- 1Department of Translational Research and Cellular Therapeutics, Diabetes and Metabolism Research Institute, Beckman Research Institute, City of Hope National Medical Center, Duarte, CA, United States
- 2Department of Pharmacology and Toxicology, College of Pharmacy, King Saud University, Riyadh, Saudi Arabia
- 3Faculty of Pharmacy, Zagazig University, Zagazig, Egypt
Type 1 diabetes (T1D) arises secondary to immune-driven destruction of pancreatic β-cells and manifests as insulin-deficient hyperglycemia. We showed that oral vaccination with live attenuated Salmonella, which simultaneously delivers autoantigens and a TGFβ expression vector to immune cells in the gut mucosa, provides protection against the progression of T1D in non-obese diabetic (NOD) mice. In this study we employed the Sleeping Beauty (SB) transposon system that is composed of a transposase and transposon encoding the td-Tomato to express red fluorescent protein (RFP) to permanently mark the cells that take up the Salmonella vaccine. After animal vaccination, the transposon labeled-dendritic cells (DCs) with red fluorescence appeared throughout the secondary lymphoid tissues. Furthermore, Sleeping Beauty containing tgfβ1 gene (SB-tgfβ1) co-expressed TGFβ and RFP. The labeled DCs were detected predominantly in Peyer’s patches (PP) and mesenteric lymph nodes (MLN) and expressed CD103 surface marker. CD103+ DCs induced tolerogenic effects and gut homing. TGFβ significantly increased programmed death-ligand-1 (PDL-1 or CD274) expression in the DCs in the MLN and PP of treated mice. Also, TGFβ increased cytotoxic T-lymphocyte-associated protein-4 (CTLA-4) levels in CD4+ cells in MLN and PP. Interestingly, DCs increased in all lymphatic organs of mice vaccinated with oral live Salmonella-based vaccine expressing preproinsulin (PPI), in combination with TGFβ, IL10, and subtherapeutic-doses of anti-CD3 mAb compared with vehicle-treated mice. These DCs are mostly tolerogenic in MLN and PP. Furthermore the DCs obtained from vaccine-treated but not vehicle-treated mice suppressed in vitro T cell proliferation. These data suggest that the MLN and the PP are a central hub for the beneficial anti-diabetic effects of an oral Salmonella-based vaccine prevention of diabetes in rodents.
Introduction
Type 1 diabetes (T1D) results from auto-reactive killing of the pancreatic islet insulin-producing β-cells (1). Studies have shown that pre-proinsulin (PPI) is an initial autoantigen in non-obese diabetic (NOD) mice (2) and humans (3–5) promoting dysfunctional autoimmunity. Using oral antigen-specific vaccines of live attenuated (non-pathogenic) Salmonella typhimurium diabetic autoantigens have been delivered to mice (6, 7). Non-specific immune response in NOD mice was reported when an infection was induced with attenuated S. typhimurium (8–10). When orally administered, Salmonella moves from the gut to the gut-associated lymphoid tissues (GALT) of antigen presenting cells (APCs), resulting in formation of Salmonella-containing vacuoles (SCV) (11–13). Salmonella has two Type 3 Secretion Systems (T3SS) to invade and disseminate in organs (14). The T3SS encoded by Salmonella Pathogenicity Island 1 (SPI1-T3SS) is expressed by extracellular bacteria and is required for the invasion of non-phagocytic cells. The T3SS encoded by Salmonella Pathogenicity Island 2 (SPI2-T3SS) is expressed by intracellular Salmonella and is required for intracellular replication and systemic pathogenesis (15). The genes of SPI2-T3SS are induced inside the SCV and express specific antigens in GALT APCs. This has promoted the development of anti-cancer vaccines and a T1D vaccine (6, 10–12, 16–18). Fusion of heterologous antigens to specific SPI2-T3SS proteins causes them to be presented to lymphocytes within the gut mucosa (12, 19, 20). This minimizes and/or bypasses intestinal lumen antigen expression and subsequent degradation of the same. Salmonella can also deliver plasmids to host APCs and this feature has led to generation of DNA vaccines (21, 22). A novel diabetes vaccine enabled direct expression of tolerogenic cytokines like TGFβ and IL10 and induced tolerance to diabetic auto-antigens (10, 23). These APCs process and produce the antigens which migrate to other organs in the gut and stimulate the immune cells (9, 24).
Initial uptake of Salmonella vaccine by GALT-associated DCs helped promote oral tolerance (25). Antigens arising in the large bowel were transported to the GALT by migrating CD103+ DCs (25, 26). Tolerogenic dendritic cells (tolDCs) are especially active at suppressing immune activation (27–30). These effects are mediated through tolerogenic APC that induce T cell anergy, T cell apoptosis as well as induction of Tregs and type 1 regulatory T cells (Tr1) (28–35). CD103+ DCs induced tolerance and gut homing, protecting against colitis in mice. Also, reduction in celiac disease was attributed to the activity of CD11c+CD103+ DCs in rodents (36–38). We hypothesized that tolerogenic CD103+ DCs are intimately involved in the sampling and trafficking of the Salmonella antigen in NOD mice (37, 39–41). To identify the initial cell population mediating the oral vaccine effect, we developed a novel approach to label the cells that take up the Salmonella vaccine. It uses a transposon system known as Sleeping Beauty (SB;) (42) which is composed of Sleeping Beauty transposase and a transposon encoding the td-Tomato red fluorescent protein-expressing gene which permanently inserts into the genome of vertebrate animals (43, 44). Treating mice with Salmonella containing both plasmids enables passing of the plasmids to the host cells resulting in permanent marking of the cells. Using this approach permitted tracking and characterization of the cells mediating the vaccine effect. Here, we define the cells that uptake Salmonella and are essential for mediating the vaccine effect and characterize these cells as tolerogenic DCs based on the expression of regulatory molecules and secretion of cytokines.
Materials and Methods
Preparation of Salmonella Strain and Plasmid Construction
Salmonella typhimurium MvP728 (ΔhtrA/ΔpurD) has function as an oral vaccine vector (10, 23, 45). Bacteria were cultured in Luria-Bertani (LB) with or without chloramphenicol and/or carbenicillin (50 μg/ml).
The pSBbi-RP (#60513) (42) and pCMV(CAT)T7-SB100 (#34879) (46) plasmids for the Sleeping Beauty transposon system were from Addgene and kindly donated from the Kowarz and Izsvak Laboratories. The two plasmids were transformed into competent double mutant S. typhimurium MvP728 using electroporation (Bio Rad) after which sensitivity to carbenicillin and chloramphenicol was employed for selection.
Mouse tgfb1 sequence was amplified from pCMV6-Entry-tgfb1 (Origene, MR227339) using forward primer 5′-CCATGGATGCCGCCCTCGGG-3′ and 5′-AAGCTTTTAAACCTTATCGTCGTCATCCTTG-3′ reverse primer. The PCR fragment was cloned using TOPO TA cloning kit (Thermo fisher, 450641) and confirmed by sequencing. Next, the tgfb1 sequence was cut by NcoI/HindIII then subcloned into NcoI/HindIII digested pSBbi-RP plasmid to yield pSBbi-RP-TGFβ (Figure 1A). The pSBbi-RP-TGFβ and pCMV(CAT)T7-SB100 plasmids were transformed into Salmonella as described (47).
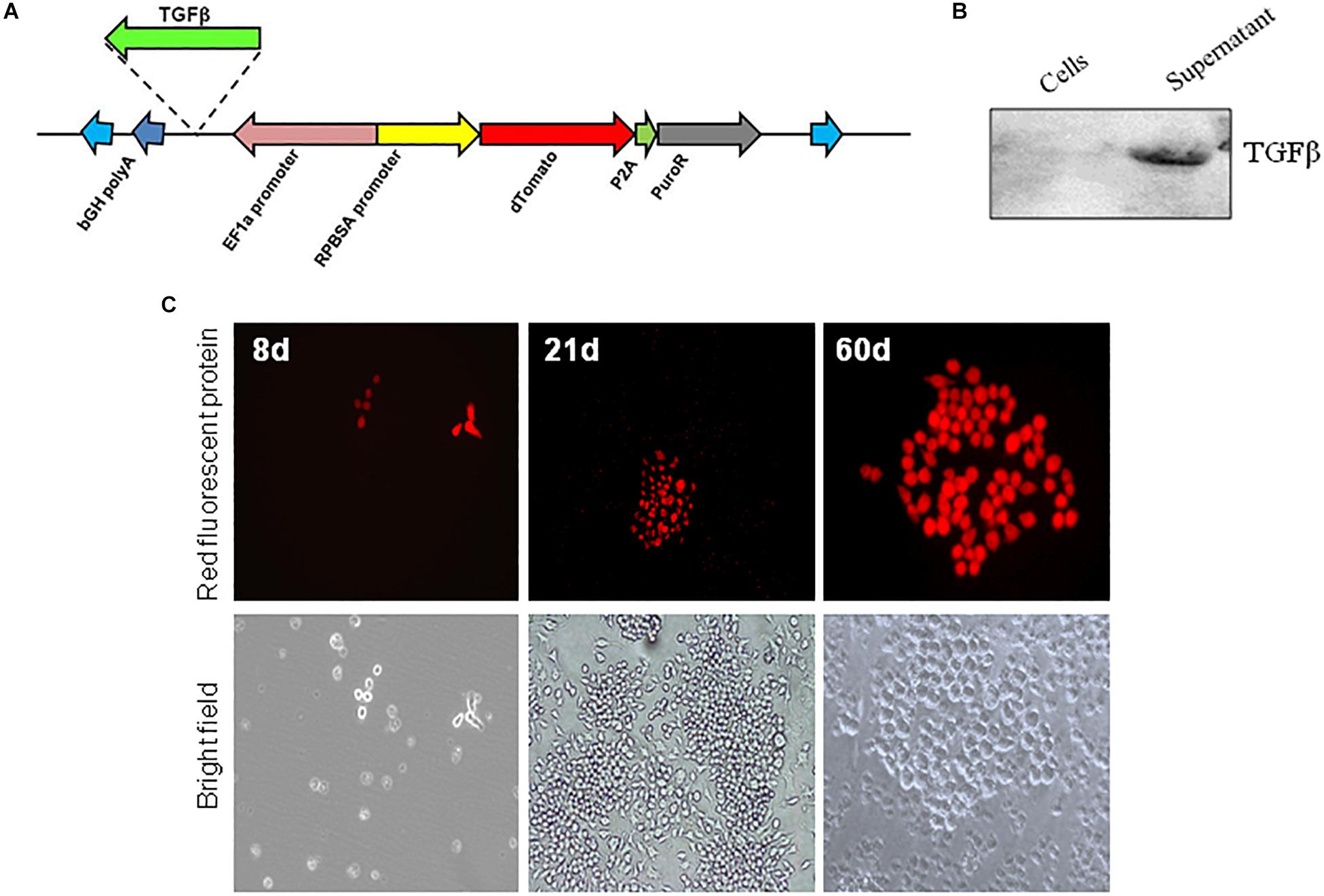
Figure 1. In vitro expression of Sleeping Beauty transposon in RAW 264.7 macrophages. (A) Plasmid (pSBbi-RP-TGFβ) construct for transposon coding for Td-Tomato and a puromycin resistance gene. The location for insertion of the mouse TGFβ on the plasmid is shown. In vitro infection of RAW 264.7 macrophages with Salmonella-carrying both the Sleeping Beauty Transposon plasmid pSBbi-RP or pSBbi-RP-TGFβ and transposase plasmid pCMV (CAT) T7 SB-100. (B) Western blot of cell lysate and supernatant performed 60 days after bacterial infection of macrophages using a DDK tag for TGFβ. (C) Expression of red fluorescent protein at 8, 21, and 60 days post-infection. Fluorescent images were acquired on a ZEISS inverted LSM700 microscope at 20x.
In vitro Infection
The murine RAW264.7 macrophage cell line was obtained from the American Type Culture Collection (ATCC no.-TIB-71). The cells were plated in 24-well plates (5 × 104 cells per well) and were allowed to attach overnight at 37°C in an atmosphere of 5% CO2. Overnight cultures of Salmonella containing pCMV(CAT)T7-SB100 and pSBbi-RP or pSBbi-RP-TGFβ were diluted and added to the cells growing in 24 well tissue culture plates at a multiplicity of infection of about 20. The bacteria were centrifuged onto the cells at 500 × g for 5 min. The plates were placed in an incubator for 25 min at 37°C for uptake of bacteria. Wells were washed three times with PBS and then incubated for 1 h in media containing 100 μg/ml of gentamicin to kill extracellular bacteria. Subsequent growth media were supplemented with 10 μg/ml of gentamicin for the remainder of the experiment. Given that pSBbi-RP confers resistance to puromycin, RAW 264.7 macrophages that incorporated the transposon were positively selected by the addition of puromycin (2 μg/ml). Cultures were treated with Salmonella-expressing both pSBbi-RP or pSBbi-RP-TGFβ and pCMV(CAT)T7-SB100 and cultured from 7 to 60 days for expression analysis. A ZEISS inverted LSM700 microscope was employed to obtain images that were processed with ZEN-lite digital imaging software (CarlZeiss, Oberkochen, Germany) for processing. The expression of TGFβ by macrophages was analyzed in cell lysate or culture supernatant by Western blot analysis.
Animal Experiments
Seven-week-old female NOD/ShiLtJ mice (Jackson Laboratory, Bar Harbor, ME, United States) were housed in pathogen-free conditions. City of Hope has been fully accredited by the Association for Assessment and Accreditation of Laboratory Animal Care International (AAALAC). The study was approved by the Institutional Animal Care and Use Committee of City of Hope (IACUC# 11032).
Eight-week-old NOD mice were vaccinated on days 0 and 7 by oral gavage with 107CFU/mouse in a total volume of 200 μl of 5% sodium bicarbonate as described (10, 23, 45). Furthermore, vaccinated animals were treated with hamster anti-CD3 mAb for five consecutive days (days 0–4; 2.5 μg i.p./mouse).
Flow Cytometry
Single cell suspensions of spleen, pancreatic lymph nodes (PLN), mesenteric lymph nodes (MLN), and Peyer’s patches (PP) were digested in collagenase D (1 mg/ml) at week 4 post-vaccination. Cells were stained directly with the following conjugated antibodies: MHC-II FITC anti-mouse I-Ak (Aβk), APC anti-mouse CD11c, Brilliant Violet 605 anti-mouse CD103, PE anti-mouse CD80, Brilliant Violet 711 anti-mouse CD86 and matching isotype controls. Cells were also stained with anti-mouse CD11b PE/Cy7, BV650 anti-mouse/human CD45R/B220, BV510 anti- mouse Ly-6G/Ly-6C (Gr-1) and matching isotype controls. Signal height and width were used to exclude doublets. Dead cells were excluded using Fixable Live/Dead Yellow Stain (Invitrogen), according to the manufacturer’s protocol. All antibodies and isotypes were obtained from Biolegend (San Diego, United States). A BD Fortessa flow cytometer (BD Biosciences) and FlowJo software gated on isotype controlled single DAPI-negative cell populations was used for FACS.
In vitro Proliferation Assay and Cytokine Assay
Responder T cells (Tresp) were generated and isolated from spleens of NOD mice (3 mice) immunized with insulin peptide B9-23 (100 μg) and emulsified in complete Freund adjuvant (CFA). After 11 days CD4+CD25– Tresp cells were isolated from pooled splenocytes using negative selection for CD4+ followed by negative selection for CD25+ using a CD4+CD25+ regulatory T Cell Isolation Kit (Miltenyi Biotec, 130-091-041) and then labeled with 1:1000 of 5 mM Cell Trace Violet (CTV) (48). CD4+CD25+ Treg cells were isolated from pooled splenocytes of non-vaccinated NOD mice (5 mice) using negative selection for CD4+ followed by positive selection for CD25+ using a CD4+CD25+ regulatory T Cell Isolation Kit (Miltenyi Biotec, 130-091-041). Dendritic cells (DCs) were isolated from pooled splenocytes (5 mice) isolated from either vaccinated or non-vaccinated mice using PE-conjugated CD11c antibody (Miltenyi, 130-110-701) followed by anti-PE microbeads (Miltenyi Biotec, 130-091-041). The Tresp cells (1 × 105 cells/well) were co-cultured in 96-well round bottom plates with or without 1 × 105 Treg cells isolated from non-vaccinated mice using different ratios of DCs isolated from either vaccinated or non-vaccinated mice in the presence of insulin peptide B9-23 (10 μg/ml). After 3 days, the proliferation of CD4 responder T cells positive for CTV and with surface expression of CD44 and CD69 (signifying cell activation) was analyzed by gating on proliferated cells using FlowJo software 10.4.
Cell free supernatants were collected for detection of IL10, TGFβ, IFNγ, and TNFα using Mouse Quantikine ELISA Kits (R&D Systems) according to the manufacturer’s instructions.
Gene Expression
RNA isolated from sorted CD11c+ and CD3+CD4+ cells obtained from splenocytes, PLN, MLN, and PP were isolated using the DirectZol kit (Zymo Research). Between 3 and 5 × 105 cells were sorted with 80 to 90% viability. RNA concentration was measured with a Nanodrop 2000 (Thermo Scientific). A 260/280 ratio of 1.8 to 2.00 was considered for RNA purity. Total RNA (500 ng) was used for cDNA synthesis using the qScript cDNA SuperMix kit (Quantabio). qPCR analysis was performed using TaqMan gene expression assays (Applied Biosystem) according to the manufacturer’s protocol. qRT-PCR was done with ABI Fast 7500 software (Applied Biosystems, ABI). Primers and probes were purchased from Applied Biosystems: CD274 (Mm03048248_m1), and CTLA4 (Mm00486849_m1). Relative changes in mRNA were calculated using the ΔΔCt method. Ct values of target genes were calculated relative to a reference control gene (TATA box binding protein, Tbp (Mm01277042_m1) using the following formula ΔCt = CtTarget-CtReference. Expression level = 2–Δ Ct.
Statistical Analyses
Two-way ANOVA were used for analysis of the percentage of positive cells between groups and to compare cell populations after FACS analysis. A p < 0.05 was considered significant. Statistical analysis was performed using GraphPad Prism 8 software.
Results
In vitro Expression of Sleeping Beauty
RAW 264.7 macrophages were infected in vitro with S. typhimurium carrying both Sleeping Beauty Td-Tomato transposon plasmid (pSBbi-RP) and the transposase plasmid pCMV(CAT)T7-SB100 (SB100) (Figure 1A). The expression of TGFβ was detected in supernatant only after infection of macrophages with pSBbi-RP-TGFβ and pCMV(CAT)T7-SB100 (Figure 1B), implying that TGFβ was secreted after expression by host cells under the control of the CMV promoter.
Additionally the expression of RFP after infection of RAW macrophages was detected. RAW macrophages infected with the pSBbi-RP transposon, but not the pCMV(CAT)T7-SB100 transposase plasmid, were negatively selected by the addition of 2 μg/ml of puromycin. pCMV(CAT)T7-SB100 which codes for transposase conferred long-term resistance to puromycin in RAW macrophages as well as permanent expression of RFP. The presence of red colonies was seen consistently at 7–8 days post-infection (Figure 1C). The expression of the RFP appeared to be permanent as the fluorescent colonies grown for more than 60 days post-infection continued to show signal (Figure 1C).
In vivo Labeling of Antigen-Presenting Cells
Although Salmonella-mediated labeling of RAW cells occurred in vitro, it was not known whether this cell labeling would occur in vivo. Mice were administered a single dose of Salmonella-containing both transposon (pSBbi-RP) and transposase (SB100) plasmids. Four weeks later, flow cytometry demonstrated red fluorescent cells in the PP, MLN, PLN, and spleen of inoculated NOD mice. Characterization showed that the majority of red-labeled cells were CD11c+ indicating that they were indeed DCs (Figure 2A). However, a minority of red-labeled cells were found to be other than DCs (Figure 2B). Further characterization of red-labeled DCs showed that they were MHCII+ APCs (Figure 2C). Overall, red-labeled MHCII+CD11c+ DCs comprised 5 to 10% of total live cells in the secondary lymphoid tissues (Figure 2C).
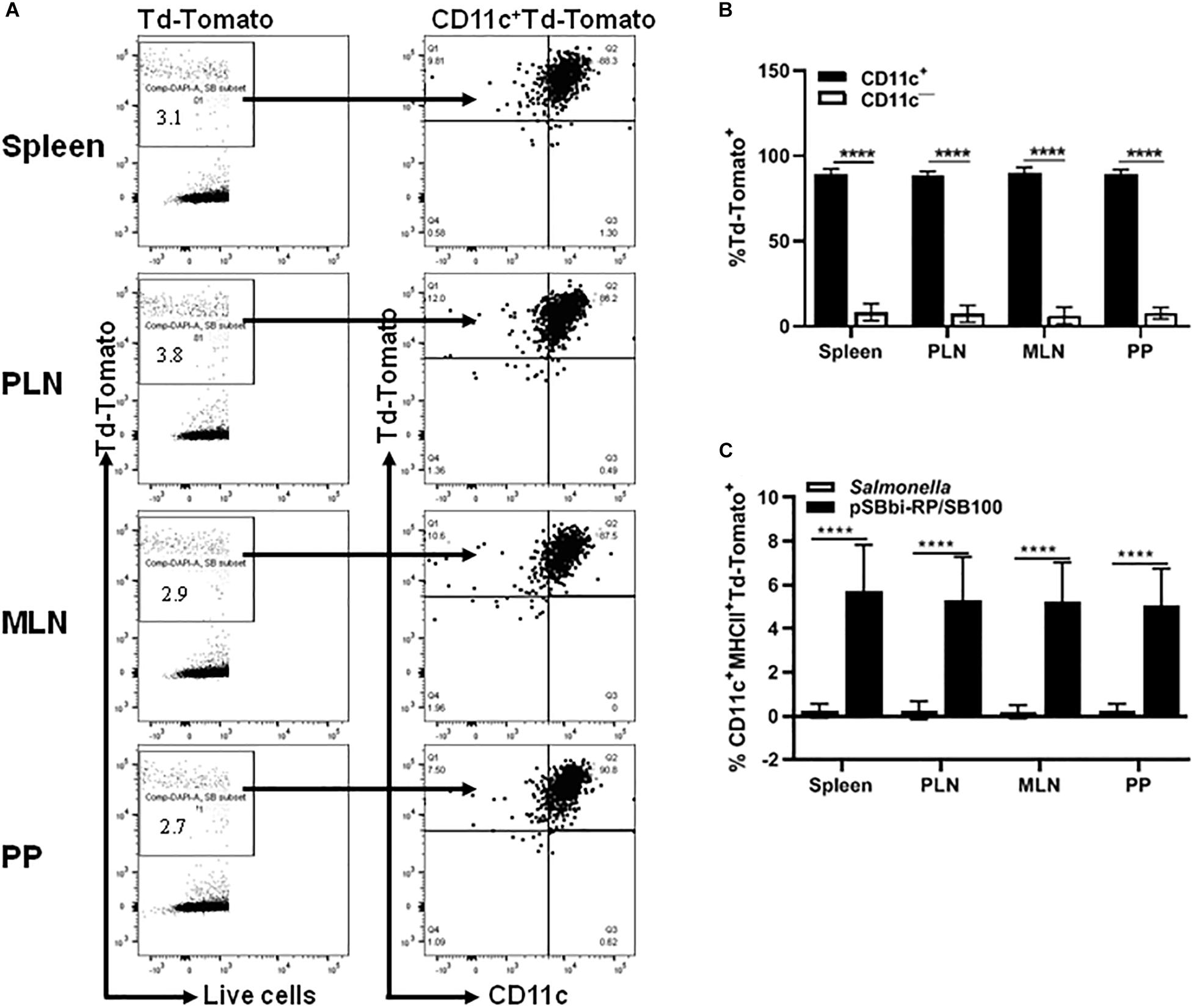
Figure 2. In vivo detection of the red fluorescent protein in different organs of vaccinated NOD mice. NOD mice were given a single oral dose of Salmonella-containing plasmid (pSBbi-RP/SB100, n = 10) compared to Salmonella only (n = 10). Four weeks post-vaccination, red fluorescent protein was detected in different organs. (A) Representative FACS plots gated the frequency of live red-labeled CD11c+ and CD11c– cells in the spleens, pancreatic lymph nodes (PLN), mesenteric lymph nodes (MLN), and Peyer’s patches (PP) of mice from each group. (B) Quantification of the percentages of the CD11c+ and CD11c– red-labeled cells in all organs. (C) Quantification of the percentages of red-labeled CD11c+MHCII+ in total live cells isolated from the spleen, PLN, MLN, and PP. The data shown are as the average with standard deviation (SD) obtained from two independent experiments. Statistical analysis using two-way ANOVA shows significance between groups (****p < 0.0001).
TGFβ Induces Higher Levels of DCs
An important component of the T1D Salmonella-based vaccine is the combination of diabetic autoantigens with TGFβ. TGFβ is used as an immunomodulator and is expressed by the host cells under the CMV promoter. To investigate the effect of TGFβ on the red-labeled cell populations, the mouse tgfβ1-coding region was inserted into the pSBbi-RP transposon plasmid upstream of the EF1a promoter to create pSBbi-RP-TGFβ (Figure 1A) that express both TFGβ and RFP. Mice were given the Salmonella-containing both pSBbi-RP-TGFβ and transposase (SB100) orally and compared with animals receiving the Salmonella-containing the pSBbi-RP/SB100 plasmids. Incorporation of the pSBbi-RP-TGFβ transposon into the host cell genome conferred both red fluorescent labeling and host cell secretion of mouse TGFβ. Four weeks after administration of Salmonella, labeled cells were found to be red fluorescent MHCII+CD11c+ DCs which subsequently migrated to the spleen, MLN, PLN, and PP (Figures 3A,B). However, in this situation the presence of TGFβ appeared to induce a significant increase in the number of red fluorescent MHCII+CD11c+ DCs in the PP and MLN (two-way ANOVA, p < 0.001, p < 0.0001, Figure 3B) compared to mice given Salmonella lacking the mouse tgfβ1-coding region.
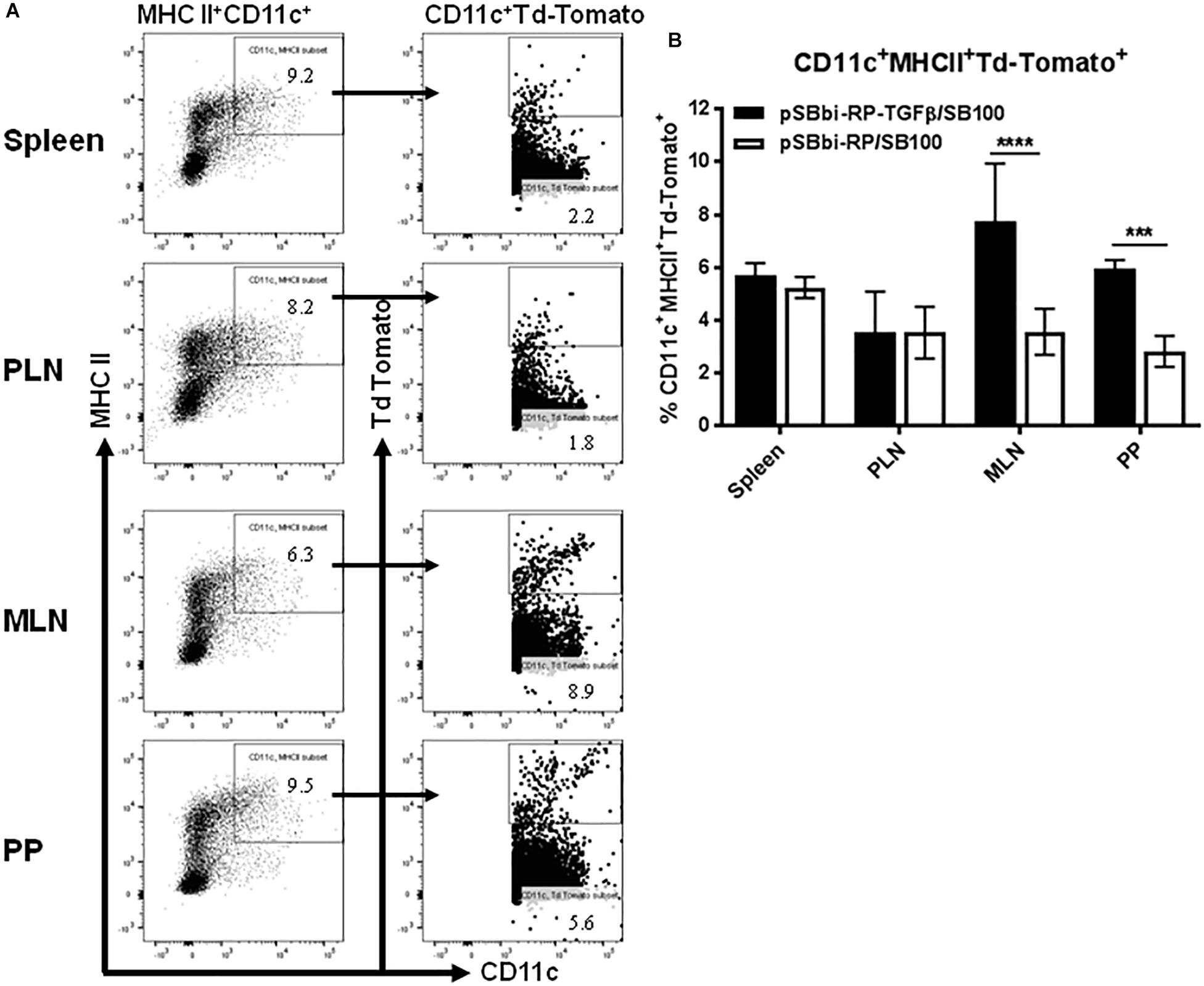
Figure 3. Vaccine-delivered TGFβ alters levels of DCs in secondary lymphoid tissues. NOD mice were given a single oral dose of Salmonella (pSBbi-RP-TGFβ/SB100, n = 10) or Salmonella (pSBbi-RP/SB100, n = 10). Four weeks post-vaccination, red fluorescent protein was detected in DCs of different organs. (A) Representative FACS plots gated the frequency of MHCII+CD11c+Td-Tomato+ cells in the spleens, PLN, MLN, and PP of mice from each group. (B) Quantification of the percentages of MHCII+CD11c+Td-Tomato+ cells in the spleen, PLN, MLN, and PP. The data shown are the average with SD from two independent experiments. Statistical analysis using two-way ANOVA shows significance between Salmonella (pSBbi-RP-TGFβ/SB100) and Salmonella (pSBbi-RP/SB100) groups (***p < 0.001, ****p < 0 0001).
TGFβ-Induces Gut CD103+ DCs
The MHCII+CD11c+CD103+ populations in mice administered Salmonella-containing pSBbi-RP-TGFβ/SB100 plasmids with and without TGFβ were assessed 4 weeks after inoculation (Figure 4A). Interestingly, very few red fluorescent DCs from mice given vaccine lacking TGFβ were CD103+. However, mice given vaccine with TGFβ showed a significant increase in PP and MLN of red-labeled DCs that were also CD103+ (two-way ANOVA, p < 0.0001, Figure 4B).
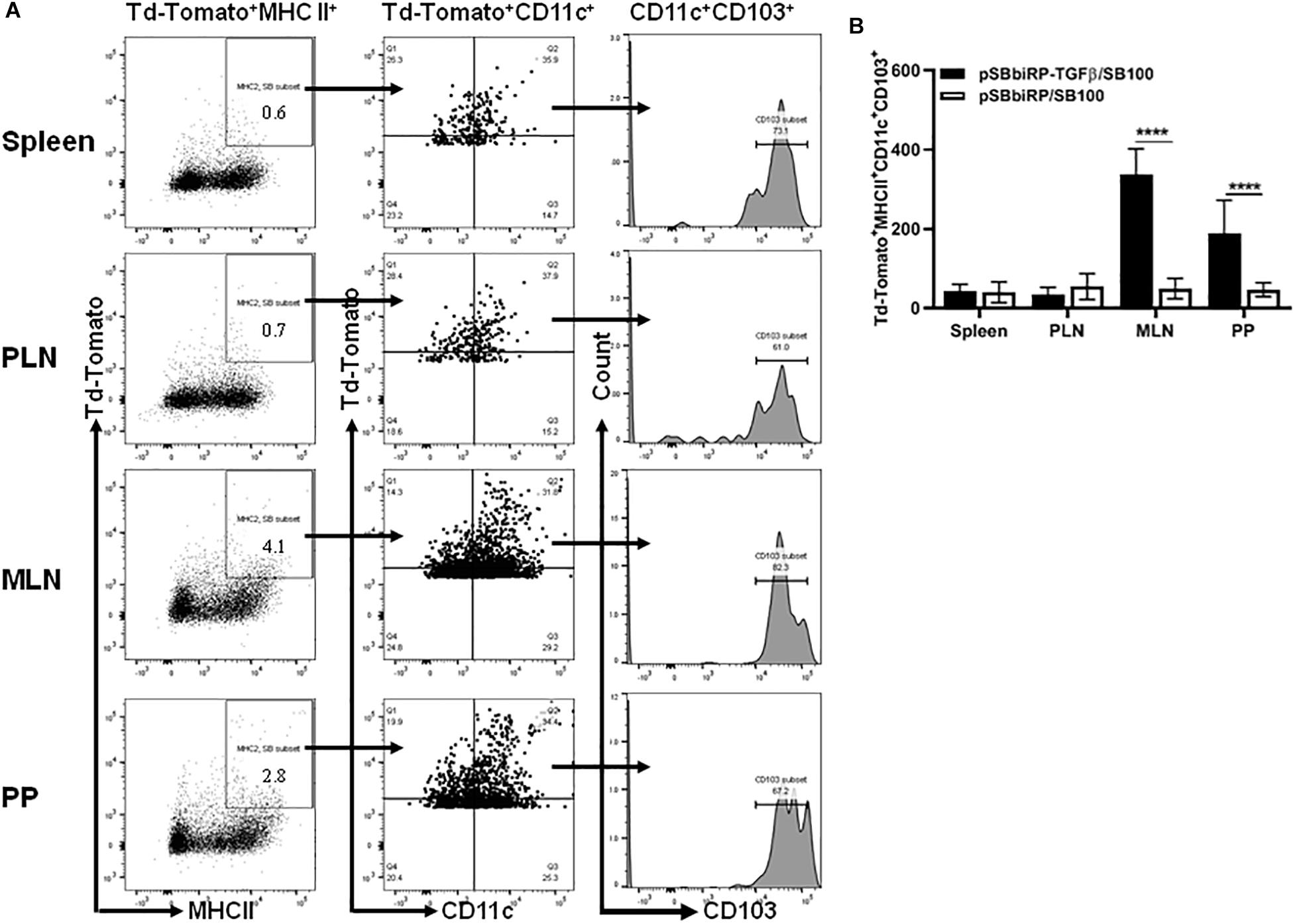
Figure 4. Vaccine-delivered TGFβ alters levels of gut DCs. NOD mice were given a single oral dose of Salmonella (pSBbi-RP-TGFβ/SB100, n = 10) or Salmonella (pSBbi-RP/SB100, n = 10). Four weeks post-vaccination, red fluorescent protein was detected in DCs of different organs. (A) Representative FACS plots gated the frequency of the live red-labeled MHCII+CD11c+CD103+ cells in the spleens, PLN, MLN, and PP of mice from each group. (B) Quantification of the numbers of MHCII+CD11c+CD103+ in red-labeled cells in the spleen, PLN, MLN, and PP. The data shown are the average with SD from two independent experiments. Statistical analysis using two-way ANOVA shows significance between Salmonella (pSBbi-RP-TGFβ/SB100) and Salmonella (pSBbi-RP/SB100) groups (****p < 0.0001).
Salmonella-Delivered TGFβ Increases PDL-1 and CTLA-4 Expression Levels in Secondary Lymphoid Tissues
Cells from animals given Salmonella-containing pSBbi-RP-TGFβ/SB100 plasmids with and without TGFβ were compared. The CD11c+ DCs and CD4+ T-cells from splenocytes, MLN, PLN and PP were sorted for qRT-PCR analysis. Programmed death-ligand-1 (PDL-1or CD274) expression was significantly increased in DCs in PLN, MLN, and PP but not the spleen of NOD mice treated with TGFβ-containing plasmid (two-way ANOVA, p = 0.001, p = 0.0003, p > 0.0001) compared with the plasmid without TGFβ (Figure 5A). Furthermore the percentage of DCs expressing PDL-1 measured by flow cytometry was significantly elevated in MLN and PP but not spleen and PLN of mice treated with TGFβ-containing plasmid (two-way ANOVA, p < 0.0001, p = 0.002) compared with the plasmid without TGFβ (Figure 5B). Interestingly, cytotoxic T-lymphocyte-associated protein-4 (CTLA-4) expression was significantly increased in CD4+ T-cells in MLN of mice treated with TGFβ-containing plasmid (two-way ANOVA, p < 0.0001) compared with the plasmid without TGFβ (Figure 5C). FACS also showed that CTLA-4 is significantly elevated on the surface of CD4+ T cells in the MLN mice treated with TGFβ-containing plasmid (two-way ANOVA, p < 0.0001) compared with the plasmid without TGFβ (Figure 5D).
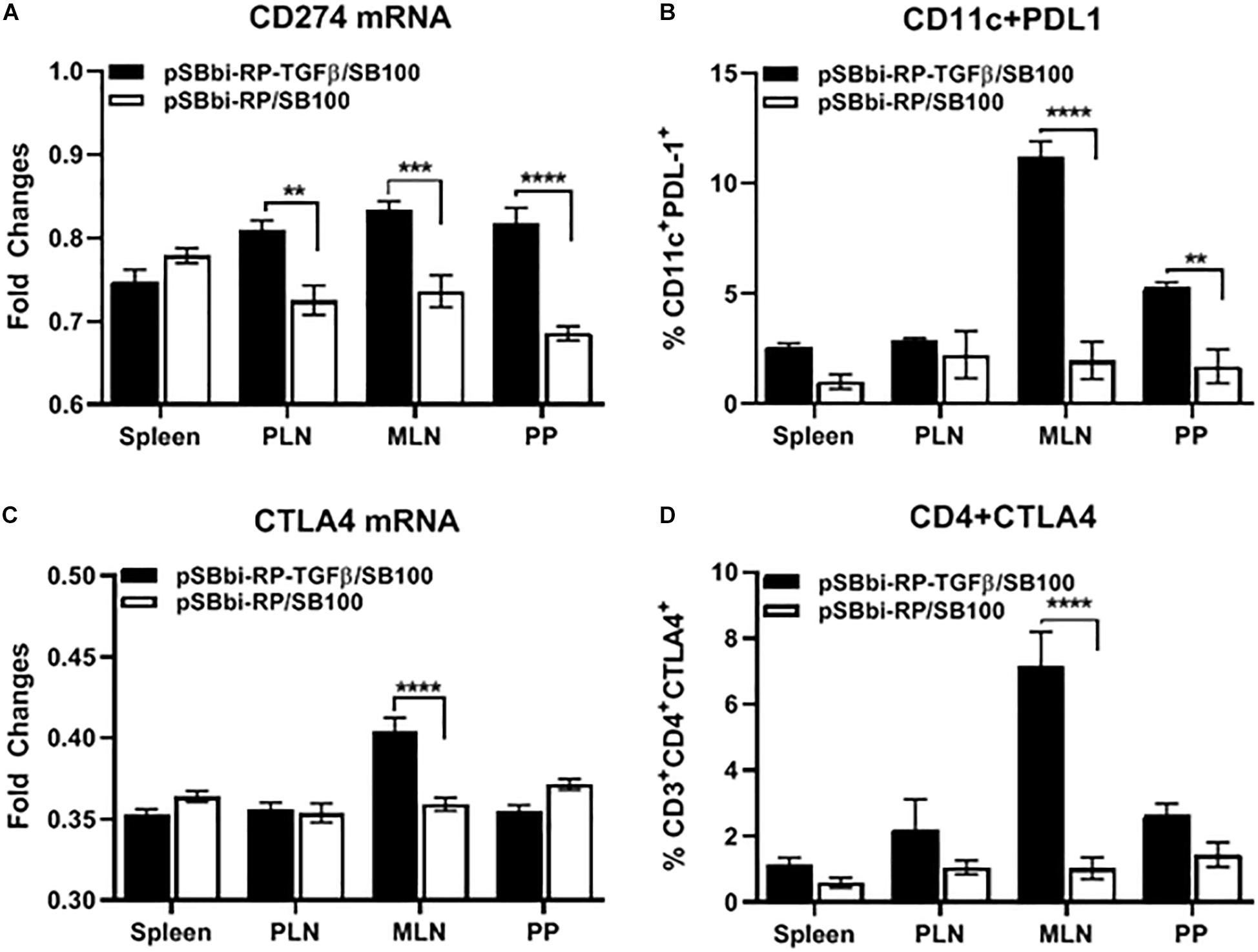
Figure 5. Endogenous TGFβ alters PDL-1 and CTLA4 expression in secondary lymphoid tissues. NOD mice were given a single oral dose of Salmonella (pSBbi-RP-TGFβ/SB100) or Salmonella (pSBbi-RP/SB100). Four weeks post-vaccination, the fold changes in gene expression of (A) CD274 (PDL-1) in sorted CD11c cells and (C) CTLA4 in sorted CD4 cells isolated from specified organs was determined. Percentage of CD11c+ cells expressing PDL-1 (B) or CD4+ cells expressing CTLA4 (D) were quantified in the spleen, PLN, MLN, and PP. The data shown are the average with SD from two independent experiments. Statistical analysis using two-way ANOVA shows significance between Salmonella (pSBbi-RP-TGFβ/SB100) and Salmonella (pSBbi-RP/SB100) groups (**p < 0.01, ***p < 0.001, ****p < 0 0001).
A Salmonella-Based Vaccine Increases the DCs in Lymphoid Organs
Pre-diabetic NOD mice were vaccinated with Salmonella-based vaccine (PPI + TGFβ + IL10 + anti-CD3) to quantify the percentage of DCs in the spleen, PLN, MLN, and PP. Figure 6A shows the frequency of CD11c+ DCs was 5 to 10% of the total number of live cells in all detected lymphoid organs. The percentage of DCs was significantly increased in all organs isolated from vaccine-treated mice compared to vehicle (two-way ANOVA, p < 0.01, p < 0.05). Additionally, we could not detect any differences between vaccine or vehicle treated mice in the percentage of cell populations that were characterized as B220+GR-1– (B cells, Supplementary Figure S1A) and CD11b+GR-1+ as polymorph nuclear macrophages (PMN, Supplementary Figure S1B) in the spleens, PLN, MLN, and PP.
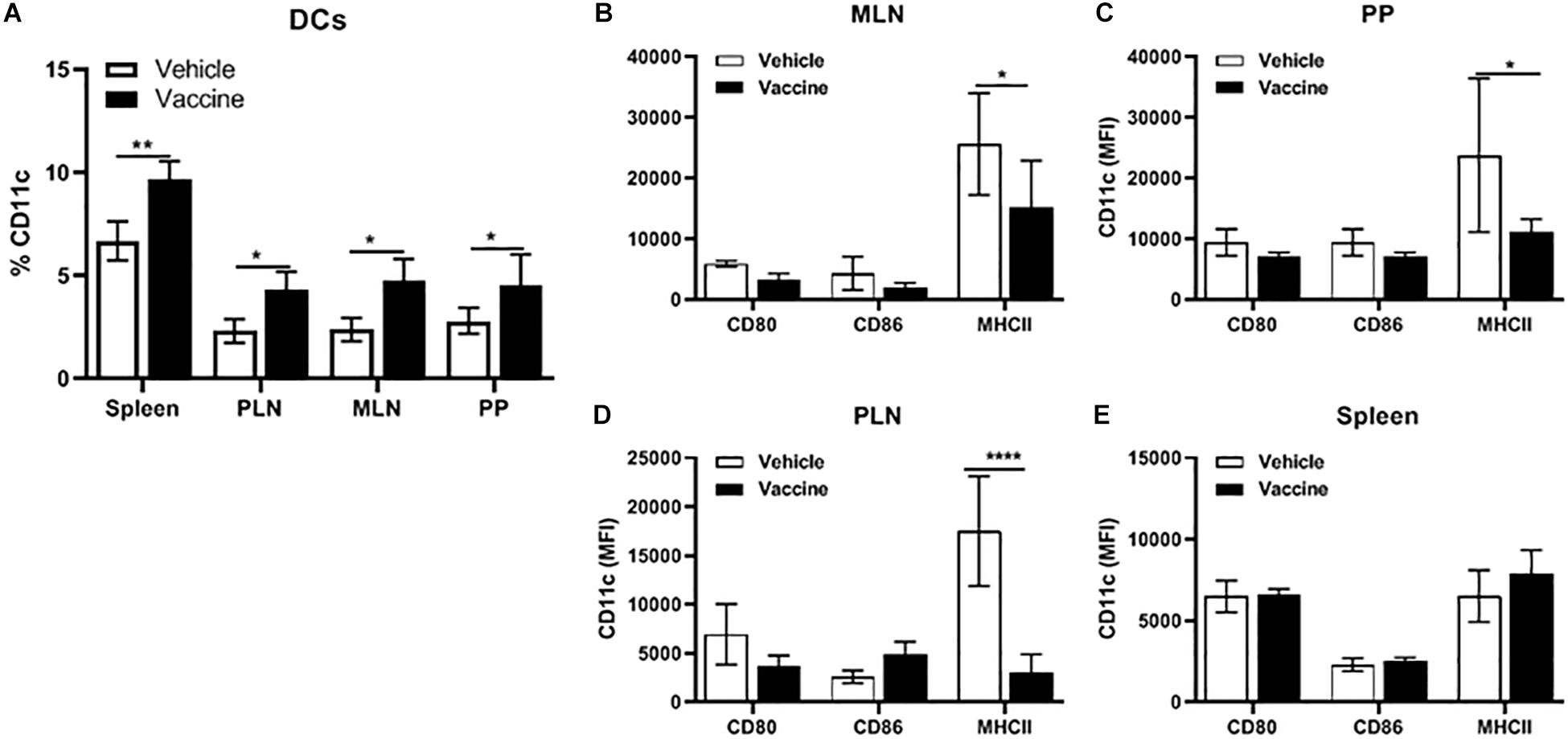
Figure 6. A Salmonella-based vaccine increase DCs in lymphoid organs. NOD mice were injected with 2.5 μg/mouse of anti-CD3 mAb for 5 days followed by 2 doses of oral vaccine containing PPI + TGFβ + IL10 (n = 5). Four weeks post-vaccination, DCs were analyzed in different organs by FACS analysis. (A) Quantification of the percentage of CD11c+ in total live cells of the spleen, PLN, MLN, and PP. (B) MLN, (C) PP, (D) PLN, and (E) spleen expression of CD80, CD86, and MHCII was measured in DCs from each organ. The data shown are the average with SD from two independent experiments. Statistical analysis using two-way ANOVA shows significance between vaccine and vehicle treated groups (*p < 0.05, **p < 0.01, ****p < 0.0001).
Moreover, CD11c+ DCs from vaccinated mice showed lowered expression of the MHCII molecule and co-stimulatory molecules CD80, and CD86 in cells from MLN (Figure 6B), PP (Figure 6C) and PLN (Figure 6D) compared to DCs from these lymphoid organs from vehicle-treated mice. In contrast, CD11c+ DCs from the spleen from vaccinated mice (Figure 6E) showed no difference in the level of expression of MHCII, CD80 and CD86 compared to DCs from vehicle-treated mice.
Importance of DCs for Salmonella-Based Vaccine Therapy
We next evaluated whether the DCs in vaccine-treated NOD mice were tolerogenic. As responder T cells, we used CD4+CD25– T cells isolated from spleens of normoglycemic B9-23 vaccinated NOD mice. We found that CD4+CD25+ Treg cells from vehicle-treated mice efficiently suppressed proliferation and activation of responder T cells when combined with equal amount of DCs from vaccinated mice but did not alter proliferation when cultured with DCs from vehicle-treated animals (Figure 7A). As well, these tolerogenic DCs lowered expression of activation markers CD44 (Figure 7B) and CD69 (Figure 7C). Additionally DCs from vaccine-treated mice, when combined with Tregs, efficiently increased regulatory cytokines such as IL10 (Figure 7D) and TGFβ (Figure 7E) and decreased the inflammatory cytokines such as IFNγ (Figure 7F) and TNFα (Figure 7G). In contrast DCs from vehicle-treated mice, when combined with Tregs decreased the regulatory cytokines IL10 (Figure 7D) and TGFβ (Figure 7E) and increased the inflammatory cytokines IFNγ (Figure 7F) and TNFα (Figure 7G). Finally, when we decreased the number of DCs to a ratio of 1:8, the suppressor activity of Tregs decreased indicating that the DCs from vaccinated animals were important to the suppressor activity of the Tregs.
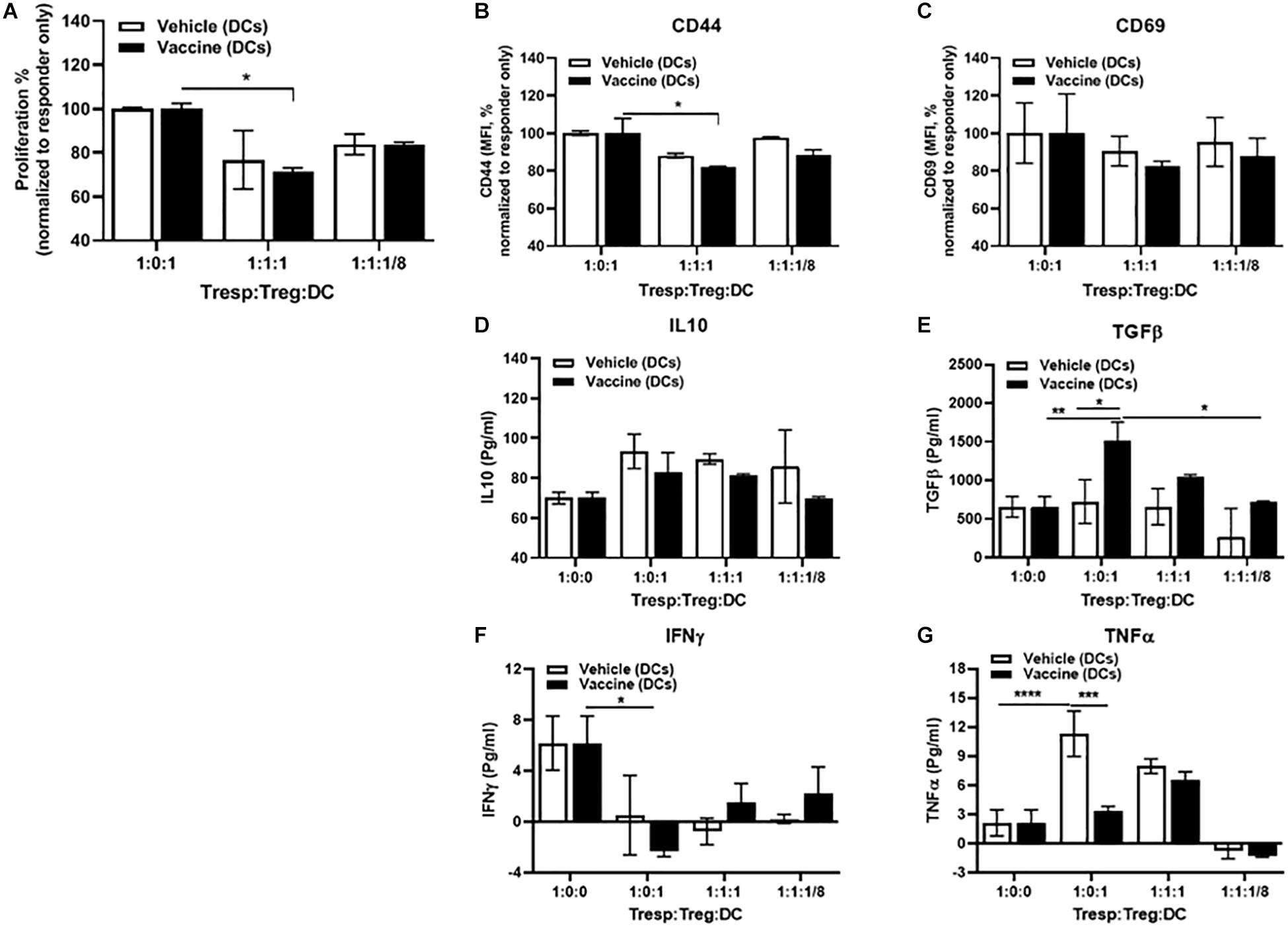
Figure 7. DCs mediated in vitro Salmonella-based anti-inflammatory effects. Female 8-week-old NOD mice were treated with 2.5 μg/mouse of anti-CD3 mAb via i.p. injection for 5 days followed by 2 doses of oral combined vaccines. Splenocytes were harvested from the indicated group at day 30 post-vaccination. CD4+CD25– Tresp cells isolated from splenocytes of B9-23 vaccinated mice were dye-labeled, co-cultured with CD4+CD25+ Treg cells isolated from non-vaccinated mice, and DCs from vaccinated or non-vaccinated mice for 3 days with insulin peptide B9-23 (10 μg/ml). (A) Proliferation of Tresp was measured by flow cytometry of dye dilution and shown as the percentage of Tresp normalized to Tresp only culture. (B) Activation of Tresps, shown as MFI of CD44 expression or (C) CD69 normalized to Tresp-only culture. ELISA for regulatory (D) IL-10, and (E) TGFβ. ELISA for inflammatory (F) IFNγ, and (G) TNFα. The data shown are the average with SD from two independent experiments. Statistical analysis was performed using two-way ANOVA (*p < 0.05, **p < 0.01, ***p < 0.001, ****p < 0.0001).
Discussion
Recently we developed an oral vaccine using live attenuated Salmonella that prevented and reverted the onset T1D in NOD mice (45). This vaccine was a combination of PPI + TGFβ + IL10 and anti-CD3 mAb. We showed that the vaccine increased Tregs and Tr1 cells in vaccinated mice (23, 45). To understand how Salmonella prevented diabetes, it was important to first identify the cell populations that interact with the microorganism and to investigate the role of Salmonella-delivered TGFβ in creation of a tolerogenic micro-environment in NOD mice. On oral administration, Salmonella gains access to the individual via DCs that phagocytosis the bacteria, through SPI1-T3SS-mediated invasion of other cells or by GALT cells. Indeed, these several mechanisms likely contribute to wider spread of the bacteria beyond the gut. In specialized lymphatic organs such as PP and MLN, the bacteria is mostly intracellular, its survival and replication dependent on the function of SPI2-T3SS (49).
Transposable elements are naturally occurring vehicles and are used for transgenesis and mutagenesis. The Sleeping Beauty transposon shows efficient transposition in a wide range of vertebrate cells, including humans (50, 51, 52). The current study employed attenuated Salmonella as a vehicle to deliver the transposon plasmid expressing a detectable fluorescent protein to host cells and allowed tracking of Salmonella-bearing host cells throughout the GALT. Secondary lymphoid organs provide the specialized microenvironment necessary for generating an immune-specific response. DCs, while present in low numbers, are distributed widely and beyond lymphatic tissues. Because DCs work very efficiently at controlling T cells, they are critical to turning on or off immune responses (53). We found that the DCs labeled after oral administration of Salmonella were present in all lymphoid tissues examined, consistent with results from others.
Previous studies have shown that tolerance to oral antigens is mediated by CD103+ DCs in the lamina propria (39, 41, 54–56). CD103+ DCs are unique to the gut. Transforming growth factor receptor 1 (TGFβR1) has a cell-intrinsic role in the development of these cells. Together, these published data suggested that MHCII+CD11c+CD103+ cells mediate the regulatory effects of the Salmonella-based vaccine. In keeping with this, TGFβ promoted the upregulation of DC cell surface CD103 in animals administered the vaccine. In the intestinal tract, CD103+ DCs carry acquired antigens to the local lymph nodes where they encounter CD8+ T cells (57). By promoting naïve T cell transformation to regulatory Foxp3+ T cells, DCs promote immune suppression (39, 54, 56). Our results demonstrate that Salmonella-delivered TGFβ recruits CD103+ DCs in the MLN and PP. These data suggest that Salmonella-autoantigen driven education of the naïve T-cells into iTregs or Tr1 occurs specifically in these secondary lymph nodes (45). Our data further indicate that TGFβ is involved in the trafficking and control of CD103+ DCs, concentrating their function within MLN and PP.
Additionally, it appears that TGFβ has an impact on PDL-1 and CTLA-4 expression in NOD mice. PD-L1 suppresses the immune system in cancer and autoimmune disease (58). Endogenous ligand-receptor pathways, such as the PD-L1/PD-1, limit T cell antigen activation and promote regulatory T cell survival (58, 59). Similarly, CTLA-4 limits T cell activation in part by blunting CD28 co-activation (60). Indeed, some data suggests that variation in the CTLA-4 gene may promote autoimmunity (61–64). Our data suggest that TGFβ increases PDL-1 and CTLA-4 at the lymphoid sites where CD103+ DCs are found to be elevated consistent with a tolerogenic microenvironment. As some time differentiation of naïve T cells into nTregs or Tr1 occurs in the MLN and the PP (31, 34, 54, 56, 65) and these lymphoid sites appear to be the central hub for Salmonella vaccine activity.
Some studies characterized semi-mature phenotype DCs that are thought to be most effective in inducing tolerance in autoimmune diseases (66, 67). In this study, our combined therapy increased DCs in all lymphatic tissues of vaccinated mice. Additionally, these DCs expressed low levels of co-stimulatory molecules. Moreover, in vitro proliferation assays conformed that the DCs from vaccinated mice were essential for suppressor activity of Tregs by decreasing proliferation and activation of effector T cells. Furthermore, these DCs secreted less inflammatory cytokines such as TNFα, and IFNγ while secreting increased tolerogenic cytokines such as IL10 and TGFβ.
Together, the data herein suggest the oral vaccine could be an effective immunotherapy to reestablish tolerance to self-antigens and of value to individuals with T1D. The vaccine may also find use in the treatment of other autoimmune disorders. Using Salmonella offers several advantages over other methods of drug delivery. First, oral delivery exploits the natural tolerogenic functions of the GALT system. Second, oral delivery is a patient-friendly treatment approach. Third, inclusion of TGFβ and possibly other tolerogenic cytokines should improve the efficacy of the vaccine. Fourth, protection of the antigen from degradation while it traverses the digestion system is important. Lastly for clinical relevance, a vaccine for typhoid fever that uses the Salmonella typhi Ty21a strain is approved by the FDA.
Data Availability Statement
The datasets generated for this study are available on request to the corresponding author.
Ethics Statement
The animal care facility at City of Hope has been fully accredited by the Association for Assessment and Accreditation of Laboratory Animal Care International (AAALAC). The study was approved by the Institutional Animal Care and Use Committee (IACUC# 11032).
Author Contributions
JM, KF, and MH conceived and designed the experiments. JM, AA, JR, PG, NG, and MH performed the experiments. KF and MH analyzed the data. JM, FK, and MH wrote the manuscript.
Funding
This work was supported by the Wanek Family Project to Cure Type 1 Diabetes Program Award (Duarte, CA, United States), and the Juvenile Diabetes Research Foundation (United States) (JDRF2-SRA-2016-314-S-B). AA was a Fulbright Visiting Scholar awarded from the Global Scholar Program.
Conflict of Interest
The authors declare that the research was conducted in the absence of any commercial or financial relationships that could be construed as a potential conflict of interest.
Acknowledgments
We extend our gratitude to Dr. Eric Kowarz and Dr. Zsuzsanna Izsvak for their kind donations of the Sleeping Beauty transposon system plasmids. We thank Jacob Cobb, City of Hope, for his technical assistance and acknowledge the expert assistance provided by the Analytical Cytometry Core facility at the Beckman Research Institute of City of Hope.
Supplementary Material
The Supplementary Material for this article can be found online at: https://www.frontiersin.org/articles/10.3389/fimmu.2020.00712/full#supplementary-material
References
1. Atkinson MA. The pathogenesis and natural history of type 1 diabetes. Cold Spring Harb Perspect Med. (2012) 2:a007641 doi: 10.1101/cshperspect.a007641
2. Nakayama M, Beilke JN, Jasinski JM, Kobayashi M, Miao D, Li M, Coulombe MG. Priming and effector dependence on insulin B:9–23 peptide in NOD islet autoimmunity. J Clin Invest. (2007) 117:1835–43.
3. Achenbach P, Koczwara K, Knopff A, Naserke H, Ziegler AG, Bonifacio E. Mature high–affinity immune responses to (pro)insulin anticipate the autoimmune cascade that leads to type 1 diabetes. J Clin Investigat. (2004) 114:589–97.
4. Kronenberg D, Knight RR, Estorninho M, Ellis RJ, Kester MG, De Ru A, Eichmann M. Circulating preproinsulin signal peptide–specific CD8 T cells restricted by the susceptibility molecule HLA–A24 are expanded at onset of type 1 diabetes and kill beta–cells. Diabetes. (2012) 61:1752–59.
5. Skowera A, Ellis RJ, Varela–Calvino R, Arif S, Huang GC, Van–Krinks C, Zaremba A, Rackham C. CTLs are targeted to kill beta cells in patients with type 1 diabetes through recognition of a glucose–regulated preproinsulin epitope (vol 118, pg 3390, 2008). J Clin Investigat. (2009) 119:2843–43.
6. Cheminay C, Hensel M. Rational design of Salmonella recombinant vaccines. Int J Med Microbiol. (2008) 298:87–98. doi: 10.1016/j.ijmm.2007.08.006
7. Cheminay C, Mohlenbrink A, Hensel M. Intracellular Salmonella inhibit antigen presentation by dendritic cells. J Immunol. (2005) 174:2892–99.
8. Raine T, Zaccone P, Mastroeni P, Cooke A. Salmonella typhimurium infection in nonobese diabetic mice generates immunomodulatory dendritic cells able to prevent type 1 diabetes. J Immunol. (2006) 177:2224–33.
9. Ashkar AA, Reid S, Verdu EF, Zhang K, Coombes BK. Interleukin–15 and NK1.1+ cells provide innate protection against acute Salmonella enterica serovar Typhimurium infection in the gut and in systemic tissues. Infect Immun. (2009) 77:214–22.
10. Husseiny MI, Rawson J, Kaye A, Nair I, Todorov I, Hensel M, Kandeel F, Ferreri K. An oral vaccine for type 1 diabetes based on live attenuated Salmonella. Vaccine. (2014) 32:2300–7.
11. Xiong G, Husseiny MI, Song L, Erdreich–Epstein A, Shackleford GM, Seeger RC, Jackel D, Hensel M, Metelitsa LS. Novel cancer vaccine based on genes of Salmonella pathogenicity island 2. Int J Cancer. (2010) 126:2622–34.
12. Husseiny MI, Hensel M. Evaluation of an intracellular–activated promoter for the generation of live Salmonella recombinant vaccines. Vaccine. (2005) 23:2580–90.
13. Xu X, Husseiny MI, Goldwich A, Hensel M. Efficacy of intracellular activated promoters for generation of Salmonella–based vaccines. Infect Immun. (2010) 78:4828–38.
14. Hansen–Wester I, Hensel M. Salmonella pathogenicity islands encoding type III secretion systems. Microbes Infect. (2001) 3:549–59.
15. Galan JE. Salmonella interactions with host cells: type III secretion at work. Annu Rev Cell Dev Biol. (2001) 17:53–86.
16. Figueira R, Holden DW. Functions of the Salmonella pathogenicity island 2 (SPI–2) type III secretion system effectors. Microbiol SGM. (2012) 158:1147–61.
17. Haga T, Kumabe S, Ikejiri A, Shimizu Y, Li H, Goto Y, Matsui H, Miyata H, Miura T. In vitro and in vivo stability of plasmids in attenuated Salmonella enterica serovar typhimurium used as a carrier of DNA vaccine is associated with its replication origin. Exp Anim Tokyo. (2006) 55:405–9.
18. Laniewski P, Jagusztyn–Krynicka EK. Subunit vaccine construction using Salmonella enterica cells as a carrier of heterologous genes. Postep Mikrobiol. (2013) 52:281–94.
19. Abrahams GL, Hensel M. Manipulating cellular transport and immune responses: dynamic interactions between intracellular Salmonella enterica and its host cells. Cell Microbiol. (2006) 8:728–37.
20. Holzer SU, Hensel M. Divergent roles of Salmonella pathogenicity island 2 and metabolic traits during interaction of S. enterica serovar typhimurium with host cells. PLoS One. (2012) 7:e33220. doi: 10.1371/journal.pone.0033220
21. Chen H, Schifferli DM. Construction, characterization, and immunogenicity of an attenuated Salmonella enterica serovar typhimurium pgtE vaccine expressing fimbriae with integrated viral epitopes from the spiC promoter. Infect Immun. (2003) 71:4664–73.
22. Kong W, Brovold M, Koeneman BA, Clark–Curtiss J, Curtiss R. Turning self–destructing Salmonella into a universal DNA vaccine delivery platform. P Natl Acad Sci USA. (2012) 109:19414–19.
23. Husseiny MI, Du W, Mbongue J, Lenz A, Rawson J, Kandeel F, Ferreri K. Factors affecting Salmonella–based combination immunotherapy for prevention of type 1 diabetes in non–obese diabetic mice. Vaccine. (2018) 36:8008–18
24. Hu Q, Coburn B, Deng W, Li Y, Shi X, Lan Q, Wang B, Coombes BK, Finlay BB. Salmonella enterica serovar Senftenberg human clinical isolates lacking SPI–1. J Clin Microbiol. (2008) 46:1330–36. doi: 10.3389/fmicb.2014.00781
25. Chistiakov DA, Bobryshev YV, Kozarov E, Sobenin IA, Orekhov AN. Intestinal mucosal tolerance and impact of gut microbiota to mucosal tolerance. Front Microbiol. (2014) 5:781.
26. Pabst O, Bernhardt G, Forster R. The impact of cell–bound antigen transport on mucosal tolerance induction. J Leuk Biol. (2007) 82:795–800. doi: 10.1189/jlb.0307144
27. Hill M, Cuturi MC. Negative vaccination by tolerogenic dendritic cells in organ transplantation. Curr Opin Organ Tran. (2010) 15:738–43.
28. Raker VK, Domogalla MP, Steinbrink K. Tolerogenic dendritic cells for regulatory T cell induction in man. Front Immunol. (2015) 6:569. doi: 10.3389/fimmu.2015.00569
29. Gregori S, Tomasoni D, Pacciani V, Scirpoli M, Battaglia M, Magnani CF, Hauben E, Roncarolo MG. Differentiation of type 1 T regulatory cells (Tr1) by tolerogenic DC–10 requires the IL–10–dependent ILT4/HLA–G pathway (vol 116, pg 935, 2010). Blood. (2011) 118:5060–60.
30. Wakkach A, Fournier N, Brun V, Breittmayer JP, Cottrez F, Groux H. Characterization of dendritic cells that induce tolerance and T regulatory 1 cell differentiation in vivo. Immunity. (2003) 18:605–17.
31. Brockmann L, Gagliani N, Steglich B, Giannou AD, Kempski J, Pelczar P, Geffken M. IL–10 receptor signaling is essential for T(R)1 cell function in vivo. J Immunol. (2017) 198:1130–41.
32. Roncarolo MG, Gregori S, Bacchetta R, Battaglia M. Tr1 cells and the counter–regulation of immunity: natural mechanisms and therapeutic applications. Int Health Dis. (2014) 380:39–68. doi: 10.1007/978-3-662-43492-5_3
33. Saito H, Tsurikisawa N, Tsuburai T, Oshikata C, Akiyama K. The proportion of regulatory T cells in the peripheral blood reflects the relapse or remission status of patients with Churg–Strauss syndrome. Int Arch Allerg Immunol. (2011) 155(Suppl. 1):46–52. doi: 10.1159/000327265
34. Yu H, Gagliani N, Ishigame H, Huber S, Zhu S, Esplugues E, Herold KC, Wen L, Flavell RA. Intestinal type 1 regulatory T cells migrate to periphery to suppress diabetogenic T cells and prevent diabetes development. Proc Natl Acad Sci USA. (2017) 114:10443–8.
35. Sakaguchi S, Yamaguchi T, Nomura T, Ono M. Regulatory T cells and immune tolerance. Cell (2008) 133:775–87.
36. Beitnes AC, Raki M, Lundin KE, Jahnsen J, Sollid LM, Jahnsen FL. Density of CD163+ CD11c+ dendritic cells increases and CD103+ dendritic cells decreases in the coeliac lesion. Scand J Immunol. (2011) 74:186–94.
37. Annacker O, Coombes JL, Malmstrom V, Uhlig HH, Bourne T, Johansson–Lindbom B, Agace WW. Essential role for CD103 in the T cell–mediated regulation of experimental colitis. J Exp Med. (2005) 202:1051–61.
38. Iliev ID, Mileti E, Matteoli G, Chieppa M, Rescigno M. Intestinal epithelial cells promote colitis–protective regulatory T–cell differentiation through dendritic cell conditioning. Mucosal Immunol. (2009) 2:340–50.
39. Schulz O, Jaensson E, Persson EK, Liu XS, Worbs T, Agace WW, Pabst O. Intestinal CD103(+), but not CX3CR1(+), antigen sampling cells migrate in lymph and serve classical dendritic cell functions. J Exp Med. (2009) 206:3101–14.
40. Yrlid U, Cerovic V, Milling S, Jenkins CD, Klavinskis LS, MacPherson CG. A distinct subset of intestinal dendritic cells responds selectively to oral TLR7/8 stimulation. Eur J Immunol. (2006) 36:2639–48.
41. Milling S, Yrlid U, Cerovic V, MacPherson G. Subsets of migrating intestinal dendritic cells. Immunol Rev. (2010) 234:259–67.
42. Kowarz E, Loscher D, Marschalek R. Optimized sleeping beauty transposons rapidly generate stable transgenic cell lines. Biotechnol J. (2015) 10:647–53.
44. Plasterk RH. The ins and outs of transposition. Molecular mechanisms of transposition and its control, sponsored by the European molecular biology organization, roscoff, France, June 24–28, 1990. New Biol. (1990) 2: 787–92.
45. Mbongue JC, Rawson J, Garcia PA, Gonzalez N, Cobb J, Kandeel F, Ferreri K, Husseiny MI. Reversal of new onset type 1 diabetes by oral Salmonella–based combination therapy and mediated by regulatory T–cells in NOD mice. Front Immunol. (2019) 10:320.
46. Mates L, Chuah MK, Belay E, Jerchow B, Manoj N, Acosta–Sanchez A, Grzela DP, Schmitt A. Molecular evolution of a novel hyperactive sleeping beauty transposase enables robust stable gene transfer in vertebrates. Nat Genet. (2009) 41:753–61.
47. Husseiny MI, Wartha F, Hensel M. Recombinant vaccines based on translocated effector proteins of Salmonella pathogenicity Island 2. Vaccine. (2007) 25:185–93.
48. Takiishi T, Korf H, Van Belle TL, Robert S, Grieco FA, Caluwaerts S, Galleri L. Reversal of autoimmune diabetes by restoration of antigen–specific tolerance using genetically modified Lactococcus lactis in mice. J Clin Invest. (2012) 122:1717–25.
49. Jantsch J, Chikkaballi D, Hensel M. Cellular aspects of immunity to intracellular Salmonella enterica. Immunol Rev. (2011) 240:185–95.
50. Grabundzija I, Wang J, Sebe A, Erdei Z, Kajdi R, Devaraj A, Steinemann D. Sleeping Beauty transposon–based system for cellular reprogramming and targeted gene insertion in induced pluripotent stem cells. Nucleic Acids Res. (2013) 41:1829–47.
51. Sumiyoshi T, Holt NG, Hollis RP, Ge S, Cannon PM, Crooks GM, Kohn DB. Stable transgene expression in primitive human CD34+ hematopoietic stem/progenitor cells, using the Sleeping Beauty transposon system. Hum Gene Ther. (2009) 20:1607–26.
52. Singh H, Huls H, Kebriaei P, Cooper LG. A new approach to gene therapy using sleeping beauty to genetically modify clinical–grade T cells to target CD19. Immunol Rev. (2014) 257:181–90.
53. Banchereau J, Steinman RM. Dendritic cells and the control of immunity. Nature. (1998) 392:245–52.
54. Badami E, Sorini C, Coccia M, Usuelli V, Molteni L, Bolla AM, Scavini M, Mariani A, King C, Bosi E, Falcone M. Defective differentiation of regulatory FoxP3(+) T cells by small–intestinal dendritic cells in patients with type 1 diabetes. Diabetes. (2011) 60:2120–4.
55. Johansson–Lindbom B, Svensson M, Pabst O, Palmqvist C, Marquez G, Forster R, Agace WW. Functional specialization of gut CD103+ dendritic cells in the regulation of tissue–selective T cell homing. J Exp Med. (2005) 202: 1063–73.
56. Siddiqui KRR, Powrie F. CD103(+) GALT DCs promote Foxp3(+) regulatory T cells. Mucosal Immunol. (2008) 1:S34–8.
57. Farache J, Koren I, Milo I, Gurevich I, Kim KW, Zigmond E, Furtado GC, Lira SA, Shakhar G. Luminal bacteria recruit CD103(+) dendritic cells into the intestinal epithelium to sample bacterial antigens for presentation. Immunity. (2013) 38:581–95.
58. Dai S, Jia R, Zhang X, Fang Q, Huang L. The PD–1/PD–Ls pathway and autoimmune diseases. Cell Immunol. (2014) 290:72–9.
59. Chemnitz JM, Parry RV, Nichols KE, June CH, Riley JL. SHP–1 and SHP–2 associate with immunoreceptor tyrosine–based switch motif of programmed death 1 upon primary human T cell stimulation, but only receptor ligation prevents T cell activation. J Immunol. (2004) 173:945–54.
60. Devarajan P, Chen ZB. Autoimmune effector memory T cells: the bad and the good. Immunol Res. (2013) 57:12–2.
61. Klocke K, Sakaguchi S, Holmdahl R, Wing K. Induction of autoimmune disease by deletion of CTLA–4 in mice in adulthood. Eur J Immunol. (2016) 46:770.
62. Salomon B, Bluestone JA. Complexities of CD28/B7: CTLA–4 costimulatory pathways in autoimmunity and transplantation. Annu Rev Immunol. (2001) 19:225–52.
63. Fife BT, Bluestone JA. Control of peripheral T–cell tolerance and autoimmunity via the CTLA–4 and PD–1 pathways. Immunol Rev. (2008) 224:166–82. doi: 10.1111/j.1600-065X.2008.00662.x
64. Romo–Tena J, Gomez–Martin D, Alcocer–Varela J. CTLA–4 and autoimmunity: New insights into the dual regulator of tolerance. Autoimmun Rev. (2013) 12:1171–6. doi: 10.1016/j.autrev.2013.07.002
65. Geem D, Ngo V, Harusato A, Chassaing B, Gewirtz AT, Newberry RD, Denning TL. Contribution of mesenteric lymph nodes and GALT to the intestinal Foxp3+ regulatory T–cell compartment. Cell Mol Gastroenterol Hepatol. (2016) 2:274–80.
66. Menges M, Rossner S, Voigtlander C, Schindler H, Kukutsch NA, Bogdan C, Erb K, Schuler G, Lutz MB. Repetitive injections of dendritic cells matured with tumor necrosis factor alpha induce antigen–specific protection of mice from autoimmunity. J Exp Med. (2002) 195:15–21.
Keywords: Salmonella-based vaccine, sleeping beauty, transposons, Type 1 Diabetes, CD103+DC, TGFβ, tolerogenic dendritic cells
Citation: Mbongue JC, Alhoshani A, Rawson J, Garcia PA, Gonzalez N, Ferreri K, Kandeel F and Husseiny MI (2020) Tracking of an Oral Salmonella-Based Vaccine for Type 1 Diabetes in Non-obese Diabetic Mice. Front. Immunol. 11:712. doi: 10.3389/fimmu.2020.00712
Received: 09 January 2020; Accepted: 30 March 2020;
Published: 28 April 2020.
Edited by:
Pedro A. Reche, Complutense University of Madrid, SpainReviewed by:
Marta Vives-Pi, Germans Trias i Pujol Health Science Research Institute (IGTP), SpainSofia A. Casares, Naval Medical Research Center, United States
Copyright © 2020 Mbongue, Alhoshani, Rawson, Garcia, Gonzalez, Ferreri, Kandeel and Husseiny. This is an open-access article distributed under the terms of the Creative Commons Attribution License (CC BY). The use, distribution or reproduction in other forums is permitted, provided the original author(s) and the copyright owner(s) are credited and that the original publication in this journal is cited, in accordance with accepted academic practice. No use, distribution or reproduction is permitted which does not comply with these terms.
*Correspondence: Mohamed I. Husseiny, bWVsc2F5ZWRAY29oLm9yZw==