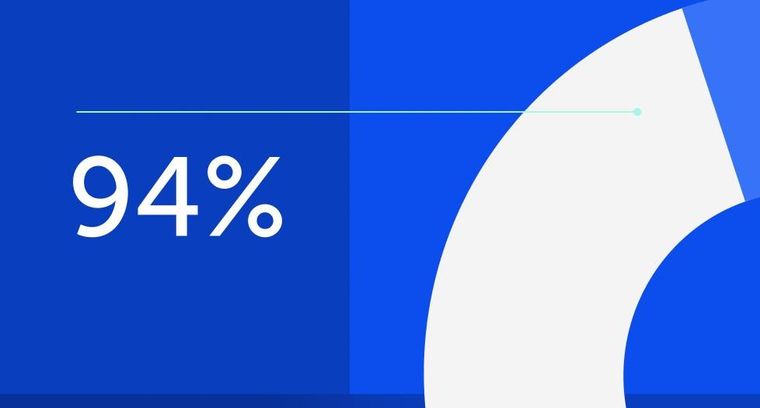
94% of researchers rate our articles as excellent or good
Learn more about the work of our research integrity team to safeguard the quality of each article we publish.
Find out more
MINI REVIEW article
Front. Immunol., 23 April 2020
Sec. Nutritional Immunology
Volume 11 - 2020 | https://doi.org/10.3389/fimmu.2020.00700
This article is part of the Research TopicImpact of Early Life Nutrition on Immune System Development and Related Health Outcomes in Later LifeView all 18 articles
Allergic diseases, such as food allergy (FA), atopic dermatitis (AD), and asthma, are heterogeneous inflammatory immune-mediated disorders that currently constitute a public health issue in many developed countries worldwide. The significant increase in the prevalence of allergic diseases reported over the last few years has closely paralleled substantial environmental changes both on a macro and micro scale, which have led to reduced microbial exposure in early life and perturbation of the human microbiome composition. Increasing evidence shows that early life interactions between the human microbiome and the immune cells play a pivotal role in the development of the immune system. Therefore, the process of early colonization by a “healthy” microbiome is emerging as a key determinant of life-long health. In stark contrast, the perturbation of such a process, which results in changes in the host-microbiome biodiversity and metabolic activities, has been associated with greater susceptibility to immune-mediated disorders later in life, including allergic diseases. Here, we outline recent findings on the potential contribution of the microbiome in the gastrointestinal tract, skin, and airways to the development of FA, AD, and asthma. Furthermore, we address how the modulation of the microbiome composition in these different body districts could be a potential strategy for the prevention and treatment of allergic diseases.
Over the last few decades, many developed and fast-growing countries worldwide have registered a dramatic increase in the prevalence of allergic diseases, such as asthma, AD, and FA, which currently pose a substantial burden to healthcare systems (1, 2). Thus far, the genetic and environmental drivers of the rapid rise in allergy prevalence remain to be more fully elucidated.
Notably, the evolution of the allergy epidemic has closely paralleled radical environmental and lifestyle changes, such as progressive industrialization and urbanization, widespread sanitation programs and antibiotics use, physical inactivity and highly processed diets. All these changes have led to reduced microbial exposure in early life and loss of microbial biodiversity (3).
Accumulating evidence points to a central role of the human microbiome perturbation in the rising prevalence of allergic diseases. The human microbiome comprises bacteria, viruses, fungi, protozoans, and archaea, which colonize primarily the gastrointestinal tract, but also the airways and the skin surface from the first days of life and gradually develop and diversify concomitantly with the physiological growth of the individual. The resident microbial communities in the human gut and other organs have been shown to modulate both the innate and acquired immune responses. Recent data show that several environmental drivers can affect the microbiome colonization, composition and metabolic activity in infancy, and alter the host functions for nutrition and immunity (4). Indeed, the process of early colonization by a “healthy” microbiome is emerging as a key determinant of life-long health, whereas the perturbation of such a process, has been associated with greater susceptibility to immune-mediated disorders later in life, including allergic diseases (5).
The recent introduction of the next-generation sequencing and genomic analysis to identify different microbial species has led to a greater knowledge of the complex role of the human microbiome in the pathogenesis of FA, AD, and asthma. Here, we review recent findings on the potential role of the human microbiome in the gastrointestinal tract, the skin, and the airways to the development of allergic diseases, and we address how the modulation of the microbiome composition could be a potential therapeutic or even preventive strategy for such disorders.
It is well established that microbiome composition changes dynamically in the first few years of life and can be influenced by several prenatal and postnatal environmental and host-related factors (Figure 1) (6). Among these factors, mounting evidence shows that some perinatal factors, such as mode of delivery, breastfeeding, early antibiotic use, and timing and type of complementary feeding, can significantly modulate the gut microbiome composition, which is emerging as a key determinant in developing immune tolerance responses to different antigens (7). The gut microbiome of newborns delivered by cesarean section shows a lower level of commensal bacteria typically found in those born vaginally and high concentrations of opportunistic pathogens typically found in the hospital environment, such as Enterococcus, Enterobacter, and Klebsiella species (8). These differences largely even by the time babies are weaned around 6 to 9 months, except for commensal bacteria Bacteroides, which remain absent or at very low levels in most cesarean section infants. Of note, the effect of the cesarean section on the infant microbiome seems to be related to maternal antibiotic exposure before the delivery (8).
Figure 1. Factors shaping the human microbiome development. The neonatal microbiome is a delicate and highly dynamic ecosystem that undergoes rapid changes in composition in the first few years of life determined by several pre and perinatal factors. The maturation of the gut microbiota toward an adult-like structure largely occurs by the age of 2 or 3 years. Therefore, early infancy could be a critical period for modulating the microbiota to promote healthy growth and development.
Breast milk contributes to the development of healthy gut microbiome. BM contains essential micronutrients and prebiotic compounds, which support the colonization and growth of commensal bacteria, and several immune active factors, oligosaccharides and microbes, which could all modulate host immune responses (9). Term infants born vaginally and breastfed exclusively seem to have the most “beneficial” gut microbiome, with the highest concentration of Bifidobacteria and lowest numbers of Clostridium difficile and Escherichia coli (10).
Shifting from exclusively breastfeeding to complementary feeding at weaning increases the prevalence of Bacteroides, Bilophila, Roseburia, Clostridium, and Anaerostipes, and progressively leads to the establishment of an adult-type microbiome (11). In particular, the introduction of solid foods modulates gut microbiome shifting from Bifidobacterium-dominant to Bacteroidetes- and Firmicutes-dominant species, such as the Clostridium coccoides and Clostridium leptum groups (12). The introduction of solid foods also induces a sustained increase in fecal SCFA levels and expression of genes associated with the adult microbiome’s core metabolic functions, such as polysaccharide breakdown, vitamin biosynthesis, and xenobiotic degradation (13).
The first 1000 days of life (i.e., the period from conception to age 2 years) seem to represent the critical window of opportunity for microbiome modulation (Figure 1) (6, 14). After this period, the gut microbiome tends to acquire an adult-like configuration with distinct microbial community composition and functions (15). However, several factors can induce significant perturbations to the gut microbiome composition later in life, such as long-term dietary changes, or frequent or prolonged use of antibiotics (13, 16). Notably, a very recent multi-omics integrative analysis showed that antibiotic use in adults induced alterations to the gut microbiome which adversely affected immunogenicity and responses to influenza vaccination (17).
Neonatal and infant gut microbiome appear to be involved in gut tolerance modulation and immune system “education” (18, 19). Germ-free animal experiments best describe this mutualistic relationship in animals (20–27). These data may support such a relationship in humans.
Indeed, some recent human studies have addressed the role of the gut microbiome on adaptive and innate immunity in the context of allergic diseases. Christmann et al. (28), reported lower IgG responses to specific clusters of microbiota antigens in infants who then developed allergic disorders in childhood (including skin, respiratory, and food allergies) compared to healthy children. West et al. (29), studied infants at high risk of atopic diseases and showed that depletion of Proteobacteria in early infancy is associated with increased Toll-like receptors (TLR)-4 induced innate inflammatory responses, whereas depletion of Ruminococcaceae is associated with increased TLR-2 induced innate inflammatory responses. Fujimura et al. (30), reported that infants at risk of asthma have a gut microbial signature with reduced abundance of certain bacteria taxa, such as Faecalibacterium and Bifidobacterium. Stimulation of adult PBMC with sterile fecal water from these infants then led to increases in CD4 + IL-4 producing cells and reduced regulatory Foxp3 cells. Similarly, Sjödin et al. (31), found that the gut symbiont Faecalibacterium correlated with the expression levels of regulatory cytokines in children with multiple allergies, suggesting an opportunity to expand such taxa to promote a regulatory tolerogenic immune response.
The composition of the microbiome varies across different body sites, which constitute unique habitats resulting in varied microbial communities within and between subjects. The greatest concentration and diversity of microorganisms are found in the gastrointestinal tract, which is dominated by facultative and strictly anaerobic bacteria of the phyla Firmicutes, Bacteroidetes, Actinobacteria, Verrucomicrobia, and Proteobacteria (32).
The mechanisms that mediate host-microbe communications are highly complex; a disrupted dialogue due to altered microbiome seems to negatively impact the immune homeostatic networks and may contribute to the development of hypersensitivity reactions to environmental allergens (33). This connection emerged over the last few decades with the proposed “hygiene hypothesis,” based on the epidemiological evidence that environmental drivers increasing early life microbial exposure (such as vaginal delivery, farming life, and furry animals exposure during childhood, large family size, unpasteurized milk consumption and absence of early antibiotic exposure) were associated with a lower risk of developing allergic disorders (34–38). Recent experimental and human investigations have strengthened the mechanistic substance to the hygiene hypothesis, providing evidence for the causal relationship between early life microbial perturbation in the gut, skin, and airways and the development of allergic diseases (Figure 2).
The composition and metabolic activities of the gut microbiome seem to be closely linked with the development of oral tolerance (39). Mortha et al. (40), showed that commensal microorganisms favor the crosstalk between innate myeloid and lymphoid cells that contributes to immune homeostasis in the gut and the development of oral tolerance to dietary antigens.
Infants with CMA have more total bacteria, in particular the anaerobic type, compared to healthy controls after 6 months of milk formula assumption. In addition, higher concentrations of Lactobacilli and lower concentrations of Enterobacteria and Bifidobacteria were observed in infants with CMA (41). Bunyavanich et al. showed that Clostridia and Firmicutes rates were particularly elevated in the gut microbiota of infants whose CMA resolved by 8 years of age (42). Fazlollahi et al. found that the gut microbiome of children with egg allergy had a greater abundance of the genera from Lachnospiraceae and Ruminococcaceae than those of healthy controls (43). A prospective study comprising 14 children with FA and 87 children with food allergens sensitization, showed that Haemophilus, Dialister, Dorea, and Clostridium genera were reduced in participants with food sensitization, whereas, the genera Citrobacter, Oscillospira, Lactococcus, and Dorea were under-represented in participants with FA (44). Furthermore, in subjects with peanut or tree nut allergy, decreased microbial richness and increased concentration of Bacteroides species were reported compared to non-allergic controls (45).
Studies in animal models showed that germ-free mice were protected from developing anaphylaxis to cow’s milk if colonized with gut microbiome from healthy infants, but not from infants with CMA (46). The transfer of specific bacterial strains, such as Bifidobacterium or Clostridium species to mice was shown to reducing the risk of food sensitization, by the induction of mucosal Treg (47). Clostridia can also stimulate innate lymphoid cells to produce IL-22, which contributes to strengthen the epithelial barrier and decrease the permeability of the gut to dietary proteins (48). Some functional effects of Clostridia in FA likely also occur through their fermentation metabolites, such as butyrate, a SCFA with known immunoregulatory and tolerogenic proprieties (49).
Experimental findings showing that the gut microbiome contributes to the development of food tolerance suggest that microbial modulation could be a potential therapeutic strategy for FA. Although the supplementation of an extensively hydrolyzed milk formula with Lactobacillus casei and Bifidobacterium lactis did not prove to accelerate the resolution of CMA (50), the administration of extensively hydrolyzed casein formula containing the probiotic Lactobacillus rhamnosus GG has been shown to promote CMA resolution at 12, 24, and 36 months, compared to non-supplemented hypoallergenic milk formula (51). Of note, the use of such Lactobacillus rhamnosus GG-supplemented formula significantly expanded butyrate-producing bacterial strains in the infant gut microbiome compared to non-supplemented formula (49). In another study, the use of an amino-acid based formula containing a specific synbiotics (i.e., a combination of prebiotic blend of fructo-oligosaccharides and the probiotic strain Bifidobacterium breve M-16V) has been shown to modulate the gut microbiome and its metabolic activities also in infants with non-IgE mediated CMA (52–54). Recently, an uncontrolled study suggested that oral supplementation with Lactobacillus rhamnosus GG could enhance the efficacy of oral immunotherapy in inducing peanut tolerance and immune changes in children with peanut allergy (55). However, further studies including a control group are required to determine whether modulation of the microbiome during immunotherapy will favor the acquisition of sustained unresponsiveness to food allergens.
Several factors, such as age, gender, ethnicity, climate, ultraviolet exposure, and lifestyle drivers, can influence the composition of skin microbiome (56). The healthy skin microbiome is represented by Propionibacterium species, which are mainly found in sebaceous sites, and Corynebacterium and Staphylococcus species, which are more abundant in moist microenvironments. Malassezia is the predominant fungal flora on human skin (56, 57).
Atopic dermatitis is a complex skin disease characterized by epidermal barrier dysfunction, altered innate/adaptive immune responses and impaired skin microbial biodiversity (58). Loss of microbial diversity, with the predominance of the Staphylococcus aureus over Staphylococcus epidermidis, is a characteristic feature at both acute and chronic skin sites of AD (59), which correlates with AD severity and the risk of allergic sensitization to common allergens (60). Staphylococcus aureus contributes to the epidermal barrier disruption through different pathways, including the downregulation of terminal differentiation of epidermal proteins, such as filaggrin and loricrin, and the promotion of the skin proteases activities, which directly damage the skin barrier (61, 62).
Coagulase-negative Staphylococci, which include S. epidermidis, S. hominis and S. lugdunensis, can secrete antimicrobial metabolites that limit S. aureus overgrowth and biofilm formation (61). In addition, S. epidermidis can also activate TLR2 signaling, which can induce the production of keratinocyte-derived antimicrobial peptides and increase the expession of epidermal tight junction proteins (63). Neonatal colonization of the skin by S. epidermidis is associated with the induction of specific Tregs that modulate local activation of host immune responses (64). Indeed, it has been recently shown that skin commensal Staphylococci species are significantly reduced at 2 months in infants who later developed AD at 1 year, suggesting that targeted topical modulation favoring early colonization with this genus might reduce the risk of later occurrence of AD (65). These findings, together with evidence that regular application of moisturizers repairs the skin barrier and restores commensal bacterial diversity (66–68), constituted the rationale for ongoing research on the application of topical probiotics, such as Vitreoscilla filiformis lysate and Roseomonas mucosa, as a potential strategy to modulate the skin microbiome and treat AD (69, 70). Preliminary data also showed that the autologous skin transplantation of antimicrobial coagulase-negative Staphylococci strains to human subjects with AD could decrease S. aureus overgrowth and colonization (71).
Changes in the gut microbiome seem also to contribute to the development of AD. Patients with AD have lower concentrations of Bifidobacterium in the gut microbiome than healthy controls, and these counts are inversely related to the severity of the disease (72). Early gut colonization with Clostridium difficile was related to the occurrence of AD (73), and lower Bacteroidetes diversity at 1 month was associated with AD at 2 years of age (74). There is evidence that pre- and post-natal supplementation with oral Lactobacillus and Bifidobacterium strains could reduce the risk of AD in infants due to changes in T cell-mediated responses (75). Finally, a recent large prospective study of gut microbiota showed that Lachnobacterium and Faecalibacterium were significantly less abundant in those children who developed AD by school-age compared to healthy controls. Notably, the differential abundance of these bacterial taxa was documented throughout infancy, which supports the likelihood of their protective role in the development of AD (76).
Accumulating evidence shows that the composition of the lung microbiome in early life can affect the development of respiratory health or disease (77, 78). Preclinical models support a protective role of bacteria against allergic airway inflammation (79, 80).
The phylum Bacteroides, particularly Prevotella spp., predominate in the lung microbiome of healthy subjects (81, 82). During the first 2 weeks of life, the lung microbiome promotes the transient expression of programmed death-ligand 1 (PDL1) in dendritic cells, which is necessary for the Treg-mediated attenuation of allergic airway responses (83). Epidemiological evidence shows that children who grow up in a farming environment and are exposed to diverse microbial communities since early life have a lower incidence of allergies (84). Notably, the airway colonization by Streptococcus, Moraxella, or Haemophilus within the first 2 months of life has been associated with the severity of lower respiratory viral infection in the first year of life, and the risk of asthma development later in life (85). The phylum Proteobacteria has also been associated with asthma and neutrophilic exacerbations, whereas Bacteroidetes with eosinophilic exacerbations, leading to the consideration that distinct mediators and microbiome profiles may represent different clusters of biological exacerbations (86, 87).
Emerging evidence shows that gut microbial perturbations in early life can also influence the development of allergic airway inflammation. Antibiotic use in neonatal mice favors variations in the microbiome composition, which have been associated with alterations in intestinal Tregs and increased susceptibility to airway hyper-responsiveness (88). Similarly, pre- and post-natal exposures to antibiotics in humans have been associated with an increased risk of developing asthma (89). In a recent longitudinal study, Galazzo et al. (76), showed that the bacterial genera Lachnobacterium, Lachnospira and Dialister were significantly decreased in the gut microbiome of infants who developed asthma by school-age compared to healthy controls. Analysis of the gut microbiome at 3 months of age within the Canadian Healthy Infant Longitudinal Development Study (CHILD) showed a reduction in bacterial taxa of the genera Lachnospira, Veillonella, Faecalibacterium, and Rothia among infants at risk of childhood asthma (90). In another recent observational cohort study, a reduction of Lachnospiraceae, Faecalibacterium, and Dialister at 1 year of age was associated with an increased risk of asthma at 5 years of age (91).
The protective effect of these bacterial taxa on asthma occurrence could be mediated by their fermentation products (92, 93). Faecalibacterium prausnitzii ferments dietary fiber to produce SCFAs, most notably butyric acid (93). Butyrate is the preferred energy source for colonocytes and has anti-inflammatory effects by inducing Tregs and promoting epithelial barrier permeability (94). SCFAs can contribute to the maturation process of dendritic cells in the bone marrow, leading to mature cells with a reduced ability to instigate Th2 responses in the lungs and to induce IgA production by mucosal B cells (94). High levels of gut microbial-derived butyrate in early life reduce the risk of allergen sensitization and asthma occurrence later in life, both in experimental and human studies (94, 95).
Finally, a recent systematic review of studies examining the effect of oral probiotic supplementation on asthma-related outcomes reported no significant differences in children receiving probiotics compared to the control groups regarding asthma control and lung function (96).
Early life is a crucial period for microbiome and immune development. The perturbation of the development and maturation of the microbiome during the first few years of life can have a variety of harmful effects on immune health, contributing to determining the development of atopic diseases. Although current understanding of the relationships between early life nutrition, microbiome, and immune system development has significantly increased in recent years, substantial knowledge gaps persist regarding the molecular mechanisms involved. Understanding these mechanisms is of the outermost importance to develop effective prevention strategies for allergic diseases.
DP, GN, IT, PC, and MD made substantial contributions to conception, design, and acquisition of data. GN, IT, PC, and MD drafted the initial manuscript. DP, PC, and MD critically reviewed it for important intellectual content. All authors gave the final approval of the version to be published.
The authors declare that the research was conducted in the absence of any commercial or financial relationships that could be construed as a potential conflict of interest.
AD, atopic dermatitis; BM, breast milk; CMA, cow’s milk allergy; FA, food allergy; SCFA, short-chain fatty acid; TLRs, Toll-like receptors; Treg, regulatory T cell.
1. Bach JF. The effect of infections on susceptibility to autoimmune and allergic diseases. N Engl J Med. (2002) 347:911–20.
2. Huang K, Yang T, Xu J, Yang L, Zhao J, Zhang X, et al. Prevalence, risk factors, and management of asthma in China: a national cross-sectional study. Lancet. (2019) 394:407–18. doi: 10.1016/S0140-6736(19)31147-X
3. Sbihi H, Boutin RC, Cutler C, Suen M, Finlay BB, Turvey SE. Thinking bigger: how early-life environmental exposures shape the gut microbiome and influence the development of asthma and allergic disease. Allergy. (2019) 74:2103–15. doi: 10.1111/all.13812
4. Gomez de Agüero M, Ganal-Vonarburg SC, Fuhrer T, Rupp S, Uchimura Y, Li H, et al. The maternal microbiota drives early postnatal innate immune development. Science. (2016) 351:1296–302.
5. Tamburini S, Shen N, Wu HC, Clemente JC. The microbiome in early life: implications for health outcomes. Nat Med. (2016) 22:713–22. doi: 10.1038/nm.4142
6. Grier A, McDavid A, Wang B, Qiu X, Java J, Bandyopadhyay S, et al. Neonatal gut and respiratory microbiota: coordinated development through time and space. Microbiome. (2018) 6:193. doi: 10.1186/s40168-018-0566-5
7. Nagpal R, Tsuji H, Takahashi T, Kawashima K, Nagata S, Nomoto K, et al. Sensitive quantitative analysis of the meconium bacterial microbiota in healthy term infants born vaginally or by cesarean section. Front Microbiol. (2016) 7:1997. doi: 10.3389/fmicb.2016.01997
8. Shao Y, Forster SC, Tsaliki E, Vervier K, Strang A, Simpson N, et al. Stunted microbiota and opportunistic pathogen colonization in caesarean-section birth. Nature. (2019) 574:117–21. doi: 10.1038/s41586-019-1560-1
9. Kumar H, du Toit E, Kulkarni A, Aakko J, Linderborg KM, Zhang Y, et al. Distinct patterns in human milk microbiota and fatty acid profiles across specific geographic locations. Front Microbiol. (2016) 7:1619.
10. Penders J, Thijs C, Vink C, Stelma FF, Snijders B, Kummeling I, et al. Factors influencing the composition of the intestinal microbiome in early infancy. Pediatrics. (2006) 118:511–21.
11. Koenig JE, Spor JA, Scalfone N, Fricker AD, Stombaugh J, Knight R, et al. Succession of microbial consortia in the developing infant gut microbiome. Proc Natl Acad Sci USA. (2011) 108:4578–85. doi: 10.1073/pnas.1000081107
12. Backhed F, Roswall J, Peng Y, Feng Q, Jia H, Kovatcheva-Datchary P, et al. Dynamics and stabilization of the human gut microbiome during the first year of life. Cell Host Microbe. (2015) 17:690–703. doi: 10.1016/j.chom.2015.04.004
13. Fallani M, Amarri S, Uusijarvi A, Adam R, Khanna S, Aguilera M, et al. Determinants of the human infant intestinal microbiome after the introduction of first complementary foods in infant samples from five European centres. Microbiology. (2011) 157:1385–9.
14. Robertson RC, Manges AR, Finlay BB, Prendergast AJ. The Human Microbiome and child growth-first 1000 days and beyond. Trends Microbiol. (2019) 27:131–47. doi: 10.1016/j.tim.2018.09.008
15. Yatsunenko T, Rey FE, Manary MJ, Trehan I, Dominguez-Bello MG, Contreras M, et al. Human gut microbiome viewed across age and geography. Nature. (2012) 486:222–7.
16. Langdon A, Crook N, Dantas G. The effects of antibiotics on the microbiome throughout development and alternative approaches for therapeutic modulation. Genome Med. (2016) 8:39. doi: 10.1186/s13073-016-0294-z
17. Hagan T, Cortese M, Rouphael N, Boudreau C, Linde C, Maddur MS, et al. Antibiotics-driven gut microbiome perturbation alters immunity to vaccines in humans. Cell. (2019) 178:1313–28. doi: 10.1016/j.cell.2019.08.010
18. Moreau MC, Ducluzeau R, Guy-Grand D, Muller MC. Increase in the population of duodenal immunoglobulin A plasmocytes in axenic mice associated with different living or dead bacterial strains of intestinal origin. Infect Immun. (1978) 21:532–9.
19. Franchi L, Warner N, Viani K, Nuñez G. Function of Nod-like receptors in microbial recognition and host defense. Immunol Rev. (2009) 227:106–28. doi: 10.1111/j.1600-065X.2008.00734.x
20. Baba N, Samson S, Bourdet-Sicard R, Rubio M, Sarfati M. Commensal bacteria trigger a full dendritic cell maturation program that promotes the expansion of non-Tr1 suppressor T cells. J Leukoc Biol. (2008) 84:468–76. doi: 10.1189/jlb.0108017
21. Fulde M, Hornef M. Maturation of the enteric mucosal innate immune system during the postnatal period. Immunol Rev. (2014) 260:21–34. doi: 10.1111/imr.12190
22. Wesemann DR, Portuguese A, Meyers RM, Gallagher MP, Cluff-Jones K, Magee LM, et al. Microbial colonization influences early B-lineage development in the gut lamina propria. Nature. (2013) 501:112–5. doi: 10.1038/nature12496
23. Inman CF, Laycock G, Mitchard L, Harley R, Warwick J, Burt R, et al. Neonatal colonisation expands a specific intestinal antigen-presenting cell subset prior to CD4 T-cell expansion, without altering T-cell repertoire. PLoS One. (2012) 7:e33707. doi: 10.1371/journal.pone.0033707
24. Okogbule-Wonodi AC, Li G, Anand B, Luzina IG, Atamas SP, Blanchard T. Human foetal intestinal fibroblasts are hyper-responsive to lipopolysaccharide stimulation. Dig Liver Dis. (2012) 44:18–23. doi: 10.1016/j.dld.2011.08.017
25. Sansonetti PJ. To be or not to be a pathogen: that is the mucosally relevant question. Mucosal Immunol. (2011) 4:8–14. doi: 10.1038/mi.2010.77
26. Manicassamy S, Reizis B, Ravindran R, Nakaya H, Salazar-Gonzalez RM, Wang YC, et al. Activation of beta-catenin in dendritic cells regulates immunity versus tolerance in the intestine. Science. (2010) 329:849–53. doi: 10.1126/science.1188510
27. Franchi L, Kamada N, Nakamura Y, Burberry A, Kuffa P, Suzuki S, et al. NLRC4-driven production of IL-1beta discriminates between pathogenic and commensal bacteria and promotes host intestinal defense. Nat Immunol. (2012) 13:449–56. doi: 10.1038/ni.2263
28. Christmann BS, Abrahamsson TR, Bernstein CN, Duck LW, Mannon PJ, Berg G, et al. Human seroreactivity to gut microbiota antigens. J Allergy Clin Immunol. (2015) 136:1378–86. doi: 10.1016/j.jaci.2015.03.036
29. West CE, Rydén P, Lundin D, Engstrand L, Tulic MK, Prescott SL. Gut microbiome and innate immune response patterns in IgE-associated eczema. Clin Exp Allergy. (2015) 45:1419–29. doi: 10.1111/cea.12566
30. Fujimura KE, Sitarik AR, Havstad S, Lin DL, Levan S, Fadrosh D, et al. Neonatal gut microbiome associates with childhood multisensitized atopy and T cell differentiation. Nat Med. (2016) 22:1187–91. doi: 10.1038/nm.4176
31. Simonyté Sjödin K, Hammarström ML, Rydén P, Sjödin A, Hernell O, Engstrand L, et al. Temporal and long-term gut microbiota variation in allergic disease: a prospective study from infancy to school age. Allergy. (2019) 74:176–85. doi: 10.1111/all.13485
32. Clavel T, Desmarchelier C, Haller D, Gérard P, Rohn S, Lepage P, et al. Intestinal microbiota in metabolic diseases: from bacterial community structure and functions to species of pathophysiological relevance. Gut Microbes. (2014) 5:544–51. doi: 10.4161/gmic.29331
33. Jatzlauk G, Bartel S, Heine H, Schloter M, Krauss -Etschmann S. Influences of environmental bacteria and their metabolites on allergies, asthma, and host microbiota. Allergy. (2017) 72:1859–67. doi: 10.1111/all.13220
34. Carpenter L, Beral V, Stachan D, Ebi-Kryston KL, Inskip H. Respiratory symptoms as predictors of 27 year mortality in a representative sample of British adults. BMJ. (1989) 299:357–61.
35. Van Neerven RJ, Knol E, Heck JM, Savelkoul HF. Which factors in raw cow’s milk contribute to protection against allergies? J Allergy Clin Immunol. (2012) 130:853–8.
36. Chatenoud L, Bertuccio P, Turati F, Galeone C, Naldi L, La Vecchia C, et al. Marker of microbial exposure lower the incidence of atopic dermatitis. Allergy. (2020) 75:104–15. doi: 10.1111/all.13990
37. Lynch SV, Wood R, Boushey H, Bacharier LB, Bloomberg GR, Kattan M, et al. Effects of earlylife exposure to allergens and bacteria on recurrent wheeze and atopy in urban children. J Allergy Clin Immunol. (2014) 134:593–601. doi: 10.1016/j.jaci.2014.04.018
38. Raciborski F, Tomaszewska A, Komorowski J, Samel-Kowalik P, Bialoszewski AZ, Walkiewicz A, et al. The relationship between antibiotic therapy in early childhood and the symptoms of allergy in children aged 6-8 years-the questionnaire study results. Int J Occup Med Environ Health. (2012) 25:470–80. doi: 10.2478/S13382-012-0056-0
39. Dzidic M, Abrahamsson T, Artacho A, Collado MC, Mira A, Jenmalm MC. Oral microbiota maturation during the first 7 years of life in relation to allergy development. Allergy. (2018) 73:2000–11. doi: 10.1111/all.13449
40. Mortha A, Chudnovskiy A, Hashimoto D, Bogunovic M, Spencer SP, Belkaid Y, et al. Microbiota-dependent crosstalk between macrophages and ILC3 promotes intestinal homeostasis. Science. (2014) 343:1249–88.
41. Thompson -Chagoyan OC, Vieites JM, Maldonado J, Edwards C, Gil A. Changes in faecal microbiota of infants with cow’s milk protein allergy–a Spanish prospective case-control 6 -month follow-up study. Pediatr Allergy Immunol. (2010) 21:394–400.
42. Bunyavanich S, Shen N, Grishin A, Wood R, Burks W, Dawson P, et al. Early-life gut microbiome composition and milk allergy resolution. J Allergy Clin Immunol. (2016) 138:1122–30.
43. Fazlollahi M, Chun Y, Grishin A, Wood RA, Burks AW, Dawson P, et al. Early-life gut microbiome and egg allergy. Allergy. (2018) 73:1515–24. doi: 10.1111/all.13389
44. Savage JH, Lee-Sarwar SK, Sordillo J, Bunyavanich S, Zhou Y, O’Connor G, et al. A prospective microbiome-wide association study of food sensitization and food allergy in early childhood. Allergy. (2018) 73:145–52.
45. Hua X, Goedert JJ, Pu A, Yu G, Shi J. Allergy associations with the adult fecal microbiota: analysis of the American Gut Project. EBioMedicine. (2016) 3:172–9. doi: 10.1016/j.ebiom.2015.11.038
46. Feehley T, Plunkett CH, Bao R, Hong SMC, Culleen E, Belda-Ferre P, et al. Healthy infants harbor intestinal bacteria that protect against food allergy. Nat Med. (2019) 25:448–53. doi: 10.1038/s41591-018-0324-z
47. Lyons A, O’Mahony D, O’Brien F, MacSharry J, Sheil B, Ceddia M, et al. Bacterial strain-specific induction of Foxp31 T regulatory cells is protective in murine allergy models. Clin Exp Allergy. (2010) 40:811–9. doi: 10.1111/j.1365-2222.2009.03437.x
48. Stefka AT, Feehley T, Tripathi P, Qiu J, McCoy K, Mazmanian SK, et al. Commensal bacteria protect against food allergen sensitization. Proc Natl Acad Sci USA. (2014) 111:13145–50. doi: 10.1073/pnas.1412008111
49. Berni Canani R, Sangwan N, Stefka AT, Nocerino R, Paparo L, Aitoro R, et al. Lactobacillus rhamnosus GG-supplemented formula expands butyrate-producing bacterial strains in food allergic infants. ISME J. (2016) 10:742–50. doi: 10.1038/ismej.2015.151
50. Hol J, van Leer EH, Elink Schuurman BE, de Ruiter LF, Samsom JN, Hop W, et al. The acquisition of tolerance toward cow’s milk through probiotic supplementation: a randomized, controlled trial. J Allergy Clin Immunol. (2008) 121:1448–54. doi: 10.1016/j.jaci.2008.03.018
51. Berni Canani R, Di Costanzo M, Bedogni G, Amoroso A, Cosenza L, Di Scala C, et al. Extensively hydrolyzed casein formula containing Lactobacillus rhamnosus GG reduces the occurrence of other allergic manifestations in children with cow’s milk allergy: 3-year randomized controlled trial. J Allergy Clin Immunol. (2017) 139:1906–13. doi: 10.1016/j.jaci.2016.10.050
52. Wopereis H, Van Ampting MTJ, Cetinyurek-Yavuz A, Slump R, Candy DCA, Butt AM, et al. A specific synbiotic-containing amino acid-based formula restores gut microbiota in non-IgE mediated cow’s milk allergic infants: a randomized controlled trial. Clin Transl Allergy. (2019) 9:27.
53. Fox AT, Wopereis H, Van Ampting MTJ, Oude Nijihuis MM, Butt AM, Peroni DG, et al. A specific synbiotic-containing amino acid-based formula in dietary management of cow’s milk allergy: a randomized controlled trial. Clin Transl Allergy. (2019) 15:9. doi: 10.1186/s13601-019-0241-3
54. Candy DCA, Van Ampting MTJ, Nijhuis O, Wopereis H, Butt AM, Peroni DG, et al. A synbiotic-containing amino acid-based formula improves gut microbiota in non-IgE mediated allergic infants. Pediatric Res. (2018) 83:677–86.
55. Tang ML, Ponsonby AL, Orsini F, Tey D, Robinson M, Su EL, et al. Administration of a probiotic with peanut oral immunotherapy: a randomized trial. J Allergy Clin Immunol. (2015) 135:737–44. doi: 10.1016/j.jaci.2014.11.034
56. Byrd AL, Belkaid Y, Segre JA. The human skin microbiome. Nat Rev Microbiol. (2018) 16:143–55. doi: 10.1038/nrmicro.2017.157
57. Zhu TH, Zhu TR, Tran KA, Sivanami RK, Shi VY. Epithelial barrier dysfunctions in atopic dermatitis: a skin -gut-lung model linking microbiome alteration and immune dysregulation. Br J Dermatol. (2018) 179:570–81. doi: 10.1111/bjd.16734
58. Huang Y, Marsland B, Bunyavanich S, O’Mahony L, Leung D, Muraro A, et al. The microbiome in allergic disease: current understanding and future opportunities -2017 PRACTALL document of the American Academy of allergy, asthma & immunology and the european academy of allergy and clinical immunology. J Allergy Clin Immunol. (2017) 139:1099–110. doi: 10.1016/j.jaci.2017.02.007
59. Baurecht H, Rühlemann MC, Rodríguez E, Thielking F, Harder I, Erkens AS, et al. Epidermal lipid composition, barrier integrity, and eczematous inflammation are associated with skin microbiome configuration. J Allergy Clin Immunol. (2018) 141:1668–76. doi: 10.1016/j.jaci.2018.01.019
60. Malhotra N, Yoon J, Leyva-Castillo JM, Galand C, Archer N, Miller LS, et al. IL -22 derived from γδ T cells restricts Staphylococcus aureus infection of mechanically injured skin. J Allergy Clin Immunol. (2016) 138:1098–107.
61. Geoghegan JA, Irvine A, Foster TJ. Staphylococcus aureus and atopic dermatitis: a complex and evolving relationship. Trends Microbiol. (2018) 26:484–97. doi: 10.1016/j.tim.2017.11.008
62. Iwamoto K, Stroisch TJ, Koch S, Hermann N, Leib N, Bieber T. Langerhans and inflammatory dendritic epidermal cells in atopic dermatitis are tolerized towards TLR2 activation. Allergy. (2018) 73:2205–13.
63. Yuki T, Yoshida H, Akazawa Y, Komiya A, Sugiyama Y, Inoue S. Activation of TLR2 enhances tight junction barrier in epidermal keratinocytes. J Immunol. (2011) 187:3230–7. doi: 10.4049/jimmunol.1100058
64. Yamazaki Y, Nakamura Y, Núñez G. Role of the microbiome in skin immunity and atopic dermatitis. Allergol Int. (2017) 66:539–44. doi: 10.1016/j.alit.2017.08.004
65. Kennedy EA, Connolly J, Hourihane JO, Fallon PG, McLeanet IWH, Murray D, et al. Skin microbiome before development of atopic dermatitis: early colonization with commensal staphylococci at 2 months is associated with a lower risk of atopic dermatitis at 1 year. J Allergy Clin Immunol. (2017) 139:166–72.
66. Lynde CW, Andriessen A, Bertucci V, McCuaig C, Skotnicki S, Weinstein M, et al. The skin microbiome in atopic dermatitis and its relationship to emollients. J Cutan Med Surg. (2016) 20:21–8. doi: 10.1177/1203475415605498
67. Seite S, Flores GE, Henley JB, Martin R, Zelenkova H, Luc A, et al. Microbiome of affected and unaffected skin of patients with atopic dermatitis before and after emollient treatment. J Drugs Dermatol. (2014) 13:1365–72.
68. Glatz M, Jo JH, Kennedy EA, Polley EP, Segre JA, Simpson EL, et al. Emollient use alters skin barrier and microbes in infants at risk for developing atopic dermatitis. PLoS One. (2018) 13:e0192443. doi: 10.1371/journal.pone.0192443
69. Gueniche A, Knaudt B, Schuck E, Volz T, Bastien P, Martin R, et al. Effects of nonpathogenic gram-negative bacterium Vitreoscilla filiformis lysate on atopic dermatitis: a prospective, randomized, double-blind, placebo-controlled clinical study. Br J Dermatol. (2008) 159:1357–63. doi: 10.1111/j.1365-2133.2008.08836.x
70. Myles IA, Earland NJ, Anderson, Moore IN, Kieh MD, Williams KW, et al. First -in-human topical microbiome transplantation with Roseomonas mucosa for atopic dermatitis. JCI Insight. (2018) 3:e120608. doi: 10.1172/jci.insight.120608
71. Nakatsuji T, Chen TH, Narala S, Chun KA, Two AM, Yun T, et al. Antimicrobials from human skin commensal bacteria protect against Staphylococcus aureus and are deficient in atopic dermatitis. Sci Transl Med. (2017) 9:378. doi: 10.1126/scitranslmed.aah4680
72. Watanabe S, Narisawa Y, Arase S, Okamatsu H, Ikenaga T, Tajiri Y, et al. Differences in fecal microflora between patients with atopic dermatitis and healthy control subjects. J Allergy Clin Immunol. (2003) 111:587–91.
73. Penders J, Thijs C, van den Brandt PA, Kummeling I, Snijders B, Stelma F, et al. Gut microbiome composition and development of atopic manifestations in infancy: the KOALA birth cohort study. Gut. (2007) 56:661–7.
74. Abrahamsson TR, Jakobsson HE, Andersson AF, Bjorksten B, Engstrand L, Jenmalm MC. Low diversity of the gut microbiota in infants with atopic eczema. J Allergy Clin Immunol. (2012) 129:434–40.
75. Rø ADB, Simpson MR, Rø TB, Storrø O, Johnsen R, Videm V, et al. Reduced Th22 cell proportion and prevention of atopic dermatitis in infants following maternal probiotic supplementation. Clin Exp Allergy. (2017) 47:1014–21. doi: 10.1111/cea.12930
76. Galazzo G, van Best N, Bervoets L, Dapaah IO, Savelkoul PH, Hornef MW, et al. Development of the microbiota and associations with birth mode, diet, and atopic disorders in a longitudinal analysis of stool samples, collected from infancy through early childhood. Gastroenterology. (2020) [Epub ahead of print]. S0016-5085(20)30113-X.
77. Bisgaard H, Hermansen MN, Buchvald F, Loland L, Brydensholt Halkjaer L, Bønnelykke K, et al. Childhood asthma after bacterial colonization of the airway in neonates. N Engl J Med. (2007) 357:1487–95.
78. Mansbach JM, Luna PN, Shaw CA, Hasegawa K, Petrosino JP, Piedra PA, et al. Increased Moraxella and Streptococcus species abundance after severe bronchiolitis is associated with recurrent wheezing. J Allergy Clin Immunol. (2020) 145:518–27. doi: 10.1016/j.jaci.2019.10.034
79. Hammad H, Chieppa M, Perros F, Willart MA, Germain RN, Lambrecht BN. House dust mite allergen induces asthma via Toll-like receptor 4 triggering of airway structural cells. Nat Med. (2009) 15:410–647. doi: 10.1038/nm.1946
80. Schuijs MJ, Willart M, Vergote K, Gras D, Deswarte K, Ege MJ, et al. Farm dust and endotoxin protect against allergy through A20 induction in lung epithelial cells. Science. (2015) 349:1106–10. doi: 10.1126/science.aac6623
81. Hilty M, Burke C, Pedro H, Cardenas P, Bush A, Bossley C, et al. Disordered microbial communities in asthmatic airways. PLoS One. (2010) 5:e8578. doi: 10.1371/journal.pone.0008578
82. Di Cicco M, Pistello M, Jacinto T, Ragazzo V, Piras M, Freer G, et al. Does lung microbiome play a causal or casual role in asthma? Pediatr Pulmonol. (2018) 53:1340–5. doi: 10.1002/ppul.24086
83. Gollwitzer ES, Saglani S, Trompette A, Yadava K, Sherburn R, McCoy KD, et al. Lung microbiome promotes tolerance to allergens in neonates via PD-L1. Nat Med. (2014) 20:642–7. doi: 10.1038/nm.3568
84. Ege MJ, Mayer M, Normand AC, Genuneit J, Cookson WO, Braun-Fahrlander C, et al. Exposure to environmental microorganisms and childhood asthma. N Engl J Med. (2011) 364:701–9. doi: 10.1056/NEJMoa1007302
85. Teo SM, Mok D, Pham K, Kusel M, Serralha M, Troy N, et al. The infant nasopharyngeal microbiome impacts severity of lower respiratory infection and risk of asthma development. Cell Host Microbe. (2015) 17:704–15. doi: 10.1016/j.chom.2015.03.008
86. Huang YJ, Narya S, Harris JM, Lynch SV, Choy DF, Arron JR, et al. The airway microbiome in patients with severe asthma: associations with disease features and severity. J Allergy Clin Immunol. (2015) 136:874–84. doi: 10.1016/j.jaci.2015.05.044
87. Ghebre MA, Pang PH, Diver S, Desai D, Bafadhel M, Haldar K, et al. Biological exacerbation clusters demonstrate asthma and chronic obstructive pulmonary disease overlap with distinct mediator and microbiome profiles. J Allergy Clin Immunol. (2018) 141:2027–36. doi: 10.1016/j.jaci.2018.04.013
88. Russell SL, Gold MJ, Hartmann M, Willing BP, Thorson L, Wlodarska M, et al. Early life antibiotic-driven changes in microbiome enhance susceptibility to allergic asthma. EMBO Rep. (2012) 13:440–7. doi: 10.1038/embor.2012.32
89. Metsälä J, Lundgvist A, Virta LJ, Kaila M, Gissler M, Virtanen SM. Prenatal and post-natal exposure to antibiotics and risk of asthma in childhood. Clin Exp Allergy. (2015) 45:137–45. doi: 10.1111/cea.12356
90. Arrieta MC, Stiemsma LT, Dimitriu PA, Thorson L, Russell S, Yurist-Doutsch S, et al. Early infancy microbial and metabolic alterations affect risk of childhood asthma. Sci Transl Med. (2015) 7:307ra152. doi: 10.1126/scitranslmed.aab2271
91. Stokholm J, Blaser MJ, Thorsen J, Rasmussen MA, Waage J, Vinding RK, et al. Maturation of the gut microbiome and risk of asthma in childhood. Nat Commun. (2018) 9:141. doi: 10.1038/s41467-017-02573-2
92. Thorburn AN, McKenzie CI, Shen S, Stanley D, Macia L, Mason LJ, et al. Evidence that asthma is a developmental origin disease influenced by maternal diet and bacterial metabolites. Nat Commun. (2015) 6:7320. doi: 10.1038/ncomms8320
93. Canani RB, Costanzo MD, Leone L, Pedata M, Meli R, Calignano A. Potential beneficial effects of butyrate in intestinal and extraintestinal diseases. World J Gastroenterol. (2011) 17:1519–28. doi: 10.3748/wjg.v17.i12
94. Trompette A, Gollwitzer ES, Yadava K, Sichelstiel AK, Sprenger N, Ngom-Bru C, et al. Gut microbiome metabolism of dietary fiber influences allergic airway disease and hematopoiesis. Nat Med. (2014) 20:159–66.
95. Roduit C, Frei R, Ferstl R, Loeliger S, Westermann P, Ryhner C, et al. High levels of butyrate and propionate in early life are associated with protection against atopy. Allergy. (2019) 74:799–809. doi: 10.1111/all.13660
Keywords: allergy, asthma, atopic dermatitis, food allergy, health outcomes, immune system, children, microbiome
Citation: Peroni DG, Nuzzi G, Trambusti I, Di Cicco ME and Comberiati P (2020) Microbiome Composition and Its Impact on the Development of Allergic Diseases. Front. Immunol. 11:700. doi: 10.3389/fimmu.2020.00700
Received: 14 January 2020; Accepted: 27 March 2020;
Published: 23 April 2020.
Edited by:
Maria Carmen Collado, Institute of Agrochemistry and Food Technology (IATA), SpainReviewed by:
Tommi Vatanen, The University of Auckland, New ZealandCopyright © 2020 Peroni, Nuzzi, Trambusti, Di Cicco and Comberiati. This is an open-access article distributed under the terms of the Creative Commons Attribution License (CC BY). The use, distribution or reproduction in other forums is permitted, provided the original author(s) and the copyright owner(s) are credited and that the original publication in this journal is cited, in accordance with accepted academic practice. No use, distribution or reproduction is permitted which does not comply with these terms.
*Correspondence: Diego G. Peroni, ZGllZ28ucGVyb25pQHVuaXBpLml0
Disclaimer: All claims expressed in this article are solely those of the authors and do not necessarily represent those of their affiliated organizations, or those of the publisher, the editors and the reviewers. Any product that may be evaluated in this article or claim that may be made by its manufacturer is not guaranteed or endorsed by the publisher.
Research integrity at Frontiers
Learn more about the work of our research integrity team to safeguard the quality of each article we publish.