- Department of Immunology, Medical University of Warsaw, Warsaw, Poland
B-cell malignancies are a heterogeneous group of hematological neoplasms derived from cells at different stages of B-cell development. Recent studies revealed that dysregulated redox metabolism is one of the factors contributing to the pathogenesis and progression of B-cell malignancies. Elevated levels of oxidative stress markers usually correlate with the advanced stage of various B-cell malignancies. In the complex tumor microenvironment, reactive oxygen species affect not only malignant cells but also bystander cells, including immune cells. Importantly, malignant cells, due to genetic dysregulation, are able to adapt to the increased demands for energy and reducing equivalents via metabolic reprogramming and upregulation of antioxidants. The immune cells, however, are more sensitive to oxidative imbalance. This may cause their dysfunction, leading to immune evasion and tumor progression. On the other hand, the already imbalanced redox homeostasis renders malignant B-cells particularly sensitive to further elevation of reactive oxygen species. Indeed, targeting antioxidant systems has already presented anti-leukemic efficacy in preclinical models. Moreover, the prooxidant treatment that triggers immunogenic cell death has been utilized to generate autologous anti-leukemic vaccines. In this article, we review novel research on the dual role of the reactive oxygen species in B-cell malignancies. We highlight the mechanisms of maintaining redox homeostasis by malignant B-cells along with the antioxidant shield provided by the microenvironment. We summarize current findings regarding therapeutic targeting of redox metabolism in B-cell malignancies. We also discuss how the oxidative stress affects antitumor immune response and how excessive reactive oxygens species influence anticancer prooxidant treatments and immunotherapies.
Introduction
Oxidative metabolism is vital for all aerobic organisms. Reactive oxygen species (ROS) formed during the oxidative metabolism are important signaling molecules. However, to maintain redox homeostasis, the levels of ROS must be tightly controlled. Oxidative stress is defined as an imbalance between the generation or uptake of ROS and their scavenging. Exaggerated, prolonged oxidative stress leads to the oxidative macromolecule damage and cell death.
Dysregulated redox homeostasis is a hallmark of cancer and may lead to higher steady-state levels of ROS in cancer cells (1, 2). This is caused by the elevated production of ROS due to oncogene activation and intensified oxidative metabolism in cancer cells, as well as by ROS generated extrinsically, within the tumor microenvironment (TME). Although dysregulation of redox homeostasis has been mainly studied in solid tumors, it also occurs in hematological malignancies. Increased levels of ROS were demonstrated in many B-cell malignancies including B-cell acute lymphoblastic leukemia (B-ALL), chronic lymphocytic leukemia (CLL), and B-cell lymphomas (3–6). Noteworthy, B-cell-derived malignant cells are particularly sensitive to prooxidant treatments, which may be caused by their specific metabolic reprogramming (7, 8) or reliance on selected antioxidant pathways (9, 10). Recent studies revealed that also the TME shapes the redox homeostasis. Selected stromal cells of the TME, e.g., mesenchymal stem cells, aid malignant cells to alleviate oxidative stress, supporting their survival (11, 12). Other cells, such as macrophages or polymorphonuclear subset of myeloid-derived suppressor cells (PMN-MDSCs), generate high amounts of ROS, contributing to elevation of ROS levels in the TME (13, 14). This may negatively affect the function of effector immune cells, causing impairment of antitumor immune response.
Here we review novel findings of the manifold effects of steady-state and therapy-induced ROS on B-cell malignancies, including their direct influence on malignant and normal cells' survival and/or function. We also discuss the possible consequences of increased ROS levels on the effectiveness of anticancer therapies, mainly prooxidant treatments and immunotherapies. The multifaceted effects of ROS on B-cell malignancies are presented in Figure 1.
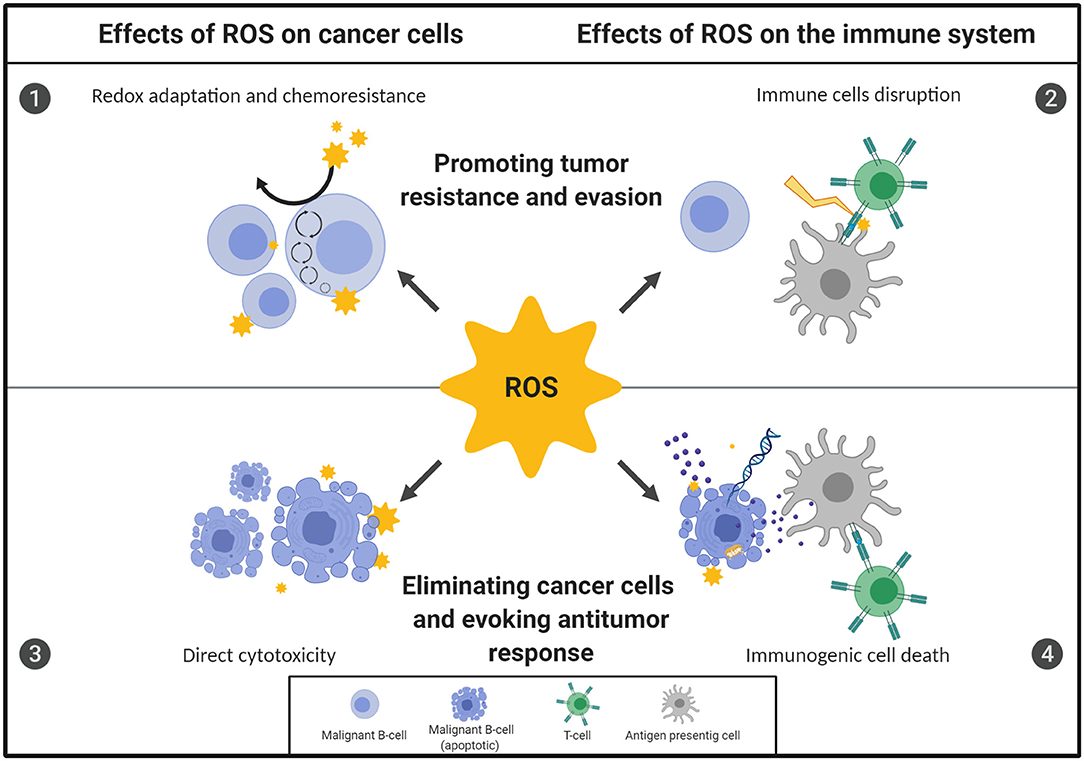
Figure 1. Oxidative stress displays a dual role in the fate of B-cell malignancies. The ultimate outcome of redox imbalance on B-cell malignancies depends on the source, concentration, location of reactive oxygen species (ROS), as well as the duration of exposure. Importantly, the overall effect of ROS on the fate of B-cell neoplasms depends on the action of ROS both on malignant B-cells and on the cells of the TME, including immune cells. However, malignant cells adapt to the increased demands for energy and reducing equivalents by increasing their antioxidant capacity and rewiring their metabolic energy pathways, which renders them therapy-resistant [1]. At the same time, the highly oxidative microenvironment may negatively affect function of the effector immune cells, causing impairment of antitumor immune response, contributing to immune evasion and further tumor progression [2]. Conversely, targeting specific antioxidant pathways in malignant B cells or inhibiting the antioxidant support provided by the TME trigger direct, oxidative-stress mediated cytotoxicity and can effectively eradicate malignant B cells [3]. Moreover, some forms of oxidative stress-mediated cell death under certain conditions are immunogenic. This leads to the activation of immune cells and subsequent development of antitumor response, what further contributes to the efficacy of prooxidant treatments [4]. The figure was created using BioRender.com.
Intrinsic and Extrinsic Sources of ROS in B-Cells Malignancies
Elevated ROS levels and subsequent oxidative stress are features of various B-cell malignancies, which in majority of cases correlate with the clinical stage of the disease and patients' survival. The accumulation of oxidation products of phospholipids and/or DNA in serum as well as elevated levels of intracellular ROS in malignant B cells were observed in patients suffering from CLL (4, 15, 16). Recently, the increased levels of oxidative stress biomarkers were also found in B-ALL (6) and different types of non-Hodgkin lymphoma (NHL) (3).
ROS come from a number of sources and are generated both by malignant cells (intrinsic) as well as the cells of the TME, nutrients, pollutants or therapies (extrinsic). Intrinsic ROS are by-products of metabolic processes such as mitochondrial oxidative phosphorylation, fatty acid oxidation (FAO), and protein folding in the endoplasmic reticulum. In humans there are over 40 different ROS-generating enzymes including various NADPH oxidases (NOXs), cytochrome P450, superoxide dismutases (SOD), and others (17–19). In CLL, the elevated ROS have been linked to increased mitochondrial biogenesis and activity (4). Although NOXs are not responsible for ROS overproduction in CLL cells (4), NOX5 is specifically expressed in hairy cell leukemia and generates H2O2, which promotes oncogenic signaling (20). High amounts of ROS also come from FAO occurring in peroxisomes and mitochondria. The lipoprotein lipase along with peroxisome proliferator activated receptor (PPAR)α are elevated in CLL, drive FAO and reprogram malignant cells to use lipids as an alternative energy source (21, 22). Other types of B-cell malignancies also display enhanced FAO. For instance, a subtype of diffuse large B-cell lymphoma (DLBCL) depends on oxidative phosphorylation and FAO for its survival and growth (23).
In addition to enhanced ROS generation, the oxidative stress in B-cell malignancies results from diminished capacity of antioxidant systems (24). Indeed, decreased activity of SOD2 and catalase (CAT) has been reported in CLL and DLBCL patients compared to healthy individuals (16, 25). Reduced CAT expression along with increased amounts of mitochondria were found in less aggressive CLL subtype (26).
Apart from cancer cells, other cells of the TME, in particular myeloid cells, may contribute to ROS production, mainly via NOX-dependent manner (27, 28). The accumulation of selected populations of monocytes and their immunosuppressive reprogramming were reported in CLL patients (29, 30) as well as in the murine Eμ-TCL1 model of human CLL (31). Moreover, Manukyan and co-workers observed functional alterations in circulating neutrophils in CLL patients including phenotype change and elevated ROS production (32).
How Malignant B-Cells Cope With Elevated ROS Levels?
The effects of ROS on cancer cells vary depending on their concentrations (33). Low ROS levels may activate malignant B-cell proliferation through stimulation of B-Cell Receptor (BCR) and pre-BCR signaling (34), while higher levels induce apoptosis. To prevent oxidative stress and subsequent cell death due to elevated ROS levels, cells utilize numerous antioxidants. They include both small non-enzymatic molecules, such as glutathione (GSH), bilirubin, ferritin, NADPH, as well as antioxidant enzymes, e.g., thioredoxins (TXNs), thioredoxin reductases (TXNRDs), peroxiredoxins (PRDXs), CAT, SODs, heme oxygenase (HO-1) and a plethora of GSH-dependent enzymes (35, 36). The renewal of reduced GSH, TXN and other antioxidant molecules relies on cellular supplies of NADPH, which is primarily generated via pentose phosphate pathway (PPP).
Various transcription factors controlling redox balance are operating in selected B-cell malignancies. Signaling related to nuclear factor erythroid 2-related factor 2 (NRF2), a master regulator orchestrating antioxidant response, is enhanced in primary CLL (37) and mantle cell lymphoma (38) cells. Enzymes most frequently activated in B-cell malignancies belong to the TXN family. PRDX1 and PRDX2 are upregulated in Burkitt lymphoma cells (39), PRDX1 and PRDX3 in primary CLL cells (10, 40), PRDX1 and TXN1 in B-ALL cells (6), and TXN1 in the OXPHOS-DLBCL (41). The increase of antioxidant response in B-ALL occurs also via serine/threonine-protein phosphatase 2A (PP2A), which negatively regulates protein kinase B (AKT) and thus activates other key transcription factors promoting antioxidant response—the Forkhead box proteins (FOXO1 and FOXO3a). The antioxidant capacity in the B-ALL cells is further supported by NADPH production via activation of PPP, which is also mediated by PP2A (8).
TME and Stromal Support in Maintaining Redox Homeostasis
Bone marrow niche plays a crucial role in survival and progression of B-cell malignancies. Primary CLL and B-ALL cells undergo apoptosis when grown in vitro without stromal support (40, 42). The co-cultures with stromal cell lines, primary mesenchymal stem cells (MSC) (6) or adipocytes (43), promote survival of primary CLL and B-ALL cells and increase their resistance to therapies (43, 44). Tumor-stroma interactions occur on many levels (45). Recent studies highlight the key role of stromal cells in alleviating oxidative stress in malignant B-cells (40). The stromal support can be delivered directly, by providing antioxidants, or indirectly, by inducing antioxidant response in malignant B-cells.
It has been found that TXN1 secreted by stromal cells in the CLL lymph nodes, promoted proliferation and survival of the primary CLL cells (12). In another study, the MSC in the bone marrow aided CLL cells by uptake of cystine via Xc- transporter and subsequent secretion of cysteine, which was then used by malignant cells to synthetize GSH and overcome oxidative stress conditions (11). The depletion of the external cysteine by recombinant cysteinase in the Eμ-TCL1 mice resulted in significantly prolonged median survival time of the mice, confirming the crucial role of the MSC-derived cysteine in leukemia progression (46). Similarly, a dependence on stromal cysteine support was also reported in B-ALL (47). The mechanisms of stromal redox support in lymphomas are less thoroughly documented, although there is some evidence that the DLBCL cells may be aided by GSH received from fibroblastic reticular cells (48).
Stromal cells can also reduce oxidative stress and protect from ROS-inducing chemotherapy by transfer of organelles to leukemic cells via tunneling nanotubes (TNTs). These cellular extensions act as bridges between cancer and stromal cells that enable intercellular transport (49, 50). Activated stromal cells transmitted mitochondria to B-ALL cells using TNT and protected B-ALL cells from cytarabine-induced apoptosis (44). However, the exact mechanism of this protection remains unclear. Presumably, it is associated with triggering of adaptive antioxidant signaling.
By comparing the transcriptomes of primary CLL cells grown in a monoculture or a co-culture with HS5 stromal cells, Yosifov et al. observed a significant differences in the expression of genes involved in ROS generation, ROS detoxification, and hypoxic signaling (40). Noteworthy, the CLL samples displaying the “co-culture-like” gene expression signature correlated with significantly worse patients' survival (40).
Alleviation of oxidative stress in the leukemic niche can also occur as a result of communication between malignant cells and stromal cells using extracellular vesicles. B-ALL cells metabolically reprogrammed stromal cells via secretion of extracellular vesicles, switching their main energy pathway from oxidative phosphorylation to aerobic glycolysis (51). Such alterations are likely to favor tumor survival by reducing oxidative stress in the microenvironment. A similar mechanism of exosome-driven metabolic reprogramming has also been discovered in CLL (52).
Therapeutic Targeting of Redox Pathways in B-Cell Malignancies
The dependence of malignant B-cells on antioxidants can be utilized in therapy. Treatments based on the generation of excessive ROS, so called “prooxidant,” are selectively toxic to malignant B-cells and some of them exert antitumor effects in vitro and in vivo, mainly in preclinical models. Inhibition of the TXN system with auranofin or SK053 selectively killed malignant B-cells and displayed anti-leukemic activity in animal models of CLL (53) and B-ALL (6). Some efficacy against B-cell malignancies has been also demonstrated for agents interfering GSH and L-Cys (46, 54). Although blocking of a single antioxidant pathway may result in some toxicity to malignant B-cells, it is usually at best partially effective (6, 53). Inhibitors of the antioxidant systems more potently kill malignant B-cells when administered in combinations with other drugs, and particularly so in combination with ROS-generating agents such as L-ascorbate (10).
Another way to evoke excessive oxidative stress in malignant B-cells is to block the antioxidant shield provided by the microenvironment. Approaches which proved effective in preclinical models include inhibition of Xc- cystine transporter with sulfasalazine (55) as well as blocking of TNTs formation and transfer of mitochondria to B-ALL cells by inhibiting microtubule polymerization with vincristine (44). The recently presented, unbiased approach aimed to find agents capable of blocking stroma-mediated protection identified ouabain and emetine, drugs perturbing redox homeostasis. Along with the increase in mitochondrial ROS levels in CLL cells and depletion of cellular NADPH pool, emetine delayed CLL development in Eμ-TCL1 murine model (40).
The important feature of prooxidant approaches is their substantial B-lineage selectivity. Although the underlying mechanisms are not fully understood, some explanations were proposed. It was shown that thiol-reactive compounds activated NRF2-mediated signaling less extensively in malignant and normal B-cells as compared to other subpopulations of leukocytes and were more cytotoxic to CLL cells (37). The unique sensitivity of B-cells to dysregulation of redox homeostasis has been recently attributed to B-cell-specific metabolic reprograming. B-lymphoid transcription factors PAX5 and IKZF1 were shown to repress the key enzymes of the PPP, which resulted in insufficient generation of NADPH. To salvage oxidative stress, malignant B-cells upregulate PP2A, a key enzyme which redirects glucose metabolism to PPP. Accordingly, inhibition of PP2A delayed development of several different B-cell malignancies and had little effect on myeloid leukemia (8).
How Oxidative Stress Affects Immune Response?
The effects of ROS on immune system are at least two-sided. Prooxidant therapies are potent inducers of immunogenic cell death (ICD) in malignant cells. However, prolonged oxidative stress may also negatively affect the functions of selected populations of immune effector cells. The concept of ICD has altered the traditional view of non-immunogenic apoptosis and revealed that some forms of cell death better stimulate immune response than others. In the cancer field, dying cells undergoing ICD elicit robust, coordinated innate and adaptive immune response against tumor-specific antigens. ICD and the molecular processes that define immunogenicity are summarized here (56). A number of prooxidant treatments such as ionizing radiation, photodynamic therapy, ROS and endoplasmic reticulum stress-inducing chemotherapy or drugs interfering with cancer-specific energy metabolism, have been tested for their ability to induce ICD and stimulate antitumor immune response against different types of cancers [for further reading see (57–59)]. Only some of these ROS-inducing treatment modalities have been investigated in B-cell malignancies. In CLL cells, the PPARα antagonists increased TNFα and decreased IL-10 release, as well as stimulated T cell proliferation in allogenic mixed lymphocytic reaction (22). However, the most extensively tested in the context of triggering immunogenicity of B-cell malignancies is ionizing radiation. As demonstrated in murine models of B-cell lymphoma, the time of local irradiation delivery has significant impact on the immunogenicity and the overall treatment efficacy. Only the irradiation delivered in an accelerated mode triggered ICD, increased infiltration of CD4+ and CD8+T cells, dendritic cells (DC), decreased the number of regulatory T cells (Tregs) in the TME and resulted in tumor regression (60). Ionizing radiation has been also used to generate antitumor vaccines. In NHL cell lines, ionizing radiation combined with heat shock, promoted expression of genes associated with antigen processing and presentation. The treatment was used to generate DC-based autologous vaccines against relapsed NHL. Although the clinical response was observed only in one third of patients, it correlated with the ability of malignant cells to undergo ICD (61). Similar approach has been already tested in CLL. Partial response, manifested by enhanced T cell proliferation and increase in a co-stimulatory molecule CD80 in CLL cells, was detected in one third of patients and correlated with lack of disease progression (62). Overall, the observed response to oxidized vaccines was relatively weak and the follow-up clinical trials have not been undertaken.
Apart from the direct cytotoxic effects on cancer cells and a positive impact on the immune response by inducing ICD, ROS can affect the course of the disease and therapy outcome by directly interacting with immune cells. Numerous findings accumulated over years (summarized in Table 1) have identified various effects of high ROS levels on different populations of immune cells. The chronic oxidative stress observed in B-cell malignancies may contribute to the immunosuppressive microenvironment and be a component of the immune evasion by malignant B-cells. Furthermore, it may negatively affect the efficacy of immunotherapies involving immune cell populations amenable to oxidative stress-mediated dysfunctions (e.g., therapeutic monoclonal antibodies, cellular therapies with autologous cytotoxic immune cells). The effects of oxidative stress on immune cells were not comprehensively studied in B-cell malignancies so far and the investigations are mostly limited to CLL. NK cells are the population of innate immune cells with remarkable sensitivity to negative ROS effects (73). CLL patients with higher ROS levels had elevated proportion of CD56brightCD16dim NK cells in their circulation (4), the subpopulation which is characterized by significantly smaller cytotoxic activity (81). Alterations in conventional T lymphocytes were also observed in CLL patients with high ROS levels. Both CD4+ and CD8+T cells had reduced expression of CD3ζ, a key subunit of T cell receptor. Additionally, CD4+T cells' activation markers (CD69, HLA-DR, and CD137) were decreased. When CD4+T cells were in vitro stimulated for proliferation and activation in the presence of primary CLL cells, the addition of a ROS scavenger, N-acetylcysteine, significantly increased the expression of the activation markers and IFNy production in the T cells (4).
The oxidative imbalance also entails changes in other T cell subpopulations. In CLL patients it is associated with reduced number of memory T cells and a shift toward Th9 cells. The Th9 cells are a subpopulation of CD4+T cells, characterized by the secretion of IL-9, the cytokine linked with leukemogenesis (82). Tregs are another CD4+T cell subpopulation which is enriched in B-cell malignancies (83, 84). In CLL, the increase in Tregs correlated with disease progression (85). It was shown that Tregs survive better in the highly oxidative TME than conventional CD4+ T cells (80), yet it remains to be elucidated whether the accumulation of Tregs in B-cell malignancies is related to increased oxidative stress.
Another important question is how treatment-related oxidative stress, which is stronger but more transient, affects antitumor immune response. It was demonstrated that anti-CD20 therapeutic monoclonal antibodies, rituximab and ofatumumab, induced potent ROS release from human monocytes cultured in vitro in the presence of primary CLL cells (72). Importantly, the mAbs triggered NK cell apoptosis when the NK cells were co-cultured with ROS-producing monocytes, reducing the efficacy of antibody-dependent cellular cytotoxicity (ADCC) and antitumor efficacy (72). These results suggest that the immunosuppressive ROS may also be generated in CLL patients during mAbs immunotherapy and may diminish its efficacy. Indeed, accumulation of immunosuppressive myeloid cell populations were detected both in murine model of CLL as well as in CLL patients (30).
Concluding Remarks
In recent years, a growing number of studies reports dysregulated redox homeostasis in various B-cell malignancies and the unique vulnerability of malignant B-cells to undergo oxidative stress-mediated apoptosis. We observe significant progress in our understanding of key antioxidant pathways in specific subtypes of B-cell malignancies. As exaggerated oxidative stress leads to apoptosis, this may be therapeutically utilized, as it renders malignant B cells susceptible to antioxidant system inhibitors and other prooxidant treatments.
Although prooxidant therapeutic approaches have already proved effective in preclinical models of B-cell malignancies, their effective use in patients requires further research. This is mainly caused by the multifaceted effects of ROS on the immune system. On one hand, ROS trigger ICD of malignant B cells, which has been utilized in the production of autologous vaccines against CLL and lymphomas. ICD may also contribute to the overall effectiveness of prooxidant therapies used in vivo, due to the stimulation of the antitumor immune response. However, prolonged exposure to high ROS levels may negatively affect the viability and function of immune effector cells, which diminishes antitumor immune response and undermines the effects of therapy. To date, in B-cell malignancies only a few studies confirm that ROS may impair viability and effector functions of NK and T cell. However, it is still poorly understood how oxidative stress contributes to the immunosuppression observed in advanced B-cell malignancies, and even more so, how oxidative stress influences the development of an antitumor immune response as a result of ROS-inducing therapies. Further studies are needed to investigate the effects of ROS elicited by prooxidant therapies on antitumor immune response in B-cell malignancies. These studies should include both immunocompetent and immunodeficient animal models, as well as monitoring the function of immune cells during clinical studies testing the effects of prooxidant therapies in patients with B-cell malignancies.
Author Contributions
MF developed the idea for the topic of the article. MF, AG, and KD contributed to the final conception, literature search, writing, editing, and critical revision of the manuscript. KD prepared a figure and a table. All authors contributed to the article and approved the submitted version.
Funding
This work was supported by grants from the National Science Centre, Poland 2016/21/B/NZ7/02041 (MF) and from the Polish Ministry of Science and Higher Education within Regional Initiative of Excellence program (013/RID/2018/19).
Conflict of Interest
The authors declare that the research was conducted in the absence of any commercial or financial relationships that could be construed as a potential conflict of interest.
References
1. Szatrowski TP, Nathan CF. Production of large amounts of hydrogen peroxide by human tumor cells. Cancer Res. (1991) 51:794–8.
2. Liou G-Y, Storz P. Reactive oxygen species in cancer. Free Radic Res. (2010) 44:479–96. doi: 10.3109/10715761003667554
3. Nojima J, Motoki Y, Tsuneoka H, Kuratsune H, Matsui T, Yamamoto M, et al. “Oxidation stress index” as a possible clinical marker for the evaluation of non-Hodgkin lymphoma. Br J Haematol. (2011) 155:528–30. doi: 10.1111/j.1365-2141.2011.08719.x
4. Jitschin R, Hofmann AD, Bruns H, Giessl A, Bricks J, Berger J, et al. Mitochondrial metabolism contributes to oxidative stress and reveals therapeutic targets in chronic lymphocytic leukemia. Blood. (2014) 123:2663–72. doi: 10.1182/blood-2013-10-532200
5. Battisti V, Maders LDK, Bagatini MD, Santos KF, Spanevello RM, Maldonado PA, et al. Measurement of oxidative stress and antioxidant status in acute lymphoblastic leukemia patients. Clin Biochem. (2008) 41:511–8. doi: 10.1016/j.clinbiochem.2008.01.027
6. Fidyt K, Pastorczak A, Goral A, Szczygiel K, Fendler W, Muchowicz A, et al. Targeting the thioredoxin system as a novel strategy against B-cell acute lymphoblastic leukemia. Mol Oncol. (2019) 13:1180–95. doi: 10.1002/1878-0261.12476
7. Chen Q, Espey MG, Sun AY, Pooput C, Kirk KL, Krishna MC, et al. Pharmacologic doses of ascorbate act as a prooxidant and decrease growth of aggressive tumor xenografts in mice. Proc Natl Acad Sci USA. (2008) 105:11105–9. doi: 10.1073/pnas.0804226105
8. Xiao G, Chan LN, Klemm L, Braas D, Chen Z, Geng H, et al. B-cell-specific diversion of glucose carbon utilization reveals a unique vulnerability in B cell malignancies. Cell. (2018) 173:470–84.e18. doi: 10.1016/j.cell.2018.02.048
9. Zhou Y, Hileman EO, Plunkett W, Keating MJ, Huang P. Free radical stress in chronic lymphocytic leukemia cells and its role in cellular sensitivity to ROS-generating anticancer agents. Blood. (2003) 101:4098–104. doi: 10.1182/blood-2002-08-2512
10. Graczyk-Jarzynka A, Goral A, Muchowicz A, Zagozdzon R, Winiarska M, Bajor M, et al. Inhibition of thioredoxin-dependent H2O2 removal sensitizes malignant B-cells to pharmacological ascorbate. Redox Biol. (2019) 21:101062. doi: 10.1016/j.redox.2018.11.020
11. Zhang W, Trachootham D, Liu J, Chen G, Pelicano H, Garcia-Prieto C, et al. Stromal control of cystine metabolism promotes cancer cell survival in chronic lymphocytic leukemia. Nat Cell Biol. (2012) 14:276–86. doi: 10.1038/ncb2432
12. Bäckman E, Bergh A-C, Lagerdahl I, Rydberg B, Sundström C, Tobin G, et al. Thioredoxin, produced by stromal cells retrieved from the lymph node microenvironment, rescues chronic lymphocytic leukemia cells from apoptosis in vitro. Haematologica. (2007) 92:1495–504. doi: 10.3324/haematol.11448
13. Ostrand-Rosenberg S, Fenselau C. Myeloid-derived suppressor cells: immune-suppressive cells that impair antitumor immunity and are sculpted by their environment. J Immunol. (2018) 200:422–31. doi: 10.4049/jimmunol.1701019
14. Gabrilovich DI. Myeloid-derived suppressor cells. Cancer Immunol Res. (2017) 5:3–8. doi: 10.1158/2326-6066.CIR-16-0297
15. Collado R, Ivars D, Oliver I, Tormos C, Egea M, Miguel A, et al. Increased oxidative damage associated with unfavorable cytogenetic subgroups in chronic lymphocytic leukemia. Biomed Res Int. (2014) 2014:686392. doi: 10.1155/2014/686392
16. Oltra AM, Carbonell F, Tormos C, Iradi A, Sáez GT. Antioxidant enzyme activities and the production of MDA and 8-oxo-dG in chronic lymphocytic leukemia. Free Radic Biol Med. (2001) 30:1286–92. doi: 10.1016/S0891-5849(01)00521-4
17. Sies H, Jones DP. Reactive oxygen species. (ROS) as pleiotropic physiological signalling agents. Nat Rev Mol Cell Biol. (2020) 21:363–83. doi: 10.1038/s41580-020-0230-3
18. Nathan C, Cunningham-Bussel A. Beyond oxidative stress: an immunologist's guide to reactive oxygen species. Nat Rev Immunol. (2013) 13:349–61. doi: 10.1038/nri3423
19. Holmström KM, Finkel T. Cellular mechanisms and physiological consequences of redox-dependent signalling. Nat Rev Mol Cell Biol. (2014) 15:411–21. doi: 10.1038/nrm3801
20. Kamiguti AS, Serrander L, Lin K, Harris RJ, Cawley JC, Allsup DJ, et al. Expression and activity of NOX5 in the circulating malignant B cells of hairy cell leukemia. J Immunol. (2005) 175:8424–30. doi: 10.4049/jimmunol.175.12.8424
21. Heintel D, Kienle D, Shehata M, Kröber A, Kroemer E, Schwarzinger I, et al. High expression of lipoprotein lipase in poor risk B-cell chronic lymphocytic leukemia. Leukemia. (2005) 19:1216–23. doi: 10.1038/sj.leu.2403748
22. Spaner DE, Lee E, Shi Y, Wen F, Li Y, Tung S, et al. PPAR-alpha is a therapeutic target for chronic lymphocytic leukemia. Leukemia. (2013) 27:1090–9. doi: 10.1038/leu.2012.329
23. Caro P, Kishan AU, Norberg E, Stanley IA, Chapuy B, Ficarro SB, et al. Metabolic signatures uncover distinct targets in molecular subsets of diffuse large B cell lymphoma. Cancer Cell. (2012) 22:547–60. doi: 10.1016/j.ccr.2012.08.014
24. D'Arena G, Seneca E, Migliaccio I, De Feo V, Giudice A, La Rocca F, et al. Oxidative stress in chronic lymphocytic leukemia: still a matter of debate. Leuk Lymphoma. (2019) 60:867–75. doi: 10.1080/10428194.2018.1509317
25. Tome ME, Johnson DF, Rimsza LM, Roberts RA, Grogan TM, Miller TP, et al. A redox signature score identifies diffuse large B-cell lymphoma patients with a poor prognosis. Blood. (2005) 106:3594–601. doi: 10.1182/blood-2005-02-0487
26. Cavallini C, Chignola R, Dando I, Perbellini O, Mimiola E, Lovato O, et al. Low catalase expression confers redox hypersensitivity and identifies an indolent clinical behavior in CLL. Blood. (2018) 131:1942–54. doi: 10.1182/blood-2017-08-800466
27. Weinberg F, Ramnath N, Nagrath D. Reactive oxygen species in the tumor microenvironment: an overview. Cancers. (2019) 11:1191. doi: 10.3390/cancers11081191
28. El-Kenawi A, Ruffell B. Inflammation, ROS, and Mutagenesis. Cancer Cell. (2017) 32:727–9. doi: 10.1016/j.ccell.2017.11.015
29. Maffei R, Bulgarelli J, Fiorcari S, Bertoncelli L, Martinelli S, Guarnotta C, et al. The monocytic population in chronic lymphocytic leukemia shows altered composition and deregulation of genes involved in phagocytosis and inflammation. Haematologica. (2013) 98:1115–23. doi: 10.3324/haematol.2012.073080
30. Jitschin R, Braun M, Büttner M, Dettmer-Wilde K, Bricks J, Berger J, et al. CLL-cells induce IDOhi CD14+HLA-DRlo myeloid-derived suppressor cells that inhibit T-cell responses and promote TRegs. Blood. (2014) 124:750–60. doi: 10.1182/blood-2013-12-546416
31. Hanna BS, McClanahan F, Yazdanparast H, Zaborsky N, Kalter V, Rößner PM, et al. Depletion of CLL-associated patrolling monocytes and macrophages controls disease development and repairs immune dysfunction in vivo. Leukemia. (2016) 30:570–9. doi: 10.1038/leu.2015.305
32. Manukyan G, Papajik T, Gajdos P, Mikulkova Z, Urbanova R, Gabcova G, et al. Neutrophils in chronic lymphocytic leukemia are permanently activated and have functional defects. Oncotarget. (2017) 8:84889–901. doi: 10.18632/oncotarget.20031
33. Hileman EO, Liu J, Albitar M, Keating MJ, Huang P. Intrinsic oxidative stress in cancer cells: a biochemical basis for therapeutic selectivity. Cancer Chemother Pharmacol. (2004) 53:209–19. doi: 10.1007/s00280-003-0726-5
34. Packham G, Krysov S, Allen A, Savelyeva N, Steele AJ, Forconi F, et al. The outcome of B-cell receptor signaling in chronic lymphocytic leukemia: proliferation or anergy. Haematologica. (2014) 99:1138–48. doi: 10.3324/haematol.2013.098384
35. Miller CG, Holmgren A, Arnér ESJ, Schmidt EE. NADPH-dependent and -independent disulfide reductase systems. Free Radic Biol Med. (2018) 127:248–61. doi: 10.1016/j.freeradbiomed.2018.03.051
36. Espinosa-Diez C, Miguel V, Mennerich D, Kietzmann T, Sánchez-Pérez P, Cadenas S, et al. Antioxidant responses and cellular adjustments to oxidative stress. Redox Biol. (2015) 6:183–97. doi: 10.1016/j.redox.2015.07.008
37. Wu RP, Hayashi T, Cottam HB, Jin G, Yao S, Wu CCN, et al. Nrf2 responses and the therapeutic selectivity of electrophilic compounds in chronic lymphocytic leukemia. Proc Natl Acad Sci USA. (2010) 107:7479–84. doi: 10.1073/pnas.1002890107
38. Weniger MA, Rizzatti EG, Pérez-Galán P, Liu D, Wang Q, Munson PJ, et al. Treatment-induced oxidative stress and cellular antioxidant capacity determine response to bortezomib in mantle cell lymphoma. Clin Cancer Res. (2011) 17:5101–12. doi: 10.1158/1078-0432.CCR-10-3367
39. Trzeciecka A, Klossowski S, Bajor M, Zagozdzon R, Gaj P, Muchowicz A, et al. Dimeric peroxiredoxins are druggable targets in human Burkitt lymphoma. Oncotarget. (2016) 7:1717–31. doi: 10.18632/oncotarget.6435
40. Yosifov DY, Idler I, Bhattacharya N, Reichenzeller M, Close V, Ezerina D, et al. Oxidative stress as candidate therapeutic target to overcome microenvironmental protection of CLL. Leukemia. (2020) 34:115–27. doi: 10.1038/s41375-019-0513-x
41. Sewastianik T, Szydlowski M, Jablonska E, Bialopiotrowicz E, Kiliszek P, Gorniak P, et al. FOXO1 is a TXN- and p300-dependent sensor and effector of oxidative stress in diffuse large B-cell lymphomas characterized by increased oxidative metabolism. Oncogene. (2016) 35:5989–6000. doi: 10.1038/onc.2016.126
42. Nishigaki H, Ito C, Manabe A, Kumagai M, Coustan-Smith E, Yanishevski Y, et al. Prevalence and growth characteristics of malignant stem cells in B-lineage acute lymphoblastic leukemia. Blood. (1997) 89:3735–44. doi: 10.1182/blood.V89.10.3735.3735_3735_3744
43. Sheng X, Tucci J, Parmentier J-H, Ji L, Behan JW, Heisterkamp N, et al. Adipocytes cause leukemia cell resistance to daunorubicin via oxidative stress response. Oncotarget. (2016) 7:73147–59. doi: 10.18632/oncotarget.12246
44. Burt R, Dey A, Aref S, Aguiar M, Akarca A, Bailey K, et al. Activated stromal cells transfer mitochondria to rescue acute lymphoblastic leukemia cells from oxidative stress. Blood. (2019) 134:1415–29. doi: 10.1182/blood.2019001398
45. Méndez-Ferrer S, Bonnet D, Steensma DP, Hasserjian RP, Ghobrial IM, Gribben JG, et al. Bone marrow niches in haematological malignancies. Nat Rev Cancer. (2020) 20:285–98. doi: 10.1038/s41568-020-0245-2
46. Cramer SL, Saha A, Liu J, Tadi S, Tiziani S, Yan W, et al. Systemic depletion of L-cyst(e)ine with cyst(e)inase increases reactive oxygen species and suppresses tumor growth. Nat Med. (2017) 23:120–7. doi: 10.1038/nm.4232
47. Boutter J, Huang Y, Marovca B, Vonderheit A, Grotzer MA, Eckert C, et al. Image-based RNA interference screening reveals an individual dependence of acute lymphoblastic leukemia on stromal cysteine support. Oncotarget. (2014) 5:11501–12. doi: 10.18632/oncotarget.2572
48. Sugimoto K, Hayakawa F, Shimada S, Morishita T, Shimada K, Katakai T, et al. Discovery of a drug targeting microenvironmental support for lymphoma cells by screening using patient-derived xenograft cells. Sci Rep. (2015) 5:13054. doi: 10.1038/srep13054
49. Polak R, de Rooij B, Pieters R, den Boer ML. B-cell precursor acute lymphoblastic leukemia cells use tunneling nanotubes to orchestrate their microenvironment. Blood. (2015) 126:2404–14. doi: 10.1182/blood-2015-03-634238
50. de Rooij B, Polak R, Stalpers F, Pieters R, den Boer ML. Tunneling nanotubes facilitate autophagosome transfer in the leukemic niche. Leukemia. (2017) 31:1651–4. doi: 10.1038/leu.2017.117
51. Johnson SM, Dempsey C, Chadwick A, Harrison S, Liu J, Di Y, et al. Metabolic reprogramming of bone marrow stromal cells by leukemic extracellular vesicles in acute lymphoblastic leukemia. Blood. (2016) 128:453–6. doi: 10.1182/blood-2015-12-688051
52. Paggetti J, Haderk F, Seiffert M, Janji B, Distler U, Ammerlaan W, et al. Exosomes released by chronic lymphocytic leukemia cells induce the transition of stromal cells into cancer-associated fibroblasts. Blood. (2015) 126:1106–17. doi: 10.1182/blood-2014-12-618025
53. Fiskus W, Saba N, Shen M, Ghias M, Liu J, Gupta SD, et al. Auranofin induces lethal oxidative and endoplasmic reticulum stress and exerts potent preclinical activity against chronic lymphocytic leukemia. Cancer Res. (2014) 74:2520–32. doi: 10.1158/0008-5472.CAN-13-2033
54. Barr PM, Miller TP, Friedberg JW, Peterson DR, Baran AM, Herr M, et al. Phase 2 study of imexon, a prooxidant molecule, in relapsed and refractory B-cell non-Hodgkin lymphoma. Blood. (2014) 124:1259–65. doi: 10.1182/blood-2014-04-570044
55. Gout P, Buckley A, Simms C, Bruchovsky N. Sulfasalazine, a potent suppressor of lymphoma growth by inhibition of the xcɤcystine transporter: a new action for an old drug. Leukemia. (2001) 15:1633–40. doi: 10.1038/sj.leu.2402238
56. Galluzzi L, Buqué A, Kepp O, Zitvogel L, Kroemer G. Immunogenic cell death in cancer and infectious disease. Nat Rev Immunol. (2017) 17:97–111. doi: 10.1038/nri.2016.107
57. Garg AD, Krysko DV, Verfaillie T, Kaczmarek A, Ferreira GB, Marysael T, et al. A novel pathway combining calreticulin exposure and ATP secretion in immunogenic cancer cell death. EMBO J. (2012) 31:1062–79. doi: 10.1038/emboj.2011.497
58. Krysko DV, Garg AD, Kaczmarek A, Krysko O, Agostinis P, Vandenabeele P. Immunogenic cell death and DAMPs in cancer therapy. Nat Rev Cancer. (2012) 12:860–75. doi: 10.1038/nrc3380
59. Li W, Yang J, Luo L, Jiang M, Qin B, Yin H, et al. Targeting photodynamic and photothermal therapy to the endoplasmic reticulum enhances immunogenic cancer cell death. Nat Commun. (2019) 10:3349. doi: 10.1038/s41467-019-11269-8
60. Dutt S, Atallah MB, Minamida Y, Filatenkov A, Jensen KP, Iliopoulou BP, et al. Accelerated, but not conventional, radiotherapy of murine B-cell lymphoma induces potent T cell-mediated remissions. Blood Adv. (2018) 2:2568–80. doi: 10.1182/bloodadvances.2018023119
61. Zappasodi R, Pupa SM, Ghedini GC, Bongarzone I, Magni M, Cabras AD, et al. Improved clinical outcome in indolent B-cell lymphoma patients vaccinated with autologous tumor cells experiencing immunogenic death. Cancer Res. (2010) 70:9062–72. doi: 10.1158/0008-5472.CAN-10-1825
62. Spaner DE, Hammond C, Mena J, Foden C, Deabreu A. A phase I/II trial of oxidized autologous tumor vaccines during the “watch and wait” phase of chronic lymphocytic leukemia. Cancer Immunol Immunother. (2005) 54:635–46. doi: 10.1007/s00262-004-0626-5
63. Griess B, Mir S, Datta K, Teoh-Fitzgerald M. Scavenging reactive oxygen species selectively inhibits M2 macrophage polarization and their pro-tumorigenic function in part, via Stat3 suppression. Free Radic Biol Med. (2020) 147:48–60. doi: 10.1016/j.freeradbiomed.2019.12.018
64. Tan H-Y, Wang N, Li S, Hong M, Wang X, Feng Y. The reactive oxygen species in macrophage polarization: reflecting its dual role in progression and treatment of human diseases. Oxid Med Cell Longev. (2016) 2016:e2795090. doi: 10.1155/2016/2795090
65. Zhang Y, Choksi S, Chen K, Pobezinskaya Y, Linnoila I, Liu Z-G. ROS play a critical role in the differentiation of alternatively activated macrophages and the occurrence of tumor-associated macrophages. Cell Res. (2013) 23:898–914. doi: 10.1038/cr.2013.75
66. Roux C, Jafari SM, Shinde R, Duncan G, Cescon DW, Silvester J, et al. Reactive oxygen species modulate macrophage immunosuppressive phenotype through the up-regulation of PD-L1. PNAS. (2019) 116:4326–35. doi: 10.1073/pnas.1819473116
67. Ohl K, Tenbrock K. Reactive oxygen species as regulators of MDSC-mediated immune suppression. Front Immunol. (2018) 9:2499. doi: 10.3389/fimmu.2018.02499
68. Corzo CA, Cotter MJ, Cheng P, Cheng F, Kusmartsev S, Sotomayor E, et al. Mechanism regulating reactive oxygen species in tumor-induced myeloid-derived suppressor cells. J Immunol. (2009) 182:5693–701. doi: 10.4049/jimmunol.0900092
69. Nefedova Y, Fishman M, Sherman S, Wang X, Beg AA, Gabrilovich DI. Mechanism of all-trans retinoic acid effect on tumor-associated myeloid-derived suppressor cells. Cancer Res. (2007) 67:11021–8. doi: 10.1158/0008-5472.CAN-07-2593
70. Cubillos-Ruiz JR, Silberman PC, Rutkowski MR, Chopra S, Perales-Puchalt A, Song M, et al. ER stress sensor XBP1 controls anti-tumor immunity by disrupting dendritic cell homeostasis. Cell. (2015) 161:1527–38. doi: 10.1016/j.cell.2015.05.025
71. Siernicka M, Winiarska M, Bajor M, Firczuk M, Muchowicz A, Bobrowicz M, et al. Adenanthin, a new inhibitor of thiol-dependent antioxidant enzymes, impairs the effector functions of human natural killer cells. Immunology. (2015) 146:173–83. doi: 10.1111/imm.12494
72. Werlenius O, Aurelius J, Hallner A, Akhiani AA, Simpanen M, Martner A, et al. Reactive oxygen species induced by therapeutic CD20 antibodies inhibit natural killer cell-mediated antibody-dependent cellular cytotoxicity against primary CLL cells. Oncotarget. (2016) 7:32046–53. doi: 10.18632/oncotarget.8769
73. Hansson M, Asea A, Ersson U, Hermodsson S, Hellstrand K. Induction of apoptosis in NK cells by monocyte-derived reactive oxygen metabolites. J Immunol. (1996) 156:42–7.
74. Harlin H, Hanson M, Johansson CC, Sakurai D, Poschke I, Norell H, et al. The CD16- CD56(bright) NK cell subset is resistant to reactive oxygen species produced by activated granulocytes and has higher antioxidative capacity than the CD16+ CD56(dim) subset. J Immunol. (2007) 179:4513–9. doi: 10.4049/jimmunol.179.7.4513
75. Hurst KE, Lawrence KA, Essman MT, Walton ZJ, Leddy LR, Thaxton JE. Endoplasmic reticulum stress contributes to mitochondrial exhaustion of CD8+ T Cells. Cancer Immunol Res. (2019) 7:476–86. doi: 10.1158/2326-6066.CIR-18-0182
76. Cemerski S, van Meerwijk JPM, Romagnoli P. Oxidative-stress-induced T lymphocyte hyporesponsiveness is caused by structural modification rather than proteasomal degradation of crucial TCR signaling molecules. Eur J Immunol. (2003) 33:2178–85. doi: 10.1002/eji.200323898
77. Thorén FB, Romero AI, Hellstrand K. Oxygen radicals induce poly(ADP-ribose) polymerase-dependent cell death in cytotoxic lymphocytes. J Immunol. (2006) 176:7301–7. doi: 10.4049/jimmunol.176.12.7301
78. Chen X, Song M, Zhang B, Zhang Y. Reactive oxygen species regulate T cell immune response in the tumor microenvironment. Oxid Med Cell Longev. (2016) 2016:1580967. doi: 10.1155/2016/1580967
79. Maj T, Wang W, Crespo J, Zhang H, Wang W, Wei S, et al. Oxidative stress controls regulatory T cell apoptosis and suppressor activity and PD-L1-blockade resistance in tumor. Nat Immunol. (2017) 18:1332–41. doi: 10.1038/ni.3868
80. Mougiakakos D, Johansson CC, Jitschin R, Böttcher M, Kiessling R. Increased thioredoxin-1 production in human naturally occurring regulatory T cells confers enhanced tolerance to oxidative stress. Blood. (2011) 117:857–61. doi: 10.1182/blood-2010-09-307041
81. Stabile H, Fionda C, Gismondi A, Santoni A. Role of distinct natural killer cell subsets in anticancer response. Front Immunol. (2017) 8:293. doi: 10.3389/fimmu.2017.00293
82. Sabry SA, El-Senduny FF, Abousamra NK, El-Din MS, Youssef MM. Oxidative stress in CLL patients leads to activation of Th9 cells: an experimental and comprehensive survey. Immunol Med. (2020) 43:36–46. doi: 10.1080/25785826.2019.1700747
83. Liu S-X, Xiao H-R, Wang G-B, Chen X-W, Li C-G, Mai H-R, et al. Preliminary investigation on the abnormal mechanism of CD4+FOXP3+CD25high regulatory T cells in pediatric B-cell acute lymphoblastic leukemia. Exp Ther Med. (2018) 16:1433–41. doi: 10.3892/etm.2018.6326
84. Chang C, Wu S-Y, Kang Y-W, Lin K-P, Chen T-Y, Medeiros LJ, et al. High levels of regulatory T cells in blood are a poor prognostic factor in patients with diffuse large B-cell lymphoma. Am J Clin Pathol. (2015) 144:935–44. doi: 10.1309/AJCPUJGMVV6ZF4GG
Keywords: oxidative stress, ROS, B-cell malignancies, immune evasion, CLL, B-ALL, lymphoma, leukemia
Citation: Domka K, Goral A and Firczuk M (2020) cROSsing the Line: Between Beneficial and Harmful Effects of Reactive Oxygen Species in B-Cell Malignancies. Front. Immunol. 11:1538. doi: 10.3389/fimmu.2020.01538
Received: 02 May 2020; Accepted: 11 June 2020;
Published: 21 July 2020.
Edited by:
Jérôme Paggetti, Luxembourg Institute of Health, LuxembourgReviewed by:
Martin Böttcher, University Hospital Erlangen, GermanyRolf Kiessling, Karolinska Institutet (KI), Sweden
Copyright © 2020 Domka, Goral and Firczuk. This is an open-access article distributed under the terms of the Creative Commons Attribution License (CC BY). The use, distribution or reproduction in other forums is permitted, provided the original author(s) and the copyright owner(s) are credited and that the original publication in this journal is cited, in accordance with accepted academic practice. No use, distribution or reproduction is permitted which does not comply with these terms.
*Correspondence: Malgorzata Firczuk, bWZpcmN6dWtAd3VtLmVkdS5wbA==