures 4F, Supplemental Figure 4D). The TCRβ+CD138- cells accelerated the disease progression in recipient animals from both age groups but their impact on older recipient mice was not as pronounced as those that received TCRβ+CD138+ cells (Figures 4D–F, Supplemental Figures 4C,D). Together with the in vitro B cell co-incubation data (Figures 4A–C), the adoptive transfer experiments in young MRL/Lpr mice suggest an immunosuppressive function for TCRβ+CD138+ cells on B cell differentiation. However, TCRβ+CD138+ cells contribute to the acceleration of disease progression if the recipient host has established lupus disease.
Next, we sought to explore the underlying mechanism for the dramatic difference in the disease-stage-specific impact of TCRβ+CD138+ cells to lupus progression in the adoptive transfer experiments. An important distinction between the young and older recipient MRL/Lpr mice in the adoptive transfer experiments is the exposure of the immune system of older mice (11–12 weeks old), but not the younger (7–8 weeks old) mice, to self-antigens as a result of apoptosis (40). As observed with the younger mice in the adoptive transfer experiments, in vitro TCR stimulation of TCRβ+CD138+ cells also resulted in less activation of autoreactive B cells than those culture conditions containing TCR-stimulated TCRβ+CD138- cells. A common feature of these in vitro and in vivo experiments is the absence of auto-antigens in the system. Earlier studies have highlighted the importance of B cells in presenting auto-antigens to T cells in activating autoreactive T cells (41). We therefore repeated the in vitro co-culture experiments in the presence of apoptotic DNA instead of anti-CD3/CD28 antibodies. Interestingly, when B cells from 12 weeks old MRL/Lpr mice with established disease were co-incubated with TCRβ+CD138+ cells from age matched MRL/Lpr mice in the presence of DNA from apoptotic cells, culture supernatants contained significantly higher autoreactive (Figure 5A) and total IgG and IgM antibodies than those co-cultured with TCRβ+CD138- cells (Supplemental Figure 5A). Inclusion of DNA in the co-culture experiments resulted in a higher percentage of Tfh cell formation from TCRβ+CD138+ cells than when cells were stimulated with anti-CD3/CD28 antibodies (Supplemental Figure 4B vs. Supplemental Figure 5B). Despite this increase, the frequencies of Tfh cells among TCRβ+CD138- and TCRβ+CD138- cells were comparable (Supplemental Figure 5B). Nevertheless, consistent with the difference in antibody responses, higher percentage of plasma cells (CD3-CD19-CD138+) emerged from the cultures containing TCRβ+CD138+ cells than from the cultures with TCRβ+CD138- cells (Figure 5B). Increased plasma cell generation and autoreactive antibody production with TCRβ+CD138+ cells were not restricted to self-DNA-containing cultures, because replacement of self-DNA with the auto-antigen SM also resulted in higher percentage of plasma cell development and increased production of anti-SM antibodies with TCRβ+CD138+ cells than with TCRβ+CD138- cells (Figure 5C, Supplemental Figure 5C). Thus, regardless of the nature of the autoantigen, TCRβ+CD138+ cells are more potent in aiding autoreactive B cells to produce self-reactive antibodies than TCRβ+CD138- cells.
Figure 5. TCRβ+CD138+ cells activate autoreactive B cells when auto-antigens are included in the culture. (A–D) Sorted splenic TCRβ+CD138+ and TCRβ+CD138- cells from 12 weeks old MRL/Lpr mice were co-cultured with purified splenic B cells from the same mice for 5 days. (A) DNA was included in the co-cultured cells and culture supernatant anti-dsDNA IgM and IgG antibodies were measured by ELISA. Mean ± SD of six mice from three independent experiments are plotted. (B) T and B cells were incubated as mixed (contact) or separated with Transwell® (separate) in the presence of DNA. After gating-out TCRβ+ T cells, the differentiation of B cells into plasma cells (CD19intCD138+) was quantified by flow cytometry. Mean percentages ± SD of six mice from three independent experiments are plotted. (C) SM was included in the co-cultured cells and differentiation of B cells into plasma cells (CD19intCD138+) were quantified by flow cytometry after gating-out TCRβ+ T cells. Mean percentages ± SD of six mice from three experiments are plotted. (D) Cells were incubated in the presence of antibody against CD4 or control IgG and the differentiation of B cells into plasma cells was quantified by flow cytometry. Mean percentages ± SD of three independent experiments are plotted. (E) Splenic TCRβ+CD138+ and TCRβ+CD138- cells from 10 to 12 weeks old MRL/Lpr mice were sorted and then adoptively transferred into 5 to 6 weeks old MRL/Lpr mice with or without DNA. Mice that received PBS or DNA only served as control. Serum anti-dsDNA IgG and IgM antibody as well as proteinuria levels at indicated days were measured. Mean ± SEM of five mice are plotted. Two tailed Mann-Whitney rank sum test was used to calculate statistical significance.
We next sought to determine whether TCRβ+CD138+ cell-enhanced B cell differentiation is mediated by direct cell contact, especially because the majority of the MRL/Lpr mice TCRβ+CD138+ cells were CD4 and CD8 negative (Figure 2A). Separation of B and TCRβ+CD138+ cells in the co-culture experiments with a Transwell® system resulted in a significant reduction in plasma cell generation compared to cells cultured without a Transwell® system (Figure 5B). Moreover, although CD4+ cells constituted only ~20% of the TCRβ+CD138+ cells, the B cell help provided by TCRβ+CD138+ cells required CD4 because inclusion of anti-CD4 blocking antibodies in the co-culture system severely reduced plasma cell development and production of anti-dsDNA IgG and IgM antibodies (Figure 5D, Supplemental Figure 5D). These experiments established the CD4+TCRβ+CD138+ cells as more potent autoreactive B cell-activating T cell subset when self-antigens are present in the culture environment.
We showed that in order for TCRβ+CD138+ cells to augment autoreactive B cell responses, they need to be stimulated by B cell-presented self-antigens (Figures 5A–D). The fact that the recipient MRL/Lpr mice used in the adoptive transfer experiments in Figure 4D were too young to have sufficient amounts of circulating self-antigens, such as DNA, could be the reason why the transferred TCRβ+CD138+ cells were not activated and did not exacerbate SLE symptoms in the recipient mice. Conversely, the acceleration of disease progression in older MRL/Lpr mice after the transfer of TCRβ+CD138+ cells could be due to the presence of circulating self-antigens. To test this possibility, we co-administered TCRβ+CD138+ cells with DNA into young (5 to 6 weeks old) MRL/Lpr mice. As observed previously (Figure 4D), the increase in anti-dsDNA IgG and IgM antibodies as well as proteinuria were significantly slower in mice injected only with TCRβ+CD138+ cells compared to PBS-injected mice or control mice injected with DNA only (Figure 5E). In contrast, and as hypothesized, anti-dsDNA antibody, and proteinuria levels were significantly higher in mice co-administered with DNA and TCRβ+CD138+ cells than in control mice injected with DNA only (Figure 5E). Taken together, TCRβ+CD138+ cells can modulate lupus development in MRL/Lpr mice in a disease-stage-dependent manner; they slow down the symptoms prior to the emergence of self-antigens and accelerate the disease progression when self-antigens are exposed.
Abnormal accumulation and differentiation of memory T cells have been reported in lupus patients (13). Memory T cells confer immediate protection and mount recall responses upon reencounter with antigens. Since adoptively transferred TCRβ+CD138+ cells promoted disease progression in an autoantigen dependent manner (Figure 5), we asked whether TCRβ+CD138+ cells have a memory T cell phenotype. The circulating memory T cell compartments are divided into Tcm and effector memory T cells (Tem) subsets based on the expression of cell surface molecules, such as CD44, CD62L, and CCR7 (42). We first characterized the expression of CD44 and CD62L on splenic TCRβ+CD138+ and TCRβ+CD138- cells in 12 weeks old MRL/Lpr mice. Using these two markers, we identified CD44-CD62L+ naïve T (Tn) cells, CD44+CD62L- Tem, and CD44+CD62L+ Tcm subsets. Among the TCRβ+CD138- cells, ~10% were Tn, 50% Tem, and 40% Tcm (Figure 6A, Supplemental Figure 6A). Interestingly, Tcm cells comprised the vast majority (95%) of the population among the TCRβ+CD138+ cells (Figure 6A, Supplemental Figure 6A). Typically, memory T cells are either CD4+ or CD8+ (43), but in MRL/Lpr mice, the majority of the CD138-expressing CD4+, CD8+ as well as CD4-CD8- cells exhibited Tcm memory phenotype, based on the elevated expression of CD44 and CD62L (Supplemental Figure 6B).
Figure 6. CD138+ T cells exhibit central memory T cell phenotype. (A,B) Splenocytes were collected from 12 weeks old MRL/Lpr mice. (A) The expression of CD44 and CD62L on TCRβ+CD138- and TCRβ+CD138+ cells were measured by flow cytometry. Representative pseudocolor plots are shown. Mean percentages ± SD of five mice from two separate experiments are plotted. (B) Representative flow cytometry histogram of CCR7 expression on TCRβ+CD138- and TCRβ+CD138+ cells are shown. Mean ± SD percentages and MFIs for CCR7 expression from six mice in two separate experiments are plotted. (C) Splenic TCRβ+CD138+ and TCRβ+CD138- cells were sorted from 12 weeks old MRL/Lpr mice and BCL-6, Bim1, and Prdm1 mRNA were quantified by Q-PCR. Mean ± SD values of five mice from two separate experiments are plotted. (D) Splenic TCRβ+CD138+ and TCRβ+CD138- cells were sorted from 12 weeks old MRL/Lpr mice and cultured with anti-CD3/CD28 antibodies overnight. Culture supernatant IFNγ and IL-2 levels were measured by ELISA. Mean ± SD of nine mice from three independent experiments are plotted. (E) Splenic TCRβ+CD138+, TCRβ+CD138- cells were sorted from 12 weeks old MRL/Lpr mice and co-cultured with sorted B cells from the same mice in the presence of DNA for 3 days. Culture supernatant IFNγ and IL-2 levels were measured by ELISA. Mean ± SD of five mice from two separate experiments are plotted. (F) Cells were collected from spleen, lymph nodes, and bone marrows of 12 weeks old MRL/Lpr mice. Frequencies of Tcm (CD44+CD62L+) and Tem (CD44+CD62L-) cells among TCRβ+CD138- and TCRβ+CD138+ cells were measured in flow cytometry. Mean percentages ± SD of five mice from two independent experiments are plotted. (G) Splenocytes were collected from 12 weeks old Balb/c, C57BL/6, and MRL/Lpr mice. Frequencies of Tcm (CD44+CD62L+) and Tem (CD44+CD62L-) cells among TCRβ+CD138- and TCRβ+CD138+ cells were measured in flow cytometry. Mean percentages ± SD of five mice from two independent experiments are plotted. Two tailed Mann-Whitney rank sum test was used to calculate statistical significance.
The chemokine receptor CCR7, which enables cells to home to secondary lymphoid organs where they encounter antigen, are highly expressed on Tcm cells (44). Further confirming their Tcm phenotype, TCRβ+CD138+ cells expressed higher CCR7 levels than TCRβ+CD138- cells (Figure 6B). The Tcm and Tem memory subsets can also be distinguished based on the expression of transcription factors Bcl-6 and Bim for Tcm and BLIMP1 (Prdm1) for Tem, respectively (45, 46). We found higher Bcl-6 and Bim1 but lower Prdm1 mRNA expression in TCRβ+CD138+ cells, compared to TCRβ+CD138- cells (Figure 6C). We also assessed Tcm phenotype based on IFNγ and IL-2 production because in humans, Tcm cells produce more IL-2, while Tem cells are distinguished by high IFNγ and TNFα production (47). Consistent with data in humans upon stimulation with anti-CD3/CD28 antibodies, MRL/Lpr mice TCRβ+CD138+ cells secreted significantly higher IL-2 but lower IFNγ than TCRβ+CD138- cells (Figure 6D). The Tcm specific IL-2 and IFNγ production profile in TCRβ+CD138+ cells were also observed in co-culture system with autoantigens and B cells (Figure 6E). Moreover, we found that in MRL/Lpr mice, the Tcm phenotype of TCRβ+CD138+ cells were not restricted to the spleen or the age because cells isolated from various organs of mice at different ages, all predominantly exhibited the Tcm phenotype (Figure 6F, Supplemental Figures 6B,C). Collectively, these data established the memory phenotype of TCRβ+CD138+ cells as Tcm in MRL/Lpr mice. Finally, to assess whether the Tcm phenotype of TCRβ+CD138+ is unique to MRL/Lpr mouse, we compared the memory phenotype of TCRβ+CD138+ cells from Balb/c and C57BL/6 mice to those of MRL/Lpr mice. Again, regardless of the mouse strain, TCRβ+CD138+ were CD44+CD62L+ Tcm cells (Figure 6G). Thus, the Tcm phenotype of TCRβ+CD138+ cells is conserved among different mouse strains.
In this study, we uncovered a disease-stage dependent accumulation of TCRβ+CD138+ cells in various organs of lupus-prone MRL/Lpr mice, which are overwhelmingly positive for B220, but negative for CD4 and CD8 expression. Although these cells are less efficient in responding to non-specific T cell stimuli, such as PMA/ionomycin and anti-CD3/CD28 antibody engagement, they are more potent in aiding antibody production from autoreactive B cells in vitro as well as in vivo when autoantigens are present. Further characterization established the phenotype of these cells as Tcm based on high expression levels of CD62L, CD44, CCR7, and Bcl-6.
CD138 is widely expressed on epithelial cells as well as on other adherent cells, but its expression on normal lymphoid cells has been thought to be restricted to plasma cells and pre-B cells. However, recent studies reported that CD138 is also present on NKT17 and GC B cells, where it may be involved in host defense or autoimmunity through IL-17 secretion or binding to death receptor 6 on Tfh cells (22, 48). In aged C3H mice, accumulation of CD138-expressing T cells was shown to be restricted to the gut epithelium, although they can expand to peripheral organs, such as lymph nodes and spleen when Fas ligand (gld) is ablated. Similar to C3H gld mice, a large population of CD138-expressing T cells accumulate in peripheral organs of Fas receptor mutant μMT/Lpr, B6/Lpr (25) and MRL/Lpr mice (Figure 1A). As previously described, the majority of CD138+ T cells also express CD3 and B220 and are negative for CD4 and CD8 (dnT). Previous reports suggested that dnT cells derive from exhausted autoreactive CD8+ cells or continuously stimulated CD8+ cells (49, 50). Differing from these reports, our observations reveal that a substantial portion of the TCRβ+CD138+ cells in MRL/Lpr mice are converted from CD4+ cells rather than CD8+ cells, as CD138+ cells emerged from in vitro cultured CD4+ cells, but not from CD8+ cells (Figure 2D). Interestingly, we found that the exposure of TCRβ+CD138- cells to rapamycin severely reduced the increase in CD138 expression on these cells (Supplemental Figure 2H). Rapamycin was also reported to inhibit the generation of CD138-expressing plasma cells (33). These observations point to mTOR-mediated regulation of CD138 expression on lymphocytes. mTOR is also implicated in the pathogenesis of SLE, especially because systemic rapamycin administration alleviates lupus symptoms (51, 52). Also, inhibition of mTOR is shown to reduce the production of dnT cells and favors the expansion of therapeutic CD4+CD25+Foxp3+ Treg cells in lupus-prone mice and SLE patients (30–32). Further studies will be needed to test whether the beneficial effect of rapamycin in SLE is through its inhibition of CD138 expression on T cells.
Studies in normal mice or autoimmune-prone lpr mice have shown that dnT cells are able to dampen CD4+ and CD8+ T cell-mediated autoimmune responses both in vitro and in vivo (53, 54). Consistent with these studies, adoptively transferred TCRβ+CD138+ T cells, of which the majority were CD4-CD8- cells, slowed down the disease progression in young MRL/Lpr mice when auto-antigens are not exposed. The TCRβ+CD138+ cell-mediated suppression of disease progression is unlikely to be due to the apoptosis of host CD4+ and CD8+ T cells induced by the transferred cells as this mechanism requires functional Fas-FasL interaction (54). Suppression of dnT cell-mediated alloimmune responses are also attributed to elevated levels of perforin and granzyme B produced by these cells (55). This mechanism is also unlikely to be at play in the slowing down of disease progression by TCRβ+CD138+ cells in young MRL/Lpr mice because we found comparable levels of perforin and granzyme B production by TCRβ+CD138+ and TCRβ+CD138- cells (Supplemental Figure 7). Although the exact molecular mechanism and cascade of events need to be deciphered, we augur that the suboptimal proliferation capacity of TCRβ+CD138+ cells as well as their diminished production of pathogenic cytokines, IL-17, TNFα, and IFNγ (56) may be responsible for the delay in disease progression afforded by these cells in young MRL/Lpr mice.
In active lupus patients or NZBxSWR mice, CD4+ as well as dnT cells augment the production of anti-dsDNA antibodies when they are co-cultured with oligoclonal autoreactive B cells (41, 57, 58). Consistent with these early observations, both TCRβ+CD138+ and TCRβ+CD138- cells enhanced plasma cell development and amplified autoreactive antibody production from MRL/Lpr mice B cells when auto-antigens were present. However, TCRβ+CD138+ were more potent than TCRβ+CD138- cells in activating autoreactive B cells both in vitro and in vivo. Interestingly, CD4-expressing TCRβ+CD138+ cells were responsible for the activation of autoreactive B cells, despite comprising <20% percent of the total TCRβ+CD138+ population. This rapid recall response upon antigen re-encounter is a typical characteristic of memory T cells (42, 47). Indeed, compared to TCRβ+CD138- cells, over 90% of TCRβ+CD138+ cells are CD44+CD62L+ Tcm cells. Although the other Tcm marker CCR7 is also higher on TCRβ+CD138+ cells than on TCRβ+CD138- cells, the frequency of CCR7- expressing TCRβ+CD138+ cells is <50%. The discrepancy between the percentage of CD44+CD62L+ cells and CCR7+ cells may be due to possible loss of CCR7 expression on TCRβ+CD138+ cells after repeated exposure to autoantigens, a phenomenon reported for CCR7+CD27+ memory T cells in lupus patients which lose CCR7 expression following repeated stimulation (13). Interestingly, clinical studies indicate that the expansion of dnT cells as well as the CD62L- CD197- effector-memory T cells and the CD62L+CD197+ central-memory cells are controlled by mTOR since rapamycin treatment decreased these populations in SLE patients (31, 32). The predominance of TCRβ+CD138+ cells presenting with memory phenotype and the suppression of CD138-expression with rapamycin (Supplemental Figure 2H) in MRL/Lpr mice suggest that mTOR mediated expansion of CD138-expressing memory T cells may be associated with lupus pathogenesis in MRL/Lpr mice as well as in SLE patients. Of note, the Tcm characteristic of TCRβ+CD138+ cells is independent of disease activity as TCRβ+CD138+ cells from healthy MRL/Lpr mice as well as different healthy mouse strains also exhibit similar phenotype. Thus, TCRβ+CD138+ cells manifest Tcm phenotype, regardless of mouse strain and disease status.
Taken together, we have identified and characterized the phenotype of TCRβ+CD138+ cells in MRL/Lpr mice and unveiled a pathogenic role for these cells in lupus disease. Both in vivo adoptive transfer experiments and in vitro co-culture experiments underpin the role of TCRβ+CD138+ cells in activating autoreactive B cells when autoantigens are exposed. Although how CD138 expression renders TCRβ+ T cells more pathogenic is still enigmatic, the discovery of a novel Tcm subset with enhanced pathogenic properties may be useful in assessing and monitoring disease severity.
All datasets presented in this study are included in the article/Supplementary Material.
The animal study was reviewed and approved by US FDA, CBER Institutional Animal Care and Use Committee.
LL and MA conceived the study and contributed to the manuscript writing. LL and KT did the laboratory work. LL, KT, and MA did the statistical analysis and contributed to the data analysis and interpretation. All authors read and approved the manuscript.
The authors declare that the research was conducted in the absence of any commercial or financial relationships that could be construed as a potential conflict of interest.
This manuscript has been released as a pre-print at bioRxiv (59). The authors thank Drs. Mark Kukuruga and Adovi Akue from CBER Flow Cytometry Core Facility. The authors also thank Drs. Roberto De Pascalis (US FDA/CBER) and Isabelle Coppens (Johns Hopkins University) for editorial review of the manuscript.
The Supplementary Material for this article can be found online at: https://www.frontiersin.org/articles/10.3389/fimmu.2020.01569/full#supplementary-material
1. Tsokos GC. Systemic lupus erythematosus. N Engl J Med. (2011) 365:2110–21. doi: 10.1056/NEJMra1100359
2. Suarez-Fueyo A, Bradley SJ, Tsokos GC. T cells in systemic lupus erythematosus. Curr Opin Immunol. (2016) 43:32–38. doi: 10.1016/j.coi.2016.09.001
3. Akahoshi M, Nakashima H, Tanaka Y, Kohsaka T, Nagano S, Ohgami E, et al. Th1/Th2 balance of peripheral T helper cells in systemic lupus erythematosus. Arthritis Rheum. (1999) 42:1644–8. doi: 10.1002/1529-013119990842:81644::AID-ANR123.0.CO;2-L
4. Koga T, Ichinose K, Tsokos GC. T cells and IL-17 in lupus nephritis. Clin Immunol. (2017) 185:95–99. doi: 10.1016/j.clim.2016.04.010
5. Linterman MA, Rigby RJ, Wong RK, Yu D, Brink R, Cannons JL, et al. Follicular helper T cells are required for systemic autoimmunity. J Exp Med. (2009) 206:561–76. doi: 10.1084/jem.20081886
6. Odegard JM, Marks BR, DiPlacido LD, Poholek AC, Kono DH, Dong C, et al. ICOS-dependent extrafollicular helper T cells elicit IgG production via IL-21 in systemic autoimmunity. J Exp Med. (2008) 205:2873–86. doi: 10.1084/jem.20080840
7. Takahashi S, Fossati L, Iwamoto M, Merino R, Motta R, Kobayakawa T, et al. Imbalance towards Th1 predominance is associated with acceleration of lupus-like autoimmune syndrome in MRL mice. J Clin Invest. (1996) 97:1597–604. doi: 10.1172/JCI118584
8. Valencia X, Yarboro C, Illei G, Lipsky PE. Deficient CD4+CD25high T regulatory cell function in patients with active systemic lupus erythematosus. J Immunol. (2007) 178:2579–88. doi: 10.4049/jimmunol.178.4.2579
9. Scalapino KJ, Tang Q, Bluestone JA, Bonyhadi ML, Daikh DI. Suppression of disease in New Zealand Black/New Zealand white lupus-prone mice by adoptive transfer of ex vivo expanded regulatory T cells. J Immunol. (2006) 177:1451–9. doi: 10.4049/jimmunol.177.3.1451
10. Xu B, Wang S, Zhou M, Huang Y, Fu R, Guo C, et al. The ratio of circulating follicular T helper cell to follicular T regulatory cell is correlated with disease activity in systemic lupus erythematosus. Clin Immunol. (2017) 183:46–53. doi: 10.1016/j.clim.2017.07.004
11. Filaci G, Bacilieri S, Fravega M, Monetti M, Contini P, Ghio M, et al. Impairment of CD8+ T suppressor cell function in patients with active systemic lupus erythematosus. J Immunol. (2001) 166:6452–7. doi: 10.4049/jimmunol.166.10.6452
12. Yin S, Mao Y, Li X, Yue C, Zhou C, Huang L, et al. Hyperactivation and in situ recruitment of inflammatory Vdelta2 T cells contributes to disease pathogenesis in systemic lupus erythematosus. Sci Rep. (2015) 5:14432. doi: 10.1038/srep14432
13. Fritsch RD, Shen X, Illei GG, Yarboro CH, Prussin C, Hathcock KS, et al. Abnormal differentiation of memory T cells in systemic lupus erythematosus. Arthritis Rheum. (2006) 54:2184–97. doi: 10.1002/art.21943
14. Sen Y, Chunsong H, Baojun H, Linjie Z, Qun L, San J, et al. Aberration of CCR7 CD8 memory T cells from patients with systemic lupus erythematosus: an inducer of T helper type 2 bias of CD4 T cells. Immunology. (2004) 112:274–89. doi: 10.1111/j.1365-2567.2004.01862.x
15. Zhou H, Hu B, Huang N, Mo X, Li W, Zhang B, et al. Aberrant T cell subsets and cytokines expression profile in systemic lupus erythematosus. Clin Rheumatol. (2018) 37:2405–13. doi: 10.1007/s10067-018-4124-0
16. Couchman JR. Syndecans: proteoglycan regulators of cell-surface microdomains? Nat Rev Mol Cell Biol. (2003) 4:926–37. doi: 10.1038/nrm1257
17. Alexopoulou AN, Multhaupt HA, Couchman JR. Syndecans in wound healing, inflammation and vascular biology. Int J Biochem Cell Biol. (2007) 39:505–28. doi: 10.1016/j.biocel.2006.10.014
18. Kim CW, Goldberger OA, Gallo RL, Bernfield M. Members of the syndecan family of heparan sulfate proteoglycans are expressed in distinct cell-, tissue-, development-specific patterns. Mol Biol Cell. (1994) 5:797–805. doi: 10.1091/mbc.5.7.797
19. Chung JS, Dougherty I, Cruz PD Jr, Ariizumi K. Syndecan-4 mediates the coinhibitory function of DC-HIL on T cell activation. J Immunol. (2007) 179:5778–84. doi: 10.4049/jimmunol.179.9.5778
20. Rovira-Clave X, Angulo-Ibanez M, Noguer O, Espel E, Reina M. Syndecan-2 can promote clearance of T-cell receptor/CD3 from the cell surface. Immunology. (2012) 137:214–25. doi: 10.1111/j.1365-2567.2012.03626.x
21. Calame KL. Plasma cells: finding new light at the end of B cell development. Nat Immunol. (2001) 2:1103–8. doi: 10.1038/ni1201-1103
22. Dai H, Rahman A, Saxena A, Jaiswal AK, Mohamood A, Ramirez L, et al. Syndecan-1 identifies and controls the frequency of IL-17-producing naive natural killer T (NKT17) cells in mice. Eur J Immunol. (2015) 45:3045–51. doi: 10.1002/eji.201545532
23. Jaiswal AK, Sadasivam M, Hamad ARA. Syndecan-1-coating of interleukin-17-producing natural killer T cells provides a specific method for their visualization and analysis. World J Diabetes. (2017) 8:130–4. doi: 10.4239/wjd.v8.i4.130
24. Mohamood AS, Bargatze D, Xiao Z, Jie C, Yagita H, Ruben D, et al. Fas-mediated apoptosis regulates the composition of peripheral alphabeta T cell repertoire by constitutively purging out double negative T cells. PLoS ONE. (2008) 3:e3465. doi: 10.1371/journal.pone.0003465
25. Seagal J, Leider N, Wildbaum G, Karin N, Melamed D. Increased plasma cell frequency and accumulation of abnormal syndecan-1plus T-cells in Igmu-deficient/lpr mice. Int Immunol. (2003) 15:1045–52. doi: 10.1093/intimm/dxg107
26. Liu L, Allman WR, Coleman AS, Takeda K, Lin TL, Akkoyunlu M. Delayed onset of autoreactive antibody production and M2-skewed macrophages contribute to improved survival of TACI deficient MRL-Fas/Lpr mouse. Sci Rep. (2018) 8:1308. doi: 10.1038/s41598-018-19827-8
27. Celhar T, Fairhurst AM. Modelling clinical systemic lupus erythematosus: similarities, differences and success stories. Rheumatology. (2017) 56:i88–99. doi: 10.1093/rheumatology/kew400
28. Jenner RG, Townsend MJ, Jackson I, Sun K, Bouwman RD, Young RA, et al. The transcription factors T-bet and GATA-3 control alternative pathways of T-cell differentiation through a shared set of target genes. Proc Natl Acad Sci USA. (2009) 106:17876–81. doi: 10.1073/pnas.0909357106
29. Moulton VR, Tsokos GC. Abnormalities of T cell signaling in systemic lupus erythematosus. Arthritis Res Ther. (2011) 13:207. doi: 10.1186/ar3251
30. Kato H, Perl A. Mechanistic target of rapamycin complex 1 expands Th17 and IL-4+ CD4-CD8- double-negative T cells and contracts regulatory T cells in systemic lupus erythematosus. J Immunol. (2014) 192:4134–44. doi: 10.4049/jimmunol.1301859
31. Lai ZW, Borsuk R, Shadakshari A, Yu J, Dawood M, Garcia R, et al. Mechanistic target of rapamycin activation triggers IL-4 production and necrotic death of double-negative T cells in patients with systemic lupus erythematosus. J Immunol. (2013) 191:2236–46. doi: 10.4049/jimmunol.1301005
32. Lai ZW, Kelly R, Winans T, Marchena I, Shadakshari A, Yu J, et al. Sirolimus in patients with clinically active systemic lupus erythematosus resistant to, or intolerant of, conventional medications: a single-arm, open-label, phase 1/2 trial. Lancet. (2018) 391:1186–96. doi: 10.1016/S0140-67361830485-9
33. Benhamron S, Pattanayak SP, Berger M, Tirosh B. mTOR activation promotes plasma cell differentiation and bypasses XBP-1 for immunoglobulin secretion. Mol Cell Biol. (2015) 35:153–66. doi: 10.1128/MCB.01187-14
34. Hung CM, Garcia-Haro L, Sparks CA, Guertin DA. mTOR-dependent cell survival mechanisms. Cold Spring Harb Perspect Biol. (2012) 4:ea008771. doi: 10.1101/cshperspect.a008771
35. Chen L, Flies DB. Molecular mechanisms of T cell co-stimulation and co-inhibition. Nat Rev Immunol. (2013) 13:227–42. doi: 10.1038/nri3405
36. Caruso A, Licenziati S, Corulli M, Canaris AD, De Francesco MA, Fiorentini S, et al. Flow cytometric analysis of activation markers on stimulated T cells and their correlation with cell proliferation. Cytometry. (1997) 27:71–6. doi: 10.1002/SICI1097-03201997010127:171::AID-CYTO93.0.CO;2-O
37. Raphael I, Nalawade S, Eagar TN, Forsthuber TG. T cell subsets and their signature cytokines in autoimmune and inflammatory diseases. Cytokine. (2015) 74:5–17. doi: 10.1016/j.cyto.2014.09.011
38. Wong CK, Lit LC, Tam LS, Li EK, Wong PT, Lam CW. Hyperproduction of IL-23 and IL-17 in patients with systemic lupus erythematosus: implications for Th17-mediated inflammation in auto-immunity. Clin Immunol. (2008) 127:385–93. doi: 10.1016/j.clim.2008.01.019
39. Crotty S. A brief history of T cell help to B cells. Nat Rev Immunol. (2015) 15:185–9. doi: 10.1038/nri3803
40. Stone RC, Feng D, Deng J, Singh S, Yang L, Fitzgerald-Bocarsly P, et al. Interferon regulatory factor 5 activation in monocytes of systemic lupus erythematosus patients is triggered by circulating autoantigens independent of type I interferons. Arthritis Rheum. (2012) 64:788–98. doi: 10.1002/art.33395
41. Mamula MJ, Fatenejad S, Craft J. B cells process and present lupus autoantigens that initiate autoimmune T cell responses. J Immunol. (1994) 152:1453–61.
42. Sallusto F, Lenig D, Forster R, Lipp M, Lanzavecchia A. Two subsets of memory T lymphocytes with distinct homing potentials and effector functions. Nature. (1999) 401:708–12. doi: 10.1038/44385
43. Mueller SN, Gebhardt T, Carbone FR, Heath WR. Memory T cell subsets, migration patterns, tissue residence. Annu Rev Immunol. (2013) 31:137–61. doi: 10.1146/annurev-immunol-032712-095954
44. Lugt BV, Tubo NJ, Nizza ST, Boes M, Malissen B, Fuhlbrigge RC, et al. CCR7 plays no appreciable role in trafficking of central memory CD4 T cells to lymph nodes. J Immunol. (2013) 191:3119–27. doi: 10.4049/jimmunol.1200938
45. Pepper M, Pagan AJ, Igyarto BZ, Taylor JJ, Jenkins MK. Opposing signals from the Bcl6 transcription factor and the interleukin-2 receptor generate T helper 1 central and effector memory cells. Immunity. (2011) 35:583–95. doi: 10.1016/j.immuni.2011.09.009
46. Ichii H, Sakamoto A, Arima M, Hatano M, Kuroda Y, Tokuhisa T. Bcl6 is essential for the generation of long-term memory CD4+ T cells. Int Immunol. (2007) 19:427–33. doi: 10.1093/intimm/dxm007
47. Farber DL, Yudanin NA, Restifo NP. Human memory T cells: generation, compartmentalization and homeostasis. Nat Rev Immunol. (2014) 14:24–35. doi: 10.1038/nri3567
48. Fujikura D, Ikesue M, Endo T, Chiba S, Higashi H, Uede T. Death receptor 6 contributes to autoimmunity in lupus-prone mice. Nat Commun. (2017) 8:13957. doi: 10.1038/ncomms13957
49. Crispin JC, Tsokos GC. Human TCR-alpha beta+ CD4- CD8- T cells can derive from CD8+ T cells and display an inflammatory effector phenotype. J Immunol. (2009) 183:4675–81. doi: 10.4049/jimmunol.0901533
50. Mehal WZ, Crispe IN. TCR ligation on CD8+ T cells creates double-negative cells in vivo. J Immunol. (1998) 161:1686–93.
51. Warner LM, Adams LM, Sehgal SN. Rapamycin prolongs survival and arrests pathophysiologic changes in murine systemic lupus erythematosus. Arthritis Rheum. (1994) 37:289–97. doi: 10.1002/art.1780370219
52. Fernandez D, Bonilla E, Mirza N, Niland B, Perl A. Rapamycin reduces disease activity and normalizes T cell activation-induced calcium fluxing in patients with systemic lupus erythematosus. Arthritis Rheum. (2006) 54:2983–8. doi: 10.1002/art.22085
53. Hamad AR, Mohamood AS, Trujillo CJ, Huang CT, Yuan E, Schneck JP. B220+ double-negative T cells suppress polyclonal T cell activation by a Fas-independent mechanism that involves inhibition of IL-2 production. J Immunol. (2003) 171:2421–6. doi: 10.4049/jimmunol.171.5.2421
54. Ford MS, Young KJ, Zhang Z, Ohashi PS, Zhang L. The immune regulatory function of lymphoproliferative double negative T cells in vitro and in vivo. J Exp Med. (2002) 196:261–7. doi: 10.1084/jem.20020029
55. Zhang D, Yang W, Degauque N, Tian Y, Mikita A, Zheng XX. New differentiation pathway for double-negative regulatory T cells that regulates the magnitude of immune responses. Blood. (2007) 109:4071–9. doi: 10.1182/blood-2006-10-050625
56. Lourenco EV, La Cava A. Cytokines in systemic lupus erythematosus. Curr Mol Med. (2009) 9:242–54. doi: 10.2174/156652409787847263
57. Sainis K, Datta SK. CD4+ T cell lines with selective patterns of autoreactivity as well as CD4- CD8- T helper cell lines augment the production of idiotypes shared by pathogenic anti-DNA autoantibodies in the NZB x SWR model of lupus nephritis. J Immunol. (1988) 140:2215–24.
58. Shivakumar S, Tsokos GC, Datta SK. T cell receptor alpha/beta expressing double-negative (CD4-/CD8-) and CD4+ T helper cells in humans augment the production of pathogenic anti-DNA autoantibodies associated with lupus nephritis. J Immunol. (1989) 143:103–12.
Keywords: lupus, syndecan-1, T cells, MRL/Lpr mouse, immunopathogenesis
Citation: Liu L, Takeda K and Akkoyunlu M (2020) Disease Stage-Specific Pathogenicity of CD138 (Syndecan 1)-Expressing T Cells in Systemic Lupus Erythematosus. Front. Immunol. 11:1569. doi: 10.3389/fimmu.2020.01569
Received: 10 April 2020; Accepted: 15 June 2020;
Published: 28 July 2020.
Edited by:
Masayuki Mizui, Osaka University Graduate School of Medicine, JapanReviewed by:
Onkar Prakash Kulkarni, Birla Institute of Technology and Science, IndiaCopyright © 2020 Liu, Takeda and Akkoyunlu. This is an open-access article distributed under the terms of the Creative Commons Attribution License (CC BY). The use, distribution or reproduction in other forums is permitted, provided the original author(s) and the copyright owner(s) are credited and that the original publication in this journal is cited, in accordance with accepted academic practice. No use, distribution or reproduction is permitted which does not comply with these terms.
*Correspondence: Mustafa Akkoyunlu, bXVzdGFmYS5ha2tveXVubHVAZmRhLmhocy5nb3Y=
Disclaimer: All claims expressed in this article are solely those of the authors and do not necessarily represent those of their affiliated organizations, or those of the publisher, the editors and the reviewers. Any product that may be evaluated in this article or claim that may be made by its manufacturer is not guaranteed or endorsed by the publisher.
Research integrity at Frontiers
Learn more about the work of our research integrity team to safeguard the quality of each article we publish.