- Department of Experimental Anesthesiology, Charité – Universitätsmedizin Berlin, Corporate Member of Freie Universität Berlin, Humboldt-Universität zu Berlin, Berlin Institute of Health, Berlin, Germany
Opioid receptors comprise μ (MOP), δ (DOP), κ (KOP), and nociceptin/orphanin FQ (NOP) receptors. Opioids are agonists of MOP, DOP, and KOP receptors, whereas nociceptin/orphanin FQ (N/OFQ) is an agonist of NOP receptors. Activation of all four opioid receptors in neurons can induce analgesia in animal models, but the most clinically relevant are MOP receptor agonists (e.g., morphine, fentanyl). Opioids can also affect the function of immune cells, and their actions in relation to immunosuppression and infections have been widely discussed. Here, we analyze the expression and the role of opioid receptors in peripheral immune cells and glia in the modulation of pain. All four opioid receptors have been identified at the mRNA and protein levels in immune cells (lymphocytes, granulocytes, monocytes, macrophages) in humans, rhesus monkeys, rats or mice. Activation of leukocyte MOP, DOP, and KOP receptors was recently reported to attenuate pain after nerve injury in mice. This involved intracellular Ca2+-regulated release of opioid peptides from immune cells, which subsequently activated MOP, DOP, and KOP receptors on peripheral neurons. There is no evidence of pain modulation by leukocyte NOP receptors. More good quality studies are needed to verify the presence of DOP, KOP, and NOP receptors in native glia. Although still questioned, MOP receptors might be expressed in brain or spinal cord microglia and astrocytes in humans, mice, and rats. Morphine acting at spinal cord microglia is often reported to induce hyperalgesia in rodents. However, most studies used animals without pathological pain and/or unconventional paradigms (e.g., high or ultra-low doses, pain assessment after abrupt discontinuation of chronic morphine treatment). Therefore, the opioid-induced hyperalgesia can be viewed in the context of dependence/withdrawal rather than pain management, in line with clinical reports. There is convincing evidence of analgesic effects mediated by immune cell-derived opioid peptides in animal models and in humans. Together, MOP, DOP, and KOP receptors, and opioid peptides in immune cells can ameliorate pathological pain. The relevance of NOP receptors and N/OFQ in leukocytes, and of all opioid receptors, opioid peptides and N/OFQ in native glia for pain control is yet to be clarified.
Introduction
Opioid receptors comprise four members, the classical μ (MOP), δ (DOP), and κ (KOP) receptors, and the non-classical nociceptin/orphanin FQ (NOP) receptor [reviewed by (1)] (Table 1). They belong to the superfamily of seven transmembrane domain, G protein-coupled receptors, are encoded by the four respective genes [(2–5), reviewed by (6)], and their structures have been cleared by crystal analysis (7–10). The classical opioid receptors are sensitive to the antagonist naloxone and their endogenous agonists are opioid peptides, such as β-endorphin, enkephalins (Met-, Leu-enkephalin), and dynorphins (dynorphin A, B, α-neoendorphin). β-endorphin and enkephalins bind MOP and DOP receptors, whereas dynorphin A 1-17 preferentially acts at KOP receptors. Opioid peptides derive from the respective precursors, proopiomelanocortin (POMC) (11, 12), proenkephalin (PENK) (13, 14), and prodynorphin (PDYN) (15–17). Endomorphins (endomorphin-1,−2) are additional, putative endogenous opioid peptides with high selectivity at MOP receptors (18); their precursor has not yet been identified [reviewed by (19)]. NOP receptors are insensitive to antagonism by naloxone, have low affinity for opioid peptides, and their selective endogenous agonist is nociceptin/orphanin FQ (N/OFQ), which derives from prepro-N/OFQ (ppN/OFQ) [(20, 21) reviewed by (6)] (Table 1).
Neuronal opioid receptors are widely distributed throughout the peripheral (trigeminal and dorsal root ganglia) and central (spinal cord, brain) nervous system. All four opioid receptors mediate analgesia in animal models. However, the majority of clinically used opioids for pain treatment are MOP receptor agonists (e.g., morphine, fentanyl, oxycodone). Centrally acting KOP receptor agonists are of limited utility due to dysphoric and psychotomimetic effects (22–24), whereas DOP and NOP receptor agonists are not available for clinical use. Mechanistically, following acute activation by an agonist (endogenous or exogenous), opioid receptors couple to the pertussis toxin-sensitive heterotrimeric Gi/o proteins, which dissociate into Gαi/o and Gβγ subunits to interact with various intracellular effectors (Figure 1A). Activation of all four MOP, DOP, KOP, and NOP receptors can result in the Gαi/o-dependent inhibition of adenylyl cyclases (AC) and cyclic adenosine monophosphate (cAMP) formation [reviewed by (25, 26)]. However, the exact pathway in which these actions result in pain inhibition has only been described for MOP receptors. Hence, the decreased cAMP production leads to the inhibition of protein kinase A (PKA) activity, which results in the suppression of various ion channels involved in pain facilitation. These channels include the heat sensor transient receptor potential cation channel subfamily V member 1 (TRPV1), hyperpolarization-activated cyclic nucleotide-gated (HCN) channels, acid-sensing ion channels (ASIC), and voltage-gated Na+ (Nav) channels (27–31). Through the Gβγ, all four receptors close voltage-gated Ca2+ (Cav) channels (32–34) and open G protein-coupled inwardly-rectifying K+ (GIRK or Kir3) channels (35–39), whereas MOP and DOP receptors also activate adenosine triphosphate-sensitive K+ (KATP) channels [(40), reviewed by (26, 41)]. Additionally, MOP receptors inhibit heat-sensing transient receptor potential cation channel subfamily M member 3 (TRPM3) (42). These opioid receptor-mediated actions lead to the hyperpolarization and decreased excitability of central and peripheral sensory neurons, as well as to the diminished release of excitatory mediators from these neurons, including substance P (43–48), calcitonin gene-related peptide (45, 49–51), and glutamate (52, 53). In addition, activation of MOP receptors in the brain activates descending noradrenergic pathways, which leads to increased release of noradrenaline in the spinal cord (54, 55). All above described effects underlay the opioid receptor-induced analgesia [reviewed by (26, 41, 56–60)]. Additionally, the activation of NOP receptors in the brain can lead to hyperalgesia or anti-opioid actions in animal models (20, 21, 61). NOP receptors also couple to pertussis toxin-insensitive Gαs, Gαz, or Gα16 proteins (62, 63), but the role of these pathways in pain modulation is unknown.
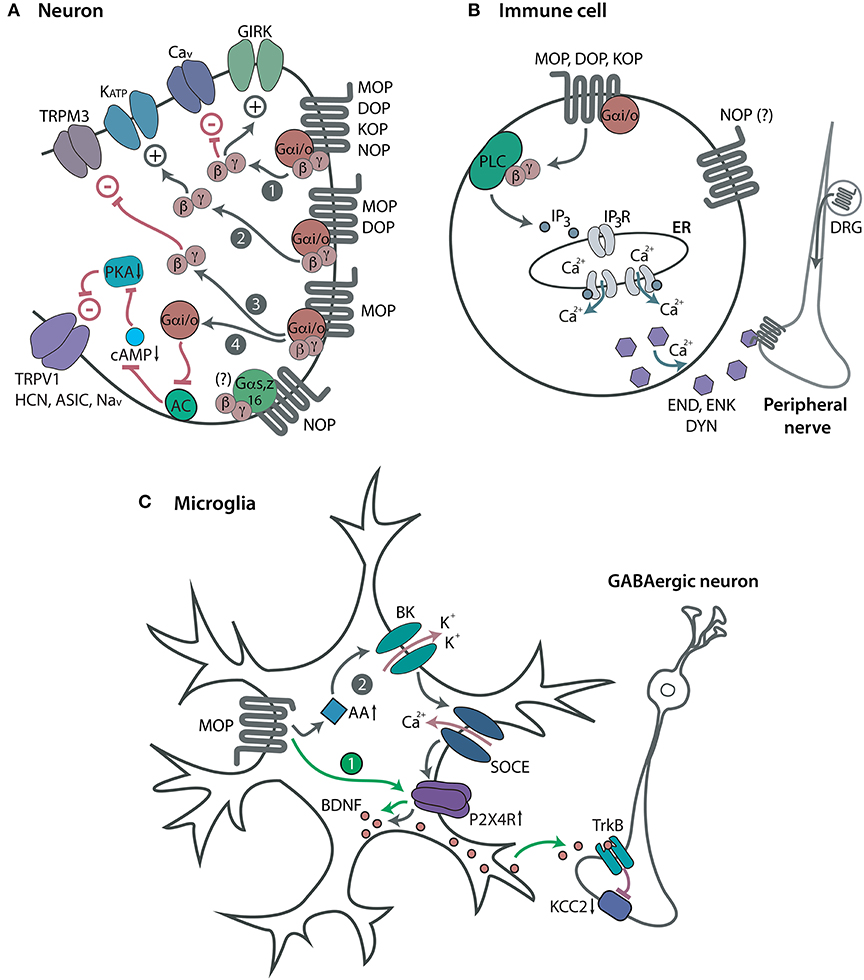
Figure 1. Opioid receptors and modulation of pain. (A) Neuronal opioid receptors. Acute activation of Gαi/o-coupled MOP, DOP, KOP, and NOP receptors in central or peripheral sensory neurons leads to the opening of GIRK channels and closing of Cav channels via the Gβγ (path 1). Through the Gβγ, MOP and DOP receptors also open KATP channels (path 2), and MOP receptors close TRPM3 channels (path 3). Through the Gαi/o, MOP receptors inhibit AC, cAMP formation and PKA activity, which leads to closing of TRPV1, HCN, ASIC, and Nav channels (path 4). All these effects decrease neuronal excitability, which results in analgesia. NOP receptors also couple to Gαs, Gαz or Gα16, but their role in pain modulation is unknown (indicated by a question mark). (B) Immune cell opioid receptors. Acute activation of Gαi/o-coupled MOP, DOP, and KOP receptors in immune cells accumulating in peripheral injured tissue leads to the Gβγ-mediated activation of PLC and production of IP3 which activates IP3R in endoplasmic reticulum (ER). This results in the intracellular Ca2+-dependent release of opioid peptides, β-endorphin (END), Met-enkephalin (ENK), and dynorphin A 1-17 (DYN). The secreted opioid peptides activate opioid receptors (MOP, DOP, KOP) in peripheral nerves and diminish pain. NOP receptors are also expressed in immune cells, but their function has not been identified (indicated by a question mark). (C) Microglial opioid receptors. Repetitive activation of MOP receptors in spinal cord microglia upregulates purinergic P2X4 receptors (P2X4R), which triggers the release of BDNF from microglia. The secreted BDNF activates the tropomyosin receptor kinase B (TrkB) to downregulate the K+-Cl− co-transporter KCC2 in GABAergic spinal neurons, which leads to their disinhibition (path 1). Microglial MOP receptor activation can also elevate AA levels to facilitate the opening of BK channels. This triggers the Ca2+ influx via store-operated Ca2+ entry (SOCE) and consequent upregulation of P2X4R and BDNF synthesis in microglia (path 2). Both signaling pathways are suggested to potentiate the neurotransmission in the spinal cord and account for OIH. However, these effects may be a consequence of opioid withdrawal rather than direct hyperalgesic opioid actions. Expression and function of DOP, KOP, and NOP receptors in glia are yet to be clarified.
Neuronal opioid receptors also mediate numerous side effects, such as respiratory depression, nausea, vomiting, reward/euphoria, dependence/withdrawal (MOP), convulsions (DOP), aversion/dysphoria, psychotomimesis (KOP), learning and memory impairment, motor disturbance, hypotension, bradycardia (NOP), sedation (MOP, KOP, NOP), constipation (MOP, NOP), and diuresis (KOP, NOP) [reviewed by (56, 57, 64–67)]. The main efforts are currently directed toward the development of novel ligands that exert analgesia with reduced side effects [reviewed by (57, 68, 69)].
Opioids (endogenous and exogenous) can also affect the function of immune cells, including proliferation, maturation, chemotaxis, trafficking, phagocytosis, cytokine, and chemokine receptor expression, cytokine synthesis and secretion. These effects were predominately assessed in vitro and the results were often contradictory, which depended on experimental conditions (e.g., cultured cell types, lines or clones, duration of cultures, media composition, doses and timing of opioid exposure) [reviewed by (70, 71)]. The immunomodulatory actions of opioids in the context of immunosuppression and infections have been widely reviewed (72–77).
In this article, we analyze the expression of opioid receptors in peripheral immune cells and glia, and discuss their contribution to the modulation of pain. Specifically, we address peripheral immune cells, such as lymphocytes, monocytes, macrophages and granulocytes in the blood and peripheral tissue. Although in pathological pain conditions some of these cells also infiltrate central nervous system (78–81), they have not been examined for the expression of opioid receptors. Glia represent immune cells of the nervous system and they include microglia, astrocytes and oligodendrocytes in the spinal cord and brain, satellite glial cells in trigeminal and dorsal root ganglia, and Schwann cells in peripheral nerves [reviewed by (82, 83)]. Of these cells, microglia, astrocytes and oligodendrocytes were so far tested for the presence of opioid receptors, and they are addressed in the following sections. Additionally, we describe the relevance of opioid peptides and N/OFQ derived from immune and glial cells to pain control.
Expression of Opioid Receptors in Immune and Glial Cells
Expression and function of opioid receptors have been extensively examined in vitro using cell lines or cultured primary immune and glial cells. Since the results obtained in such conditions often vary with experimental setups, including cell origin and density (84–86), and do not reflect the in vivo situation, we focus on ex vivo studies which examined tissue or freshly isolated, not cultured primary immune and glial cells.
Immune Cells
Expression of opioid receptors in peripheral immune cells has been postulated since the early 1980s [reviewed by (71, 76, 87)], but some findings still remain controversial. For example, some authors using various methods, such as radioligand binding, flow cytometry, polymerase chain reaction (PCR) and real-time quantitative PCR (qPCR) did not detect MOP, DOP, and KOP receptors in peripheral blood mononuclear cells (PBMC) or all blood cells from healthy human donors; only NOP receptor mRNA was found by PCR and qPCR (88, 89). Similarly, MOP and DOP mRNAs were not identified in healthy human blood lymphocytes or monocytes (90, 91), and MOP and KOP mRNAs were not found in mouse splenocytes and T lymphocytes (90) (Table 2).
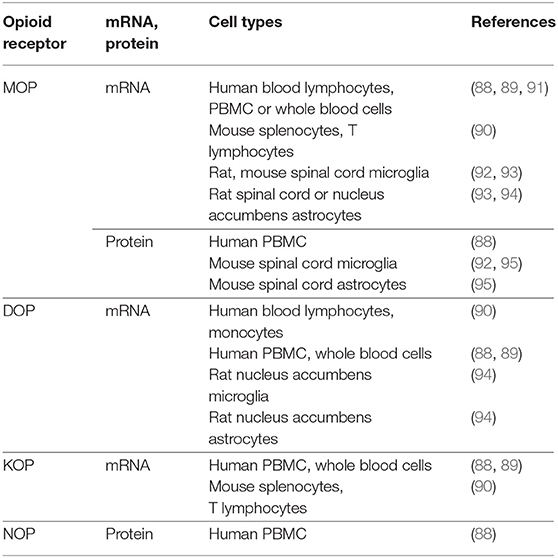
Table 2. Studies that did not detect opioid receptors in native, not cultured immune and glial cells.
However, many other studies did detect MOP, DOP, and KOP receptors in immune cells of various species (Table 3). MOP receptor mRNA was identified by PCR in blood CD4+ T lymphocytes, monocytes/macrophages and granulocytes from healthy human donors, in PBMC and granulocytes from rhesus monkeys (96), and in rat peritoneal macrophages (98). Decreased levels of MOP receptor mRNA were found in blood lymphocytes of heroin addicts on methadone maintenance compared to healthy controls (97). In the latter study, similar results were obtained for DOP receptor mRNA (97). DOP receptor mRNA was additionally found in mouse splenocytes and enriched T lymphocyte fraction (90, 109), in lymph node dendritic cells and CD4+ T lymphocytes of mice immunized with ovalbumin (but not in non-immunized mice) (110), and in human blood lymphocytes (assessed by Northern blot) (91). Also KOP receptor mRNA was detected by PCR or Northern blot in blood lymphocytes (90, 91), monocytes (111), PBMC and CD4+ T lymphocytes from healthy human donors, and in PBMC from rhesus monkeys (113). In rheumatoid arthritis patients, KOP receptor mRNA was found in blood T and B lymphocytes, monocytes/macrophages and natural killer cells. Analysis of the whole blood cell samples revealed that the mRNA levels were lower in patients with high pain scores compared to those with less severe pain (112). For all three receptors, the mRNA transcripts were cloned and sequenced, and found to be nearly (98%) or completely (100%) homologous to the human, rat or mouse brain receptors (90, 91, 96, 98, 109, 113). Additionally, qPCR revealed mRNAs of MOP, DOP, and KOP receptors in blood leukocytes, and at higher levels in leukocytes (comprising macrophages, neutrophils and T lymphocytes) isolated from injured sciatic nerves in a mouse model of neuropathic pain. Activation of each receptor by selective agonists led to the secretion of opioid peptides from immune cells isolated from wild-type mice, but not from the MOP, DOP or KOP receptor knockout mice [(99); see also below]. This suggests that leukocyte MOP, DOP, and KOP receptors were functional and encoded by the same genes as neuronal receptors (99). NOP receptor mRNA was identified by PCR or qPCR in blood lymphocytes, monocytes and granulocytes from healthy human donors (88, 115, 116). The qPCR showed decreased NOP receptor mRNA levels in blood granulocytes from patients with sepsis (117), and comparable levels in blood eosinophils in patients with asthma and healthy volunteers (118). The sequencing of PCR products revealed that NOP receptor transcripts from immune cells and brain were identical (115, 116). Some studies detected the NOP receptor mRNA transcripts in leukocytes at levels comparable to those in human cerebral cortex (115), whereas others found them at very low amounts (88, 121). Together, opioid receptor mRNAs appear to be expressed at relatively low levels in immune cells as compared to neuronal tissue or opioid receptor-expressing immune cell lines (88–91, 109, 110, 121, 122). Additionally, opioid receptor mRNA levels in immune cells can be modified (elevated or diminished) by pathological conditions or pharmacological treatments in vivo (97, 99, 110, 112, 117). These issues need to be considered in order to obtain sufficient amount of tissue (leukocyte numbers) for the analysis.
Detection of MOP, DOP, and KOP receptor proteins is more challenging due to the low expression levels mentioned above and poor specificity of antibodies (123–125). Nevertheless, a few studies described stereospecific and high-affinity opioid binding sites in leukocytes (Table 3). The binding of radiolabeled naloxone displaceable by naltrexone was found in healthy human blood lymphocytes (97), although the receptor type is unclear, since these ligands do not distinguish MOP, DOP, and KOP receptors (Table 1). In contrast, binding of radiolabeled MOP receptor agonist (dihydromorphine) or of radiolabeled DOP receptor agonist (deltorphin I) suggested the presence of MOP receptors in monocytes and granulocytes, and of DOP receptors in granulocytes from blood of healthy human donors (101, 102). In rat splenocytes, MOP receptors were detected using radiolabeled agonist DAMGO whose binding was displaced by the antagonist CTAP, both MOP receptor selective ligands. Similarly, DOP receptors were identified using its selective ligands, the radiolabeled antagonist naltrindole whose binding was displaced by the agonist SNC80 (103). KOP receptor protein was found in mouse peritoneal macrophages by flow cytometry and fluorescently labeled KOP receptor agonist, and the labeling intensity was diminished by the selective antagonist norBNI (114) (see also Table 1 for ligands). NOP receptor protein detection appears variable, with high affinity radiolabeled N/OFQ binding in granulocytes (116), but lack of such binding in PBMC (88) from human blood. The latter research group recently detected NOP receptor binding (reversible by selective NOP receptor antagonist SB-612111) in human blood granulocytes using a novel fluorescent probe for the receptor (a red fluorophore-ATTO594 conjugated to the N/OFQ) (121).
In summary, all four MOP, DOP, KOP, and NOP receptors have been identified ex vivo at the mRNA and protein levels in various types of immune cells in humans, rhesus monkeys, rats and mice (Table 3).
Glia
Compared to peripheral immune cells, the expression of opioid receptors in glia has been less examined, most studies focused on MOP receptors, and the findings are contradictory. MOP receptor mRNA was not detected in microglia and astrocytes in the spinal cord of rats chronically treated with vehicle or morphine, using in situ hybridization for MOP receptor mRNA combined with immunofluorescent staining of microglia and astrocyte markers (93). MOP receptor mRNA was also not found using qPCR in astrocytes isolated from nucleus accumbens of rats after acute injection with vehicle or morphine (94). It was also undetected by transcriptomic profiling of microglia from the spinal cord of naïve or morphine-treated mice (92). Additionally, double-immunofluorescence did not detect MOP receptor protein in spinal cord microglia and astrocytes following single application of vehicle or morphine in mice (95). Similarly, MOP receptor mRNA or protein were not found in spinal cord microglia of naïve transgenic mice with fluorescently labeled microglia (CX3CR1-eGFP) or with fluorescently tagged MOP receptors (MOP-mCherry) (92) (Table 2).
In contrast, several other studied have identified MOP receptors in human and rodent microglia (Table 3). Using qPCR, very low MOP receptor mRNA levels were found in microglia isolated from nucleus accumbens of rats acutely treated with vehicle or morphine (94). Transcriptomic analysis revealed MOP receptor mRNA in microglia in cerebral cortex of humans with no pain history, and in various brain areas and spinal cord of naïve mice or rats. Using immunofluorescence in transgenic mice expressing MOP receptors in microglia (CX3CR1-eGFP–MOP-mCherry), the percentage of MOP receptor-positive microglial cells ranged between 35 and 52% in brain, and between 37 and 42% in the spinal cord. The presence of MOP receptor protein in Golgi apparatus suggested that the receptors might be synthetized by microglia (100). Utilizing MOP-mCherry mice and double labeling in wild-type mice using astrocyte marker and MOP receptor antibodies (whose staining specificity was confirmed in MOP receptor knockout mice), MOP receptor protein was detected in astrocytes in various brain regions (107). Additional studies detected MOP receptor protein in naïve rat and mouse microglia or astrocytes in the spinal cord, or in mouse brain astrocytes and oligodendrocytes using antibody-based double labeling of the glial cell markers and MOP receptors, but the staining specificity of antibodies was not convincingly verified or not tested at all (104–106, 108).
Only a few studies assessed DOP, KOP, and NOP receptors in glia. No DOP receptor mRNA and low levels of KOP receptor mRNA were detected by qPCR in microglia and astrocytes isolated from nucleus accumbens of rats acutely injected with vehicle or morphine (94). NOP receptor mRNA was found in microglia and astrocytes in adult rat brain (119) or in astrocytes in rat brain until the third postnatal week, and in fetal human brain (120) by in situ hybridization for NOP receptor mRNA combined with immunofluorescent staining of microglia and astrocyte markers. Proteins of DOP, KOP, and NOP receptors were detected in astrocytes or oligodendrocytes in mouse or rat brain, but the staining specificity of antibodies was not unequivocally proven or not tested (108, 120).
Together, although there is still a controversy (Table 2), MOP receptors might be expressed in microglia and astrocytes, but more well-controlled studies are needed to verify the presence of DOP, KOP, and NOP receptors in native glia (Table 3).
Modulation of Pain by Opioid Receptors in Immune and Glial Cells
Immune Cells
A recent study expands the classical model of neuronal opioid receptor-mediated analgesia by showing the contribution of MOP, DOP, and KOP receptors in immune cells to the amelioration of pain (99). The activation of leukocyte opioid receptors led to the secretion of opioid peptides (β-endorphin, Met-enkephalin and dynorphin A 1-17), which subsequently acted at peripheral neuronal opioid receptors in injured tissue, and relieved pain (Figure 1B). Specifically, in a mouse model of the sciatic nerve injury, exogenous agonists selective at MOP (DAMGO), DOP (DPDPE), and KOP receptors (U50,488; Table 1) inhibited mechanical hypersensitivity following injection at the damaged nerve infiltrated by immune cells (neutrophils, macrophages, T lymphocytes). The analgesia was attenuated by opioid peptide antibodies injected at the injured nerve or by leukocyte depletion in wild-type mice. This effect was also diminished in mice lacking opioid peptides (β-endorphin-, PENK-, PDYN-knockout) compared to wild-type mice. This decrease in analgesia was restored by the transfer of wild-type, but not opioid receptor-lacking leukocytes (from MOP, DOP, or KOP receptor knockout mice). Ex vivo, exogenous opioids triggered the release of opioid peptides from immune cells isolated from damaged nerves of wild-type mice, measured by immunoassays. The release was dependent on Gαi/o and Gβγ proteins, phospholipase C (PLC), inositol 1,4,5-trisphosphate (IP3) receptors and intracellular Ca2+. This exogenous opioid-induced secretion of opioid peptides did not occur in immune cells isolated from nerves of mice lacking opioid peptides or receptors, which confirmed the specificity of opioid peptide antibodies used in immunoassays. Importantly, the opioid receptor-coupled intracellular Ca2+ pathway in immune cells mediated analgesia in vivo (99). Together, in addition to opioid receptors on peripheral sensory neurons [e.g., (99, 126–130)], analgesia can be mediated by MOP, DOP, and KOP receptors in immune cells (99). In contrast to the conventional action of neuronal opioid receptors (i.e., the inhibition of the release of pain-inducing mediators; analyzed in the introduction), analgesia mediated by leukocyte opioid receptors involves the secretion of pain-inhibiting opioid peptides (99). These effects may explain the enhanced analgesia of intra-articular morphine in patients with synovial tissue infiltrated by immune cells, following knee surgery (131). There are currently no data on the modulation of pain by leukocyte NOP receptors.
Glia
The actions of opioids on glial cells are typically discussed in relation to analgesic tolerance and paradoxical hyperalgesia termed opioid-induced hyperalgesia (OIH). Analgesic tolerance represents a progressive decrease of analgesia with prolonged agonist use or the need to increase the agonist dose to maintain analgesia. The OIH is usually described as hypersensitivity to painful stimuli upon chronic opioid use [reviewed by (132, 133)]. Nevertheless, since the abrupt discontinuation of prolonged opioid use can result in a withdrawal syndrome, including enhanced pain, the OIH may in fact represent the opioid withdrawal-induced hyperalgesia [reviewed by (134)]. The majority of studies have focused on the effects of morphine and on MOP receptors, but only a few directly addressed the microglia following chronic morphine treatment, and showed that tolerance and/or OIH were reduced by microglia depletion (105, 135) (Figure 1C). The former study proposed that morphine activated MOP receptors on spinal cord microglia to increase the expression of purinergic P2X4 receptors, which triggered the (MOP receptor-independent) release of brain-derived neurotrophic factor (BDNF) from microglia. The secreted BDNF induced downregulation of the K+-Cl− co-transporter KCC2 in GABAergic neurons, which resulted in their disinhibition. These actions were implied to mediate OIH, but not tolerance (105). Hayashi et al. (135) suggested that both tolerance and OIH involved microglia MOP receptor-induced secretion of arachidonic acid (AA) and subsequent activation of microglial large conductance Ca2+-activated K+ (BK) channels in the spinal cord. Several other studies showed the correlation between tolerance or OIH and the involvement of spinal cord microglia and/or astrocytes in morphine-treated animals. These observations were based on the increased glia numbers or elevated expression of nuclear factors and protein kinases in these glial cells (95, 136–138). Similar indirect effects were reported for other opioids acting at MOP receptors, including remifentanil, fentanyl and buprenorphine (139–141). Agonists of DOP and KOP receptors have not been tested. One study found elevated expression of astrocytes, but not microglia, in the spinal cord following N/OFQ treatment (142).
Nevertheless, it is unclear how opioids or N/OFQ would affect the glia, since the expression of opioid receptors in native glia is still debatable (see above), most of those studies did not directly examine the involvement of glial MOP or NOP receptors, OIH was observed in triple MOP, DOP, and KOP receptor knockout mice (143, 144), and the effects of morphine on toll-like receptor 4 in glia remain controversial [reviewed by (133)]. Furthermore, except for Chang et al. (141) who used postoperative pain model, all other studies exclusively tested naïve mice or rats. Additionally, opioids were used at unconventional ultra-low doses or high doses which exceeded the analgesic doses used in pathological pain models, they were sometimes injected repetitively every few minutes, and hyperalgesia was measured 12 h, 24 h or 4 days following the last dose, which indicates opioid withdrawal-induced hyperalgesia (95, 105, 135–142). Therefore, these effects can be viewed in the context of dependence rather than pain management, which is in line with clinical findings [reviewed by (134, 145)]. It is thus essential to examine actions of opioids on glia in models of pathological pain using analgesia-relevant paradigms. Accordingly, clinical studies suggested that OIH may not be a significant concern if opioids are used at regular doses in pathological pain conditions, and when their use is discontinued gradually (146, 147).
Opioid Peptides and N/OFQ in Immune and Glial Cells
Immune Cells
Immune cells also contain endogenous ligands of opioid receptors. The opioid peptide-containing leukocytes have been extensively investigated over the last decades [reviewed by (58, 148, 149)] and will only be briefly addressed here. Transcripts of POMC, PENK and PDYN, as well as enzymes required for POMC and PENK processing are expressed in T and B lymphocytes, macrophages or granulocytes in peripheral inflamed tissue in rats and mice (150–154). Consequently, the corresponding opioid peptides β-endorphin, enkephalins and dynorphin A 1-17 were detected in various immune cells from blood, lymph nodes or peripheral injured tissue in rats, mice and humans (99, 155–158). Opioid peptide-containing immune cells extravasate using adhesion molecules (P- and E-selectins, integrins α4 and β2, intercellular adhesion molecule-1) and chemokines (CXCL1, CXCL2/3) to accumulate in damaged tissue. Subsequently, the leukocytes secrete opioid peptides spontaneously or in response to stressful stimuli (experimental stress, surgery) and releasing agents, such as corticotropin-releasing factor, IL-1β, chemokines CXCL1, and CXCL2/3, noradrenaline, mycobacteria, and exogenous opioids. The released opioid peptides activate opioid receptors on peripheral sensory neurons and locally inhibit pain. Such analgesic actions have been demonstrated in rodent models of somatic and visceral inflammatory, neuropathic and bone cancer pain (99, 156, 159–170), as well as in patients with arthritis undergoing knee surgery (171–173). Notably, due to the continuous presence of immune cell-derived opioid peptides and enhanced MOP receptor recycling, the analgesic tolerance at peripheral MOP receptors in inflamed tissue is greatly reduced, in animals and humans (131, 174).
The mRNA of the N/OFQ precursor, ppN/OFQ, was detected in porcine splenocytes, blood granulocytes and eosinophils from healthy volunteers and patients with sepsis or asthma (117, 118, 175). Additionally, the ppN/OFQ mRNA was found in blood neutrophils, monocytes and lymphocytes from healthy donors, and neutrophils secreted the N/OFQ upon degranulation ex vivo (176). However, the functional relevance of immune cell-derived N/OFQ in vivo has not been elucidated.
Glia
There are numerous studies reporting expression of opioid peptides or their precursors in cultured glia [reviewed by (177)], but very few examined native tissue. The laboratory of Wang suggested analgesic effects of spinal cord microglia-derived β-endorphin and dynorphin A in rat models of inflammatory, neuropathic and bone cancer pain. However, they examined opioids in cultured microglia or in the spinal cord homogenates (178–180). Only in one study, β-endorphin was shown in spinal cord microglia ex vivo, but the staining specificity of the antibody was not tested (179). Therefore, the conclusive data on the expression of opioid peptides in native glia and their contribution to pain inhibition are still needed. The mRNA and protein of PENK were detected in cerebellum astrocytes in young rats (181), whereas N/OFQ was found in astrocytes of postnatal rat and fetal human brains (120), suggesting their role in brain development. The PDYN mRNA and protein were shown in astrocytes in human cerebral cortex, but their biological significance was not addressed (182).
Collectively, there is convincing evidence of analgesic actions mediated by immune cell-derived opioid peptides in animal models and in humans. The role of immune cell-derived N/OFQ and glia-derived opioids and N/OFQ in the context of pain is yet to be elucidated.
Conclusions
The classical opioid receptors MOP, DOP, and KOP, and their endogenous ligands opioid peptides are expressed in immune cells accumulating in peripheral inflamed tissue. Activation of all three leukocyte opioid receptors by exogenous opioids has been shown to release opioid peptides, which acted at peripheral neuronal receptors to diminish pain. This has wide clinical implications, since most painful conditions are associated with immune response, including inflammatory neuropathies, arthritis, cancer and postoperative pain. Therefore, the broad-spectrum inhibition of immune responses should be avoided, as this may exacerbate pain and diminish exogenous opioid analgesia. Furthermore, as these actions occur in peripheral tissue, the detrimental side effects resulting from the activation of MOP, DOP, and KOP receptors in the brain are precluded. It will be interesting to find out whether this can also apply to NOP receptors and N/OFQ. First, however, the involvement of immune cell NOP receptors and N/OFQ in pain modulation needs to be shown. The widely discussed OIH is often linked to the actions of opioids (mostly morphine) via glia (primarily microglia, but also astrocytes). Nevertheless, even if these effects are mediated (indirectly or directly) via glial MOP receptors, they seem to be related to dependence/withdrawal rather than pain treatment, in line with clinical findings. More well-controlled studies are needed to verify the presence of DOP, KOP, and NOP receptors, opioid peptides and N/OFQ in native glia, and to elucidate their role in vivo. Considering that there is strong evidence of discrepancies between in vivo and in vitro conditions, which is particularly relevant to the immune system, examination of native cells and tissue, without culturing, is preferable whenever possible. Factors that can influence the expression of the opioid system in immune and glial cells, and that may contribute to the inconsistencies among the studies include the examined cell population (e.g., lymphocytes, granulocytes, monocytes, macrophages, microglia or astrocytes), their subpopulations (e.g., T or B lymphocytes, T helper 1 or T helper 2 lymphocytes, M1 or M2 macrophages or microglia), the tissue they originate from, the in vivo physiological vs. pathological conditions, type and duration of the pathological state, and in vivo pharmacological treatments. Furthermore, methodological procedures need to be carefully designed, including protocols to obtain sufficient number of cells, verification of cell viability, the isolation techniques that enable high RNA and protein yields without contaminants (e.g., DNA contamination, remaining sample preparation reagents, excessive protein amounts), optimal design of PCR primers and probes for efficient qPCR, and the use of stringent controls to avoid false positive or negative results (e.g., no-RT controls for qPCR, antibody staining specificity controls for immunostaining) [(183); Celik and Machelska, submitted].
Author Contributions
HM conceptualized and wrote the manuscript with the input from MC. MC prepared the figure. HM and MC approved the final version of the manuscript.
Funding
The authors were supported by grants from the Deutsche Forschungsgemeinschaft (MA 2437/4-1, MA 6432/2-1) and the Bundesministerium für Bildung und Forschung (01EC1403E).
Conflict of Interest
The authors declare that the research was conducted in the absence of any commercial or financial relationships that could be construed as a potential conflict of interest.
References
1. Cox BM, Christie MJ, Devi L, Toll L, Traynor JR. Challenges for opioid receptor nomenclature: IUPHAR Review 9. Br J Pharmacol. (2015) 172:317–23. doi: 10.1111/bph.12612
2. Evans CJ, Keith DE Jr, Morrison H, Magendzo K, Edwards RH. Cloning of a delta opioid receptor by functional expression. Science. (1992) 258:1952–5. doi: 10.1126/science.1335167
3. Simonin F, Gavériaux-Ruff C, Befort K, Matthes H, Lannes B, Micheletti G, et al. kappa-Opioid receptor in humans: cDNA and genomic cloning, chromosomal assignment, functional expression, pharmacology, and expression pattern in the central nervous system. Proc Natl Acad Sci USA. (1995) 92:7006–10. doi: 10.1073/pnas.92.15.7006
4. Kieffer BL, Befort K, Gaveriaux-Ruff C, Hirth CG. The delta-opioid receptor: isolation of a cDNA by expression cloning and pharmacological characterization. Proc Natl Acad Sci USA. (1992) 89:12048–52. doi: 10.1073/pnas.89.24.12048
5. Mestek A, Hurley JH, Bye LS, Campbell AD, Chen Y, Tian M, et al. The human mu opioid receptor: modulation of functional desensitization by calcium/calmodulin-dependent protein kinase and protein kinase C. J Neurosci. (1995) 15:2396–406. doi: 10.1523/JNEUROSCI.15-03-02396.1995
6. Mogil JS, Pasternak GW. The molecular and behavioral pharmacology of the orphanin FQ/nociceptin peptide and receptor family. Pharmacol Rev. (2001) 53:381–415.
7. Granier S, Manglik A, Kruse AC, Kobilka TS, Thian FS, Weis WI, et al. Structure of the δ-opioid receptor bound to naltrindole. Nature. (2012) 485:400–4. doi: 10.1038/nature11111
8. Thompson AA, Liu W, Chun E, Katritch V, Wu H, Vardy E, et al. Structure of the nociceptin/orphanin FQ receptor in complex with a peptide mimetic. Nature. (2012) 485:395–9. doi: 10.1038/nature11085
9. Huang W, Manglik A, Venkatakrishnan AJ, Laeremans T, Feinberg EN, Sanborn AL, et al. Structural insights into μ-opioid receptor activation. Nature. (2015) 524:315–21. doi: 10.1038/nature14886
10. Che T, Majumdar S, Zaidi SA, Ondachi P, McCorvy JD, Wang S, et al. Structure of the nanobody-stabilized active state of the kappa opioid receptor. Cell. (2018) 172:55–67.e15. doi: 10.1016/j.cell.2017.12.011
11. Nakanishi S, Inoue A, Kita T, Nakamura M, Chang AC, Cohen SN, et al. Nucleotide sequence of cloned cDNA for bovine corticotropin-beta-lipotropin precursor. Nature. (1979) 278:423–7. doi: 10.1038/278423a0
12. Li CH, Chung D, Doneen BA. Isolation, characterization and opiate activity of β-endorphin from human pituitary glands. Biochem Biophys Res Commun. (1976) 72:1542–7. doi: 10.1016/S0006-291X(76)80189-1
13. Hughes J, Smith TW, Kosterlitz HW, Fothergill LA, Morgan BA, Morris HR. Identification of two related pentapeptides from the brain with potent opiate agonist activity. Nature. (1975) 258:577–80. doi: 10.1038/258577a0
14. Noda M, Teranishi Y, Takahashi H, Toyosato M, Notake M, Nakanishi S, et al. Isolation and structural organization of the human preproenkephalin gene. Nature. (1982) 297:431–4. doi: 10.1038/297431a0
15. Kakidani H, Furutani Y, Takahashi H, Noda M, Morimoto Y, Hirose T, et al. Cloning and sequence analysis of cDNA for porcine beta-neo-endorphin/dynorphin precursor. Nature. (1982) 298:245–9. doi: 10.1038/298245a0
16. Goldstein A, Fischli W, Lowney LI, Hunkapiller M, Hood L. Porcine pituitary dynorphin: complete amino acid sequence of the biologically active heptadecapeptide. Proc Natl Acad Sci USA. (1981) 78:7219–23. doi: 10.1073/pnas.78.11.7219
17. Kangawa K, Minamino N, Chino N, Sakakibara S, Matsuo H. The complete amino acid sequence of alpha-neo-endorphin. Biochem Biophys Res Commun. (1981) 99:871–8. doi: 10.1016/0006-291X(81)91244-4
18. Zadina JE, Hackler L, Ge LJ, Kastin AJ. A potent and selective endogenous agonist for the mu-opiate receptor. Nature. (1997) 386:499–502. doi: 10.1038/386499a0
19. Akil H, Owens C, Gutstein H, Taylor L, Curran E, Watson S. Endogenous opioids: overview and current issues. Drug Alcohol Depend. (1998) 51:127–40. doi: 10.1016/S0376-8716(98)00071-4
20. Meunier JC, Mollereau C, Toll L, Suaudeau C, Moisand C, Alvinerie P, et al. Isolation and structure of the endogenous agonist of opioid receptor-like ORL1 receptor. Nature. (1995) 377:532–5. doi: 10.1038/377532a0
21. Reinscheid RK, Nothacker HP, Bourson A, Ardati A, Henningsen RA, Bunzow JR, et al. Orphanin FQ: a neuropeptide that activates an opioidlike G protein-coupled receptor. Science. (1995) 270:792–4. doi: 10.1126/science.270.5237.792
22. Pfeiffer A, Brantl V, Herz A, Emrich HM. Psychotomimesis mediated by kappa opiate receptors. Science. (1986) 233:774–6. doi: 10.1126/science.3016896
23. Rimoy GH, Wright DM, Bhaskar NK, Rubin PC. The cardiovascular and central nervous system effects in the human of U-62066E. A selective opioid receptor agonist. Eur J Clin Pharmacol. (1994) 46:203–7. doi: 10.1007/BF00192549
24. Walsh SL, Strain EC, Abreu ME, Bigelow GE. Enadoline, a selective kappa opioid agonist: comparison with butorphanol and hydromorphone in humans. Psychopharmacology. (2001) 157:151–62. doi: 10.1007/s002130100788
25. Standifer KM, Pasternak GW. G proteins and opioid receptor-mediated signalling. Cell Signal. (1997) 9:237–48. doi: 10.1016/S0898-6568(96)00174-X
26. Schröder W, Lambert DG, Ko MC, Koch T. Functional plasticity of the N/OFQ-NOP receptor system determines analgesic properties of NOP receptor agonists. Br J Pharmacol. (2014) 171:3777–800. doi: 10.1111/bph.12744
27. Vetter I, Wyse BD, Monteith GR, Roberts-Thomson SJ, Cabot PJ. The μ opioid agonist morphine modulates potentiation of capsaicin-evoked TRPV1 responses through a cyclic AMP-dependent protein kinase A pathway. Mol Pain. (2006) 2:22. doi: 10.1186/1744-8069-2-22
28. Endres-Becker J, Heppenstall PA, Mousa SA, Labuz D, Oksche A, Schäfer M, et al. Mu-opioid receptor activation modulates transient receptor potential vanilloid 1 (TRPV1) currents in sensory neurons in a model of inflammatory pain. Mol Pharmacol. (2007) 71:12–8. doi: 10.1124/mol.106.026740
29. Cai Q, Qiu CY, Qiu F, Liu TT, Qu ZW, Liu YM, et al. Morphine inhibits acid-sensing ion channel currents in rat dorsal root ganglion neurons. Brain Res. (2014) 1554:12–20. doi: 10.1016/j.brainres.2014.01.042
30. Gold MS, Levine JD. DAMGO inhibits prostaglandin E2-induced potentiation of a TTX-resistant Na+ current in rat sensory neurons in vitro. Neurosci Lett. (1996) 212:83–6. doi: 10.1016/0304-3940(96)12791-9
31. Ingram SL, Williams JT. Opioid inhibition of Ih via adenylyl cyclase. Neuron. (1994) 13:179–86. doi: 10.1016/0896-6273(94)90468-5
32. Schroeder JE, Fischbach PS, Zheng D, McCleskey EW. Activation of mu opioid receptors inhibits transient high- and low-threshold Ca2+ currents, but spares a sustained current. Neuron. (1991) 6:13–20. doi: 10.1016/0896-6273(91)90117-I
33. Borgland SL, Connor M, Christie MJ. Nociceptin inhibits calcium channel currents in a subpopulation of small nociceptive trigeminal ganglion neurons in mouse. J Physiol. (2001) 536:35–47. doi: 10.1111/j.1469-7793.2001.t01-1-00035.x
34. Acosta CG, López HS. δ opioid receptor modulation of several voltage-dependent Ca(2+) currents in rat sensory neurons. J Neurosci. (1999) 19:8337–48. doi: 10.1523/JNEUROSCI.19-19-08337.1999
35. Grudt TJ, Williams JT. κ-Opioid receptors also increase potassium conductance. Proc Natl Acad Sci USA. (1993) 90:11429–32. doi: 10.1073/pnas.90.23.11429
36. Vaughan CW, Christie MJ. Increase by the ORL1 receptor (opioid receptor-like1) ligand, nociceptin, of inwardly rectifying K conductance in dorsal raphe nucleus neurones. Br J Pharmacol. (1996) 117:1609–11. doi: 10.1111/j.1476-5381.1996.tb15329.x
37. Schneider SP, Eckert WA III, Light AR. Opioid-activated postsynaptic, inward rectifying potassium currents in whole cell recordings in substantia gelatinosa neurons. J Neurophysiol. (1998) 80:2954–62. doi: 10.1152/jn.1998.80.6.2954
38. Luo C, Kumamoto E, Furue H, Yoshimura M. Nociceptin-induced outward current in substantia gelatinosa neurones of the adult rat spinal cord. Neuroscience. (2001) 108:323–30. doi: 10.1016/s0306-4522(01)00398-0
39. Marker CL, Luján R, Loh HH, Wickman K. Spinal G-protein-gated potassium channels contribute in a dose-dependent manner to the analgesic effect of mu- and delta- but not kappa-opioids. J Neurosci. (2005) 25:3551–9. doi: 10.1523/JNEUROSCI.4899-04.2005
40. Cunha TM, Roman-Campos D, Lotufo CM, Duarte HL, Souza GR, Verri WA Jr, et al. Morphine peripheral analgesia depends on activation of the PI3Kgamma/AKT/nNOS/NO/KATP signaling pathway. Proc Natl Acad Sci USA. (2010) 107:4442–7. doi: 10.1073/pnas.0914733107
41. Ocaña M, Cendán CM, Cobos EJ, Entrena JM, Baeyens JM. Potassium channels and pain: present realities and future opportunities. Eur J Pharmacol. (2004) 500:203–19. doi: 10.1016/j.ejphar.2004.07.026
42. Dembla S, Behrendt M, Mohr F, Goecke C, Sondermann J, Schneider FM, et al. Anti-nociceptive action of peripheral mu-opioid receptors by G-beta-gamma protein-mediated inhibition of TRPM3 channels. Elife. (2017) 6:e26280. doi: 10.7554/eLife.26280
43. Yaksh TL, Jessell TM, Gamse R, Mudge AW, Leeman SE. Intrathecal morphine inhibits substance P release from mammalian spinal cord in vivo. Nature. (1980) 286:155–6. doi: 10.1038/286155a0
44. Hirota N, Kuraishi Y, Hino Y, Sato Y, Satoh M, Takagi H. Met-enkephalin and morphine but not dynorphin inhibit the noxious stimuli-induced release of substance P from rabbit dorsal horn in situ. Neuropharmacology. (1985) 24:567–70. doi: 10.1016/0028-3908(85)90065-6
45. Helyes Z, Nemeth J, Pinter E, Szolcsanyi J. Inhibition by nociceptin of neurogenic inflammation and the release of SP and CGRP from sensory nerve terminals. Br J Pharmacol. (1997) 121:613–5. doi: 10.1038/sj.bjp.0701209
46. Kondo I, Marvizon JC, Song B, Salgado F, Codeluppi S, Hua XY, et al. Inhibition by spinal μ- and δ-opioid agonists of afferent-evoked substance P release. J Neurosci. (2005) 25:3651–60. doi: 10.1523/JNEUROSCI.0252-05.2005
47. Beaudry H, Dubois D, Gendron L. Activation of spinal μ- and δ opioid receptors potently inhibits substance P release induced by peripheral noxious stimuli. J Neurosci. (2011) 31:13068–77. doi: 10.1523/JNEUROSCI.1817-11.2011
48. Yaksh TL. Substance P release from knee joint afferent terminals: modulation by opioids. Brain Res. (1988) 458:319–24. doi: 10.1016/0006-8993(88)90474-X
49. Khasabova IA, Harding-Rose C, Simone DA, Seybold VS. Differential effects of CB1 and opioid agonists on two populations of adult rat dorsal root ganglion neurons. J Neurosci. (2004) 24:1744–53. doi: 10.1523/JNEUROSCI.4298-03.2004
50. Patwardhan AM, Berg KA, Akopain AN, Jeske NA, Gamper N, Clarke WP, et al. Bradykinin-induced functional competence and trafficking of the δ-opioid receptor in trigeminal nociceptors. J Neurosci. (2005) 25:8825–32. doi: 10.1523/JNEUROSCI.0160-05.2005
51. Börzsei R, Pozsgai G, Bagoly T, Elekes K, Pintér E, Szolcsányi J, et al. Inhibitory action of endomorphin-1 on sensory neuropeptide release and neurogenic inflammation in rats and mice. Neuroscience. (2008) 152:82–8. doi: 10.1016/j.neuroscience.2007.12.005
52. Ueda M, Sugimoto K, Oyama T, Kuraishi Y, Satoh M. Opioidergic inhibition of capsaicin-evoked release of glutamate from rat spinal dorsal horn slices. Neuropharmacology. (1995) 34:303–8. doi: 10.1016/0028-3908(94)00160-T
53. Jin YH, Nishioka H, Wakabayashi K, Fujita T, Yonehara N. Effect of morphine on the release of excitatory amino acids in the rat hind instep: pain is modulated by the interaction between the peripheral opioid and glutamate systems. Neuroscience. (2006) 138:1329–39. doi: 10.1016/j.neuroscience.2005.12.049
54. Takagi H, Shiomi H, Kuraishi Y, Fukui K, Ueda H. Pain and the bulbospinal noradrenergic system: pain-induced increase in normetanephrine content in the spinal cord and its modification by morphine. Eur J Pharmacol. (1979) 54:99–107. doi: 10.1016/0014-2999(79)90412-6
55. Bouaziz H, Tong C, Yoon Y, Hood DD, Eisenach JC. Intravenous opioids stimulate norepinephrine and acetylcholine release in spinal cord dorsal horn. Systematic studies in sheep and an observation in a human. Anesthesiology. (1996) 84:143–54. doi: 10.1097/00000542-199601000-00017
56. Yaksh TL. Pharmacology and mechanisms of opioid analgesic activity. Acta Anaesthesiol Scand. (1997) 41:94–111. doi: 10.1111/j.1399-6576.1997.tb04623.x
57. Machelska H, Celik MÖ. Advances in achieving opioid analgesia without side effects. Front Pharmacol. (2018) 9:1388. doi: 10.3389/fphar.2018.01388
58. Stein C, Machelska H. Modulation of peripheral sensory neurons by the immune system: implications for pain therapy. Pharmacol Rev. (2011) 63:860–81. doi: 10.1124/pr.110.003145
59. Millan MJ. Descending control of pain. Prog Neurobiol. (2002) 66:355–474. doi: 10.1016/s0301-0082(02)00009-6
60. Ossipov MH, Dussor GO, Porreca F. Central modulation of pain. J Clin Invest. (2010) 120:3779–87. doi: 10.1172/JCI43766
61. Pan Z, Hirakawa N, Fields HL. A cellular mechanism for the bidirectional pain-modulating actions of orphanin FQ/nociceptin. Neuron. (2000) 26:515–22. doi: 10.1016/S0896-6273(00)81183-6
62. Chan JS, Yung LY, Lee JW, Wu YL, Pei G, Wong YH. Pertussis toxin-insensitive signaling of the ORL1 receptor: coupling to Gz and G16 proteins. J Neurochem. (1998) 71:2203–10. doi: 10.1046/j.1471-4159.1998.71052203.x
63. Klukovits A, Tekes K, Gündüz Cinar O, Benyhe S, Borsodi A, Deák BH, et al. Nociceptin inhibits uterine contractions in term-pregnant rats by signaling through multiple pathways. Biol Reprod. (2010) 83:36–41. doi: 10.1095/biolreprod.109.082222
64. Kapusta DR. Opioid mechanisms controlling renal function. Clin Exp Pharmacol Physiol. (1995) 22:891–902. doi: 10.1111/j.1440-1681.1995.tb02324.x
65. Lambert DG. The nociceptin/orphanin FQ receptor: a target with broad therapeutic potential. Nat Rev Drug Discov. (2008) 7:694–710. doi: 10.1038/nrd2572
66. Imam MZ, Kuo A, Ghassabian S, Smith MT. Progress in understanding mechanisms of opioid-induced gastrointestinal adverse effects and respiratory depression. Neuropharmacology. (2017) 131:238–55. doi: 10.1016/j.neuropharm.2017.12.032
67. Darcq E, Kieffer BL. Opioid receptors: drivers to addiction? Nat Rev Neurosci. (2018) 19:499–514. doi: 10.1038/s41583-018-0028-x
68. Hunter P. New therapies to relieve pain: The search for more efficient and safer alternatives to opioid pain killers. EMBO Rep. (2018) 19:e46925. doi: 10.15252/embr.201846925
69. Negus SS, Freeman KB. Abuse potential of biased mu opioid receptor agonists. Trends Pharmacol Sci. (2018) 39:916–9. doi: 10.1016/j.tips.2018.08.007
70. Sacerdote P, Limiroli E, Gaspani L. Experimental evidence for immunomodulatory effects of opioids. Adv Exp Med Biol. (2003) 521:106–16.
71. Sharp BM. Multiple opioid receptors on immune cells modulate intracellular signaling. Brain Behav Immun. (2006) 20:9–14. doi: 10.1016/j.bbi.2005.02.002
72. Salzet M, Vieau D, Day R. Crosstalk between nervous and immune systems through the animal kingdom: focus on opioids. Trends Neurosci. (2000) 23:550–5. doi: 10.1016/S0166-2236(00)01642-8
73. Welters ID. Is immunomodulation by opioid drugs of clinical relevance? Curr Opin Anaesthesiol. (2003) 16:509–13. doi: 10.1097/00001503-200310000-00011
74. Finley MJ, Happel CM, Kaminsky DE, Rogers TJ. Opioid and nociceptin receptors regulate cytokine and cytokine receptor expression. Cell Immunol. (2008) 252:146–54. doi: 10.1016/j.cellimm.2007.09.008
75. Al-Hashimi M, Scott SW, Thompson JP, Lambert DG. Opioids and immune modulation: more questions than answers. Br J Anaesth. (2013) 111:80–8. doi: 10.1093/bja/aet153
76. Ninković J, Roy S. Role of the mu-opioid receptor in opioid modulation of immune function. Amino Acids. (2013) 45:9–24. doi: 10.1007/s00726-011-1163-0
77. Plein LM, Rittner HL. Opioids and the immune system - friend or foe. Br J Pharmacol. (2018) 175:2717–25. doi: 10.1111/bph.13750
78. Sweitzer SM, Hickey WF, Rutkowski MD, Pahl JL, DeLeo JA. Focal peripheral nerve injury induces leukocyte trafficking into the central nervous system: potential relationship to neuropathic pain. Pain. (2002) 100:163–70. doi: 10.1016/S0304-3959(02)00257-9
79. Costigan M, Moss A, Latremoliere A, Johnston C, Verma-Gandhu M, Herbert TA, et al. T-cell infiltration and signaling in the adult dorsal spinal cord is a major contributor to neuropathic pain-like hypersensitivity. J Neurosci. (2009) 29:14415–22. doi: 10.1523/JNEUROSCI.4569-09.2009
80. Sorge RE, Mapplebeck JC, Rosen S, Beggs S, Taves S, Alexander JK, et al. Different immune cells mediate mechanical pain hypersensitivity in male and female mice. Nat Neurosci. (2015) 18:1081–3. doi: 10.1038/nn.4053
81. Lopes DM, Malek N, Edye M, Jager SB, McMurray S, McMahon SB, et al. Sex differences in peripheral not central immune responses to pain-inducing injury. Sci Rep. (2017) 7:16460. doi: 10.1038/s41598-017-16664-z
82. Ji RR, Chamessian A, Zhang YQ. Pain regulation by non-neuronal cells and inflammation. Science. (2016) 354:572–7. doi: 10.1126/science.aaf8924
83. Allen NJ, Lyons DA. Glia as architects of central nervous system formation and function. Science. (2018) 362:181–5. doi: 10.1126/science.aat0473
84. Stiene-Martin A, Zhou R, Hauser KF. Regional, developmental, and cell cycle-dependent differences in μ, δ, and κ-opioid receptor expression among cultured mouse astrocytes. Glia. (1998) 22:249–59. doi: 10.1002/(SICI)1098-1136(199803)22:3<249::AID-GLIA4>3.0.CO;2-0
85. Sharp BM, Li MD, Matta SG, McAllen K, Shahabi NA. Expression of delta opioid receptors and transcripts by splenic T cells. Ann N Y Acad Sci. (2000) 917:764–70. doi: 10.1111/j.1749-6632.2000.tb05441.x
86. Beutner C, Linnartz-Gerlach B, Schmidt SV, Beyer M, Mallmann MR, Staratschek-Jox A, et al. Unique transcriptome signature of mouse microglia. Glia. (2013) 61:1429–42. doi: 10.1002/glia.22524
87. Bidlack JM, Khimich M, Parkhill AL, Sumagin S, Sun B, Tipton CM. Opioid receptors and signaling on cells from the immune system. J Neuroimmune Pharmacol. (2006) 1:260–9. doi: 10.1007/s11481-006-9026-2
88. Williams JP, Thompson JP, McDonald J, Barnes TA, Cote T, Rowbotham DJ, Lambert DG. Human peripheral blood mononuclear cells express nociceptin/orphanin FQ, but not mu, delta, or kappa opioid receptors. Anesth Analg. (2007) 105:998–1005. doi: 10.1213/01.ane.0000278865.11991.9d
89. Al-Hashimi M, McDonald J, Thompson JP, Lambert DG. Evidence for nociceptin/orphanin FQ (NOP) but not μ (MOP), δ (DOP) or κ (KOP) opioid receptor mRNA in whole human blood. Br J Anaesth. (2016) 116:423–9. doi: 10.1093/bja/aev540
90. Gaveriaux C, Peluso J, Simonin F, Laforet J, Kieffer B. Identification of κ- and δ-opioid receptor transcripts in immune cells. FEBS Lett. (1995) 369:272–6. doi: 10.1016/0014-5793(95)00766-3
91. Wick MJ, Minnerath SR, Roy S, Ramakrishnan S, Loh HH. Differential expression of opioid receptor genes in human lymphoid cell lines and peripheral blood lymphocytes. J Neuroimmunol. (1996) 64:29–36. doi: 10.1016/0165-5728(95)00144-1
92. Corder G, Tawfik VL, Wang D, Sypek EI, Low SA, Dickinson JR, et al. Loss of μ opioid receptor signaling in nociceptors, but not microglia, abrogates morphine tolerance without disrupting analgesia. Nat Med. (2017) 23:164–73. doi: 10.1038/nm.4262
93. Kao SC, Zhao X, Lee CY, Atianjoh FE, Gauda EB, Yaster M, et al. Absence of μ opioid receptor mRNA expression in astrocytes and microglia of rat spinal cord. Neuroreport. (2012) 23:378–84. doi: 10.1097/WNR.0b013e3283522e1b
94. Schwarz JM, Smith SH, Bilbo SD. FACS analysis of neuronal-glial interactions in the nucleus accumbens following morphine administration. Psychopharmacology. (2013) 230:525–35. doi: 10.1007/s00213-013-3180-z
95. Sanna MD, Ghelardini C, Galeotti N. Activation of JNK pathway in spinal astrocytes contributes to acute ultra-low-dose morphine thermal hyperalgesia. Pain. (2015) 156:1265–75. doi: 10.1097/j.pain.000000000000016
96. Chuang TK, Killam KF Jr, Chuang LF, Kung HF, Sheng WS, Chao CC, et al. Mu opioid receptor gene expression in immune cells. Biochem Biophys Res Commun. (1995) 216:922–30. doi: 10.1006/bbrc.1995.2709
97. Toskulkao T, Pornchai R, Akkarapatumwong V, Vatanatunyakum S, Govitrapong P. Alteration of lymphocyte opioid receptors in methadone maintenance subjects. Neurochem Int. (2010) 56:285–90. doi: 10.1016/j.neuint.2009.10.013
98. Sedqi M, Roy S, Ramakrishnan S, Elde R, Loh HH. Complementary DNA cloning of a μ-opioid receptor from rat peritoneal macrophages. Biochem Biophys Res Commun. (1995) 209:563–74. doi: 10.1006/bbrc.1995.1538
99. Celik MÖ, Labuz D, Henning K, Busch-Dienstfertig M, Gaveriaux-Ruff C, Kieffer BL, et al. Leukocyte opioid receptors mediate analgesia via Ca(2+)-regulated release of opioid peptides. Brain Behav Immun. (2016) 57:227–42. doi: 10.1016/j.bbi.2016.04.018
100. Maduna T, Audouard E, Dembélé D, Mouzaoui N, Reiss D, Massotte D, et al. Microglia express mu opioid receptor: insights from transcriptomics and fluorescent reporter mice. Front Psychiatry. (2019) 9:726. doi: 10.3389/fpsyt.2018.00726
101. Lopker A, Abood LG, Hoss W, Lionetti FJ. Stereoselective muscarinic acetylcholine and opiate receptors in human phagocytic leukocytes. Biochem Pharmacol. (1980) 29:1361–5. doi: 10.1016/0006-2952(80)90431-1
102. Stefano GB, Melchiorri P, Negri L, Hughes TK Jr, Scharrer B. [D-Ala2]deltorphin I binding and pharmacological evidence for a special subtype of delta opioid receptor on human and invertebrate immune cells. Proc Natl Acad Sci USA. (1992) 89:9316–20. doi: 10.1073/pnas.89.19.9316
103. Boyadjieva NI, Chaturvedi K, Poplawski MM, Sarkar DK. Opioid antagonist naltrexone disrupts feedback interaction between mu and delta opioid receptors in splenocytes to prevent alcohol inhibition of NK cell function. J Immunol. (2004) 173:42–9. doi: 10.4049/jimmunol.173.1.42
104. Horvath RJ, Romero-Sandoval EA, De Leo JA. Inhibition of microglial P2X4 receptors attenuates morphine tolerance, Iba1, GFAP and mu opioid receptor protein expression while enhancing perivascular microglial ED2. Pain. (2010) 150:401–13. doi: 10.1016/j.pain.2010.02.042
105. Ferrini F, Trang T, Mattioli TA, Laffray S, Del'Guidice T, Lorenzo LE, et al. Morphine hyperalgesia gated through microglia-mediated disruption of neuronal Cl? homeostasis. Nat Neurosci. (2013) 16:183–92. doi: 10.1038/nn.3295
106. Vacca V, Marinelli S, Luvisetto S, Pavone F. Botulinum toxin A increases analgesic effects of morphine, counters development of morphine tolerance and modulates glia activation and μ opioid receptor expression in neuropathic mice. Brain Behav Immun. (2013) 32:40–50. doi: 10.1016/j.bbi.2013.01.088
107. Nam MH, Han KS, Lee J, Bae JY, An H, Park S, et al. Expression of μ-opioid receptor in CA1 hippocampal astrocytes. Exp Neurobiol. (2018) 27:120–8. doi: 10.5607/en.2018.27.2.120
108. Stiene-Martin A, Knapp PE, Martin K, Gurwell JA, Ryan S, Thornton SR, et al. Opioid system diversity in developing neurons, astroglia, and oligodendroglia in the subventricular zone and striatum: impact on gliogenesis in vivo. Glia. (2001) 36:78–88. doi: 10.1002/glia.1097
109. Sharp BM, Shahabi N, McKean D, Li MD, McAllen K. Detection of basal levels and induction of delta opioid receptor mRNA in murine splenocytes. J Neuroimmunol. (1997) 78:198–202. doi: 10.1016/S0165-5728(97)00101-X
110. Jaume M, Laffont S, Chapey E, Blanpied C, Dietrich G. Opioid receptor blockade increases the number of lymphocytes without altering T cell response in draining lymph nodes in vivo. J Neuroimmunol. (2007) 188:95–102. doi: 10.1016/j.jneuroim.2007.06.013
111. Gavériaux-Ruff C, Peluso J, Befort K, Simonin F, Zilliox C, Kieffer BL. Detection of opioid receptor mRNA by RT-PCR reveals alternative splicing for the δ- and κ-opioid receptors. Brain Res Mol Brain Res. (1997) 48:298–304. doi: 10.1016/S0169-328X(97)00109-5
112. Gunji N, Nagashima M, Asano G, Yoshino S. Expression of κ-opioid receptor mRNA in human peripheral blood lymphocytes and the relationship between its expression and the inflammatory changes in rheumatoid arthritis. Rheumatol Int. (2000) 19:95–100. doi: 10.1007/s002960050110
113. Chuang LF, Chuang TK, Killam KF Jr, Qiu Q, Wang XR, Lin JJ, et al. Expression of κ opioid receptors in human and monkey lymphocytes. Biochem Biophys Res Commun. (1995) 209:1003–10. doi: 10.1006/bbrc.1995.1597
114. Ignatowski TA, Bidlack JM. Differential κ-opioid receptor expression on mouse lymphocytes at varying stages of maturation and on mouse macrophages after selective elicitation. J Pharmacol Exp Ther. (1999) 290:863–70.
115. Peluso J, LaForge KS, Matthes HW, Kreek MJ, Kieffer BL, Gavériaux-Ruff C. Distribution of nociceptin/orphanin FQ receptor transcript in human central nervous system and immune cells. J Neuroimmunol. (1998) 81:184–92. doi: 10.1016/S0165-5728(97)00178-1
116. Serhan CN, Fierro IM, Chiang N, Pouliot M. Cutting edge: nociceptin stimulates neutrophil chemotaxis and recruitment: inhibition by aspirin-triggered-15-epi-lipoxin A4. J Immunol. (2001) 166:3650–4. doi: 10.4049/jimmunol.166.6.3650
117. Thompson JP, Serrano-Gomez A, McDonald J, Ladak N, Bowrey S, Lambert DG. The Nociceptin/Orphanin FQ system is modulated in patients admitted to ICU with sepsis and after cardiopulmonary bypass. PLoS ONE. (2013) 8:e76682. doi: 10.1371/journal.pone.0076682
118. Singh SR, Sullo N, Matteis M, Spaziano G, McDonald J, Saunders R, et al. Nociceptin/orphanin FQ (N/OFQ) modulates immunopathology and airway hyperresponsiveness representing a novel target for the treatment of asthma. Br J Pharmacol. (2016) 173:1286–301. doi: 10.1111/bph.13416
119. Zhao H, Huang HW, Wu GC, Cao XD. Effect of orphanin FQ on interleukin-1beta mRNA transcripts in the rat CNS. Neuroscience. (2002) 114:1019–31. doi: 10.1016/S0306-4522(02)00233-6
120. Meyer LC, Paisley CE, Mohamed E, Bigbee JW, Kordula T, Richard H, et al. Novel role of the nociceptin system as a regulator of glutamate transporter expression in developing astrocytes. Glia. (2017) 65:2003–23. doi: 10.1002/glia.23210
121. Bird MF, Guerrini R, Willets JM, Thompson JP, Caló G, Lambert DG. Nociceptin/Orphanin FQ (N/OFQ) conjugated to ATTO594: a novel fluorescent probe for the N/OFQ (NOP) receptor. Br J Pharmacol. (2018) 175:4496–506. doi: 10.1111/bph.14504
122. Bénard A, Cavaillès P, Boué J, Chapey E, Bayry J, Blanpied C, et al. μ-Opioid receptor is induced by IL-13 within lymph nodes from patients with Sézary syndrome. J Invest Dermatol. (2010) 130:1337–44. doi: 10.1038/jid.2009.433
123. Scherrer G, Imamachi N, Cao YQ, Contet C, Mennicken F, O'Donnell D, et al. Dissociation of the opioid receptor mechanisms that control mechanical and heat pain. Cell. (2009) 137:1148–59. doi: 10.1016/j.cell.2009.04.019
124. Niwa H, Rowbotham DJ, Lambert DG. Evaluation of primary opioid receptor antibodies for use in western blotting. Br J Anaesth. (2012) 108:530–2. doi: 10.1093/bja/aes015
125. Schmidt Y, Gavériaux-Ruff C, Machelska H. μ-Opioid receptor antibody reveals tissue-dependent specific staining and increased neuronal μ-receptor immunoreactivity at the injured nerve trunk in mice. PLoS ONE. (2013) 8:e79099. doi: 10.1371/journal.pone.0079099
126. Obara I, Parkitna JR, Korostynski M, Makuch W, Kaminska D, Przewlocka B, et al. Local peripheral opioid effects and expression of opioid genes in the spinal cord and dorsal root ganglia in neuropathic and inflammatory pain. Pain. (2009) 141:283–91. doi: 10.1016/j.pain.2008.12.006
127. Hervera A, Negrete R, Leánez S, Martín-Campos JM, Pol O. Peripheral effects of morphine and expression of m-opioid receptors in the dorsal root ganglia during neuropathic pain: nitric oxide signaling. Mol Pain. (2011) 7:25. doi: 10.1186/1744-8069-7-25
128. Gaveriaux-Ruff C, Nozaki C, Nadal X, Hever XC, Weibel R, Matifas A, et al. Genetic ablation of delta opioid receptors in nociceptive sensory neurons increases chronic pain and abolishes opioid analgesia. Pain. (2011) 152:1238–48. doi: 10.1016/j.pain.2010.12.031
129. Keïta H, Kayser V, Guilbaud G. Antinociceptive effect of a kappa-opioid receptor agonist that minimally crosses the blood-brain barrier (ICI 204448) in a rat model of mononeuropathy. Eur J Pharmacol. (1995) 277:275–80. doi: 10.1016/0014-2999(95)00122-2
130. Labuz D, Machelska H. Stronger antinociceptive efficacy of opioids at the injured nerve trunk than at its peripheral terminals in neuropathic pain. J Pharmacol Exp Ther. (2013) 346:535–44. doi: 10.1124/jpet.113.205344
131. Stein C, Pflüger M, Yassouridis A, Hoelzl J, Lehrberger K, Welte C, et al. No tolerance to peripheral morphine analgesia in presence of opioid expression in inflamed synovia. J Clin Invest. (1996) 98:793–9. doi: 10.1172/JCI118852
132. Hutchinson MR, Shavit Y, Grace PM, Rice KC, Maier SF, Watkins LR. Exploring the neuroimmunopharmacology of opioids: an integrative review of mechanisms of central immune signaling and their implications for opioid analgesia. Pharmacol Rev. (2011) 63:772–810. doi: 10.1124/pr.110.004135
133. Roeckel LA, Le Coz GM, Gavériaux-Ruff C, Simonin F. Opioid-induced hyperalgesia: Cellular and molecular mechanisms. Neuroscience. (2016) 338:160–82. doi: 10.1016/j.neuroscience.2016.06.029
134. Angst MS, Clark JD. Opioid-induced hyperalgesia: a qualitative systematic review. Anesthesiology. (2006) 104:570–87. doi: 10.1097/00000542-200603000-00025
135. Hayashi Y, Morinaga S, Zhang J, Satoh Y, Meredith AL, Nakata T, et al. BK channels in microglia are required for morphine-induced hyperalgesia. Nat Commun. (2016) 7:11697. doi: 10.1038/ncomms11697
136. Tumati S, Largent-Milnes TM, Keresztes AI, Yamamoto T, Vanderah TW, Roeske WR, et al. Tachykinin NK1 receptor antagonist co-administration attenuates opioid withdrawal-mediated spinal microglia and astrocyte activation. Eur J Pharmacol. (2012) 684:64–70. doi: 10.1016/j.ejphar.2012.03.025
137. Bai L, Zhai C, Han K, Li Z, Qian J, Jing Y, et al. Toll-like receptor 4-mediated nuclear factor-κB activation in spinal cord contributes to chronic morphine-induced analgesic tolerance and hyperalgesia in rats. Neurosci Bull. (2014) 30:936–48. doi: 10.1007/s12264-014-1483-7
138. Jokinen V, Sidorova Y, Viisanen H, Suleymanova I, Tiilikainen H, Li Z, et al. Differential spinal and supraspinal activation of glia in a rat model of morphine tolerance. Neuroscience. (2018) 375:10–24. doi: 10.1016/j.neuroscience.2018.01.048
139. Gerhold KJ, Drdla-Schutting R, Honsek SD, Forsthuber L, Sandkühler J. Pronociceptive and antinociceptive effects of buprenorphine in the spinal cord dorsal horn cover a dose range of four orders of magnitude. J Neurosci. (2015) 35:9580–94. doi: 10.1523/JNEUROSCI.0731-14.2015
140. Ye L, Xiao L, Yang SY, Duan JJ, Chen Y, Cui Y, et al. Cathepsin S in the spinal microglia contributes to remifentanil-induced hyperalgesia in rats. Neuroscience. (2017) 344:265–75. doi: 10.1016/j.neuroscience.2016.12.030
141. Chang L, Ye F, Luo Q, Tao Y, Shu H. Increased hyperalgesia and proinflammatory cytokines in the spinal cord and dorsal root ganglion after surgery and/or fentanyl administration in rats. Anesth Analg. (2018) 126:289–97. doi: 10.1213/ANE.0000000000002601
142. Micheli L, Lucarini E, Corti F, Ciccocioppo R, Calò G, Rizzi A, et al. Involvement of the N/OFQ-NOP system in rat morphine antinociceptive tolerance: Are astrocytes the crossroad? Eur J Pharmacol. (2018) 823:79–86. doi: 10.1016/j.ejphar.2018.01.039
143. Waxman AR, Arout C, Caldwell M, Dahan A, Kest B. Acute and chronic fentanyl administration causes hyperalgesia independently of opioid receptor activity in mice. Neurosci Lett. (2009) 462:68–72. doi: 10.1016/j.neulet.2009.06.061
144. Juni A, Klein G, Pintar JE, Kest B. Nociception increases during opioid infusion in opioid receptor triple knock-out mice. Neuroscience. (2007) 147:439–44. doi: 10.1016/j.neuroscience.2007.04.030
145. Fletcher D, Martinez V. Opioid-induced hyperalgesia in patients after surgery: a systematic review and a meta-analysis. Br J Anaesth. (2014) 112:991–1004. doi: 10.1093/bja/aeu137
146. Chu LF, D'Arcy N, Brady C, Zamora AK, Young CA, Kim JE, et al. Analgesic tolerance without demonstrable opioid-induced hyperalgesia: a double-blinded, randomized, placebo-controlled trial of sustained-release morphine for treatment of chronic nonradicular low-back pain. Pain. (2012) 153:1583–92. doi: 10.1016/j.pain.2012.02.028
147. McPherson S, Lederhos Smith C, Dobscha SK, Morasco BJ, Demidenko MI, Meath THA, et al. Changes in pain intensity after discontinuation of long-term opioid therapy for chronic noncancer pain. Pain. (2018) 159:2097–104. doi: 10.1097/j.pain.0000000000001315
148. Stein C, Schäfer M, Machelska H. Attacking pain at its source: new perspectives on opioids. Nat Med. (2003) 9:1003–8. doi: 10.1038/nm908
149. Hua S. Neuroimmune interaction in the regulation of peripheral opioid-mediated analgesia in inflammation. Front Immunol. (2016) 7:293. doi: 10.3389/fimmu.2016.00293
150. Przewlocki R, Hassan AHS, Lason W, Epplen C, Herz A, Stein C. Gene expression and localization of opioid peptides in immune cells of inflamed tissue. Functional role in antinociception. Neuroscience. (1992) 48:491–500. doi: 10.1016/0306-4522(92)90509-Z
151. Mousa SA, Shakibaei M, Sitte N, Schäfer M, Stein C. Subcellular pathways of beta-endorphin synthesis, processing, and release from immunocytes in inflammatory pain. Endocrinology. (2004) 145:1331–41. doi: 10.1210/en.2003-1287
152. Chadzinska M, Starowicz K, Scislowska-Czarnecka A, Bilecki W, Pierzchala-Koziec K, Przewlocki R, et al. Morphine-induced changes in the activity of proopiomelanocortin and prodynorphin systems in zymosan-induced peritonitis in mice. Immunol Lett. (2005) 101:185–92. doi: 10.1016/j.imlet.2005.05.009
153. Sitte N, Busch M, Mousa SA, Labuz D, Rittner H, Gore C, et al. Lymphocytes upregulate signal sequence-encoding proopiomelanocortin mRNA and beta-endorphin during painful inflammation in vivo. J Neuroimmunol. (2007) 183:133–45. doi: 10.1016/j.jneuroim.2006.11.033
154. Maddila SC, Busch-Dienstfertig M, Stein C. B lymphocytes express Pomc mRNA, processing enzymes and β-endorphin in painful inflammation. J Neuroimmune Pharmacol. (2017) 12:180–6. doi: 10.1007/s11481-016-9715-4
155. Cabot PJ, Carter L, Schäfer M, Stein C. Methionine-enkephalin- and Dynorphin A-release from immune cells and control of inflammatory pain. Pain. (2001) 93:207–12. doi: 10.1016/S0304-3959(01)00322-0
156. Rittner HL, Brack A, Machelska H, Mousa SA, Bauer M, Schäfer M, et al. Opioid peptide-expressing leukocytes: identification, recruitment, and simultaneously increasing inhibition of inflammatory pain. Anesthesiology. (2001) 95:500–8. doi: 10.1097/00000542-200108000-00036
157. Mousa SA, Straub RH, Schäfer M, Stein C. Beta-endorphin, Met-enkephalin and corresponding opioid receptors within synovium of patients with joint trauma, osteoarthritis and rheumatoid arthritis. Ann Rheum Dis. (2007) 66:871–9. doi: 10.1136/ard.2006.067066
158. Schreiter A, Gore C, Labuz D, Fournie-Zaluski MC, Roques BP, Stein C, et al. Pain inhibition by blocking leukocytic and neuronal opioid peptidases in peripheral inflamed tissue. FASEB J. (2012) 26:5161–71. doi: 10.1096/fj.12-208678
159. Stein C, Gramsch C, Herz A. Intrinsic mechanisms of antinociception in inflammation: local opioid receptors and beta-endorphin. J Neurosci. (1990) 10:1292–8. doi: 10.1523/JNEUROSCI.10-04-01292.1990
160. Schäfer M, Carter L, Stein C. Interleukin-1 beta and corticotropin-releasing-factor inhibit pain by releasing opioids from immune cells in inflamed tissue. Proc Natl Acad Sci USA. (1994) 91:4219–23. doi: 10.1073/pnas.91.10.4219
161. Machelska H, Cabot PJ, Mousa SA, Zhang Q, Stein C. Pain control in inflammation governed by selectins. Nat Med. (1998) 4:1425–8. doi: 10.1038/4017
162. Machelska H, Mousa SA, Brack A, Schopohl JK, Rittner HL, Schäfer M, et al. Opioid control of inflammatory pain regulated by intercellular adhesion molecule-1. J Neurosci. (2002) 22:5588–96. doi: 10.1523/JNEUROSCI.22-13-05588.2002
163. Machelska H, Brack A, Mousa SA, Schopohl JK, Rittner HL, Schäfer M, et al. Selectins and integrins but not platelet-endothelial cell adhesion molecule-1 regulate opioid inhibition of inflammatory pain. Br J Pharmacol. (2004) 142:772–80. doi: 10.1038/sj.bjp.0705837
164. Rittner HL, Labuz D, Schaefer M, Mousa SA, Schulz S, Schäfer M, et al. Pain control by CXCR2 ligands through Ca2+-regulated release of opioid peptides from polymorphonuclear cells. FASEB J. (2006) 20:2627–9. doi: 10.1096/fj.06-6077fje
165. Rittner HL, Hackel D, Voigt P, Mousa S, Stolz A, Labuz D, et al. Mycobacteria attenuate nociceptive responses by formyl peptide receptor triggered opioid peptide release from neutrophils. PLoS Pathog. (2009) 5:e1000362. doi: 10.1371/journal.ppat.1000362
166. Binder W, Mousa SA, Sitte N, Kaiser M, Stein C, Schäfer M. Sympathetic activation triggers endogenous opioid release and analgesia within peripheral inflamed tissue. Eur J Neurosci. (2004) 20:92–100. doi: 10.1111/j.1460-9568.2004.03459.x
167. Baamonde A, Lastra A, Juarez L, Garcia-Suarez O, Meana A, Hidalgo A, et al. Endogenous β-endorphin induces thermal analgesia at the initial stages of a murine osteosarcoma. Peptides. (2006) 27:2778–85. doi: 10.1016/j.peptides.2006.07.004
168. Labuz D, Schmidt Y, Schreiter A, Rittner HL, Mousa SA, Machelska H. Immune cell-derived opioids protect against neuropathic pain in mice. J Clin Invest. (2009) 119:278–86. doi: 10.1172/JCI36246C1
169. Labuz D, Schreiter A, Schmidt Y, Brack A, Machelska H. T lymphocytes containing β-endorphin ameliorate mechanical hypersensitivity following nerve injury. Brain Behav Immun. (2010) 24:1045–53. doi: 10.1016/j.bbi.2010.04.001
170. Boué J, Basso L, Cenac N, Blanpied C, Rolli-Derkinderen M, Neunlist M, et al. Endogenous regulation of visceral pain via production of opioids by colitogenic CD4(+) T cells in mice. Gastroenterology. (2014) 146:166–75. doi: 10.1053/j.gastro.2013.09.020
171. Stein C, Hassan AHS, Lehrberger K, Giefing J, Yassouridis A. Local analgesic effect of endogenous opioid peptides. Lancet. (1993) 342:321–4. doi: 10.1016/0140-6736(93)91471-W
172. Kager I, Mousa SA, Sieper J, Stein C, Pipam W, Likar R. Blockade of intra-articular adrenergic receptors increases analgesic demands for pain relief after knee surgery. Rheumatol Int. (2011) 31:1299–306. doi: 10.1007/s00296-010-1489-z
173. Likar R, Mousa SA, Steinkellner H, Koppert W, Philippitsch G, Stein C, et al. Involvement of intra-articular corticotropin-releasing hormone in postoperative pain modulation. Clin J Pain. (2007) 23:136–42. doi: 10.1097/01.ajp.0000210954.93878.0d
174. Zöllner C, Mousa SA, Fischer O, Rittner HL, Shaqura M, Brack A, et al. Chronic morphine use does not induce peripheral tolerance in a rat model of inflammatory pain. J Clin Invest. (2008) 118:1065–73. doi: 10.1172/JCI25911
175. Pampusch MS, Serie JR, Osinski MA, Seybold VS, Murtaugh MP, Brown DR. Expression of nociceptin/OFQ receptor and prepro-nociceptin/OFQ in lymphoid tissues. Peptides. (2000) 21:1865–70. doi: 10.1016/S0196-9781(00)00332-6
176. Fiset ME, Gilbert C, Poubelle PE, Pouliot M. Human neutrophils as a source of nociceptin: a novel link between pain and inflammation. Biochemistry. (2003) 42:10498–505. doi: 10.1021/bi0300635
177. Hauser KF, Knapp PE. Opiate drugs with abuse liability hijack the endogenous opioid system to disrupt neuronal and glial maturation in the central nervous system. Front Pediatr. (2018) 5:294. doi: 10.3389/fped.2017.00294
178. Gong N, Xiao Q, Zhu B, Zhang CY, Wang YC, Fan H, et al. Activation of spinal glucagon-like peptide-1 receptors specifically suppresses pain hypersensitivity. J Neurosci. (2014) 34:5322–34. doi: 10.1523/JNEUROSCI.4703-13.2014
179. Wu HY, Mao XF, Tang XQ, Ali U, Apryani E, Liu H, et al. Spinal interleukin-10 produces antinociception in neuropathy through microglial β-endorphin expression, separated from antineuroinflammation. Brain Behav Immun. (2018) 73:504–19. doi: 10.1016/j.bbi.2018.06.015
180. Li TF, Fan H, Wang YX. Aconitum-derived Bulleyaconitine A exhibits antihypersensitivity through direct stimulating dynorphin A expression in spinal microglia. J Pain. (2016) 17:530–48. doi: 10.1016/j.jpain.2015.12.015
181. Spruce BA, Curtis R, Wilkin GP, Glover DM. A neuropeptide precursor in cerebellum: proenkephalin exists in subpopulations of both neurons and astrocytes. EMBO J. (1990) 9:1787–95. doi: 10.1002/j.1460-2075.1990.tb08303.x
182. Bazov I, Sarkisyan D, Kononenko O, Watanabe H, Taqi MM, Stålhandske L, et al. Neuronal expression of opioid gene is controlled by dual epigenetic and transcriptional mechanism in human brain. Cereb Cortex. (2018) 28:3129–42. doi: 10.1093/cercor/bhx181
Keywords: analgesia, astrocytes, microglia, nociceptin/orphanin FQ, oligodendrocytes, opioid-induced hyperalgesia, opioid peptides, opioid receptor signaling
Citation: Machelska H and Celik MÖ (2020) Opioid Receptors in Immune and Glial Cells—Implications for Pain Control. Front. Immunol. 11:300. doi: 10.3389/fimmu.2020.00300
Received: 15 May 2019; Accepted: 06 February 2020;
Published: 04 March 2020.
Edited by:
Sabita Roy, University of Miami, United StatesReviewed by:
Lawrence Toll, Florida Atlantic University, United StatesEyup Akgun, University of Minnesota, United States
Copyright © 2020 Machelska and Celik. This is an open-access article distributed under the terms of the Creative Commons Attribution License (CC BY). The use, distribution or reproduction in other forums is permitted, provided the original author(s) and the copyright owner(s) are credited and that the original publication in this journal is cited, in accordance with accepted academic practice. No use, distribution or reproduction is permitted which does not comply with these terms.
*Correspondence: Halina Machelska, aGFsaW5hLm1hY2hlbHNrYUBjaGFyaXRlLmRl