- 1Department of Microbiology and Immunology, Georgetown University School of Medicine, Washington, DC, United States
- 2CMRS/Laboratory of Immunoregulation, National Institutes of Allergy and Infectious Diseases, Bethesda, MD, United States
Immune activation is the hallmark of HIV infection and plays a role in the pathogenesis of the disease. In the context of suppressed HIV RNA replication by combination antiretroviral therapy (cART), there remains immune activation which is associated to the HIV reservoirs. Persistent virus contributes to a sustained inflammatory environment promoting accumulation of “activated/exhausted” T cells with diminished effector function. These T cells show increased expression of immunomodulatory receptors including Programmed cell death protein (PD1), Cytotoxic T Lymphocyte Associated Protein 4 (CTLA4), Lymphocyte activation gene 3 (LAG3), T cell immunoglobulin and ITIM domain (TIGIT), T cell immunoglobulin and mucin domain containing 3 (TIM3) among others. More importantly, recent reports had demonstrated that, HIV infected T cells express checkpoint receptors, contributing to their survival and promoting maintenance of the viral reservoir. Therapeutic strategies are focused on viral reservoir elimination and/or those to achieve sustained cART-free virologic remission. In this review, we will discuss the immunological basis and the latest advances of the use of checkpoint inhibitors to treat HIV infection.
Introduction
The immune system is designed to regulate the balance between immunity and tissue damage. This process is mediated by receptor-ligand interactions that provide positive or negative co-stimulatory signals. Particularly, the inhibitory receptors function as “brakes” for the adaptive immune response and serve as checkpoints to control tissue immunopathology. The signaling through these inhibitory receptors regulates the immune response and, in case of failure of pathogen elimination these pathways have profound impact on the host homeostasis contributing to the disease. Because of their impact during immune responses, checkpoint receptors became major therapeutic targets in a wide spectrum of diseases including cancer and chronic viral infections such as HIV (1–3).
Different to other human chronic viral infections, HIV targets the immune system altering immune mechanisms, and is characterized by chronic immune activation, the main player in the pathogenesis of the disease (4–6). In the context of suppressed HIV replication by combination antiretroviral therapy (cART), immune activation is associated with poor clinical outcomes such as the non-AIDS defining illnesses which are today the leading cause of mortality and morbidity in patients with HIV infection.
Persistent infection contributes to a sustained inflammatory environment promoting accumulation of activated T cells. HIV-specific T cells and other virus specific T cells such as EBV, CMV, and influenza show an immune activated phenotype (4, 6–8). The main feature of these activated T cells is an “exhausted phenotype” suggested by increased expression of one or co-expression of several immunomodulatory receptors including PD1, CTLA4, LAG3, TIGIT, TIM3, CD160 among others. Exhausted HIV-specific T cells have diminished effector function and fail to control viral replication (9–25).
The essential role that HIV/SIV-specific cytotoxic T lymphocytes (CTL) play to control viral replication was demonstrated by in vivo depletion of CD8 T cells that resulted in lack of viral control during acute and chronic Simian Immunodeficiency Virus (SIV) infection (26–30). In addition, in human infection, viral escape mechanisms emerge early during infection and are contributing factors for the failure of CD8 T cell mediated immunity (8, 31, 32).
HIV-specific CD4 T cells are important in the immunity against HIV, however their role is hampered by being the major targets of HIV/SIV infection (13, 33–38). In addition, CD4 T cells are the main cell type harboring the HIV/SIV reservoirs in tissues and recent evidence determined that HIV latently infected CD4 T cells express checkpoint receptors promoting viral persistence (22, 23, 39).
This evidence suggests that immune therapeutic approaches directed to block immune checkpoint receptors will have two-level effect on the viral reservoir and HIV-specific T cell responses. In this review, we will discuss the latest advances in this area.
The Role of Checkpoint Receptors in HIV Infection
The checkpoint receptors PD1 and CTLA4 are the most extensively studied in vitro and in vivo in the context of HIV/ SIV infection. The checkpoint receptors such as LAG3, TIGIT, TIM3, and others are also expressed by T cells and their role in the pathogenesis of the infection is not well-defined. More importantly, the observation that several checkpoint receptors are co-expressed by latently infected CD4 T cells, suggest new roles of these molecules in viral persistence and their potential to be used as reversal agents have emerged in the last few years (Figure 1).
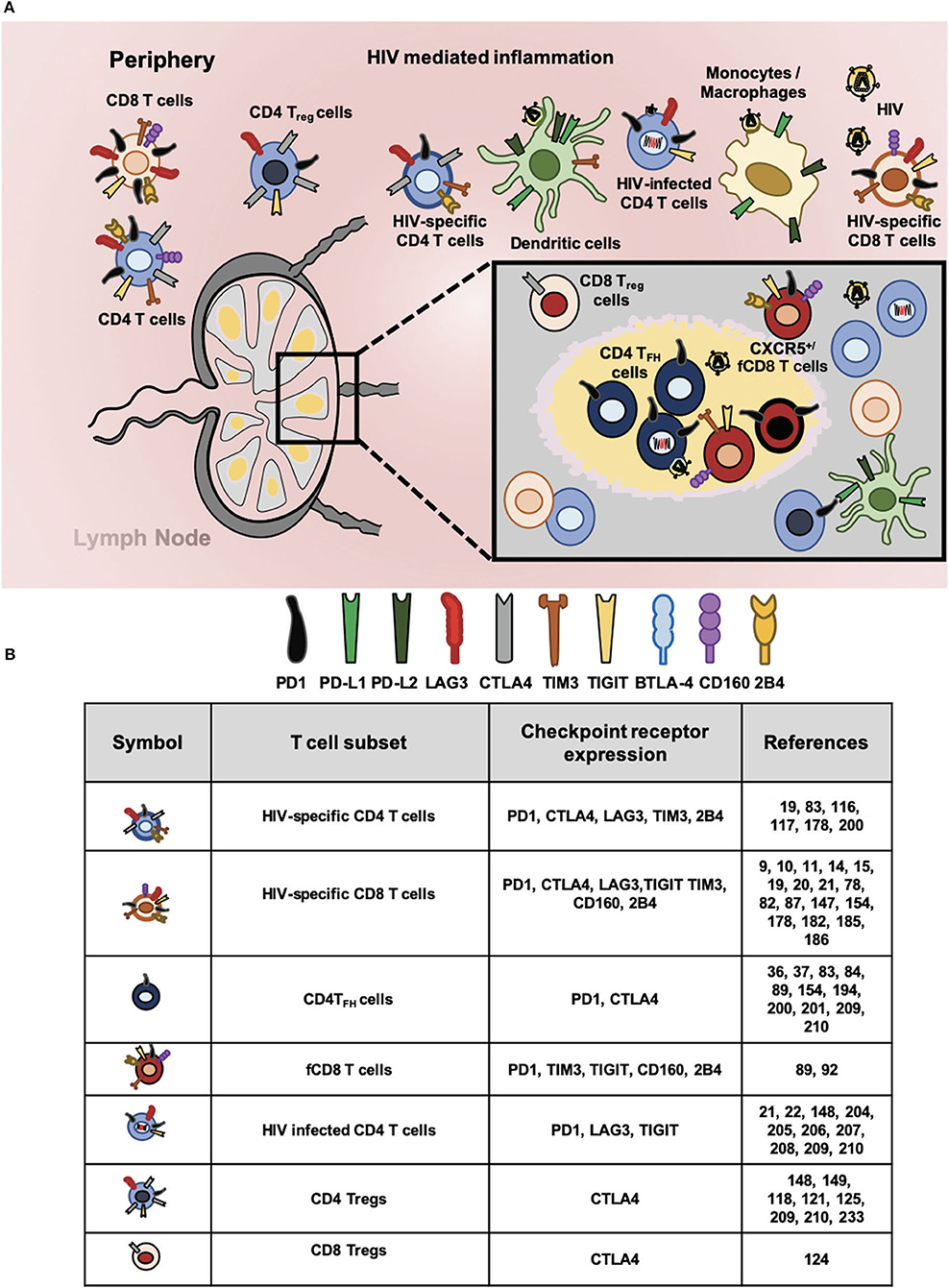
Figure 1. Checkpoint receptors expression in HIV-specific T cells and latently infected CD4 T cells. (A) Chronic immune activation and inflammation are the hallmark of HIV infection. In this context, cells of innate and adaptive immune system became dysfunctional and express aberrant levels of checkpoint receptors that hampers HIV-specific responses. Proportionally to antigen abundance and persistence, several checkpoints receptors became upregulated particularly in different T cell subsets. In circulation and lymphoid tissues, total CD4 and CD8 T cells; regulatory CD4 T (Treg) and CD8 (Treg) T cells; follicular helper CD4 T (TFH), and follicular CD8 T (fCD8 T) cells; HIV-specific CD4 and CD8 T cells. In addition, HIV infected CD4 T cells express surface checkpoint receptors such as Programmed cell death protein 1 (PD1), Cytotoxic T lymphocyte antigen 4 (CTLA4), Lymphocyte activation gene 3 protein (LAG3), T cell immunoglobulin and mucin domain receptor 3 (TIM3), T cell immunoreceptor with immunoglobulin and ITIM domains (TIGIT), B and T lymphocyte attenuator (BTLA), CD160, and 2B4. Antigen presenting cells (APC, mainly monocytes/macrophages and dendritic cells) upregulate checkpoints receptors that bind to the ligands expressed by lymphocytes. Accordingly, Programmed cell death protein ligand 1 (PD-L1) and ligand 2 (PD-L2) along with other inhibitory receptors are upregulated by APCs regulating T cell mediated immunity against HIV. (B) Expression of checkpoint receptors by T cell subsets. The wide spectrum of T cell subsets that express checkpoint receptors suggest their blockade will promote latency reversal and elimination by invigorated HIV-specific T cells.
PD1 (CD279)
PD1 was discovered by Ishida et al. in 1992 and its function in regulating the immune response was elucidated few years later when the Pdcd1 deficient mice was developed and showed a lupus-like autoimmune disease (40–42). PD1 binds to two ligands, PD-L1 (B7-H1) and PD-L2 (B7-DC). PD-L1 is expressed by a variety of hematopoietic cells including, T and B cells, DCs, macrophages, and non-hematopoietic cells including mesenchymal stem cells, lung epithelial cells, vascular endothelium, liver non-parenchymal cells, placental synctiotrophoblasts, and keratinocytes (1, 43, 44). In contrast, PD-L2 expression is more restricted to antigen presenting cells such as dendritic cells, macrophages, and germinal center B cells and its expression is modulated by inflammatory signals (45–47). The most characterized function of the PD1/PD-L1 pathway is tuning T cell responses, however the wide range of cells that express PD-L1 suggests other unexplored functions in regulating immune responses. The effects of PD-L2 interaction with PD1 is not well-defined. PD-L2 binds with higher affinity to PD1 indicating that may compete with PD-L1 (48). In addition, PD-L2 expression is inducible by Th2 cytokines and may play a role in regulating Th2 responses (48, 49). However, PD-L2 has shown inhibitory effects on proliferation and cytokine secretion including IFNγ suggesting a role in modulating also Th1 responses (50–52).
PD1 signaling occurs through its intracytoplasmic domain that contains an immunoreceptor tyrosine-based inhibitory motif (ITIM) and an immunoreceptor tyrosine-based switch motif (ITSM) (53, 54). PD1 exerts its inhibitory function when signals in combination with TCR engagement. The tyrosine residue located in the ITSM is phosphorylated recruiting protein tyrosine phosphatases. The tyrosine phosphatase SHP2 interferes with molecules involved in TCR signaling (ZAP70, PKCθ, and CD3ζ) and downstream pathways of the TCR-CD28 signaling including phosphoinositide 3-kinase (PI3K)–AKT, and RAS. In addition, it was shown that in absence of SHP-2, SHP-1 phosphatase can be recruited and promote similar effects on T cells (55, 56). The interference of these pathways leads to inhibition of T cell activation, effector function, proliferation, and survival (45, 53, 57–64).
PD1 is expressed by many subsets of T cells including memory CD4 and CD8 T cells, T regulatory cells (Tregs), T follicular helper (TFH), and T follicular regulatory (TFR) cells (65–67). In addition, expression of PD1 has been also shown in B cells, NK cells and myeloid cells suggesting that blockade of this receptor has wide impact on the immune response (47). In addition to attenuating T cell activation in many subsets of T cells, new roles for PD1 have been reported. Particularly, it has been shown that its expression by CD4 TFH cells is required for their positioning inside the B cell zone and for the secretion of IL-21. In contrast, PD1 signaling prevents activated T cells from entering into the follicles (68).
PD1 has been mainly studied in CD8 T cells in the context of chronic infection and cancer. In an acute infection, PD1 expression is modulated by TCR signaling and its expression is reduced after elimination of the pathogen (47, 69). In contrast, in chronic infections and cancer, continuous antigen stimulation leads to sustained expression of PD1 and diminished effector function (70–74).
The effector CD8 T cells that develop in these conditions undergo a distinct “exhausted” transcriptional and epigenetic program than CD8 effector T cells developed during an acute infection. Recent studies had shown that “exhausted” CD8 T cells are in fact a heterogenous population, and some of these CD8 T cells possess stem-like properties defined by expression of the transcription factor T-cell factor 1 (TCF1) (75, 76). These CD8 T cells are responsible to maintain the immune response during chronic infection, and in contrast to exhausted cells they have self-renewal properties and expand during PD1 therapy (75). The features of “exhausted” T cells, in terms of their transcriptional, epigenetic, metabolic, and others properties, are discussed in depth by 18 experts in the field in a recent point-of-view article that highlights their heterogeneity and pathways driving their differentiation (77).
PD1 Expression in HIV Infection
In human chronic infections such as HIV infection, PD1/PD-L1 pathway is one of the checkpoint receptors that has been extensively studied in an attempt to restore T cell mediated immunity against HIV. PD1 expression in CD8 T cells is associated with the levels of viremia and disease progression, and its expression is only partially reduced after successfully suppressed viremia by cART treatment (18, 78–81). Exhausted HIV-specific CD8 T cells have diminished effector function, proliferative capacity, and increased susceptibility to undergo apoptosis. This evidence suggests that this pathway plays a critical role in the failure of CD8 T cells to mediate viral clearance (9, 10, 78).
The antigen dependency in the expression of PD1 by CD8 T cells during chronic HIV infection was further demonstrated in a study of a longitudinal cohort of HIV infected patients. HIV-specific CD8 T cells for those epitopes that underwent mutational escape during infection showed decreased expression of PD1. In contrast, HIV-specific CD8 T cells with TCR specificities for conserved epitopes expressed higher levels of PD1 and showed poor effector function (82). These data confirmed that chronic TCR stimulation plays a major role in the sustained expression of PD1 and the development of exhausted CD8 T cells in HIV infection.
Other important aspect of checkpoint receptors in the context of HIV infection is that they are expressed by several subsets of infected CD4 T cells. For instance, CD4 TFH cells are the major HIV cell reservoir and are localized in the B cell follicles. This micro-compartmentalization of the viral reservoir inside the lymphoid organs has drawn the focus toward CD8 T cell immunity against HIV mediated by CD8 follicular (fCD8) T cells. fCD8 T cells are defined by expression of CXCR5, and they have ability to migrate into the B cell follicle where the ligand of CXCR5 is expressed. In addition, another important subset is the tissue resident memory CD8 T (CD8 TRM) cells which are also present in lymphoid organs (83–86).
In SIV/HIV infection, most of the virus-specific CD8 T cells are localized in extrafollicular area around the B cell follicles (87, 88). In contrast, CXCR5+ fCD8 T cells are enriched in the follicles and their frequency is associated with local immune activation rather than viral replication (89). fCD8 T cells expressed high levels of PD1 and some co-expressed TIGIT (89). fCD8 T cells, showed diminished polyfunctionality measured by cytokine secretion that was restored by blockade of the PD1/PD-L1 pathway. Studies in SIV infection, showed that fCD8 T cells localized inside and around the follicles and have cytotoxic potential (90, 91).
The role of PD1 in fCD8 T cells is not well-understood. The recent evidence that PD1 signaling is involved in preventing activated T cells to traffic into the B cell follicles suggests that PD1 blockade can be a strategy to promote trafficking of HIV-specific T cells to the sites of viral reservoirs. In this regard, in vivo blockade of PD1 led to expansion of SIV-specific fCD8 T cells co-expressing cytolytic molecules in the lymph nodes, although the study did not evaluate the frequency of these cells inside the follicles (24).
The potential of fCD8 T cells to traffic into the B cell follicles where the HIV infected CD4 TFH cells are localized suggests that therapies enhancing differentiation, and effector function of fCD8 T cells can be an important intervention to be considered in cure strategies (92, 93).
Another important subset of CD8 T cells involved in tissue immune surveillance is the tissue resident memory T cells. CD8 TRM cells were first described in peripheral tissues and represent the first line of defense during memory immune responses. These T cells do not circulate and their transcriptional profile varies between tissue resident cells from different tissues suggesting tissue-specific properties of these cells (94). Recent reports have identified populations of CD4 TRM cells and CD8 TRM cells in lymph nodes, they express CD69, a tissue residency marker, and inhibitory receptors including PD1 indicating a physiological role of PD1 in balancing tissue damage during immune responses (95–97).
In the context of HIV infection, higher frequencies of HIV-specific CD8 TRM are present in the lymphoid nodes from HIV infected elite controllers compared with HIV infected progressors highlighting a central role for these cells in limiting viral replication (85, 86, 98, 99). In addition, CD8 TRM cells from the lymph nodes expressed higher levels of CD69 and their transcriptional profile was consistent with tissue residency rather than activation. While most of the CD69+ CD8 TRM cells are localized in the extrafollicular areas, a smaller fraction of these cells are found inside the B cell follicles suggesting that they can migrate into the sites of HIV viral reservoir (85, 86). This evidence has led to the hypothesis that enhancing trafficking into the follicle of CD8 T cells and/or promoting differentiation into fCD8 T cell subset can be an efficacious strategy for viral control/elimination.
CTLA4 (CD152)
CTLA4 belongs to the CD28 family of proteins and its expression is upregulated on T cells upon TCR stimulation. In contrast to conventional T cells, Tregs constitutively express CTLA4 suggesting a prominent role of this checkpoint receptor in Treg function.
CTLA4 competes with CD28 for the binding to CD80 and CD86 expressed on the surface of antigen presenting cells attenuating T cell activation (100, 101). In Tregs, CTLA4 is stored in a vesicular intracellular compartment and upon TCR activation is released at the immunological synapse (102). The regulated secretion of CTLA4 requires calcium influx and is modulated by adaptor molecules including T cell receptor-interacting molecule (TRIM), Linker for activation of X cells (LAX) and others (103–106). CTLA4 YVKM motif interacts with tyrosine phosphatase SHP-2 and serine-threonine phosphatase protein phosphatase 2A inhibiting TCR signaling (60, 105, 107, 108). The signaling pathway of CTLA4 is largely inconclusive, and has been suggested that CTLA4 cytoplasmic domain function is not to transmit downstream regulatory signals but rather control the turnover, cellular localization, and to regulate its secretion at the immunological synapse and control the binding of CD28 to CD80 and CD86 shared ligands (109–111).
CTLA4 Expression in HIV Infection
The role of CTLA4 in the context of HIV infection has been mostly described in CD4 T cells, and its expression is increased in both total and HIV-specific CD4 T cells. The role of CTLA4 in the pathogenesis of infection is suggested by the positive association with viral loads, and depletion of CD4 T cells (13, 112–116). In addition, CD4 T cells from HIV infected progressors were more likely to co-express multiple checkpoint receptors (PD1, CTLA4, and TIGIT), and at higher levels to that observed in elite controllers (117, 118). In contrast to CD4 T cells, HIV-specific CD8 T cells show lower expression of CTLA4 suggesting a more prominent role of this checkpoint receptor in CD4 T cells (13, 112–115, 117).
In Tregs, CTLA4 plays a major role in their regulatory function. In the context of SIV/HIV infection, Tregs have two-side function, while they can exert beneficial effects on reducing immune activation, they limit viral clearance (119–122). Accordantly, Tregs are present at the sites of viral replication such as lymph nodes, spleen, and gut associated lymphoid tissues, and their frequency is correlated with tissue SIV RNA levels (123). In addition, an accumulation of CD8 Tregs (CD25+FoxP3+CTLA4high) with suppressive effector function has been observed in lymphoid and colorectal mucosal tissues from viremic SIV infected rhesus macaques (RM) (124). Similar observations were made in humans in which Tregs expressed higher levels of CTLA4 compared with Tregs from individuals with suppressed viremia by cART (125). This evidence suggests that manipulating suppressor function by targeting CTLA4 and/or depletion of Tregs could be considered as a new approach for cure strategies (119).
LAG3 (CD223)
Lymphocyte activation gene 3 (LAG3, CD223) belongs to the immunoglobulin superfamily and it is expressed by conventional T lymphocytes, Tregs, NK cells and plasmacytoid DCs upon activation (126, 127). The extracellular region of LAG3 protein consists of four immunoglobulin-like domains (D1–D4) with high homology and higher binding affinity to MHC than the CD4 co-receptor (128). In T cells, LAG3 expression is upregulated upon TCR activation and exerts its immunomodulatory function mainly via MHC dependent TCR inhibition (128–131). LAG3 binds to CD3/TCR complex, suppressing calcium flux, cytokine secretion, and proliferation (132). In contrast, the blockade of the D2 domain (outside of MHC binding site) of LAG3 molecule improved T cell function suggesting possible involvement of other ligands for LAG3 particularly in case of CD8 T cells and NK cells (133). Several alternative ligands have been proposed for LAG3 including, Galectin-3, liver sinusoidal endothelial cell lectin (LSECtin) and fibrinogen-related protein (FGL-1) a soluble factor (134–136).
The intracytoplasmic region of LAG3 contains three domains: the first is a serine-phosphorylation site, the second region is a KIEELE motif, and the third a glutamic acid-proline (EP) repeats (137). In effector CD4 T cells, the KIEELE motif is essential for its inhibitory function, however, the downstream signaling molecules involved are not well-defined, and if this domain is participating in the signaling in other cells such as CD8 T cells is largely unknown (137). In addition, tissues metalloproteases can cleave LAG3 between the D4 transmembrane domain and the transmembrane domain, generating a soluble LAG3 and this mechanism can regulate T cell responses (138).
LAG3 Expression in HIV Infection
In the context of SIV/HIV infection, the expression of LAG3 has been reported to be elevated in T cells and iNKT cells in the tissues and blood, and its expression is associated with levels of viremia and disease progression (139, 140). In the context of suppressed viremia, T cell expression of LAG3 alone or in combination with PD1 has been associated with cardiovascular disease. While the mechanism of this association is largely unknown, it may reflect the state of chronic immune activation which is associated to increased risk of cardiovascular disease (19, 139–146). Similar expression of LAG3 has been described in HIV- and CMV-specific T cells from patients with HIV infection suggesting distinct roles of LAG3 in regulating HIV-specific immune responses (147, 148). In addition, expression of LAG3 has been also described in a subset of Tregs that co-express CD49b. These regulatory T cells are called Type I Tregs, and they secrete large amounts of IL-10. In patients with HIV infection, increase frequencies of Type I Tregs were associated with disease progression (149).
Co-expression of LAG3 and other inhibitory receptors including PD1 and TIGIT are enriched in latently infected CD4 T cells from HIV infected patients with suppressed viral loads suggesting that immune checkpoint receptors contribute to viral persistence. While the role of LAG3 in the pathogenesis of HIV is not well-understood, this evidence suggests that blockade of LAG3 can have an impact in cell mediated immunity and the viral reservoir (22, 148, 150).
TIGIT
TIGIT is a member of the immunoglobulin superfamily and belongs to the family of poliovirus receptors. TIGIT, CD226, and CD96 are expressed by NK cells and some T cells subsets including effector T cells, memory T cells, and TFH (127, 151–153). TIGIT inhibitory function is mediated by binding to its ligand the poliovirus receptor (PVR, CD155), and poliovirus receptor-relate 2/Nectin-2 (PVRL2, CD112) which are present on the surface of APCs and non-hematopoietic cells including tumor cells. TIGIT binds to the same ligands than CD226 (DNAM-1) and CD96 (Tactile), and together with CD226 promotes a positive co-stimulatory signal while CD96 and TIGIT transduce inhibitory signals. In addition, TIGIT inhibit the costimulatory signals of CD226 either by competition, binds with higher affinity to CD155; or by binding in cis to CD226, and blocks the costimulatory signals (127, 151, 154).
The intracellular domain of TIGIT contains an ITIM and an immunoglobulin tail tyrosine (ITT)-like motif. In mice, phosphorylation of the tyrosine residue in either motif is sufficient for transduce the inhibitory signal. In contrast in humans, phosphorylation of the tyrosine residue in ITIM or the ITT-like motif seems to be essential, and the contribution of either motif in the inhibitory signal remain undefined. TIGIT binding to CD155 promotes recruitment of SH2-containing tyrosine phosphatase SHP1 inhibiting phosphoinositide 3-kinase (PI3K) and mitogen-activated protein kinase (MAPK). Of note is that most of the signaling pathways have been described in NK cells, and in these cells, TIGIT inhibit both cytotoxicity and cytokine secretion (152, 155, 156). In T cells, TIGIT mediates its regulatory function by downregulation of the TCR complex and inhibition of PLCγ. While these effects of TIGIT leads to inhibition of T cell activation, proliferation, and effector function, also, promotes survival of these inhibited T cells by upregulation of γc cytokine receptors (IL-2, IL-7, and IL-15) and Bcl-xL (157).
TIGIT Expression in HIV Infection
In the context of HIV/SIV infection, a large portion of virus-specific CD8 T cells express TIGIT and have diminished effector function (21, 154). Increased frequencies of CD8 T cells expressing TIGIT alone or with PD1 are associated with disease progression. Moreover, in vitro blockade of TIGIT and the PD1/PD-L1 pathway restored HIV-specific CD8 T cell function suggesting that blockade of TIGIT in combination with other checkpoint receptors that may be a potential therapy to enhance T cell immunity against HIV (21).
Expression of TIGIT and PD1 by CD4 T cells have been associated with immune activation and increased risk of cardiovascular diseases in HIV infected patients as measured by 2-year change in coronary artery calcium. However, the mechanism in which TIGIT can contributes to cardiovascular disease is largely unknown (158). TIGIT is expressed in other T cell subsets from HIV infected patients. For instance, γδ T cells expressed TIGIT and its expression is associated with immune activation and pro-inflammatory function (159). In NK cells from HIV infected individuals, expression of TIGIT is correlated with disease progression and NK cell dysfunction (160). Although the role of TIGIT is not well-defined in HIV infection, TIGIT ligands such as PVR are increased by CD4 TFH cells in lymph nodes from HIV infected patients compared to heathy donors suggesting it may play a role in viral persistence (161).
TIM3 (CD366)
TIM3, also known as Hepatitis A virus cellular receptor 2 (HAVCR2), belongs to T cell immunoglobulin and mucin domain (TIM) family. TIM3 is expressed in Th1 and Th17 CD4 T cells, CD8 T cells, and it is constitutively expressed by Tregs (162–165). In addition, TIM3 expression has been observed in NK cells and myeloid cells including monocytes, dendritic cells and macrophages (166, 167). TIM3 has several ligands including galectin-9, phosphatidylserine (PtdSer), high-mobility group box 1 (HMGB1), and carcinoembryonic antigen related cell adhesion molecule 1 (Ceacam-1) (168–171). Therefore, the cell type and the ligand that TIM3 engages determines the inhibitory mechanism. TIM3 binding to galectin has inhibitory function by inducing cell death of autoreactive Th1 T cells in an experimental autoimmune encephalomyelitis (EAE) model (169). Binding of TIM3 to PtdSer leads to the uptake of apoptotic cells and this pathway promotes cross-presentation of antigens by dendritic cells (172). HMGB1 which normally binds to DNA released from dying cells induces activation of innate cells and secretion of inflammatory cytokines, and TIM3 binding interferes with this pathway (171). Ceacam-1 binds to TIM3 in cis and trans and both type of interactions drive inhibitory signals of TIM3.
TIM3 lack of classical inhibitory motifs, and its cytoplasmic domain has five conserved tyrosine residues that can be phosphorylated by Src kinases or interleukin inducible T cell kinase. In absence of interaction with its ligand, TIM3 is bound to the HLA-B associated transcript 3 (Bat3) and recruits the catalytic form of Lck, in this state TIM3 does not interferes with TCR signaling. In contrast, when TIM3 binds to the ligands such as galectin 3 and Ceacam-1, triggers phosphorylation of the tyrosine Y256 and Y263 and this results in the release of Bat3 allowing the recruitment of SH2 domain-containing proteins and inhibiting TCR signaling (127, 168, 173–175).
In addition, TIM3 interacts with the receptor phosphatases CD45 and CD148 disrupting the immunological synapse, suggesting additional mechanisms involved in the inhibitory function of this checkpoint receptor. In human CD8 T cells, TIM3 is localized in lipid rafts and its blockade allows formation of a stable immunological synapse (176).
TIM3 Expression in HIV Infection
In the context of HIV infection, polymorphism in HAVCR2, the gene that encodes TIM3, was partially associated with the susceptibility to HIV infection (177). In HIV/SIV acute infection, T cells expressed higher levels of TIM3 that is reduced following initiation of cART (178–180). In addition, expression of Bat3, the regulator of TIM3 activity, is reduced suggesting an active inhibitory signal by TIM3 during HIV infection (173). Accordantly, TIM3+ HIV-specific CD8 T cells are dysfunctional and have defective degranulation (80, 178, 179, 181–184). Blockade of TIM3 restore proliferation and functional capacity of HIV-specific CD8 T cells (178, 184, 185).
In CD4 T cells, TIM3 mainly regulates Th1 responses (162, 165, 169). In acute and chronically HIV infected patients, TIM3 expression in CD4 T cells was associated with immune activation markers such as CD38, viral loads, and CD4 T cell depletion (80, 178). CD4 T cells express higher levels of TIM3 than CD8 T cells and its expression was significantly reduced after viral suppression by cART (179). In HIV-specific CD4 T cells, TIM3 upregulation is associated with diminished effector function including cytokine production, proliferation, and cell survival (178, 180, 181, 186). This evidence suggests that TIM3 can regulate CD4 and CD8 T cell responses in the context of infection.
Other Checkpoint Receptors
Checkpoint receptors are expressed by distinct T cell subsets and their mode of action determines the wide arrays of effects in T cell immune response and their therapeutic potential. Other checkpoint receptors that have been shown to have inhibitory effects on HIV-specific T cell responses are CD160 and 2B4 (15, 20, 147). CD160 is expressed by HIV-specific CD8 T cells and its blockade improved proliferation and cytokine production (15). In addition, combination of checkpoint inhibitors such as anti-PD1 and anti-2B4 showed a synergistic effect in enhancing the proliferation capacity of HIV-specific CD8 T cells (147). In HIV elite controllers, a subset of HIV-specific CD8 T cells that co-express 2B4 and CD160 has cytolytic capacity, as measured by perforin expression suggesting that these receptors may have distinct role in regulating T cell responses in the context of HIV infection (20).
Therefore, the levels and the variety of checkpoint receptors expressed by HIV-specific T cells reflects immune activation and determines the extend of T cell exhaustion differentiation in the context of HIV infection (15, 116, 147).
The Role of the Checkpoint Receptors in Viral Persistence and Elimination
The main obstacle to cure HIV infection is elimination of the viral reservoirs which are established very early during infection (187). The viral reservoirs consist of replication-competent and defective form of viruses that accumulate and persist at several anatomical sites, preferentially the lymphoid tissues (188–192). Several reports have shown that despite successfully viral suppression by cART, there is a high heterogeneity among individuals in terms of the frequency of latently infected cells and residual viremia. Accordantly, viral blips are suggestive of higher HIV reservoirs, highlighting potential challenges in the role of these factors in cure strategies (193–196).
The immune system, particularly HIV-specific CD8 T cells are confronted with several challenges to eliminate the viral reservoirs. Latently infected cells contain viruses with escape mutations selected at early stages of infection (197). The presence of defective proviruses contributes to the immune activation, and creates a diversion in the elimination of replication competent viruses by CD8 T cells (189, 195). Chronic immune activation and exhaustion of HIV-specific T cells leads to immune dysfunction and failure of viral elimination (6, 198, 199).
The compartmentalization of the reservoirs inside the B cell follicles in the lymph nodes is a contributing factor for viral persistence. In the follicles, follicular dendritic cells can harbor infectious virus for extended periods of time promoting infection of newly recruited CD4 TFH cells. In addition, to gain access to the follicles, acquisition of CXCR5 expression by HIV-specific CD8 T cells is required representing another factor that contributes to HIV persistence (83, 84, 87, 88, 200–203).
Recent evidence had demonstrated that multiple immune checkpoint receptors are preferentially expressed by latently infected cells presenting an additional challenge to overcome by the virus-specific CD8 T cells (21, 22, 148, 204–207). In patients with suppressed viremia by cART, CD4 T cells co-expressing several checkpoint receptors such as PD1, TIGIT, and LAG3 contain ten-times more HIV-DNA than the checkpoint receptor negative CD4 T cell counterparts (21, 22, 208). Moreover, CTLA4 expression was observed in a subset of CD4 T cells that share a Treg phenotype and harbor replication competent viruses suggesting that many T cell subsets and checkpoint receptor dependent mechanisms are involved in viral persistence (209, 210).
Approaches targeting the viral reservoirs are under intensive investigation and blockade of checkpoint receptors can be one of them to “unshield” HIV infected cells and enhance effector function of HIV-specific CD8 T cells at tissue sites (23, 211).
The Impact of Targeting Immune Checkpoint Receptors in HIV-specific Responses and the Viral Reservoir
The compelling evidence about the role of the checkpoint receptors in HIV-specific T cell responses and viral persistence has driven pre- and clinical studies to assess the effects of immune checkpoint blockade for the treatment of HIV infection (summarized in Table 1, Figure 2).
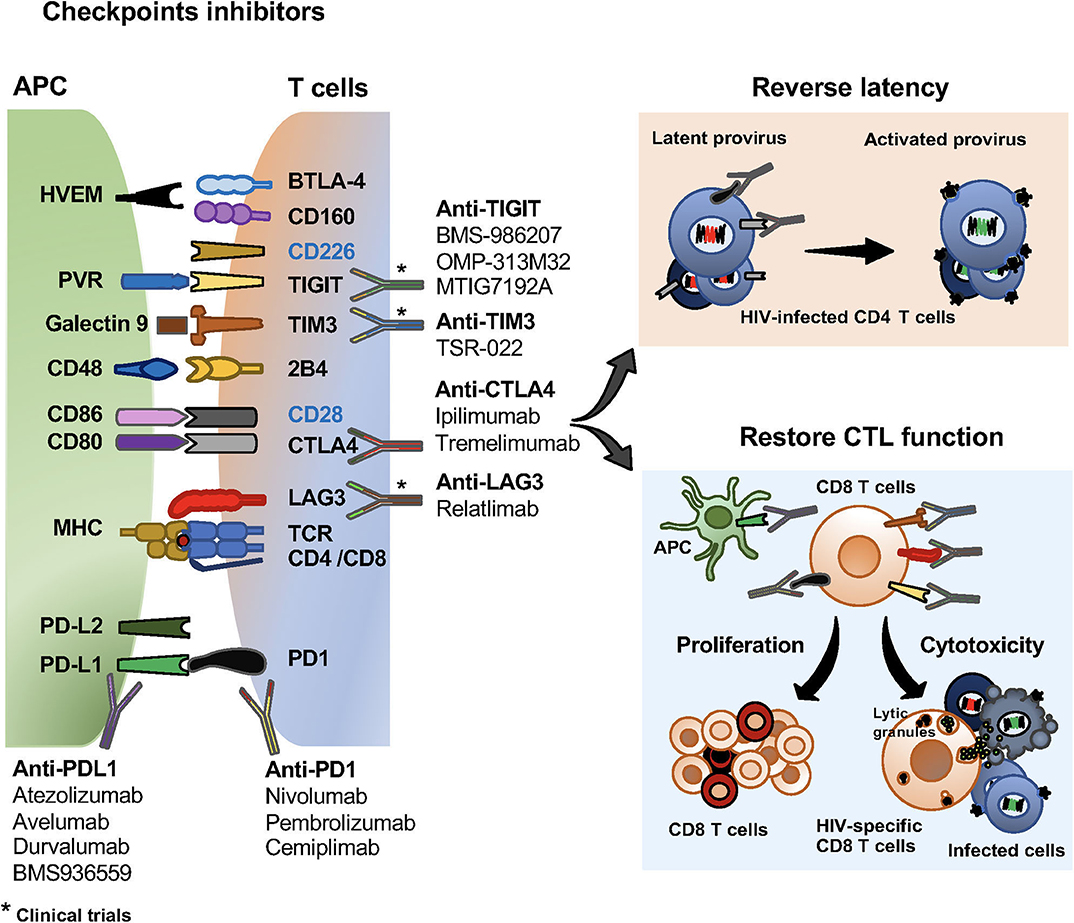
Figure 2. Checkpoint inhibitors and their effects on HIV specific T cells and the viral reservoir. Checkpoint inhibitors in clinical trials for the treatment of malignancies and HIV infection. Monoclonal antibodies blocking PD1, PD-L1, CTLA4, LAG3, TIGIT and TIM3 currently use in clinical trials. Effects of checkpoint receptors in reversing latency of the viral reservoir, and restoring CD8 T cell proliferation and cytotoxic functions.
Pre-clinical Studies
In vitro Studies
Recent studies using an in vitro infection model of mDCs-CD4 T cells co-culture, had shown the contribution of PD1 engagement in the establishment of HIV latency. In these culture conditions, blockade of PD1/PD-L1 pathway with pembrolizumab (anti-PD1) led to a modest but significant decrease in latently infected cell numbers (206). Pembrolizumab showed a synergistic effect when used in combination with a latency reversing agent bryostatin without enhancing T cell activation (207). In contrast, other report had shown small effects on viral replication in an ex-vivo stimulation of CD8 depleted PBMCs with CD3/CD28 in the presence of mAb BMS-936559 (anti-PD-L1) or Nivolumab (anti-PD1) (212). New studies are evaluating the effects of blockade of multiple checkpoint receptors. A recent report, showed that reversal of HIV latency was achieved by blockade of several checkpoint receptors PD-1, CTLA4, TIM3, and TIGIT without T cell stimulation, and this effect was significantly higher than using latent reversal agents such as vorinostat and bryostatin (231).
Therapeutic target of Tregs has been also considered in HIV cure strategies because they are susceptible to HIV infection and has regulatory functions on other cells such as HIV-specific T cells (119). In SIV/HIV infection viral DNA was detected in Tregs (209, 232–234). In co-culture experiments of Tregs and conventional CD4 T cells, Tregs reduced DC mediated viral infection of CD4 T cells. This effect was mediated by Treg inhibition of DC maturation, and actin polymerization that prevented the delivery of infectious viruses at the DC-T cell synapse. These effects were reversed by CTLA4 blockade (226). Moreover, in vitro CTLA4 blockade promoted HIV-specific CD4 T cell proliferation and cytokine production (13, 235). While Tregs can have both detrimental and beneficial effects in the context of HIV infection, new studies are ongoing to assess cure strategies targeting Tregs (119).
Checkpoint Inhibitor Treatment in SIV Infected RM
PD1/PD-L1 pathway
The blockade of the PD1/PD-L1 pathway is one of the most studied in the context of SIV infection as a single treatment or in combination with cytokines and other checkpoint inhibitors.
In chronically SIV infected macaques, administration of anti-PD1 (mouse anti-human clone EH12-1540) was well-tolerated, and enhanced anti-SIV immunity and viral control by improving SIV-specific CD8 T cell and B cell function (16, 213–215). Anti-PD1 treatment in combination with cART, reduced immune activation and improved memory B cell function (16, 214). In addition, PD1 blockade using a non-human primate anti-PD1, clone EH12-2132/2133, enhanced the expansion of CXCR5+ CD8 T cells expressing perforin and granzyme B, and reduced regulatory T cells resulting in decreased viral replication. These data suggested that blockade of PD1 have a potential effect in enhancing trafficking of CD8 T cells into the B cell follicles (24).
We and others have shown that administration of anti-PD-L1 antibodies (Avelumab and BMS-936559) in RM infected with SIV was safe and well-tolerated (222, 225). The effects on viral replication after discontinuation of cART showed that anti-PD-L1 (BMS-936559) led to a delay in the viral rebound although the effect was transient (225). In the Avelumab study, three animals were treated and showed no significant effect on viral rebound (222).
These pre-clinical studies blocking the PD1/PD-L1 pathway as single therapy suggested that combination therapies may achieve better outcomes in controlling viral replication. In this regard, rhIL-15 is a candidate to use with a checkpoint inhibitor. In in vitro, and in vivo studies in non-human primates, IL-15 promoted homeostatic proliferation, survival, and enhancing effector function of NK and memory CD8 T cells (236–241).
In SIV infected RM, while transient proliferation of CD8 T cells was observed, IL-15 did not enhanced CD4 T cell reconstitution at the lymph nodes and mucosal sites (242). A recent study used rhIL-15 in combination with anti-PD-L1 (Avelumab) in SIV infected RM. The treatment was safe and well-tolerated, and promoted expansion of CXCR3+PD1−/low SIVGag-specific CD8 T cells with effector function capacity and potential to traffic in peripheral tissues. However, no effect on viral rebound was observed after cART discontinuation (223). These data suggest that regimens promoting sustained expansion of this population may be more efficacious. In addition, the CXCR3+PD1−/low SIVGag-specific CD8 T cells may be targeted as a potential source of more effective virus-specific CD8 T cells, and this combination can be used as a tool to enhanced functionality of virus-specific cell preparation for adoptive T cell transfer therapies.
CTLA4 blockade
Accordantly to the in vitro data reported, in vivo CTLA4 blockade mostly impacts conventional CD4 T cells and Tregs. The effects of in vivo blockade of CTLA4 are difficult to interpret because the constitutive expression of this receptor by CD4+CD25highFoxP3+ Tregs, and conventional CD4+CD25− T cells express CTLA4 upon activation. Studies aiming to block Treg mediated immune suppression through CTLA4 were carried out using an anti-CTLA4 mAb (human antibody, MDX-010) in virally suppressed SIV infected RM (227). CTLA4 blockade facilitated viral control in lymphoid tissues when compared with cART only treated macaques (227). In contrast, another study showed that CTLA4 blockade during primary and chronic SIV infection, increased T cell immune activation and viral replication in tissues particularly at the mucosal sites suggesting that blockade of CTLA4 can have wider effects on T cells responses (228).
Administration of anti-CTLA4 in combination with PD1 blockade in SIV infected macaques, drove expansion of effector T cells, increased of plasma viremia and decreased cell associated DNA in B cell follicles indicating that this treatment facilitated viral activation and promote T cell function (217, 244).
CTLA4 and other checkpoint has been assessed in the context of vaccines. CTLA4 blockade in cynomolgus macaques administered at the time of an HIV envelope vaccine enhanced antibody responses. This study opens new avenues for the transient use of checkpoint receptors blockade as adjuvants in the context of HIV vaccines (245).
Clinical Studies
There are few reported and on-going clinical studies using checkpoint receptors to treat HIV infection (Table 2, Figure 2). Most of the published studies in HIV infected patients receiving checkpoint inhibitors are those for treatment of malignancies, and in addition to safety and efficacy, the effects on the viral load and HIV-specific responses are being evaluated.
The safety profile of anti-PD1 (Pembrolizumab) was assessed in an open-label, non-randomized, phase 1 study in HIV infected patients (n = 30) with advanced cancer. Pembrolizumab treatment was shown to be safe in this patient population (CD4 counts > 100 cells/μl and viral loads <200 copies/ml) (218). Similar observations were noted in a systematic review of published literature about anti-PD1 and anti-CTLA4 antibodies used for the treatment of advanced-stage cancer in patients with HIV infection (n = 73). This study showed similar safety characteristics to that observed in the non-HIV infected individuals (246).
In the studies in which virological and immunological parameters were monitored, the reported effects were not consistent among them. A case report study showed that administration of Nivolumab (anti-PD1) in one HIV infected patient with advanced non-small cell lung cancer (NSCLC) led to a mild and transient increase in cell-associated HIV DNA (219). Another case report using Nivolumab for treatment of lung cancer, reported transient increase in plasma viremia, accompanied by enhanced viral-specific CD8 T cell function. After multiple doses, a decrease in HIV cell-associated DNA was observed (220). In contrast, other studies reported none or inconsistent changes in cell associated RNA/DNA when blocking the PD1/PD-L1 pathway (221, 224). In addition, in patients with lung cancer treated with three or four doses of Nivolumab, no changes in plasma viremia and CD4 T cell counts (247). These inconsistencies are likely due to the small number of patients and differences in the methods to assess the viral reservoir.
The observation that multiple doses of anti-CTLA4 (Ipilimumab) for treatment of metastatic melanoma in an HIV infected patient, led to increase in cell-associated unspliced HIV RNA and a decreased in levels of viremia suggested that Ipilimumab may play a role in the elimination of latently infected CD4 T cells (229). Similarly, another individual treated with a single dose of Nivolumab showed increase of cell-associated HIV RNA in CD4 T cells without changes in HIV DNA or viremia (206). These observations indicate that PD1 blockade in combination with other checkpoint receptors can have an effect on reactivation of the viral reservoir.
Only two phase I studies have been published using checkpoint inhibitors to treat HIV infection and some more are ongoing (Table 2). A phase I (NCT02028403) randomized, double-blind, placebo-controlled, dose-escalating study using BMS-936559 was reported (224). In this study, a single dose administration of anti PD-L1 mAb (BMS-936559, 0.3 mg/kg) in 6 HIV-infected participants on cART and HIV RNA between 0.4 and 40 copies/mL by single copy assay (SCA) showed that treatment was well-tolerated and there was an increased trend of the proportion of HIVGag-specific CD8 T cells in two individuals. No changes were noted in the SCA over the 28 days post-infusion. This study was discontinued due to retinal toxicity observed in a parallel non-human primate study (224).
The second study is a dose escalating clinical trial using Ipilimumab, a fully human anti-CTLA4 antibody in HIV infected patients with uncontrolled viremia (NCT03407105). Ipilimumab monotherapy appeared to be safe and well-tolerated with mild to moderate adverse events at all doses tested. Treatment resulted in no significant changes in CD4 counts. The impact on HIV RNA varied among the patients, trending to a decrease in the lower dose group and an increased trend in the higher dose groups was observed (230).
The immunological and virological outcome of the treatment with checkpoint receptors in HIV infected patients remains unclear and more comprehensive studies are needed. The evidence that several checkpoint receptors may play a role in the pathogenesis of HIV infection suggests that combination therapy may achieve better outcomes (19, 23, 140, 211, 248). In this regard, ongoing clinical studies are evaluating the safety and efficacy of anti-LAG3, anti-TIGIT and anti-TIM3 antibodies alone or in combination with anti-PD1 for treatment of various types of malignancies (2). These checkpoint receptors are enriched in latently infected cells and the safety data of these studies will determine the potential use of these checkpoint receptors in HIV infection as reversal agents and/or to enhance HIV-specific T cell responses.
Immune Checkpoint Inhibitors Treatment for Malignancies in HIV Infected Patients
HIV infected patients are at higher risk of developing both AIDS defining malignancies as well as non-AIDS defining malignancies (249–253). Particularly, non-AIDS malignancies are the leading cause of morbidity and mortality (249–251, 254–257). Patients with HIV infection and malignancies are at higher risk of cancer related mortality than the general population (256, 258). The mechanisms by which HIV increased risk of development of cancer and its impact in the disease progression are largely unknown. Studies directed to understand the effect of HIV in the tumor microenvironment and its impact on treatment response to checkpoint inhibitors are lacking.
Patients with HIV infection were generally excluded from the clinical trials with immune checkpoint blockade, because of the safety concerns and the potential adverse outcomes (259–262). Case reports and clinical trials focusing on various types of malignancies for HIV infected patients started to emerge since 2017 (218, 263–273).
A recent systemic review summarized all published cases until April 2018, including 73 HIV-infected patients that received several immune checkpoint inhibitor treatments (including Pembrolizumab, Nivolumab, and Ipilimumab) for advanced cancer, evaluating the safety, antitumor efficacy as well as the impact on HIV viral load and CD4 counts. The review showed the immune checkpoint inhibitors are generally safe and well-tolerated for HIV infected patients with advanced cancers, with a similar adverse events profile to that observed in uninfected cancer patients. Nine percentage of patients reported grade 3 or higher immune-related toxicities, mostly occurring in patients receiving Ipilimumab as part of their regimen. The response rate to tumor is also comparable between HIV-infected and non-HIV-infected cancer patients. Ninety-three percentage of the patients starting with undetectable viral load remained suppressed during immune checkpoint inhibitor treatment. Among patients with a detectable HIV viral load, five out of six patients had a decrease in viral load (246, 274). Other report, showed stable viral loads and CD4 cell counts in 176 HIV infected patients reported in the literature (275).
The data reported from a phase I clinical trial (NCT02595866) evaluating the safety and tolerability of anti-PD1 antibody Pembrolizumab in 30 HIV patients with various advanced cancer including AIDS defining malignancy such as non-Hodgkin lymphoma, Kaposi sarcoma, and non-AIDS defining malignancy including anal cancer, advanced skin squamous cell carcinoma and others. Pembrolizumab was safe and showed similar tumor response rate to the cancer patients without HIV infection. In this study, an unexpected lethal herpesvirus-associated multicentric Castleman disease with polyclonal B-cell lymphoproliferation was reported for one patient with Kaposi sarcoma indicating a risk of using Pembrolizumab in this patient population. In addition, Pembrolizumab administration did not induce changes in CD4 counts and HIV viral loads in 77% of the patients. Seven patients showed viral blips although lower than 400 copies/ml (218). So far current data support the extended use of immune checkpoint inhibitors in HIV infected patients. More clinical trials focusing on HIV infected population are ongoing and data will be reported in the next years to better understand the efficacy and anti-tumor response of immune checkpoint blockade for the treatment of cancer in patients with HIV infection (Table 2).
Conclusions and Future Directions
The use of checkpoint inhibitors for the treatment of HIV infection is currently under intensive investigation. Blockade of checkpoint receptors restore immune function, and new opportunities had surfaced from the data suggesting that checkpoint inhibitors act as latency reversal agents to “unshield” HIV in infected cells.
The use of a checkpoint blockade in chronic HIV/SIV infection had shown transient effects in T cell mediated immunity compared to the success observed in the treatment of malignancies.
While T cell immunity in the setting of cancer and chronic HIV infection share similar immune mechanisms, the exhaustion in HIV-specific T cell seems to be difficult to reverse compared to the exhausted cells in the setting of cancer. Studies had shown that transcriptional and epigenetic programs are imprinted in exhausted cells, and this differentiation program limit their response to checkpoint receptor blockade. Therefore, duration of infection, chronic immune activation, and expression of several checkpoint receptors suggest that HIV-specific T cells may have limited plasticity. How to overcome these challenges requires further investigation, whether T cells at early stages of infection will be more susceptible to reverse exhaustion, or combination of several checkpoint receptors will achieve better results. Future studies directed to address transcriptional and epigenetic regulation of the differentiation pathways will unravel new targets to complement checkpoint blockade therapies that may be more advantageous in the setting of chronic infections.
Author Contributions
HC, MM, and MC wrote the review and edited the text. HC prepared the tables. MM prepared the figures. MC composed the manuscript. All authors listed have made intellectual contribution and approved it for submission.
Funding
This work was supported by Leidos Biomedical Research, Inc. and has been funded in whole or in part with federal funds. MC is also supported by the National Institute of Allergy and Infectious Diseases of the National Institutes of Health under the award AI145549-02.
Disclaimer
The content of this publication does not necessarily reflect the views or policies of the Department of Health and Human Services, nor does mention of trade names, commercial products, or organizations imply endorsement by the U.S. Government.
Conflict of Interest
The authors declare that the research was conducted in the absence of any commercial or financial relationships that could be construed as a potential conflict of interest.
References
1. Keir ME, Butte MJ, Freeman GJ, Sharpe AH. PD-1 and its ligands in tolerance and immunity. Annu Rev Immunol. (2008) 26:677–704. doi: 10.1146/annurev.immunol.26.021607.090331
2. Andrews LP, Yano H, Vignali DAA. Inhibitory receptors and ligands beyond PD-1, PD-L1 and CTLA-4: breakthroughs or backups. Nat Immunol. (2019) 20:1425–34. doi: 10.1038/s41590-019-0512-0
3. Pardoll DM Immunology beats cancer: a blueprint for successful translation. Nat Immunol. (2012) 13:1129–32. doi: 10.1038/ni.2392
4. Catalfamo M, Di Mascio M, Hu Z, Srinivasula S, Thaker V, Adelsberger J, et al. HIV infection-associated immune activation occurs by two distinct pathways that differentially affect CD4 and CD8 T cells. Proc Natl Acad Sci USA. (2008) 105:19851–6. doi: 10.1073/pnas.0810032105
5. Catalfamo M, Le Saout C, Lane HC. The role of cytokines in the pathogenesis and treatment of HIV infection. Cytokine Growth Factor Rev. (2012) 23:207–14. doi: 10.1016/j.cytogfr.2012.05.007
6. Klatt NR, Chomont N, Douek DC, Deeks SG. Immune activation and HIV persistence: implications for curative approaches to HIV infection. Immunol Rev. (2013) 254:326–42. doi: 10.1111/imr.12065
7. Doisne JM, Urrutia A, Lacabaratz-Porret C, Goujard C, Meyer L, Chaix ML, et al. CD8+ T cells specific for EBV, cytomegalovirus, and influenza virus are activated during primary HIV infection. J Immunol. (2004) 173:2410–8. doi: 10.4049/jimmunol.173.4.2410
8. Hersperger AR, Migueles SA, Betts MR, Connors M. Qualitative features of the HIV-specific CD8+ T-cell response associated with immunologic control. Curr Opin HIV AIDS. (2011) 6:169–73. doi: 10.1097/COH.0b013e3283454c39
9. Trautmann L, Janbazian L, Chomont N, Said EA, Gimmig S, Bessette B, et al. Upregulation of PD-1 expression on HIV-specific CD8+ T cells leads to reversible immune dysfunction. Nat Med. (2006) 12:1198–202. doi: 10.1038/nm1482
10. Petrovas C, Casazza JP, Brenchley JM, Price DA, Gostick E, Adams WC, et al. PD-1 is a regulator of virus-specific CD8+ T cell survival in HIV infection. J Exp Med. (2006) 203:2281–92. doi: 10.1084/jem.20061496
11. Petrovas C, Price DA, Mattapallil J, Ambrozak DR, Geldmacher C, Cecchinato V, et al. SIV-specific CD8+ T cells express high levels of PD1 and cytokines but have impaired proliferative capacity in acute and chronic SIVmac251 infection. Blood. (2007) 110:928–36. doi: 10.1182/blood-2007-01-069112
12. Zhang JY, Zhang Z, Wang X, Fu JL, Yao J, Jiao Y, et al. PD-1 up-regulation is correlated with HIV-specific memory CD8+ T-cell exhaustion in typical progressors but not in long-term nonprogressors. Blood. (2007) 109:4671–8. doi: 10.1182/blood-2006-09-044826
13. Kaufmann DE, Kavanagh DG, Pereyra F, Zaunders JJ, Mackey EW, Miura T, et al. Upregulation of CTLA-4 by HIV-specific CD4+ T cells correlates with disease progression and defines a reversible immune dysfunction. Nat Immunol. (2007) 8:1246–54. doi: 10.1038/ni1515
14. Quigley M, Pereyra F, Nilsson B, Porichis F, Fonseca C, Eichbaum Q, et al. Transcriptional analysis of HIV-specific CD8+ T cells shows that PD-1 inhibits T cell function by upregulating BAT. Nat Med F. (2010) 16:1147–51. doi: 10.1038/nm.2232
15. Peretz Y, He Z, Shi Y, Yassine-Diab B, Goulet JP, Bordi R, et al. CD160 and PD-1 co-expression on HIV-specific CD8 T cells defines a subset with advanced dysfunction. PLoS Pathog. (2012) 8:e1002840. doi: 10.1371/journal.ppat.1002840
16. Dyavar Shetty R, Velu V, Titanji K, Bosinger SE, Freeman GJ, Silvestri G, et al. PD-1 blockade during chronic SIV infection reduces hyperimmune activation and microbial translocation in rhesus macaques. J Clin Invest. (2012) 122:1712–6. doi: 10.1172/JCI60612
17. Hatano H, Jain V, Hunt PW, Lee TH, Sinclair E, Do TD, et al. Cell-based measures of viral persistence are associated with immune activation and programmed cell death protein 1 (PD-1)-expressing CD4+ T cells. J Infect Dis. (2013) 208:50–6. doi: 10.1093/infdis/jis630
18. Cockerham LR, Jain V, Sinclair E, Glidden DV, Hartogenesis W, Hatano H, et al. Programmed death-1 expression on CD4(+) and CD8(+) T cells in treated and untreated HIV disease. AIDS. (2014) 28:1749–58. doi: 10.1097/QAD.0000000000000314
19. Tian X, Zhang A, Qiu C, Wang W, Yang Y, Qiu C, et al. The upregulation of LAG-3 on T cells defines a subpopulation with functional exhaustion and correlates with disease progression in HIV-infected subjects. J Immunol. (2015) 194:3873–82. doi: 10.4049/jimmunol.1402176
20. Pombo C, Wherry EJ, Gostick E, Price DA, Betts MR. Elevated expression of CD160 and 2B4 defines a cytolytic HIV-specific CD8+ T-cell population in elite controllers. J Infect Dis. (2015) 212:1376–86. doi: 10.1093/infdis/jiv226
21. Chew GM, Fujita T, Webb GM, Burwitz BJ, Wu HL, Reed JS, et al. TIGIT marks exhausted t cells, correlates with disease progression, and serves as a target for immune restoration in HIV and SIV infection. PLoS Pathog. (2016) 12:e1005349. doi: 10.1371/journal.ppat.1005349
22. Fromentin R, Bakeman W, Lawani MB, Khoury G, Hartogensis W, DaFonseca S, et al. CD4+ Tcells expressing PD-1, TIGIT and LAG-3 contribute to HIV persistence during ART. PLoS Pathog. (2016) 12:e1005761. doi: 10.1371/journal.ppat.1005761
23. Wykes MN, Lewin SR. Immune checkpoint blockade in infectious diseases. Nat Rev Immunol. (2018) 18:91–4. doi: 10.1038/nri.2017.112
24. Mylvaganam GH, Chea LS, Tharp GK, Hicks S, Velu V, Iyer SS, et al. Combination anti-PD-1 and antiretroviral therapy provides therapeutic benefit against SIV. JCI Insight. (2018) 3:18. doi: 10.1172/jci.insight.122940
25. Fenwick C, Joo V, Jacquier P, Noto A, Banga R, Perreau M, et al. T-cell exhaustion in HIV infection. Immunol Rev. (2019) 292:149–63. doi: 10.1111/imr.12823
26. Koup RA, Safrit JT, Cao Y, Andrews CA, McLeod G, Borkowsky W, et al. Temporal association of cellular immune responses with the initial control of viremia in primary human immunodeficiency virus type 1 syndrome. J Virol. (1994) 68:4650–5. doi: 10.1128/JVI.68.7.4650-4655.1994
27. Schmitz JE, Kuroda MJ, Santra S, Sasseville VG, Simon MA, Lifton MA, et al. Control of viremia in simian immunodeficiency virus infection by CD8+ lymphocytes. Science. (1999) 283:857–60.
28. Lifson JD, Rossio JL, Piatak M Jr, Parks T, Li L, et al. Role of CD8(+) lymphocytes in control of simian immunodeficiency virus infection and resistance to rechallenge after transient early antiretroviral treatment. J Virol. (2001) 75:10187–99. doi: 10.1128/JVI.75.21.10187-10199.2001
29. Jin X, Bauer DE, Tuttleton SE, Lewin S, Gettie A, Blanchard J, et al. Dramatic rise in plasma viremia after CD8(+) T cell depletion in simian immunodeficiency virus-infected macaques. J Exp Med. (1999) 189:991–8. doi: 10.1084/jem.189.6.991
30. McDermott AB, Koup RA. CD8(+) T cells in preventing HIV infection and disease. AIDS. (2012) 26:1281–92. doi: 10.1097/QAD.0b013e328353bcaf
31. Betts MR, Nason MC, West SM, De Rosa SC, Migueles SA, Abraham J, et al. HIV nonprogressors preferentially maintain highly functional HIV-specific CD8+ T cells. Blood. (2006) 107:4781–9. doi: 10.1182/blood-2005-12-4818
32. Migueles SA, Connors M. Success and failure of the cellular immune response against HIV-1. Nat Immunol. (2015) 16:563–70. doi: 10.1038/ni.3161
33. Soghoian DZ, Streeck H. Cytolytic CD4(+) T cells in viral immunity. Expert Rev Vaccines. (2010) 9:1453–63. doi: 10.1586/erv.10.132
34. Morou A, Palmer BE, Kaufmann DE. Distinctive features of CD4+ T cell dysfunction in chronic viral infections. Curr Opin HIV AIDS. (2014) 9:446–51. doi: 10.1097/COH.0000000000000094
35. Buggert M, Frederiksen J, Lund O, Betts MR, Biague A, Nielsen M, et al. C.group, CD4+ T cells with an activated and exhausted phenotype distinguish immunodeficiency during aviremic HIV-2 infection. AIDS. (2016) 30:2415–26. doi: 10.1097/QAD.0000000000001223
36. Niessl J, Kaufmann DE. Harnessing Tfollicular helper cell responses for HIV vaccine development. Viruses. (2018) 10:6. doi: 10.3390/v10060336
37. Buggert M, Nguyen S, McLane LM, Steblyanko M, Anikeeva N, Paquin-Proulx D, et al. Limited immune surveillance in lymphoid tissue by cytolytic CD4+ T cells during health and HIV disease. PLoS Pathog. (2018) 14:e1006973. doi: 10.1371/journal.ppat.1006973
38. Morou A, Brunet-Ratnasingham E, Dube M, Charlebois R, Mercier E, Darko S, et al. Altered differentiation is central to HIV-specific CD4(+) T cell dysfunction in progressive disease. Nat Immunol. (2019) 20:1059–70. doi: 10.1038/s41590-019-0418-x
39. Churchill MJ, Deeks SG, Margolis DM, Siliciano RF, Swanstrom R. HIV reservoirs: what, where and how to target them. Nat Rev Microbiol. (2016) 14:55–60. doi: 10.1038/nrmicro.2015.5
40. Ishida Y, Agata Y, Shibahara K, Honjo T. Induced expression of PD-1, a novel member of the immunoglobulin gene superfamily, upon programmed cell death. EMBO J. (1992) 11:3887–95. doi: 10.1002/j.1460-2075.1992.tb05481.x
41. Nishimura H, Minato N, Nakano T, Honjo T. Immunological studies on PD-1 deficient mice: implication of PD-1 as a negative regulator for B cell responses. Int Immunol. (1998) 10:1563–72. doi: 10.1093/intimm/10.10.1563
42. Nishimura H, Nose M, Hiai H, Minato N, Honjo T. Development of lupus-like autoimmune diseases by disruption of the PD-1 gene encoding an ITIM motif-carrying immunoreceptor. Immunity. (1999) 11:141–51. doi: 10.1016/S1074-7613(00)80089-8
43. Hirahara K, Ghoreschi K, Yang XP, Takahashi H, Laurence A, Vahedi G, et al. Interleukin-27 priming of T cells controls IL-17 production in trans via induction of the ligand PD-L1. Immunity. (2012) 36:1017–30. doi: 10.1016/j.immuni.2012.03.024
44. Butte MJ, Keir ME, Phamduy TB, Sharpe AH, Freeman GJ. Programmed death-1 ligand 1 interacts specifically with the B7-1 costimulatory molecule to inhibit T cell responses. Immunity. (2007) 27:111–22. doi: 10.1016/j.immuni.2007.05.016
45. Latchman Y, Wood CR, Chernova T, Chaudhary D, Borde M, Chernova I, et al. PD-L2 is a second ligand for PD-1 and inhibits T cell activation. Nat Immunol. (2001) 2:261–8. doi: 10.1038/85330
46. Liang SC, Latchman YE, Buhlmann JE, Tomczak MF, Horwitz BH, Freeman GJ, et al. Regulation of PD-1, PD-L1, and PD-L2 expression during normal and autoimmune responses. Eur J Immunol. (2003) 33:2706–16. doi: 10.1002/eji.200324228
47. Sharpe AH, Pauken KE. The diverse functions of the PD1 inhibitory pathway. Nat Rev Immunol. (2018) 18:153–67. doi: 10.1038/nri.2017.108
48. Loke P, Allison JP. PD-L1 and PD-L2 are differentially regulated by Th1 and Th2 cells. Proc Natl Acad Sci USA. (2003) 100:5336–41. doi: 10.1073/pnas.0931259100
49. Youngnak P, Kozono Y, Kozono H, Iwai H, Otsuki N, Jin H, et al. Differential binding properties of B7-H1 and B7-DC to programmed death-1. Biochem Biophys Res Commun. (2003) 307:672–7. doi: 10.1016/S0006-291X(03)01257-9
50. Ghiotto M, Gauthier L, Serriari N, Pastor S, Truneh A, Nunes JA, et al. PD-L1 and PD-L2 differ in their molecular mechanisms of interaction with PD-1. Int Immunol. (2010) 22:651–60. doi: 10.1093/intimm/dxq049
51. Messal N, Serriari NE, Pastor S, Nunes JA, Olive D. PD-L2 is expressed on activated human T cells and regulates their function. Mol Immunol. (2011) 48:2214–9. doi: 10.1016/j.molimm.2011.06.436
52. Saunders PA, Hendrycks VR, Lidinsky WA, Woods ML. PD-L2:PD-1 involvement in T cell proliferation, cytokine production, and integrin-mediated adhesion. Eur J Immunol. (2005) 35:3561–9. doi: 10.1002/eji.200526347
53. Okazaki T, Maeda A, Nishimura H, Kurosaki T, Honjo T. PD-1 immunoreceptor inhibits B cell receptor-mediated signaling by recruiting src homology 2-domain-containing tyrosine phosphatase 2 to phosphotyrosine. Proc Natl Acad Sci USA. (2001) 98:13866–71. doi: 10.1073/pnas.231486598
54. Okazaki T, Honjo T. PD-1 and PD-1 ligands: from discovery to clinical application. Int Immunol. (2007) 19:813–24. doi: 10.1093/intimm/dxm057
55. Rota G, Niogret C, Dang AT, Barros CR, Fonta NP, Alfei F, et al. SHP-2 Is dispensable for establishing T cell exhaustion and for PD-1 signaling in vivo. Cell Rep. (2018) 23:39–49. doi: 10.1016/j.celrep.2018.03.026
56. Celis-Gutierrez J, Blattmann P, Zhai Y, Jarmuzynski N, Ruminski K, Gregoire C, et al. Quantitative interactomics in primary T cells provides a rationale for concomitant PD-1 and BTLA coinhibitor blockade in cancer immunotherapy. Cell Rep. (2019) 27:3315–30.e7. doi: 10.1016/j.celrep.2019.05.041
57. Sharpe AH, Freeman GJ. The B7-CD28 superfamily. Nat Rev Immunol. (2002) 2:116–26. doi: 10.1038/nri727
58. Sheppard KA, Fitz LJ, Lee JM, Benander C, George JA, Wooters J, et al. PD-1 inhibits T-cell receptor induced phosphorylation of the ZAP70/CD3zeta signalosome and downstream signaling to PKCtheta. FEBS Lett. (2004) 574:37–41. doi: 10.1016/j.febslet.2004.07.083
59. Chemnitz JM, Parry RV, Nichols KE, June CH, Riley JL. SHP-1 and SHP-2 associate with immunoreceptor tyrosine-based switch motif of programmed death 1 upon primary human T cell stimulation, but only receptor ligation prevents T cell activation. J Immunol. (2004) 173:945–54. doi: 10.4049/jimmunol.173.2.945
60. Parry RV, Chemnitz JM, Frauwirth KA, Lanfranco AR, Braunstein I, Kobayashi SV, et al. CTLA-4 and PD-1 receptors inhibit T-cell activation by distinct mechanisms. Mol Cell Biol. (2005) 25:9543–53. doi: 10.1128/MCB.25.21.9543-9553.2005
61. Patsoukis N, Brown J, Petkova V, Liu F, Li L, Boussiotis VA. Selective effects of PD-1 on Akt and Ras pathways regulate molecular components of the cell cycle and inhibit T cell proliferation. Sci Signal. (2012) 5:ra46. doi: 10.1126/scisignal.2002796
62. Yokosuka T, Takamatsu M, Kobayashi-Imanishi W, Hashimoto-Tane A, Azuma M, Saito T. Programmed cell death 1 forms negative costimulatory microclusters that directly inhibit T cell receptor signaling by recruiting phosphatase SHP2. J Exp Med. (2012) 209:1201–17. doi: 10.1084/jem.20112741
63. Patsoukis N, Sari D, Boussiotis VA. PD-1 inhibits T cell proliferation by upregulating p27 and p15 and suppressing Cdc25. Cell Cycle. (2012) 11:4305–9. doi: 10.4161/cc.22135
64. Hui E, Cheung J, Zhu J, Su X, Taylor MJ, Wallweber HA, et al. T cell costimulatory receptor CD28 is a primary target for PD-1-mediated inhibition. Science. (2017) 355:1428–33. doi: 10.1126/science.aaf1292
65. Sage PT, Francisco LM, Carman CV, Sharpe AH. The receptor PD-1 controls follicular regulatory T cells in the lymph nodes and blood. Nat Immunol. (2013) 14:152–61. doi: 10.1038/ni.2496
66. Sage PT, Sharpe AH. T follicular regulatory cells. Immunol Rev. (2016) 271:246–59. doi: 10.1111/imr.12411
67. Francisco LM, Salinas VH, Brown KE, Vanguri VK, Freeman GJ, Kuchroo VK, et al. PD-L1 regulates the development, maintenance, and function of induced regulatory T cells. J Exp Med. (2009) 206:3015–29. doi: 10.1084/jem.20090847
68. Shi J, Hou S, Fang Q, Liu X, Liu X, Qi H. PD-1 controls follicular T helper cell positioning and function. Immunity. (2018) 49:264–74.e4. doi: 10.1016/j.immuni.2018.06.012
69. Agata Y, Kawasaki A, Nishimura H, Ishida Y, Tsubata T, Yagita H, et al. Expression of the PD-1 antigen on the surface of stimulated mouse T and B lymphocytes. Int Immunol. (1996) 8:765–72. doi: 10.1093/intimm/8.5.765
70. Barber DL, Wherry EJ, Masopust D, Zhu B, Allison JP, Sharpe AH, et al. Restoring function in exhausted CD8 T cells during chronic viral infection. Nature. (2006) 439:682–7. doi: 10.1038/nature04444
71. Wherry EJ, Ha SJ, Kaech SM, Haining WN, Sarkar S, Kalia V, et al. Molecular signature of CD8+ T cell exhaustion during chronic viral infection. Immunity. (2007) 27:670–84. doi: 10.1016/j.immuni.2007.09.006
72. Crawford A, Angelosanto JM, Kao C, Doering TA, Odorizzi PM, Barnett BE, et al. Molecular and transcriptional basis of CD4(+) T cell dysfunction during chronic infection. Immunity. (2014) 40:289–302. doi: 10.1016/j.immuni.2014.01.005
73. Pauken KE, Wherry EJ. Overcoming T cell exhaustion in infection and cancer. Trends Immunol. (2015) 36:265–76. doi: 10.1016/j.it.2015.02.008
74. McLane LM, Abdel-Hakeem MS, Wherry EJ. CD8 Tcell exhaustion during chronic viral infection and cancer. Annu Rev Immunol. (2019) 37:457–95. doi: 10.1146/annurev-immunol-041015-055318
75. Im SJ, Hashimoto M, Gerner MY, Lee J, Kissick HT, Burger MC, et al. Defining CD8+ T cells that provide the proliferative burst after PD-1 therapy. Nature. (2016) 537:417–21. doi: 10.1038/nature19330
76. Utzschneider DT, Charmoy M, Chennupati V, Pousse L, Ferreira DP, Calderon-Copete S, et al. T cell factor 1-expressing memory-like CD8(+) T cells sustain the immune response to chronic viral infections. Immunity. (2016) 45:415–27. doi: 10.1016/j.immuni.2016.07.021
77. Blank CU, Haining WN, Held W, Hogan PG, Kallies A, Lugli E, et al. Defining 'T cell exhaustion'. Nat Rev Immunol. (2019) 19:665–74. doi: 10.1038/s41577-019-0221-9
78. Day CL, Kaufmann DE, Kiepiela P, Brown JA, Moodley ES, Reddy S, et al. PD-1 expression on HIV-specific T cells is associated with T-cell exhaustion and disease progression. Nature. (2006) 443:350–4. doi: 10.1038/nature05115
79. Breton G, Chomont N, Takata H, Fromentin R, Ahlers J, Filali-Mouhim A, et al. Programmed death-1 is a marker for abnormal distribution of naive/memory T cell subsets in HIV-1 infection. J Immunol. (2013) 191:2194–204. doi: 10.4049/jimmunol.1200646
80. Rallon N, Garcia M, Garcia-Samaniego J, Cabello A, Alvarez B, Restrepo C, et al. Expression of PD-1 and Tim-3 markers of T-cell exhaustion is associated with CD4 dynamics during the course of untreated and treated HIV infection. PLoS ONE. (2018) 13:e0193829. doi: 10.1371/journal.pone.0193829
81. Grabmeier-Pfistershammer K, Steinberger P, Rieger A, Leitner J, Kohrgruber N. Identification of PD-1 as a unique marker for failing immune reconstitution in HIV-1-infected patients on treatment. J Acquir Immune Defic Syndr. (2011) 56:118–24. doi: 10.1097/QAI.0b013e3181fbab9f
82. Streeck H, Brumme ZL, Anastario M, Cohen KW, Jolin JS, Meier A, et al. Antigen load and viral sequence diversification determine the functional profile of HIV-1-specific CD8+ T cells. PLoS Med. (2008) 5:e100. doi: 10.1371/journal.pmed.0050100
83. Perreau M, Savoye AL, De Crignis E, Corpataux JM, Cubas R, Haddad EK, et al. Follicular helper T cells serve as the major CD4 T cell compartment for HIV-1 infection, replication, and production. J Exp Med. (2013) 210:143–56. doi: 10.1084/jem.20121932
84. Fukazawa Y, Lum R, Okoye AA, Park H, Matsuda K, Bae JY, et al. B cell follicle sanctuary permits persistent productive simian immunodeficiency virus infection in elite controllers. Nat Med. (2015) 21:132–9. doi: 10.1038/nm.3781
85. Buggert M, Nguyen S, de Oca S-MG, Bengsch B, Darko S, Ransier A, et al. Identification and characterization of HIV-specific resident memory CD8(+) T cells in human lymphoid tissue. Sci Immunol. (2018) 3:24. doi: 10.1126/sciimmunol.aar4526
86. Buggert M, Japp AS, Betts MR. Everything in its right place: resident memory CD8+ T cell immunosurveillance of HIV infection. Curr Opin HIV AIDS. (2019) 14:93–99. doi: 10.1097/COH.0000000000000523
87. Fukazawa Y, Park H, Cameron MJ, Lefebvre F, Lum R, Coombes N, et al. Lymph node T cell responses predict the efficacy of live attenuated SIV vaccines. Nat Med. (2012) 18:1673–81. doi: 10.1038/nm.2934
88. Connick E, Mattila T, Folkvord JM, Schlichtemeier R, Meditz AL, Ray MG, et al. CTL fail to accumulate at sites of HIV-1 replication in lymphoid tissue. J Immunol. (2007) 178:6975–83. doi: 10.4049/jimmunol.178.11.6975
89. Petrovas C, Ferrando-Martinez S, Gerner MY, Casazza JP, Pegu A, Deleage C, et al. Follicular CD8 T cells accumulate in HIV infection and can kill infected cells in vitro via bispecific antibodies. Sci Transl Med. (2017) 9:373. doi: 10.1126/scitranslmed.aag2285
90. Mylvaganam GH, Rios D, Abdelaal HM, Iyer S, Tharp G, Mavigner M, et al. Dynamics of SIV-specific CXCR5+ CD8 T cells during chronic SIV infection. Proc Natl Acad Sci USA. (2017) 114:1976–81. doi: 10.1073/pnas.1621418114
91. Ferrando-Martinez S, Moysi E, Pegu A, Andrews S, Nganou Makamdop K, Ambrozak D, et al. Accumulation of follicular CD8+ T cells in pathogenic SIV infection. J Clin Invest. (2018) 128:2089–103. doi: 10.1172/JCI96207
92. Xiao M, Chen X, He R, Ye L. Differentiation and function of follicular CD8 T cells during human immunodeficiency virus infection. Front Immunol. (2018) 9:1095. doi: 10.3389/fimmu.2018.01095
93. Petrovas C, Velu V. Editorial: lymph node T cell dynamics and novel strategies for HIV cure. Front Immunol. (2018) 9:2950. doi: 10.3389/fimmu.2018.02950
94. Mueller SN, Mackay LK. Tissue-resident memory T cells: local specialists in immune defence. Nat Rev Immunol. (2016) 16:79–89. doi: 10.1038/nri.2015.3
95. Hombrink P, Helbig C, Backer RA, Piet B, Oja AE, Stark R, et al. Programs for the persistence, vigilance and control of human CD8(+) lung-resident memory T cells. Nat Immunol. (2016) 17:1467–78. doi: 10.1038/ni.3589
96. Mackay LK, Kallies A. Transcriptional regulation of tissue-resident lymphocytes. Trends Immunol. (2017) 38:94–103. doi: 10.1016/j.it.2016.11.004
97. Szabo PA, Levitin HM, Miron M, Snyder ME, Senda T, Yuan J, et al. Single-cell transcriptomics of human T cells reveals tissue and activation signatures in health and disease. Nat Commun. (2019) 10:4706. doi: 10.1038/s41467-019-12464-3
98. Lederman MM, Margolis L. The lymph node in HIV pathogenesis. Semin Immunol. (2008) 20:187–95. doi: 10.1016/j.smim.2008.06.001
99. Altfeld M, van Lunzen J, Frahm N, Yu XG, Schneider C, Eldridge RL, et al. Expansion of pre-existing, lymph node-localized CD8+ T cells during supervised treatment interruptions in chronic HIV-1 infection. J Clin Invest. (2002) 109:837–43. doi: 10.1172/JCI0214789
100. Schildberg FA, Klein SR, Freeman GJ, Sharpe AH. Coinhibitory pathways in the B7-CD28 ligand-receptor family. Immunity. (2016) 44:955–72. doi: 10.1016/j.immuni.2016.05.002
101. Wei SC, Levine JH, Cogdill AP, Zhao Y, Anang NAS, Andrews MC, et al. Distinct cellular mechanisms underlie anti-CTLA-4 and anti-PD-1 checkpoint blockade. Cell. (2017) 170:1120–33.e17. doi: 10.1016/j.cell.2017.07.024
102. Catalfamo M, Tai X, Karpova T, McNally J, Henkart PA. TcR-induced regulated secretion leads to surface expression of CTLA-4 in CD4+CD25+ T cells. Immunology. (2008) 125:70–9. doi: 10.1111/j.1365-2567.2008.02822.x
103. Valk E, Leung R, Kang H, Kaneko K, Rudd CE, Schneider H. T cell receptor-interacting molecule acts as a chaperone to modulate surface expression of the CTLA-4 coreceptor. Immunity. (2006) 25:807–21. doi: 10.1016/j.immuni.2006.08.024
104. Linsley PS, Bradshaw J, Greene J, Peach R, Bennett KL, Mittler RS. Intracellular trafficking of CTLA-4 and focal localization towards sites of TCR engagement. Immunity. (1996) 4:535–43. doi: 10.1016/S1074-7613(00)80480-X
105. Rudd CE, Taylor A, Schneider H. CD28 and CTLA-4 coreceptor expression and signal transduction. Immunol Rev. (2009) 229:12–26. doi: 10.1111/j.1600-065X.2009.00770.x
106. Banton MC, Inder KL, Valk E, Rudd CE, Schneider H. Rab8 binding to immune cell-specific adaptor LAX facilitates formation of trans-Golgi network-proximal CTLA-4 vesicles for surface expression. Mol Cell Biol. (2014) 34:1486–99. doi: 10.1128/MCB.01331-13
107. Marengere LE, Waterhouse P, Duncan GS, Mittrucker HW, Feng GS, Mak TW. Regulation of T cell receptor signaling by tyrosine phosphatase SYP association with CTLA-4. Science. (1996) 272:1170–3. doi: 10.1126/science.272.5265.1170
108. Chuang E, Fisher TS, Morgan RW, Robbins MD, Duerr JM, Vander Heiden MG, et al. The CD28 and CTLA-4 receptors associate with the serine/threonine phosphatase PP2A. Immunity. (2000) 13:313–22. doi: 10.1016/S1074-7613(00)00031-5
109. Cinek T, Sadra A, Imboden JB. Cutting edge: tyrosine-independent transmission of inhibitory signals by CTLA-4. J Immunol. (2000) 164:5–8. doi: 10.4049/jimmunol.164.1.5
110. Baroja ML, Luxenberg D, Chau T, Ling V, Strathdee CA, Carreno BM, et al. The inhibitory function of CTLA-4 does not require its tyrosine phosphorylation. J Immunol. (2000) 164:49–55. doi: 10.4049/jimmunol.164.1.49
111. Walker LS, Sansom DM. Confusing signals: recent progress in CTLA-4 biology. Trends Immunol. (2015) 36:63–70. doi: 10.1016/j.it.2014.12.001
112. Steiner K, Waase I, Rau T, Dietrich M, Fleischer B, Broker BM. Enhanced expression of CTLA-4 (CD152) on CD4+ T cells in HIV infection. Clin Exp Immunol. (1999) 115:451–7. doi: 10.1046/j.1365-2249.1999.00806.x
113. Leng Q, Bentwich Z, Magen E, Kalinkovich A, Borkow G. CTLA-4 upregulation during HIV infection: association with anergy and possible target for therapeutic intervention. AIDS. (2002) 16:519–29. doi: 10.1097/00002030-200203080-00002
114. Zaunders JJ, Ip S, Munier ML, Kaufmann DE, Suzuki K, Brereton C, et al. Infection of CD127+ (interleukin-7 receptor+) CD4+ cells and overexpression of CTLA-4 are linked to loss of antigen-specific CD4 T cells during primary human immunodeficiency virus type 1 infection. J Virol. (2006) 80:10162–72. doi: 10.1128/JVI.00249-06
115. Kaufmann DE, Walker BD. PD-1 and CTLA-4 inhibitory cosignaling pathways in HIV infection and the potential for therapeutic intervention. J Immunol. (2009) 182:5891–7. doi: 10.4049/jimmunol.0803771
116. Kassu A, Marcus RA, D'Souza MB, Kelly-McKnight EA, Golden-Mason L, Akkina R, et al. Regulation of virus-specific CD4+ T cell function by multiple costimulatory receptors during chronic HIV infection. J Immunol. (2010) 185:3007–18. doi: 10.4049/jimmunol.1000156
117. Teigler JE, Zelinskyy G, Eller MA, Bolton DL, Marovich M, Gordon AD, et al. Differential inhibitory receptor expression on T cells delineates functional capacities in chronic viral infection. J Virol. (2017) 91:23. doi: 10.1128/JVI.01263-17
118. Noyan K, Nguyen S, Betts MR, Sonnerborg A, Buggert M. Human immunodeficiency virus type-1 elite controllers maintain low co-expression of inhibitory receptors on CD4+ T cells. Front Immunol. (2018) 9:19. doi: 10.3389/fimmu.2018.00019
119. Kleinman AJ, Sivanandham R, Pandrea I, Chougnet CA, Apetrei C. Regulatory T Cells as potential targets for HIV cure research. Front Immunol. (2018) 9:734. doi: 10.3389/fimmu.2018.00734
120. Imamichi H, Lane HC. Regulatory T cells in HIV-1 infection: the good, the bad, and the ugly. J Infect Dis. (2012) 205:1479–82. doi: 10.1093/infdis/jis238
121. Lopez-Abente J, Correa-Rocha R, Pion M. Functional mechanisms of treg in the context of HIV infection and the janus face of immune suppression. Front Immunol. (2016) 7:192. doi: 10.3389/fimmu.2016.00192
122. Wing JB, Sakaguchi S. Multiple treg suppressive modules and their adaptability. Front Immunol. (2012) 3:178. doi: 10.3389/fimmu.2012.00178
123. Boasso A, Vaccari M, Hryniewicz A, Fuchs D, Nacsa J, Cecchinato V, et al. Regulatory T-cell markers, indoleamine 2,3-dioxygenase, and virus levels in spleen and gut during progressive simian immunodeficiency virus infection. J Virol. (2007) 81:11593–603. doi: 10.1128/JVI.00760-07
124. Nigam P, Velu V, Kannanganat S, Chennareddi L, Kwa S, Siddiqui M, et al. Expansion of FOXP3+ CD8 T cells with suppressive potential in colorectal mucosa following a pathogenic simian immunodeficiency virus infection correlates with diminished antiviral T cell response and viral control. J Immunol. (2010) 184:1690–701. doi: 10.4049/jimmunol.0902955
125. Andersson J, Boasso A, Nilsson J, Zhang R, Shire NJ, Lindback S, et al. The prevalence of regulatory T cells in lymphoid tissue is correlated with viral load in HIV-infected patients. J Immunol. (2005) 174:3143–7. doi: 10.4049/jimmunol.174.6.3143
126. Huang CT, Workman CJ, Flies D, Pan X, Marson AL, Zhou G, et al. Role of LAG-3 in regulatory T cells. Immunity. (2004) 21:503–13. doi: 10.1016/j.immuni.2004.08.010
127. Anderson AC, Joller N, Kuchroo VK. Lag-3, Tim-3, and TIGIT: co-inhibitory receptors with specialized functions in immune regulation. Immunity. (2016) 44:989–1004. doi: 10.1016/j.immuni.2016.05.001
128. Huard B, Mastrangeli R, Prigent P, Bruniquel D, Donini S, El-Tayar N, et al. Characterization of the major histocompatibility complex class II binding site on LAG-3 protein. Proc Natl Acad Sci USA. (1997) 94:5744–9. doi: 10.1073/pnas.94.11.5744
129. De Sousa Linhares, Leitner J, Grabmeier-Pfistershammer K, Steinberger P. Not all immune checkpoints are created equal. Front Immunol. (2018) 9:1909. doi: 10.3389/fimmu.2018.01909
130. Hannier S, Triebel F. The MHC class II ligand lymphocyte activation gene-3 is co-distributed with CD8 and CD3-TCR molecules after their engagement by mAb or peptide-MHC class I complexes. Int Immunol. (1999) 11:1745–52. doi: 10.1093/intimm/11.11.1745
131. Annunziato F, Manetti R, Tomasevic I, Guidizi MG, Biagiotti R, Gianno V, et al. Expression and release of LAG-3-encoded protein by human CD4+ T cells are associated with IFN-gamma production. FASEB J. (1996) 10:769–76. doi: 10.1096/fasebj.10.7.8635694
132. Hannier S, Tournier M, Bismuth G, Triebel F. CD3/TCR complex-associated lymphocyte activation gene-3 molecules inhibit CD3/TCR signaling. J Immunol. (1998) 161:4058–65.
133. Workman CJ, Rice DS, Dugger KJ, Kurschner C, Vignali DA. Phenotypic analysis of the murine CD4-related glycoprotein, CD223 (LAG-3). Eur J Immunol. (2002) 32:2255–63. doi: 10.1002/1521-4141(200208)32:8<2255::AID-IMMU2255>3.0.CO;2-A
134. Xu F, Liu J, Liu D, Liu B, Wang M, Hu Z, et al. LSECtin expressed on melanoma cells promotes tumor progression by inhibiting antitumor T-cell responses. Cancer Res. (2014) 74:3418–28. doi: 10.1158/0008-5472.CAN-13-2690
135. Kouo T, Huang L, Pucsek AB, Cao M, Solt S, Armstrong T, et al. Galectin-3 shapes antitumor immune responses by suppressing CD8+ T cells via LAG-3 and inhibiting expansion of plasmacytoid dendritic cells. Cancer Immunol Res. (2015) 3:412–23. doi: 10.1158/2326-6066.CIR-14-0150
136. Wang J, Sanmamed MF, Datar I, Su TT, Ji L, Sun J, et al. Fibrinogen-like protein 1 is a major immune inhibitory ligand of LAG-3. Cell. (2019) 176:334–47.e12. doi: 10.1016/j.cell.2018.11.010
137. Workman CJ, Dugger KJ, Vignali DA. Cutting edge: molecular analysis of the negative regulatory function of lymphocyte activation gene-3. J Immunol. (2002) 169:5392–5. doi: 10.4049/jimmunol.169.10.5392
138. Li N, Workman CJ, Martin SM, Vignali DA. Biochemical analysis of the regulatory T cell protein lymphocyte activation gene-3 (LAG-3; CD223). J Immunol. (2004) 173:6806–12. doi: 10.4049/jimmunol.173.11.6806
139. Hoffmann M, Pantazis N, Martin GE, Hickling S, Hurst J, Meyerowitz J, et al. Exhaustion of activated CD8 T cells predicts disease progression in primary HIV-1 infection. PLoS Pathog. (2016) 12:e1005661. doi: 10.1371/journal.ppat.1005661
140. Hurst J, Hoffmann M, Pace M, Williams JP, Thornhill J, Hamlyn E, et al. Immunological biomarkers predict HIV-1 viral rebound after treatment interruption. Nat Commun. (2015) 6:8495. doi: 10.1038/ncomms9495
141. Bosinger SE, Li Q, Gordon SN, Klatt NR, Duan L, Xu L, et al. Global genomic analysis reveals rapid control of a robust innate response in SIV-infected sooty mangabeys. J Clin Invest. (2009) 119:3556–72. doi: 10.1172/JCI40115
142. Li Q, Smith AJ, Schacker TW, Carlis JV, Duan L, Reilly CS, et al. Microarray analysis of lymphatic tissue reveals stage-specific, gene expression signatures in HIV-1 infection. J Immunol. (2009) 183:1975–82. doi: 10.4049/jimmunol.0803222
143. Rotger M, Dalmau J, Rauch A, McLaren P, Bosinger SE, Martinez R, et al. Comparative transcriptomics of extreme phenotypes of human HIV-1 infection and SIV infection in sooty mangabey and rhesus macaque. J Clin Invest. (2011) 121:2391–400. doi: 10.1172/JCI45235
144. Juno JA, Stalker AT, Waruk JL, Oyugi J, Kimani M, Plummer FA, et al. Elevated expression of LAG-3, but not PD-1, is associated with impaired iNKT cytokine production during chronic HIV-1 infection and treatment. Retrovirology. (2015) 12:17. doi: 10.1186/s12977-015-0142-z
145. Nunnari G, Fagone P, Condorelli F, Nicoletti F, Malaguarnera L, Di rosa M. CD4+ T-cell gene expression of healthy donors, HIV-1 and elite controllers: immunological chaos. Cytokine. (2016) 83:127–35. doi: 10.1016/j.cyto.2016.04.007
146. Pallikkuth S, Pahwa R, Kausalya B, Saravanan S, Pan L, Vignesh R, et al. Cardiac morbidity in HIV infection is associated with checkpoint inhibitor LAG-3 on CD4 T cells. PLoS ONE. (2018) 13:e0206256. doi: 10.1371/journal.pone.0206256
147. Yamamoto T, Price DA, Casazza JP, Ferrari G, Nason M, Chattopadhyay PK, et al. Surface expression patterns of negative regulatory molecules identify determinants of virus-specific CD8+ T-cell exhaustion in HIV infection. Blood. (2011) 117:4805–15. doi: 10.1182/blood-2010-11-317297
148. Graydon CG, Balasko AL, Fowke KR. Roles, function and relevance of LAG3 in HIV infection. PLoS Pathog. (2019) 15:e1007429. doi: 10.1371/journal.ppat.1007429
149. Koch K, Koch N, Sandaradura U, de Silva, Jung NJ, Schulze zur W, et al. Increased frequency of CD49b/LAG-3(+) type 1 regulatory T cells in HIV-infected individuals. AIDS Res Hum Retroviruses. (2015) 31:1238–46. doi: 10.1089/aid.2014.0356
150. Bhagwat B, Cherwinski H, Sathe M, Seghezzi W, McClanahan TK, de Waal R, et al. Establishment of engineered cell-based assays mediating LAG3 and PD1 immune suppression enables potency measurement of blocking antibodies and assessment of signal transduction. J Immunol Methods. (2018) 456:7–14. doi: 10.1016/j.jim.2018.02.003
151. Yu X, Harden K, Gonzalez LC, Francesco M, Chiang E, Irving B, et al. The surface protein TIGIT suppresses T cell activation by promoting the generation of mature immunoregulatory dendritic cells. Nat Immunol. (2009) 10:48–57. doi: 10.1038/ni.1674
152. Stanietsky N, Simic H, Arapovic J, Toporik A, Levy O, Novik A, et al. The interaction of TIGIT with PVR and PVRL2 inhibits human NK cell cytotoxicity. Proc Natl Acad Sci USA. (2009) 106:17858–63. doi: 10.1073/pnas.0903474106
153. Boles KS, Vermi W, Facchetti F, Fuchs A, Wilson TJ, Diacovo TG, et al. A novel molecular interaction for the adhesion of follicular CD4 T cells to follicular DC. Eur J Immunol. (2009) 39:695–703. doi: 10.1002/eji.200839116
154. Tauriainen J, Scharf L, Frederiksen J, Naji A, Ljunggren HG, Sonnerborg A, et al. Perturbed CD8(+) T cell TIGIT/CD226/PVR axis despite early initiation of antiretroviral treatment in HIV infected individuals. Sci Rep. (2017) 7:40354. doi: 10.1038/srep40354
155. Liu S, Zhang H, Li M, Hu D, Li C, Ge B, et al. Recruitment of Grb2 and SHIP1 by the ITT-like motif of TIGIT suppresses granule polarization and cytotoxicity of NK cells. Cell Death Differ. (2013) 20:456–64. doi: 10.1038/cdd.2012.141
156. Li M, Xia P, Du Y, Liu S, Huang G, Chen J, et al. T-cell immunoglobulin and ITIM domain (TIGIT) receptor/poliovirus receptor (PVR) ligand engagement suppresses interferon-gamma production of natural killer cells via beta-arrestin 2-mediated negative signaling. J Biol Chem. (2014) 289:17647–57. doi: 10.1074/jbc.M114.572420
157. Joller N, Hafler JP, Brynedal B, Kassam N, Spoerl S, Levin SD, et al. Cutting edge: TIGIT has T cell-intrinsic inhibitory functions. J Immunol. (2011) 186:1338–42. doi: 10.4049/jimmunol.1003081
158. Bowler S, Chew GM, Budoff M, Chow D, Mitchell BI, D'Antoni ML, et al. PD-1+ and TIGIT+ CD4 T cells are associated with coronary artery calcium progression in HIV-infected treated adults. J Acquir Immune Defic Syndr. (2019) 81:e21–3. doi: 10.1097/QAI.0000000000002001
159. Belkina AC, Starchenko A, Drake KA, Proctor EA, Pihl RMF, Olson A, et al. Multivariate computational analysis of gamma delta T cell inhibitory receptor signatures reveals the divergence of healthy and ART-Suppressed HIV+ aging. Front Immunol. (2018) 9:2783. doi: 10.3389/fimmu.2018.02783
160. Yin X, Liu T, Wang Z, Ma M, Lei J, Zhang Z, et al. Expression of the inhibitory receptor TIGIT is up-regulated specifically on NK cells with CD226 activating receptor from HIV-infected individuals. Front Immunol. (2018) 9:2341. doi: 10.3389/fimmu.2018.02341
161. Poultsidi A, Dimopoulos Y, He TF, Chavakis T, Saloustros E, Lee PP, et al. Lymph node cellular dynamics in cancer and HIV: what can we learn for the follicular CD4 (Tfh) cells? Front Immunol. (2018) 9:2233. doi: 10.3389/fimmu.2018.02233
162. Monney L, Sabatos CA, Gaglia JL, Ryu A, Waldner H, Chernova T, et al. Th1-specific cell surface protein Tim-3 regulates macrophage activation and severity of an autoimmune disease. Nature. (2002) 415:536–41. doi: 10.1038/415536a
163. Sabatos CA, Chakravarti S, Cha E, Schubart A, Sanchez-Fueyo A, Zheng XX, et al. Interaction of Tim-3 and Tim-3 ligand regulates T helper type 1 responses and induction of peripheral tolerance. Nat Immunol. (2003) 4:1102–10. doi: 10.1038/ni988
164. Wolf Y, Anderson AC, Kuchroo VK. TIM3 comes of age as an inhibitory receptor. Nat Rev Immunol. (2019) 20:173–85. doi: 10.1038/s41577-019-0224-6
165. Hastings WD, Anderson DE, Kassam N, Koguchi K, Greenfield EA, Kent SC, et al. TIM-3 is expressed on activated human CD4+ T cells and regulates Th1 and Th17 cytokines. Eur J Immunol. (2009) 39:2492–501. doi: 10.1002/eji.200939274
166. Das M, Zhu C, Kuchroo VK. Tim-3 and its role in regulating anti-tumor immunity. Immunol Rev. (2017) 276:97–111. doi: 10.1111/imr.12520
167. Anderson AC, Anderson DE, Bregoli L, Hastings WD, Kassam N, Lei C, et al. Promotion of tissue inflammation by the immune receptor Tim-3 expressed on innate immune cells. Science. (2007) 318:1141–3. doi: 10.1126/science.1148536
168. Huang YH, Zhu C, Kondo Y, Anderson AC, Gandhi A, Russell A, et al. CEACAM1 regulates TIM-3-mediated tolerance and exhaustion. Nature. (2015) 517:386–90. doi: 10.1038/nature13848
169. Zhu C, Anderson AC, Schubart A, Xiong H, Imitola J, Khoury SJ, et al. The Tim-3 ligand galectin-9 negatively regulates T helper type 1 immunity. Nat Immunol. (2005) 6:1245–52. doi: 10.1038/ni1271
170. DeKruyff RH, Bu X, Ballesteros A, Santiago C, Chim YL, Lee HH, et al. T cell/transmembrane, Ig, and mucin-3 allelic variants differentially recognize phosphatidylserine and mediate phagocytosis of apoptotic cells. J Immunol. (2010) 184:1918–30. doi: 10.4049/jimmunol.0903059
171. Chiba S, Baghdadi M, Akiba H, Yoshiyama H, Kinoshita I, Dosaka-Akita H, et al. Tumor-infiltrating DCs suppress nucleic acid-mediated innate immune responses through interactions between the receptor TIM-3 and the alarmin HMGB1. Nat Immunol. (2012) 13:832–42. doi: 10.1038/ni.2376
172. Nakayama M, Akiba H, Takeda K, Kojima Y, Hashiguchi M, Azuma M, et al. Tim-3 mediates phagocytosis of apoptotic cells and cross-presentation. Blood. (2009) 113:3821–30. doi: 10.1182/blood-2008-10-185884
173. Rangachari M, Zhu C, Sakuishi K, Xiao S, Karman J, Chen A, et al. Bat3 promotes T cell responses and autoimmunity by repressing Tim-3-mediated cell death and exhaustion. Nat Med. (2012) 18:1394–400. doi: 10.1038/nm.2871
174. Lee J, Su EW, Zhu C, Hainline S, Phuah J, Moroco JA, et al. Phosphotyrosine-dependent coupling of Tim-3 to T-cell receptor signaling pathways. Mol Cell Biol. (2011) 31:3963–74. doi: 10.1128/MCB.05297-11
175. van de Weyer PS, Muehlfeit M, Klose C, Bonventre JV, Walz G, Kuehn EW. A highly conserved tyrosine of Tim-3 is phosphorylated upon stimulation by its ligand galectin-9. Biochem Biophys Res Commun. (2006) 351:571–6. doi: 10.1016/j.bbrc.2006.10.079
176. Clayton KL, Haaland MS, Douglas-Vail MB, Mujib S, Chew GM, Ndhlovu LC, et al. T cell Ig and mucin domain-containing protein 3 is recruited to the immune synapse, disrupts stable synapse formation, and associates with receptor phosphatases. J Immunol. (2014) 192:782–91. doi: 10.4049/jimmunol.1302663
177. Sironi M, Biasin M, Gnudi F, Cagliani R, Saulle I, Forni D, et al. A regulatory polymorphism in HAVCR2 modulates susceptibility to HIV-1 infection. PLoS ONE. (2014) 9:e106442. doi: 10.1371/journal.pone.0106442
178. Jones RB, Ndhlovu LC, Barbour JD, Sheth PM, Jha AR, Long BR, et al. Tim-3 expression defines a novel population of dysfunctional T cells with highly elevated frequencies in progressive HIV-1 infection. J Exp Med. (2008) 205:2763–79. doi: 10.1084/jem.20081398
179. Kassu A, Marcus RA, D'Souza MB, Kelly-McKnight EA, Palmer BE. Suppression of HIV replication by antiretroviral therapy reduces TIM-3 expression on HIV-specific CD8(+) T cells. AIDS Res Hum Retroviruses. (2011) 27:1–3. doi: 10.1089/aid.2010.0156
180. Amancha PK, Hong JJ, Ansari AA, Villinger F. Up-regulation of Tim-3 on T cells during acute simian immunodeficiency virus infection and on antigen specific responders. AIDS. (2015) 29:531–6. doi: 10.1097/QAD.0000000000000589
181. Zhang ZN, Zhu ML, Chen YH, Fu YJ, Zhang TW, Jiang YJ, et al. Elevation of Tim-3 and PD-1 expression on T cells appears early in HIV infection, and differential Tim-3 and PD-1 expression patterns can be induced by common gamma -chain cytokines. Biomed Res Int. (2015) 2015:916936. doi: 10.1155/2015/916936
182. Fujita T, Burwitz BJ, Chew GM, Reed JS, Pathak R, Seger E, et al. Expansion of dysfunctional Tim-3-expressing effector memory CD8+ T cells during simian immunodeficiency virus infection in rhesus macaques. J Immunol. (2014) 193:5576–83. doi: 10.4049/jimmunol.1400961
183. Jin HT, Anderson AC, Tan WG, West EE, Ha SJ, Araki K, et al. Cooperation of Tim-3 and PD-1 in CD8 T-cell exhaustion during chronic viral infection. Proc Natl Acad Sci USA. (2010) 107:14733–8. doi: 10.1073/pnas.1009731107
184. Grabmeier-Pfistershammer K, Stecher C, Zettl M, Rosskopf S, Rieger A, Zlabinger GJ, et al. Antibodies targeting BTLA or TIM-3 enhance HIV-1 specific T cell responses in combination with PD-1 blockade. Clin Immunol. (2017) 183:167–73. doi: 10.1016/j.clim.2017.09.002
185. Sakhdari A, Mujib S, Vali B, Yue FY, MacParland S, Clayton K, et al. Tim-3 negatively regulates cytotoxicity in exhausted CD8+ T cells in HIV infection. PLoS ONE. (2012) 7:e40146. doi: 10.1371/journal.pone.0040146
186. Hafler DA, Kuchroo V. TIMs: central regulators of immune responses. J Exp Med. (2008) 205:2699–701. doi: 10.1084/jem.20082429
187. Chun TW, Engel D, Mizell SB, Ehler LA, Fauci AS. Induction of HIV-1 replication in latently infected CD4+ T cells using a combination of cytokines. J Exp Med. (1998) 188:83–91. doi: 10.1084/jem.188.1.83
188. Chun TW, Carruth L, Finzi D, Shen X, DiGiuseppe JA, Taylor H, et al. Quantification of latent tissue reservoirs and total body viral load in HIV-1 infection. Nature. (1997) 387:183–8. doi: 10.1038/387183a0
189. Imamichi H, Dewar RL, Adelsberger JW, Rehm CA, O'Doherty U, Paxinos EE, et al. Defective HIV-1 proviruses produce novel protein-coding RNA species in HIV-infected patients on combination antiretroviral therapy. Proc Natl Acad Sci USA. (2016) 113:8783–8. doi: 10.1073/pnas.1609057113
190. Finzi D, Blankson J, Siliciano JD, Margolick JB, Chadwick K, Pierson T, et al. Latent infection of CD4+ T cells provides a mechanism for lifelong persistence of HIV-1, even in patients on effective combination therapy. Nat Med. (1999) 5:512–7. doi: 10.1038/8394
191. Finzi D, Hermankova M, Pierson T, Carruth LM, Buck C, Chaisson RE, et al. Identification of a reservoir for HIV-1 in patients on highly active antiretroviral therapy. Science. (1997) 278:1295–300. doi: 10.1126/science.278.5341.1295
192. Wong JK, Hezareh M, Gunthard HF, Havlir DV, Ignacio CC, Spina CA, et al. Recovery of replication-competent HIV despite prolonged suppression of plasma viremia. Science. (1997) 278:1291–5. doi: 10.1126/science.278.5341.1291
193. Bachmann N, von Siebenthal C, Vongrad V, Turk T, Neumann K, Beerenwinkel N, et al. Determinants of HIV-1 reservoir size and long-term dynamics during suppressive AR. Nat Commun. (2019) 10:3193. doi: 10.1038/s41467-019-10884-9
194. Sengupta S, Siliciano RF. Targeting the latent reservoir for HIV-1. Immunity. (2018) 48:872–95. doi: 10.1016/j.immuni.2018.04.030
195. Pollack RA, Jones RB, Pertea M, Bruner KM, Martin AR, Thomas AS, et al. Defective HIV-1 proviruses are expressed and can be recognized by cytotoxic T lymphocytes, which shape the proviral landscape. Cell Host Microbe. (2017) 21:494–506.e4. doi: 10.1016/j.chom.2017.03.008
196. Wang FS, Zhang L, Douek D, McMichael A, Xu XN, Lewin SR. Strategies for an HIV cure: progress and challenges. Nat Immunol. (2018) 19:1155–8. doi: 10.1038/s41590-018-0242-8
197. Deng K, Pertea M, Rongvaux A, Wang L, Durand CM, Ghiaur G, et al. Broad CTL response is required to clear latent HIV-1 due to dominance of escape mutations. Nature. (2015) 517:381–5. doi: 10.1038/nature14053
198. Peluso MJ, Deeks SG, McCune JM. HIV “cure”: A shot in the arm? EBioMedicine. (2019) 42:3–5. doi: 10.1016/j.ebiom.2019.04.011
199. McManus WR, Bale MJ, Spindler J, Wiegand A, Musick A, Patro SC, et al. HIV-1 in lymph nodes is maintained by cellular proliferation during antiretroviral therapy. J Clin Invest. (2019) 130:4629–42. doi: 10.1172/JCI126714
200. Lindqvist M, van Lunzen J, Soghoian DZ, Kuhl BD, Ranasinghe S, Kranias G, et al. Expansion of HIV-specific T follicular helper cells in chronic HIV infection. J Clin Invest. (2012) 122:3271–80. doi: 10.1172/JCI64314
201. Petrovas C, Yamamoto T, Gerner MY, Boswell KL, Wloka K, Smith EC, et al. CD4 T follicular helper cell dynamics during SIV infection. J Clin Invest. (2012) 122:3281–94. doi: 10.1172/JCI63039
202. Smith BA, Gartner S, Liu Y, Perelson AS, Stilianakis NI, Keele BF, et al. Persistence of infectious HIV on follicular dendritic cells. J Immunol. (2001) 166:690–6. doi: 10.4049/jimmunol.166.1.690
203. Banga R, Procopio FA, Noto A, Pollakis G, Cavassini M, Ohmiti K, et al. PD-1(+) and follicular helper T cells are responsible for persistent HIV-1 transcription in treated aviremic individuals. Nat Med. (2016) 22:754–61. doi: 10.1038/nm.4113
204. Chomont N, El-Far M, Ancuta P, Trautmann L, Procopio FA, Yassine-Diab B, et al. HIV reservoir size and persistence are driven by T cell survival and homeostatic proliferation. Nat Med. (2009) 15:893–900. doi: 10.1038/nm.1972
205. El-Far M, Ancuta P, Routy JP, Zhang Y, Bakeman W, Bordi R, et al. Nef promotes evasion of human immunodeficiency virus type 1-infected cells from the CTLA-4-mediated inhibition of T-cell activation. J Gen Virol. (2015) 96:1463–77. doi: 10.1099/vir.0.000065
206. Evans VA, van der Sluis RM, Solomon A, Dantanarayana A, McNeil C, Garsia R, et al. Programmed cell death-1 contributes to the establishment and maintenance of HIV-1 latency. AIDS. (2018) 32:1491–7. doi: 10.1097/QAD.0000000000001849
207. Fromentin R, DaFonseca S, Costiniuk CT, El-Far M, Procopio FA, Hecht FM, et al. PD-1 blockade potentiates HIV latency reversal ex vivo in CD4(+) T cells from ART-suppressed individuals. Nat Commun. (2019) 10:814. doi: 10.1038/s41467-019-08798-7
208. Cohn LB, da Silva IT, Valieris R, Huang AS, Lorenzi JCC, Cohen YZ, et al. Clonal CD4(+) T cells in the HIV-1 latent reservoir display a distinct gene profile upon reactivation. Nat Med. (2018) 24:604–9. doi: 10.1038/s41591-018-0017-7
209. McGary CS, Deleage C, Harper J, Micci L, Ribeiro SP, Paganini S, et al. CTLA-4(+)PD-1(-) memory CD4(+) T cells critically contribute to viral persistence in antiretroviral therapy-suppressed, SIV-infected rhesus macaques. Immunity. (2017) 47:776–88.e5. doi: 10.1016/j.immuni.2017.09.018
210. Hoang TN, Harper JL, Pino M, Wang H, Micci L, King CT, et al. Bone marrow-derived CD4(+) T cells are depleted in simian immunodeficiency virus-infected macaques and contribute to the size of the replication-competent reservoir. J Virol. (2019) 93:18. doi: 10.1128/JVI.01344-18
211. Boyer Z, Palmer S. Targeting immune checkpoint molecules to eliminate latent HIV. Front Immunol. (2018) 9:2339. doi: 10.3389/fimmu.2018.02339
212. Bui JK, Cyktor JC, Fyne E, Campellone S, Mason SW, Mellors JW. Blockade of the PD-1 axis alone is not sufficient to activate HIV-1 virion production from CD4+ T cells of individuals on suppressive ART. PLoS ONE. (2019) 14:e0211112. doi: 10.1371/journal.pone.0211112
213. Velu V, Titanji K, Zhu B, Husain S, Pladevega A, Lai L, et al. Enhancing SIV-specific immunity in vivo by PD-1 blockade. Nature. (2009) 458:206–10. doi: 10.1038/nature07662
214. Titanji K, Velu V, Chennareddi L, Vijay-Kumar M, Gewirtz AT, Freeman GJ, et al. Acute depletion of activated memory B cells involves the PD-1 pathway in rapidly progressing SIV-infected macaques. J Clin Invest. (2010) 120:3878–90. doi: 10.1172/JCI43271
215. Finnefrock AC, Tang A, Li F, Freed DC, Feng M, Cox KS, et al. PD-1 blockade in rhesus macaques: impact on chronic infection and prophylactic vaccination. J Immunol. (2009) 182:980–7. doi: 10.4049/jimmunol.182.2.980
216. Bekerman E, Hesselgesser J, Carr B, Nagel M, Hung M, Wang A, et al. PD-1 blockade and TLR7 activation lack therapeutic benefit in chronic simian immunodeficiency virus-infected macaques on antiretroviral therapy. Antimicrob Agents Chemother. (2019) 63:11. doi: 10.1128/AAC.01163-19
217. Harper J, Gordon S, Chan CN, Wang H, Lindemuth E, Galardi C, et al. CTLA-4 and PD-1 dual blockade induces SIV reactivation without control of rebound after antiretroviral therapy interruption. Nat Med. (2020) 26:519–28. doi: 10.1038/s41591-020-0782-y
218. Uldrick TS, Goncalves PH, Abdul-Hay M, Claeys AJ, Emu B, Ernstoff MS, et al. Assessment of the safety of pembrolizumab in patients with HIV and advanced cancer-A phase 1 study. JAMA Oncol. (2019) 5:1332–9. doi: 10.1001/jamaoncol.2019.2244
219. Le Garff G, Samri A, Lambert-Niclot S, Even S, Lavole A, Cadranel J, et al. Transient HIV-specific T cells increase and inflammation in an HIV-infected patient treated with nivolumab. AIDS. (2017) 31:1048–51. doi: 10.1097/QAD.0000000000001429
220. Guihot A, Marcelin AG, Massiani MA, Samri A, Soulie C, Autran B, et al. Drastic decrease of the HIV reservoir in a patient treated with nivolumab for lung cancer. Ann Oncol. (2018) 29:517–8. doi: 10.1093/annonc/mdx696
221. Scully EP, Rutishauser RL, Simoneau CR, Delagreverie H, Euler Z, Thanh C, et al. Inconsistent HIV reservoir dynamics and immune responses following anti-PD-1 therapy in cancer patients with HIV infection. Ann Oncol. (2018) 29:2141–2. doi: 10.1093/annonc/mdy259
222. Gill AL, Green SA, Abdullah S, Le Saout C, Pittaluga S, Chen H, et al. Programed death-1/programed death-ligand 1 expression in lymph nodes of HIV infected patients: results of a pilot safety study in rhesus macaques using anti-programed death-ligand 1 (Avelumab). AIDS. (2016) 30:2487–93. doi: 10.1097/QAD.0000000000001217
223. Chen P, Chen H, Moussa M, Cheng J, Li T, Qin J, et al. Recombinant human interleukin-15 and anti-PD-L1 combination therapy expands a CXCR3+PD1-/low CD8 T-cell subset in simian immunodeficiency virus-infected rhesus macaques. J Infect Dis. (2020) 221:523–33. doi: 10.1093/infdis/jiz485
224. Gay CL, Bosch RJ, Ritz J, Hataye JM, Aga E, Tressler RL, et al. Clinical trial of the anti-PD-L1 antibody BMS-936559 in HIV-1 infected participants on suppressive antiretroviral therapy. J Infect Dis. (2017) 215:1725–33. doi: 10.1093/infdis/jix191
225. Mason SW, Sanisetty S, Osuna-Gutierrez C, Lim S-Y, ChaniewskiS Campellone S, et al. Viral suppression was induced by anti-PD-L1 following ARV-interruption in SIV-infected monkeys. In: 21st Conference on Retroviruses Opportunistic Infections. Boston, MA. (2014).
226. Moreno-Fernandez ME, Joedicke JJ, Chougnet CA. Regulatory T cells diminish HIV infection in dendritic cells - conventional CD4(+) T cell clusters. Front Immunol. (2014) 5:199. doi: 10.3389/fimmu.2014.00199
227. Hryniewicz A, Boasso A, Edghill-Smith Y, Vaccari M, Fuchs D, Venzon D, et al. CTLA-4 blockade decreases TGF-beta, IDO, and viral RNA expression in tissues of SIVmac251-infected macaques. Blood. (2006) 108:3834–42. doi: 10.1182/blood-2006-04-010637
228. Cecchinato V, Tryniszewska E, Ma ZM, Vaccari M, Boasso A, Tsai WP, et al. Immune activation driven by CTLA-4 blockade augments viral replication at mucosal sites in simian immunodeficiency virus infection. J Immunol. (2008) 180:5439–47. doi: 10.4049/jimmunol.180.8.5439
229. Wightman F, Solomon A, Kumar SS, Urriola N, Gallagher K, Hiener B, et al. Effect of ipilimumab on the HIV reservoir in an HIV-infected individual with metastatic melanoma. AIDS. (2015) 29:504–6. doi: 10.1097/QAD.0000000000000562
230. Colston E, Grasela D, Gardiner D, Bucy RP, Vakkalagadda B, Korman AJ, et al. An open-label, multiple ascending dose study of the anti-CTLA-4 antibody ipilimumab in viremic HIV patients. PLoS ONE. (2018) 13:e0198158. doi: 10.1371/journal.pone.0198158
231. Van der Sluis RM, Kumar NA, Pascoe RD, Zerbato JM, Evans VA, Dantanarayana AI, et al. Combination immune checkpoint blockade to reverse HIV latency. J Immunol. (2020) 204:1242–54. doi: 10.4049/jimmunol.1901191
232. Allers K, Loddenkemper C, Hofmann J, Unbehaun A, Kunkel D, Moos V, et al. Gut mucosal FOXP3+ regulatory CD4+ T cells and nonregulatory CD4+ T cells are differentially affected by simian immunodeficiency virus infection in rhesus macaques. J Virol. (2010) 84:3259–69. doi: 10.1128/JVI.01715-09
233. Zhang M, Robinson TO, Duverger A, Kutsch O, Heath SL, Cron RQ. Regulatory CD4 T cells inhibit HIV-1 expression of other CD4 T cell subsets via interactions with cell surface regulatory proteins. Virology. (2018) 516:21–9. doi: 10.1016/j.virol.2017.12.036
234. Tran TA, de Goer de Herve MG, Hendel-Chavez H, Dembele B, Le Nevot E, Abbed K, et al. Resting regulatory CD4 T cells: a site of HIV persistence in patients on long-term effective antiretroviral therapy. PLoS ONE. (2008) 3:e3305. doi: 10.1371/journal.pone.0003305
235. Larsson M, Shankar EM, Che KF, Saeidi A, Ellegard R, Barathan M, et al. Molecular signatures of T-cell inhibition in HIV-1 infection. Retrovirology. (2013) 10:31. doi: 10.1186/1742-4690-10-31
236. Waldmann TA The biology of interleukin-2 and interleukin-15: implications for cancer therapy and vaccine design. Nat Rev Immunol. (2006) 6:595–601. doi: 10.1038/nri1901
237. Liu K, Catalfamo M, Li Y, Henkart PA, Weng NP. IL-15 mimics T cell receptor crosslinking in the induction of cellular proliferation, gene expression, and cytotoxicity in CD8+ memory T cells. Proc Natl Acad Sci USA. (2002) 99:6192–7. doi: 10.1073/pnas.092675799
238. Pilipow K, Roberto A, Roederer M, Waldmann TA, Mavilio D, Lugli E. IL15 and T-cell Stemness in T-cell-Based Cancer Immunotherapy. Cancer Res. (2015) 75:5187–93. doi: 10.1158/0008-5472.CAN-15-1498
239. Berger C, Berger M, Hackman RC, Gough M, Elliott C, Jensen MC, et al. Safety and immunologic effects of IL-15 administration in nonhuman primates. Blood. (2009) 114:2417–26. doi: 10.1182/blood-2008-12-189266
240. Sneller MC, Kopp WC, Engelke KJ, Yovandich JL, Creekmore SP, Waldmann TA, et al. IL-15 administered by continuous infusion to rhesus macaques induces massive expansion of CD8+ T effector memory population in peripheral blood. Blood. (2011) 118:6845–8. doi: 10.1182/blood-2011-09-377804
241. Waldmann TA, Lugli E, Roederer M, Perera LP, Smedley JV, Macallister RP, et al. Safety (toxicity), pharmacokinetics, immunogenicity, and impact on elements of the normal immune system of recombinant human IL-15 in rhesus macaques. Blood. (2011) 117:4787–95. doi: 10.1182/blood-2010-10-311456
242. Lugli E, Mueller YM, Lewis MG, Villinger F, Katsikis PD, Roederer M. IL-15 delays suppression and fails to promote immune reconstitution in virally suppressed chronically SIV-infected macaques. Blood. (2011) 118:2520–9. doi: 10.1182/blood-2011-05-351155
243. Gonzalez-Cao M, Moran T, Dalmau J, Garcia-Corbacho J, Bracht JWP, Bernabe R, et al. Assessment of the feasibility and safety of durvalumab for treatment of solid tumors in patients with HIV-1 infection: the phase 2 DURVAST study. JAMA Oncol. (2020) 9:e200465. doi: 10.1001/jamaoncol.2020.046
244. Justin SG, Harper L, Galardi C, Wang H, McGary CS, King CT, et al. PD-1 and CTLA-4 blockade in macaques induces t-cell expansion and siv reactivation. Conference on Retroviruses and Opportunistic Infections. Seattle, Washington DC. (2019).
245. Bradley T, Kuraoka M, Yeh CH, Tian M, Chen H, Cain DW, et al. Immune checkpoint modulation enhances HIV-1 antibody induction. Nat Commun. (2020) 11:948. doi: 10.1038/s41467-020-14670-w
246. Cook MR, Kim C. Safety and efficacy of immune checkpoint inhibitor therapy in patients with HIV infection and advanced-stage cancer: a systematic review. JAMA Oncol. (2019) 5:1049–54. doi: 10.1001/jamaoncol.2018.6737
247. Lavole A, Guihot A, Veyri M, Lambotte O, Autran B, Cloarec N, et al. PD-1 blockade in HIV-infected patients with lung cancer: a new challenge or already a strategy? Ann Oncol. (2018) 29:1065–6. doi: 10.1093/annonc/mdx817
248. Pardons M, Baxter AE, Massanella M, Pagliuzza A, Fromentin R, Dufour C, et al. Single-cell characterization and quantification of translation-competent viral reservoirs in treated and untreated HIV infection. PLoS Pathog. (2019) 15:e1007619. doi: 10.1371/journal.ppat.1007619
249. Engels EA, Brock MV, Chen J, Hooker CM, Gillison M, Moore RD. Elevated incidence of lung cancer among HIV-infected individuals. J Clin Oncol. (2006) 24:1383–8. doi: 10.1200/JCO.2005.03.4413
250. Rubinstein PG, Aboulafia DM, Zloza A. Malignancies in HIV/AIDS: from epidemiology to therapeutic challenges. AIDS. (2014) 28:453–65. doi: 10.1097/QAD.0000000000000071
251. Shiels MS, Engels EA. Evolving epidemiology of HIV-associated malignancies. Curr Opin HIV AIDS. (2017) 12:6–11. doi: 10.1097/COH.0000000000000327
252. Adashek JJ, Junior PNA, Galanina N, Kurzrock R. Remembering the forgotten child: the role of immune checkpoint inhibition in patients with human immunod eficiency virus and cancer. J Immunother Cancer. (2019) 7:130. doi: 10.1186/s40425-019-0618-9
253. Hessol NA, Whittemore H, Vittinghoff E, Hsu LC, Ma D, Scheer S, et al. Incidence of first and second primary cancers diagnosed among people with HIV, 1985-2013: a population-based, registry linkage study. Lancet HIV. (2018), 5:e647–55. doi: 10.1016/S2352-3018(18)30179-6
254. Antiretroviral Therapy Cohort Collaboration. Causes of death in HIV-1-infected patients treated with antiretroviral therapy, 1996-2006: collaborative analysis of 13 HIV cohort studies. Clin Infect Dis. (2010) 50:1387–96. doi: 10.1086/652283
255. Smith CJ, Ryom L, Weber R, Morlat P, Pradier C, Reiss P, et al. Trends in underlying causes of death in people with HIV from 1999 to 2011 (D:A:D): a multicohort collaboration. Lancet. (2014) 384:241–8. doi: 10.1016/S0140-6736(14)60604-8
256. Croxford S, Kitching A, Desai S, Kall M, Edelstein M, Skingsley A, et al. Mortality and causes of death in people diagnosed with HIV in the era of highly active antiretroviral therapy compared with the general population: an analysis of a national observational cohort. Lancet Public Health. (2017) 2:e35–46. doi: 10.1016/S2468-2667(16)30020-2
257. Ji Y, Lu H. Malignancies in HIV-Infected and AIDS patients. Adv Exp Med Biol. (2017) 1018:167–79. doi: 10.1007/978-981-10-5765-6_10
258. Coghill AE, Shiels MS, Suneja G, Engels EA. Elevated cancer-specific mortality among HIV-infected patients in the United States. J Clin Oncol. (2015) 33:2376–83. doi: 10.1200/JCO.2014.59.5967
259. Suneja G, Shiels MS, Angulo R, Copeland GE, Gonsalves L, Hakenewerth AM, et al. Cancer treatment disparities in HIV-infected individuals in the United States. J Clin Oncol. (2014) 32:2344–50. doi: 10.1200/JCO.2013.54.8644
260. Johnson DB, Sullivan RJ, Menzies AM. Immune checkpoint inhibitors in challenging populations. Cancer. (2017) 123:1904–11. doi: 10.1002/cncr.30642
261. Uldrick TS, Ison G, Rudek MA, Noy A, Schwartz K, Bruinooge S, et al. Modernizing clinical trial eligibility criteria: recommendations of the American society of clinical oncology-friends of cancer research HIV working group. J Clin Oncol. (2017) 35:3774–80. doi: 10.1200/JCO.2017.73.7338
262. Gonzalez-Cao M, Martinez-Picado J, Karachaliou N, Rosell R, Meyerhans A. Cancer immunotherapy of patients with HIV infection. Clin Transl Oncol. (2019) 21:713–20. doi: 10.1007/s12094-018-1981-6
263. Little RF. Cancer clinical trials in persons with HIV infection. Curr Opin HIV AIDS. (2017) 12:84–8. doi: 10.1097/COH.0000000000000321
264. Ostios-Garcia L, Faig J, Leonardi GC, Adeni AE, Subegdjo SJ, Lydon CA, et al. Safety and efficacy of PD-1 inhibitors among HIV-positive patients with non-small cell lung cancer. J Thorac Oncol. (2018) 13:1037–42. doi: 10.1016/j.jtho.2018.03.031
265. Husnain M, Park W, Ramos JC, Johnson TE, Chan J, Dasari A, et al. Complete response to ipilimumab and nivolumab therapy in a patient with extensive extrapulmonary high-grade small cell carcinoma of the pancreas and HIV infection. J Immunother Cancer. (2018), 6:66. doi: 10.1186/s40425-018-0379-x
266. Puronen CE, Ford ES, Uldrick TS. Immunotherapy in people with HIV and cancer. Front Immunol. (2019) 10:2060. doi: 10.3389/fimmu.2019.02060
267. Heppt MV, Schlaak M, Eigentler TK, Kahler KC, Kiecker F, Loquai C, et al. Checkpoint blockade for metastatic melanoma and merkel cell carcinoma in HIV-positive patients. Ann Oncol. (2017) 28:3104–6. doi: 10.1093/annonc/mdx538
268. Dalla Pria A, Pinato DJ, Bracchi M, Bower M. Recent advances in HIV-associated Kaposi sarcoma. F1000Res. (2019) 8:970. doi: 10.12688/f1000research.17401.1
269. Bari S, Muzaffar J, Chan A, Jain SR, Haider AM, Adams Curry M, et al. Outcomes of programmed cell death protein 1 (PD-1) and programmed death-ligand 1(PD-L1) inhibitor therapy in HIV patients with advanced cancer. J Oncol. (2019) 2019:2989048. doi: 10.1155/2019/7921582
270. Spano JP, Veyri M, Gobert A, Guihot A, Perre P, Kerjouan M, et al. Immunotherapy for cancer in people living with HIV: safety with an efficacy signal from the series in real life experience. AIDS. (2019) 33:F13–9. doi: 10.1097/QAD.0000000000002298
271. Bender Ignacio RA, Lin LL, Rajdev L, Chiao E. Evolving paradigms in HIV malignancies: review of ongoing clinical trials. J Natl Compr Canc Netw. (2018) 16:1018–26. doi: 10.6004/jnccn.2018.7064
272. Galanina N, Goodman AM, Cohen PR, Frampton GM, Kurzrock R. Successful treatment of HIV-associated kaposi sarcoma with immune checkpoint blockade. Cancer Immunol Res. (2018) 6:1129–35. doi: 10.1158/2326-6066.CIR-18-0121
273. Sorotsky H, Hogg D, Amir E, Araujo DV. Characteristics of immune checkpoint inhibitors trials associated with inclusion of patients with HIV: a systematic review and meta-analysis. JAMA Netw Open. (2019) 2:e1914816. doi: 10.1001/jamanetworkopen.2019.14816
274. Cook MR, Kim C. Quality assessment of a systematic review for HIV infection and advanced-stage cancer-in reply. JAMA Oncol. (2019) 5:1369–70. doi: 10.1001/jamaoncol.2019.2206
Keywords: CD8 T cells, HIV, checkpoint receptors, checkpoint inhibition therapy, HIV pathogenesis
Citation: Chen H, Moussa M and Catalfamo M (2020) The Role of Immunomodulatory Receptors in the Pathogenesis of HIV Infection: A Therapeutic Opportunity for HIV Cure? Front. Immunol. 11:1223. doi: 10.3389/fimmu.2020.01223
Received: 05 December 2019; Accepted: 15 May 2020;
Published: 02 July 2020.
Edited by:
Ezequiel Ruiz-Mateos, Institute of Biomedicine of Seville (IBIS), SpainReviewed by:
Amarendra Pegu, National Institutes of Health (NIH), United StatesSuresh Pallikkuth, University of Miami, United States
Copyright © 2020 Chen, Moussa and Catalfamo. This is an open-access article distributed under the terms of the Creative Commons Attribution License (CC BY). The use, distribution or reproduction in other forums is permitted, provided the original author(s) and the copyright owner(s) are credited and that the original publication in this journal is cited, in accordance with accepted academic practice. No use, distribution or reproduction is permitted which does not comply with these terms.
*Correspondence: Marta Catalfamo, bWMyMTUxJiN4MDAwNDA7Z2VvcmdldG93bi5lZHU=
†These authors have contributed equally to this work