- VIB Laboratory of Translational Immunomodulation, VIB Center for Inflammation Research (IRC), University of Hasselt, Hasselt, Belgium
The rise in the prevalence of autoimmune diseases in developed societies has been associated with a change in lifestyle patterns. Among other factors, increased consumption of certain dietary components, such as table salt and fatty acids and excessive caloric intake has been associated with defective immunological tolerance. Dietary nutrients have shown to modulate the immune response by a direct effect on the function of immune cells or, indirectly, by acting on the microbiome of the gastrointestinal tract. FOXP3+ regulatory T cells (Tregs) suppress immune responses and are critical for maintaining peripheral tolerance and immune homeostasis, modulating chronic tissue inflammation and autoimmune disease. It is now well-recognized that Tregs show certain degree of plasticity and can gain effector functions to adapt their regulatory function to different physiological situations during an immune response. However, plasticity of Tregs might also result in conversion into effector T cells that may contribute to autoimmune pathogenesis. Yet, which environmental cues regulate Treg plasticity and function is currently poorly understood, but it is of significant importance for therapeutic purposes. Here we review the current understanding on the effect of certain dietary nutrients that characterize Western diets in Treg metabolism, stability, and function. Moreover, we will discuss the role of Tregs linking diet and autoimmunity and the potential of dietary-based interventions to modulate Treg function in disease.
Introduction
An appropriate balance between pro- and anti-inflammatory immune responses is required to protect organisms from invading pathogens and tumor development without incurring in autoimmune and allergic diseases. While different cell populations with anti-inflammatory activity have been identified, CD4+FOXP3+ regulatory T cells (Tregs) are the most well-defined. FOXP3 transcription factor determines Treg cell lineage and is essential for appropriate immune homeostasis. Loss-of-function mutations in foxp3 lead to fatal immune disorders in humans (IPEX) (1, 2) and mice (Scurfy phenotype) (3).
Tregs suppress innate and adaptive immune responses using a broad array of molecular mechanisms which e.g., involve cell-contact dependent mechanisms (4), the release of soluble factors (5, 6), deprivation of growth factors (7), induction of apoptosis of target cells (8), and ATP hydrolysis and adenosine production (9, 10). Although there is versatility in the Treg response that allows for a specialized response according to the environment, the anatomical location, and the type of the cell to suppress (11, 12), increasing evidence suggests lack of Treg stability as a culprit of autoimmunity (13). Tregs isolated for instance from T1D (14, 15), MS (16–19) and SLE (20) patients showed acquisition of pro-inflammatory functions and reduced suppressive potency in vitro.
Whereas, genetic factors clearly predispose to autoimmune development, the dramatic increase in the incidence of autoimmune diseases in Western countries suggests Western lifestyle patterns as important triggers of disease [reviewed in (21, 22)]. A variety of factors have been proposed to favor autoimmune development such as decrease pathogen exposure, smoke, hormones, stress, pollutants, dietary components and obesity (23–27). Moreover, increasing data highlight the complex interplay between nutrition, metabolic state and the immune response. Caloric restriction ameliorates disease severity and increases the lifespan in experimental animal models of inflammation and autoimmunity (28–30). By contrast, obesity is one of the most consisting factors that predispose for autoimmunity, having being linked with MS (31), T1D (32), psoriasis (33), and Chron's disease (34) (Figure 1). In addition, diet alters the gut microbial composition. Gut bacteria and their metabolites regulate pro-inflammatory and regulatory T cell responses in the gut, which could exert systemic effects in the individual (35–37).
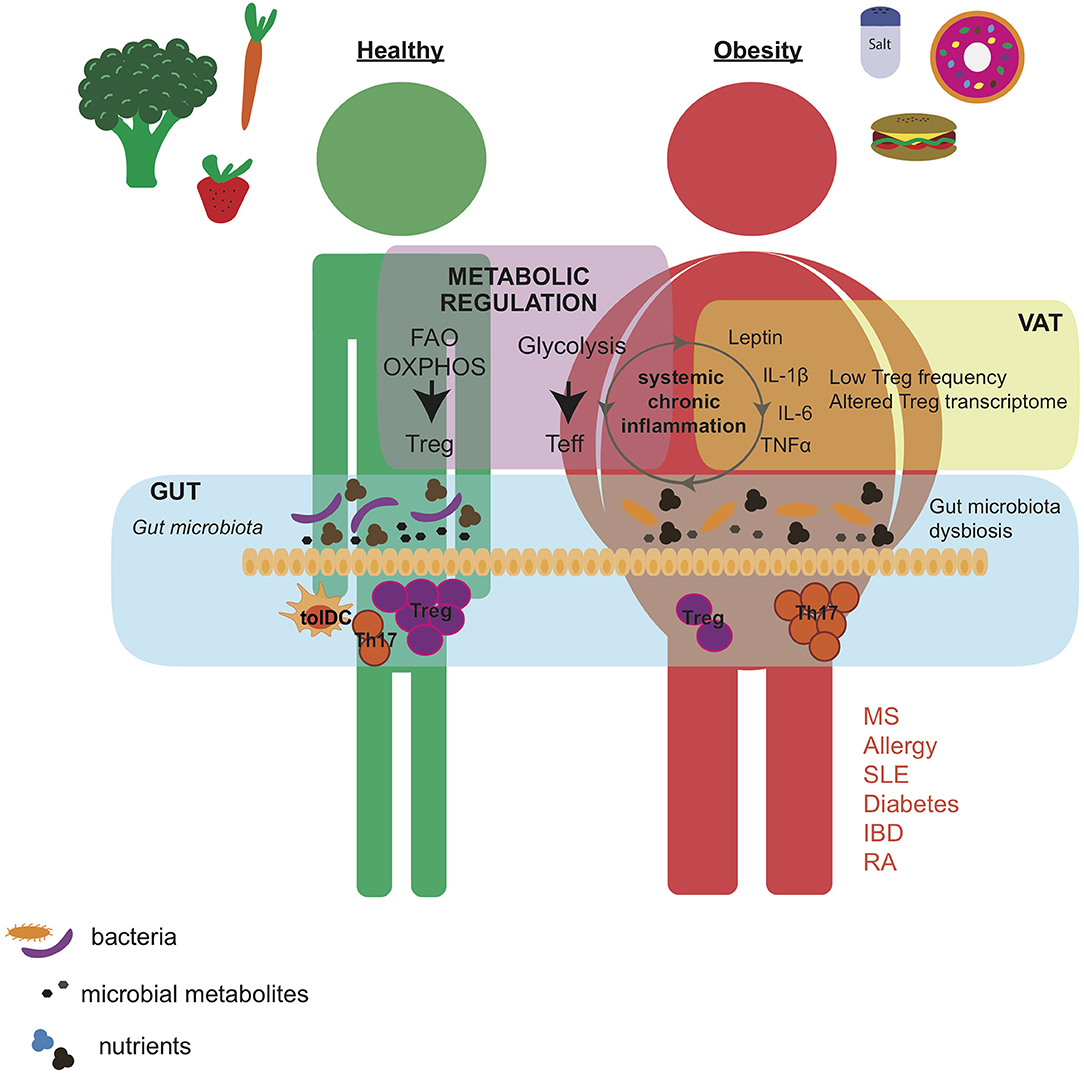
Figure 1. Western diet affects gut microbial composition and induces low-degree chronic inflammation that alters the metabolic status of the individual. Healthy diet supports the growth of bacterial species that, by the production of immunomodulatory metabolites, promotes Treg induction over Th17 cell development in the intestine. By contrast, western diet, characterized by high caloric intake, and high levels of salt and cholesterol, leads to obesity, increased secretion of pro-inflammatory adipokines and cytokines, and altered gut microbial composition. These changes are associated with an altered Treg phenotype and higher Th17 cell differentiation in the VAT and intestine. Importantly, Treg and Teff cells developed in the gut and VAT have a systemic effect and may contribute to exacerbation of autoimmune pathogenicity. Moreover, the frequency and function of Tregs may also be regulated by metabolic pathways such as FAO, OXPHOS, and glycolysis that depend on the nutritional state of the individual. VAT, visceral adipose tissue; Teff, effector T cell; FAO, fatty acid oxidation; OXPHOS, oxidative phosphorylation; MS, multiple sclerosis; SLE, systemic lupus erythematosus; IBD, inflammatory bowel disease; RA, rheumatoid arthritis.
Although there are many other cell types and environmental factors involved in triggering autoimmunity, given their crucial role in disease regulation, we will summarize the evidence provided by experimental and epidemiological studies associating nutrition, regulatory T cell function and autoimmunity.
Treg Regulation and Heterogeneity
We and others have shown before that different cell subsets can be distinguished within the pool of Tregs (9, 38). Recent immune phenotyping by mass cytometry and single cell transcriptomic analysis have further demonstrated the heterogeneity of the FOXP3+ Treg population (39, 40). Therefore, these technologies could potentially aid in the identification of novel markers involved in Treg function, stability and migration and in gaining a better understanding of Treg biology. Tregs are typically categorized according to their origin into two subsets; those that develop in the thymus (tTregs) as a distinct cell lineage, and those induced from CD4+CD25−FOXP3− naive T cells in peripheral tissues (pTregs). In vitro, FOXP3+ Tregs can also be generated from CD4+FOXP3− T cells by e.g., culturing them in the presence of TGF-β, IL-2, and anti-CD3 stimulation (41, 42) being generally named as iTregs, although their functional activity is not well-defined in humans.
FOXP3 is regulated at transcriptional and post-transcriptional level in response to environmental cues [reviewed in (43)]. Demethylation at specific regions of the foxp3 locus is pivotal for regulating FOXP3 expression in different Treg subsets (44). Moreover, distinct FOXP3 splicing variants have been described in humans (45–49) and variations in their relative expression are present in autoimmune disease patients (50–54), suggesting a link between FOXP3 post-transcriptional regulation and autoimmune pathogenicity.
Signals driven by the cytokine milieu (55–59), co-stimulatory molecules (60–62) and the strength of the TCR signaling (63–65) allow Tregs to adapt to the immune environment through e.g., changes in FOXP3 expression. Several studies have shown that, under certain inflammatory conditions, some Tregs secrete pro-inflammatory cytokines and lose their suppressive function (13, 66–72). Interestingly, phenotypically distinct Treg subsets in humans and mice have been described that mirror CD4+ Th cell populations by specific co-expression of chemokine receptors, cytokines, and lineage specifying-transcription factors classically associated with Th cells (18, 73–76). The acquisition of Th-specific markers may allow Tregs to co-localize and regulate particular Th cell subsets in vivo (76). However, it might also be an indication of loss of function. Indeed, an increase in IFN-γ-producing Tregs has been associated with e.g., T1D, MS and autoimmune hepatitis (15, 18, 77). Also, the frequency of Tregs expressing IL-17 is increased in e.g., human patients with psoriasis, IBD and RA (62, 78–83). These data suggest that some Th-like Tregs may lose their ability to suppress immune responses and, instead, may contribute to autoimmunity.
Additionally, Tregs show phenotypical differences depending on which tissue they reside in, with the best non-lymphoid-tissue Treg populations described being those residing in visceral adipose tissue (VAT), skeletal muscle, colonic lamina propria and skin [reviewed in (84–86)]. In general, tissue-resident Tregs are characterized by higher frequency, self-antigen TCR repertoire with clonal micro-expansion, and a specific transcriptional profile different from Tregs in lymphoid organs (87–90). Moreover, by the use of single cell transcriptomics it was revealed that Tregs are highly homogenous within each tissue (40). These distinct phenotypes allow for cell accumulation in specific tissues and dedicated function within the microenvironment [reviewed in (84)].
The existence of multiple Treg subsets with specialized function dependent on environmental signals shows the complexity of Treg biology, but it also makes Treg plasticity and function susceptible to pharmaceutical intervention. Whether changes in diet or microbial composition associated with a Western lifestyle can control Treg function is being actively studied.
Obesity and Vat Tregs
Excessive calories are stored as fat in adipose tissue, which also acts as an “endocrine organ” releasing pro-inflammatory adipokines and cytokines such as TNF-α, IL-6, IL-1β, and leptin, resulting in systemic low-degree chronic inflammation (91, 92) (Figure 1). Multiple immune cell types reside in the adipose tissue and play a role in inflammation and metabolic dysregulation (87, 93–99). In particular, obese mice display a dramatic reduction in Treg numbers specifically in adipose tissue, but not in other fat depots, nor in other non-lymphoid tissues and spleen or lymph nodes (87, 100). Cytokines and adipokines may be involved in controlling Treg fluctuations in obese VAT. Obesity positively correlates with IL-6 and IL-17 expression in mice and humans (101, 102). IL-6 promotes Th17 over Treg development (103) and obese-induced Th17 cell expansion was correlated with exacerbated disease symptoms in autoimmune disease models of experimental autoimmune encephalomyelitis (EAE) and colitis (102, 104). Leptin favors Th1 responses (105–109) and Th17 differentiation (110), but inhibits Treg proliferation (111). Moreover, leptin deficient mice showed a decrease in pathogenic inflammation in most of experimental models of IBD (112), RA (113, 114) and MS (108, 109). Similarly, relapsing-remitting MS patients also displayed an inverse correlation between frequency of Tregs and serum leptin levels (115), indicating that leptin may act as a link between obesity, Treg numbers and immunological tolerance.
In addition to differences in frequency, it was demonstrated that VAT-Tregs isolated from genetically promoted (leptin-deficient) or diet-induced (high fat chow) insulin-resistant mouse models of obesity have an altered transcriptional signature compared to lean mice (116). Phenotypical changes driven by obesity highlight the adaptability of VAT-Tregs to metabolic perturbations and suggest that obesity might alter Treg plasticity. Although most of the molecular mechanisms still need to be elucidated, it has been shown that excessive caloric intake leads to a dysregulation of intracellular nutrient-energy-sensing pathways and metabolic overload in immune cells (117, 118).
Metabolic Regulation of Tregs
Cellular metabolism regulates cell development, proliferation and function and is controlled by environmental cues and nutrient availability (119, 120). Tregs have a specific metabolic profile, which is mainly dependent on mitochondrial metabolism through fatty acid oxidation (FAO) or pyruvate dependent oxidative phosphorylation (OXPHOS) (121–125). mTOR, one of the main pathways linking nutritional availability with cellular activity, promotes glycolysis (126, 127) and regulates differentiation of Th1, Th17, and Tregs (128–130). Studies showed that in vitro over-activation of mTOR, by culturing in media containing high concentration of nutrients or leptin, impaired Treg proliferation and the induction of FOXP3 expression (131). Treatment with rapamycin or neutralizing anti-leptin mAb reversed this effect and resulted in increased Treg frequencies and lessened EAE severity. However, continuous treatment with rapamycin or genetic mTOR silencing impeded Treg proliferation in the long term in vivo (131). Hence, periods of high and low nutrient levels, required for oscillatory changes in mTOR activity, may be necessary for Treg homeostasis and immunotolerance [reviewed in (132)].
Deletion of PTEN, a negative regulator of PI3K, also contributes to Treg regulation by enhancing glycolysis, decreasing FOXP3 expression and inducing the generation of effector T cells (133, 134). Additionally, the metabolic sensor LKB1 acts through AMPK promoting OXPHOS over glycolysis, and its deletion on Tregs led to alterations in cellular metabolism and the development of autoimmune diseases associated with dampened FOXP3 expression (124, 135). On the other hand, AMPK is considered an antagonist of mTOR activity with the ability to promote FAO (136, 137). Berod et al. showed that deletion of ACC1, a key enzyme in fatty acid synthesis, promoted AMPK activity in CD4+ T cells leading to increases in FAO and Treg development, and ACC1 inhibition under EAE conditions improved disease severity by increasing Treg/Th17 ratio (138).
Vitamins and indoles also modulate Treg function (59). For example, retinoic acid (vitamin A metabolite) acts in conjunction with TGF-β promoting the induction of Tregs from naive T cells and stabilizing FOXP3 expression, which prevented their conversion into Th1/Th17 cells in the presence of IL-1β/IL-6 (139, 140). Calcitriol, a vitamin D metabolite, enhanced the growth of Tregs (141, 142). Vitamin C has been found to increase the generation of FOXP3+ iTregs on alloantigen-specific Treg induction cultures and to cause a pronounced TSDR demethylation, resulting in an elevated FOXP3 stability (143). Interestingly, vitamin C treatment may act in a distinct manner on tTreg and iTreg function. A recent study by Oyarce et al. showed that tTreg cells pretreated with vitamin C before coculturing with effector CD4+ T cells did not enhanced Treg ability to suppress T cell proliferation regardless of their increased FOXP3 expression. By contrast, in vitro-induced iTregs generated in presence of vitamin C showed improved suppressive capacities (144).
Metabolites associated with aryl hydrogen receptor (AHR) also control Treg function. Kynurenine is important for the generation, expansion and function of Tregs (145–147) and indole-3-carbanole (I3C) and 3,3′-diindolylmethane (DIM) promoted Treg infiltration to the CNS under EAE conditions, improving disease severity, and progression (147).
These data highlight the potential of targeting Treg metabolism to ameliorate autoimmune disease progression. However, more research regarding the therapeutic level of such modulation still has to be conducted. Besides, the limitations associated with studying Treg metabolism, due to their plasticity, culturing method, and biological source prompt a big challenge in the field.
The Effect of Salt in Treg Plasticity
Increased intake of salt that is also common in Western diets has been linked with cardiovascular disease (148, 149) and autoimmunity (22, 150–154). Although the specific mechanisms are still being revealed, several studies in murine EAE and colitis models have demonstrated that elevated NaCl intake could exacerbate disease by promoting the induction of pathogenic Th17 cells via the SGK1-FOXO1 pathway (35, 36, 155). Besides, Wu et al. have recently described a direct effect of SGK1-FOXO1 in controlling Treg function, such as SGK1 deficiency in Tregs protected mice from the development of autoimmunity (156).
High-salt also induced secretion of IFN-ɤ and repressed IL-10 expression in Tregs, which resulted in impaired suppressive function in vitro and in vivo (155, 157, 158). In mice fed with high-salt diet, Tregs secreted more IFN-γ and failed to control colitis and xenogeneic GvHD, which was dependent on SGK1 signaling (157). IFN-γ-secreting FOXP3+ Tregs with reduced IL-10 expression have been found in MS and T1D patients and are thought to contribute to disease (15, 18, 158). Interestingly, the imbalance between IFN-γ- and IL-10-expressing-Tregs in MS patients was also observed when Tregs from healthy donors were exposed to high-salt in vitro (158), suggesting that a high-salt environment could skew Tregs toward a dysfunctional state. Moreover, PTGER2 and β-catenin appeared as upstream regulators of the SGK1-FOXO1 axis in response to high-salt concentration, and constitutive expression of active β-catenin in Tregs caused the development of Scurfy-like autoimmunity (158). Interestingly, stabilized active β-catenin has been also found in MS patients (158), suggesting that similar regulation may exist in humans. Recently, Luo et al. have proposed that, while high-salt alters the function of tTregs, it has no effect on pTregs or iTreg, which maintained unaltered transcriptional signature and stable FOXP3 expression, cytokine profile and suppressive function under high salt conditions (159). However, Wu et al. reported higher FOXP3 expression and regulatory function in iTregs and pTregs that lack SGK1 function (156), indicating that iTregs and pTregs could also be affected by high-salt via SGK1-FOXO1 axis. More studies are therefore required to clarify the role of high salt in Treg subpopulations.
Based on these findings, multiple studies have attempted to study the relation between salt intake and autoimmune disease in humans. In MS patients, Farez et al. have reported a positive correlation between disease activity and increased dietary sodium intake (150). Moreover, higher sodium concentration was observed in acute MS lesions than in chronic lesions (160). However, studies in larger cohorts have shown no significant correlation between salt consumption and the risk of MS development (161) or disease severity (162). Limitations in accurate measurement of NaCl levels and in the identification of the specific effect of salt independently of other dietary components may account for these controversial results [as discussed in (163)]. Since moderate increases in salt intake has proven to affect human immune cells, including T cells in vivo (26, 37), more specific analysis are needed to establish the role of NaCl in human autoimmune disease.
The Gut Microbiota Affects Disease-Controlling Tregs
The human gastrointestinal tract is the major reservoir of microorganisms including bacteria, microeukaryotes, archaea, and viruses, all of which collectively constitute the commensal microbiota (164). Extensive research has demonstrated the intimate crosstalk between commensal microbiota and immune balance. Tregs residing in the intestine are critical for maintaining intestinal immune homeostasis (165–167). Increasing evidence shows that Tregs in the colonic lamina propria are mostly peripherally-induced and depend on microbiota-derived signals for proper development and function (90, 168, 169). In fact, germ-free mice or antibiotic-treated mice show a substantial reduction in colonic Treg frequency (168, 170, 171). Interestingly, induction of pTregs in the small intestine appears more dependent on dietary antigens than on microbial signals (172). By investigating germ-free mice fed on an antigen-free diet, Kim et al. elegantly showed reduction in pTreg numbers in small intestinal lamina propria compared to germ-free mice fed on conventional diet. Importantly, pTregs residing in small intestinal lamina propria suppressed immune responses against dietary antigens (172).
Haghikia et al. elegantly demonstrated that dietary fatty acids profoundly impact T cell subset differentiation in the gut, which had a subsequent impact on central nervous system autoimmunity. The authors showed that SCFAs increased Treg proliferation while long-chain fatty acids (LCFAs) supported Th1 and Th17 differentiation in the gut, which had a significant effect in EAE severity (173). The short-chain fatty acid (SCFA) butyrate, derived from fermentation of dietary carbohydrates by gut microbiota, is an important promotor of colonic Treg differentiation through epigenetic modifications in the foxp3 locus, which induces FOXP3 expression and pTreg conversion (171, 174–176). Clostridia are known to produce high levels of butyrate and colonization of germ-free mice with these bacteria increased colonic Treg frequency and protected mice from colitis (177). By contrast, segmented filamentous bacteria (SFB) induced Th17 cell development in the gut promoting systemic autoimmunity (178–180). In a recent study, Luu et al. have shown that the SCFA pentanoate inhibited SFB-promoted Th17 cell induction by metabolic and epigenetically reprogramming CD4+ T cells to suppress IL-17 production and fostering IL-10 production in CD4+ T cells and B cells (181). Furthermore, Häger et al. reported increased Treg numbers in 36 RA patients after receiving high-fiber dietary supplementation for 28 days, which correlated with a higher Th1/Th17 ratio and decreased expression of markers associated with bone erosion (182).
Several studies have shown that administration of probiotic bacteria containing members of the Lactobacillus, Streptococcus, and Bifidobacterium genera primed DCs to induce the development of FOXP3+ Tregs and IL-10-secreting regulatory T cells (183, 184) (Figure 1). Poutahidis et al. showed that mice fed with Westernized “fast food”-style chow developed obesity and had increased IL-17 levels. By contrast, the addition of probiotic yogurt containing Lactobacillus reuteri into the diet was sufficient to induce weight loss by a Treg dependent mechanism (174). Importantly, diet alters the gut microbiome (185–187) and dysregulation of intestinal microbiota is associated with autoimmunity [reviewed in (188, 189)]. Wilck et al. have shown that increased salt consumption affects intestinal bacterial composition in mice and humans. Lactobacillus spp. was suppressed in high salt condition, but its supplementation prevented high salt-induced Th17 differentiation and ameliorated salt-sensitive hypertension and EAE severity (37, 154). Cekanaviciute et al. found that MS patients have a high presence of the Akkermansia calcoaceticus and Akkermansia muciniphila, and the exposure of healthy donor PBMC to these bacteria impaired Treg conversion while enhancing Th1 differentiation (190). These data connect diet with microbiota composition and autoimmune pathogenesis, raising the potential of microbiota-targeted therapies.
Diet as a Therapeutic Aid to Control Autoimmunity
It is becoming clear that nutrition, metabolic state, microbiota, and autoimmunity are deeply interconnected. In addition to genetic factors, the Western diet characterized by high caloric intake in the form of processed food enriched in protein, sugar, fat and salt, is widely believed to contribute to the rise in autoimmune diseases in the last decades (Figure 1). However, one of the major challenges in investigating the effect of diet in human health is the impossibility to address the role of individual nutrients, which maybe the reason why a definite association between dietary interventions and outcomes in human autoimmune disease has not been established yet. Besides, dietary nutrients and microbial metabolites alter the immune response by acting on different immune cell populations, challenging our aim to identify underlying immunological mechanisms targeted during dietary interventions. For instance, we have recently corroborated that high salt diet lead to alterations in T cell populations in murine tumor transplantation models (191). However, inhibition of tumor growth given by high salt diet was largely independent of T cells in these models. Instead, high salt blocked the suppressive function of myeloid derived suppressor cells (MDSCs) in vitro and seems to promote thereby more pronounced anti-tumor immunity in vivo (191).
Obesity alters the balance between pro-inflammatory and suppressive T cells responses in adipose tissue, with Tregs losing their phenotypic identity and function (116), and resulting in break of self-tolerance (131) (Figure 1). Caloric restriction exerts immunoregulatory effects but is not suitable as general therapy for humans. Interestingly, Cignarella et al. have recently reported that intermittent fasting also improves disease outcomes in the EAE model as caloric restriction does (192). This effect was partially mediated by changes in the gut microbiota, since microbiota transplantation from mice under intermittent fasting into normally-fed mice could induce protection from EAE (192). Microbiota is a major determinant in the regulation of pro-inflammatory and regulatory T cell plasticity in the gut (35–37). Importantly, gut-resident T cells have the ability to traffic between different organs and exert a systemic effect in the organism (193, 194). Furthermore, these findings were translated into a small trial studying 16 MS patients that were on intermittent fasting for 15 days. Although no significant changes in gut bacteria composition was observed, a trend toward increased abundance of the Treg-inducer Clostridia bacteria was reported (177, 192).
As indicated by these data, dietary interventions and the use of probiotics may aid in the control of Treg stability and function by altering the milieu in which Tregs act in vivo, and help to restore immune responses in individuals with autoimmune prone Western lifestyle.
Concluding Remarks
Although it is clear that Treg function is frequently altered in human autoimmunity, it should be noted that Tregs are a heterogenous population with distinct tissue-specific features, multiple functions and differential degree of plasticity in response to environmental cues. Moreover, autoimmune diseases are highly heterogenous and it is likely that different defects in Treg-mediated regulation are involved in different types of autoimmune disease and even in each individual depending on the specific genetic background (195). Increasing progress in purifying and subdividing Treg subsets and defining the mechanisms that dictate their function and plasticity will likely contribute to a better understanding on the role of Tregs in autoimmunity.
Dietary factors, via direct effects on immune cells or by acting indirectly through modulation of the gut microbiota, may regulate Treg plasticity and function and, therefore, may have the potential to control disease outcome. However, more research and tightly controlled studies are needed to assess the impact of specific dietary nutrients and bacteria or microbial metabolites on Tregs, autoimmunity, and human health.
Author Contributions
RA, IH, BC-R, and MK wrote the manuscript.
Funding
MK was supported by the European Research Council (ERC) under the European Union's Horizon 2020 research and innovation program (640116), by a SALK-grant from the government of Flanders, Belgium and by an Odysseus-grant of the Research Foundation Flanders (FWO), Belgium.
Conflict of Interest
The authors declare that the research was conducted in the absence of any commercial or financial relationships that could be construed as a potential conflict of interest.
References
1. Bennett CL, Christie J, Ramsdell F, Brunkow ME, Ferguson PJ, Whitesell L, et al. The immune dysregulation, polyendocrinopathy, enteropathy, X-linked syndrome (IPEX) is caused by mutations of FOXP3. Nat Genet. (2001) 27:20–1. doi: 10.1038/83713
2. Wildin RS, Ramsdell F, Peake J, Faravelli F, Casanova JL, Buist N, et al. X-linked neonatal diabetes mellitus, enteropathy and endocrinopathy syndrome is the human equivalent of mouse scurfy. Nat Genet. (2001) 27:18–20. doi: 10.1038/83707
3. Brunkow ME, Jeffery EW, Hjerrild KA, Paeper B, Clark LB, Yasayko SA, et al. Disruption of a new forkhead/winged-helix protein, scurfin, results in the fatal lymphoproliferative disorder of the scurfy mouse. Nat Genet. (2001) 27:68–73. doi: 10.1038/83784
4. Qureshi OS, Zheng Y, Nakamura K, Attridge K, Manzotti C, Schmidt EM, et al. Trans-endocytosis of CD80 and CD86: a molecular basis for the cell-extrinsic function of CTLA-4. Science. (2011) 332:600–3. doi: 10.1126/science.1202947
5. Asseman C, Mauze S, Leach MW, Coffman RL, Powrie F. An essential role for interleukin 10 in the function of regulatory T cells that inhibit intestinal inflammation. J Exp Med. (1999) 190:995–1004. doi: 10.1084/jem.190.7.995
6. Banchereau J, Pascual V, O'Garra A. From IL-2 to IL-37: the expanding spectrum of anti-inflammatory cytokines. Nat Immunol. (2012) 13:925–31. doi: 10.1038/ni.2406
7. Barthlott T, Moncrieffe H, Veldhoen M, Atkins CJ, Christensen J, O'Garra A, et al. CD25+ CD4+ T cells compete with naive CD4+ T cells for IL-2 and exploit it for the induction of IL-10 production. Int Immunol. (2005) 17:279–88. doi: 10.1093/intimm/dxh207
8. Grossman WJ, Verbsky JW, Barchet W, Colonna M, Atkinson JP, Ley TJ. Human T regulatory cells can use the perforin pathway to cause autologous target cell death. Immunity. (2004) 21:589–601. doi: 10.1016/j.immuni.2004.09.002
9. Borsellino G, Kleinewietfeld M, Di Mitri D, Sternjak A, Diamantini A, Giometto R, et al. Expression of ectonucleotidase CD39 by Foxp3+ Treg cells: hydrolysis of extracellular ATP and immune suppression. Blood. (2007) 110:1225–32. doi: 10.1182/blood-2006-12-064527
10. Bodor J, Bopp T, Vaeth M, Klein M, Serfling E, Hunig T, et al. Cyclic AMP underpins suppression by regulatory T cells. Eur J Immunol. (2012) 42:1375–84. doi: 10.1002/eji.201141578
11. Sakaguchi S, Wing K, Onishi Y, Prieto-Martin P, Yamaguchi T. Regulatory T cells: how do they suppress immune responses? Int Immunol. (2009) 21:1105–11. doi: 10.1093/intimm/dxp095
12. Tang Q, Bluestone JA. The Foxp3+ regulatory T cell: a jack of all trades, master of regulation. Nat Immunol. (2008) 9:239–44. doi: 10.1038/ni1572
13. Zhou X, Bailey-Bucktrout SL, Jeker LT, Penaranda C, Martinez-Llordella M, Ashby M, et al. Instability of the transcription factor Foxp3 leads to the generation of pathogenic memory T cells in vivo. Nat Immunol. (2009) 10:1000–7. doi: 10.1038/ni.1774
14. Lawson JM, Tremble J, Dayan C, Beyan H, Leslie RD, Peakman M, et al. Increased resistance to CD4+CD25hi regulatory T cell-mediated suppression in patients with type 1 diabetes. Clin Exp Immunol. (2008) 154:353–9. doi: 10.1111/j.1365-2249.2008.03810.x
15. McClymont SA, Putnam AL, Lee MR, Esensten JH, Liu W, Hulme MA, et al. Plasticity of human regulatory T cells in healthy subjects and patients with type 1 diabetes. J Immunol. (2011) 186:3918–26. doi: 10.4049/jimmunol.1003099
16. Haas J, Hug A, Viehover A, Fritzsching B, Falk CS, Filser A, et al. Reduced suppressive effect of CD4+CD25high regulatory T cells on the T cell immune response against myelin oligodendrocyte glycoprotein in patients with multiple sclerosis. Eur J Immunol. (2005) 35:3343–52. doi: 10.1002/eji.200526065
17. Viglietta V, Baecher-Allan C, Weiner HL, Hafler DA. Loss of functional suppression by CD4+CD25+ regulatory T cells in patients with multiple sclerosis. J Exp Med. (2004) 199:971–9. doi: 10.1084/jem.20031579
18. Dominguez-Villar M, Baecher-Allan CM, Hafler DA. Identification of T helper type 1-like, Foxp3+ regulatory T cells in human autoimmune disease. Nat Med. (2011) 17:673–5. doi: 10.1038/nm.2389
19. Costantino CM, Baecher-Allan CM, Hafler DA. Human regulatory T cells and autoimmunity. Eur J Immunol. (2008) 38:921–4. doi: 10.1002/eji.200738104
20. Vargas-Rojas MI, Crispin JC, Richaud-Patin Y, Alcocer-Varela J. Quantitative and qualitative normal regulatory T cells are not capable of inducing suppression in SLE patients due to T-cell resistance. Lupus. (2008) 17:289–94. doi: 10.1177/0961203307088307
21. Javierre BM, Hernando H, Ballestar E. Environmental triggers and epigenetic deregulation in autoimmune disease. Discov Med. (2011) 12:535–45.
22. Manzel A, Muller DN, Hafler DA, Erdman SE, Linker RA, Kleinewietfeld M. Role of “Western diet” in inflammatory autoimmune diseases. Curr Allergy Asthma Rep. (2014) 14:404. doi: 10.1007/s11882-013-0404-6
23. Bach JF. The effect of infections on susceptibility to autoimmune and allergic diseases. N Engl J Med. (2002) 347:911–20. doi: 10.1056/NEJMra020100
24. Okada H, Kuhn C, Feillet H, Bach JF. The “hygiene hypothesis” for autoimmune and allergic diseases: an update. Clin Exp Immunol. (2010) 160:1–9. doi: 10.1111/j.1365-2249.2010.04139.x
25. Matveeva O, Bogie JFJ, Hendriks JJA, Linker RA, Haghikia A, Kleinewietfeld M. Western lifestyle and immunopathology of multiple sclerosis. Ann N Y Acad Sci. (2018) 1417:71–86. doi: 10.1111/nyas.13583
26. Willebrand R, Kleinewietfeld M. The role of salt for immune cell function and disease. Immunology. (2018) 154:346–53. doi: 10.1111/imm.12915
27. Procaccini C, Carbone F, Galgani M, La Rocca C, De Rosa V, Cassano S, et al. Obesity and susceptibility to autoimmune diseases. Expert Rev Clin Immunol. (2011) 7:287–94. doi: 10.1586/eci.11.18
28. Matsuzaki J, Kuwamura M, Yamaji R, Inui H, Nakano Y. Inflammatory responses to lipopolysaccharide are suppressed in 40% energy-restricted mice. J Nutr. (2001) 131:2139–44. doi: 10.1093/jn/131.8.2139
29. Muthukumar A, Sun D, Zaman K, Barnes JL, Haile D, Fernandes G. Age associated alterations in costimulatory and adhesion molecule expression in lupus-prone mice are attenuated by food restriction with n-6 and n-3 fatty acids. J Clin Immunol. (2004) 24:471–80. doi: 10.1023/B:JOCI.0000040918.92219.d1
30. Piccio L, Stark JL, Cross AH. Chronic calorie restriction attenuates experimental autoimmune encephalomyelitis. J Leukoc Biol. (2008) 84:940–8. doi: 10.1189/jlb.0208133
31. Mokry LE, Ross S, Timpson NJ, Sawcer S, Davey Smith G, Richards JB. Obesity and multiple sclerosis: a mendelian randomization study. PLoS Med. (2016) 13:e1002053. doi: 10.1371/journal.pmed.1002053
32. Ferrara PM, Stoner L, Cornwall J. Diagnosis of childhood obesity using BMI: potential ethicolegal implications and downstream effects. Obes Rev. (2017) 18:380–1. doi: 10.1111/obr.12509
33. Sterry W, Strober BE, Menter A, International Psoriasis Council. Obesity in psoriasis: the metabolic, clinical and therapeutic implications. Report of an interdisciplinary conference and review. Br J Dermatol. (2007) 157:649–55. doi: 10.1111/j.1365-2133.2007.08068.x
34. Hass DJ, Brensinger CM, Lewis JD, Lichtenstein GR. The impact of increased body mass index on the clinical course of Crohn's disease. Clin Gastroenterol Hepatol. (2006) 4:482–8. doi: 10.1016/j.cgh.2005.12.015
35. Kleinewietfeld M, Manzel A, Titze J, Kvakan H, Yosef N, Linker RA, et al. Sodium chloride drives autoimmune disease by the induction of pathogenic TH17 cells. Nature. (2013) 496:518–22. doi: 10.1038/nature11868
36. Wu C, Yosef N, Thalhamer T, Zhu C, Xiao S, Kishi Y, et al. Induction of pathogenic TH17 cells by inducible salt-sensing kinase SGK1. Nature. (2013) 496:513–7. doi: 10.1038/nature11984
37. Wilck N, Matus MG, Kearney SM, Olesen SW, Forslund K, Bartolomaeus H, et al. Salt-responsive gut commensal modulates TH17 axis and disease. Nature. (2017) 551:585–9. doi: 10.1038/nature24628
38. Miyara M, Yoshioka Y, Kitoh A, Shima T, Wing K, Niwa A, et al. Functional delineation and differentiation dynamics of human CD4+ T cells expressing the FoxP3 transcription factor. Immunity. (2009) 30:899–911. doi: 10.1016/j.immuni.2009.03.019
39. Mason GM, Lowe K, Melchiotti R, Ellis R, de Rinaldis E, Peakman M, et al. Phenotypic complexity of the human regulatory T cell compartment revealed by mass cytometry. J Immunol. (2015) 195:2030–7. doi: 10.4049/jimmunol.1500703
40. Miragaia RJ, Gomes T, Chomka A, Jardine L, Riedel A, Hegazy AN, et al. Single-cell transcriptomics of regulatory T cells reveals trajectories of tissue adaptation. Immunity. (2019) 50:493–504 e7. doi: 10.1016/j.immuni.2019.01.001
41. Abbas AK, Benoist C, Bluestone JA, Campbell DJ, Ghosh S, Hori S, et al. Regulatory T cells: recommendations to simplify the nomenclature. Nat Immunol. (2013) 14:307–8. doi: 10.1038/ni.2554
42. Shevach EM, Thornton AM. tTregs, pTregs, and iTregs: similarities and differences. Immunol Rev. (2014) 259:88–102. doi: 10.1111/imr.12160
43. Lu L, Barbi J, Pan F. The regulation of immune tolerance by FOXP3. Nat Rev Immunol. (2017) 17:703–17. doi: 10.1038/nri.2017.75
44. Zheng Y, Josefowicz S, Chaudhry A, Peng XP, Forbush K, Rudensky AY. Role of conserved non-coding DNA elements in the Foxp3 gene in regulatory T-cell fate. Nature. (2010) 463:808–12. doi: 10.1038/nature08750
45. Allan SE, Passerini L, Bacchetta R, Crellin N, Dai M, Orban PC, et al. The role of 2 FOXP3 isoforms in the generation of human CD4+ Tregs. J Clin Invest. (2005) 115:3276–84. doi: 10.1172/JCI24685
46. Smith EL, Finney HM, Nesbitt AM, Ramsdell F, Robinson MK. Splice variants of human FOXP3 are functional inhibitors of human CD4+ T-cell activation. Immunology. (2006) 119:203–11. doi: 10.1111/j.1365-2567.2006.02425.x
47. Du J, Huang C, Zhou B, Ziegler SF. Isoform-specific inhibition of ROR alpha-mediated transcriptional activation by human FOXP3. J Immunol. (2008) 180:4785–92. doi: 10.4049/jimmunol.180.7.4785
48. Kaur G, Goodall JC, Jarvis LB, Hill Gaston JS. Characterisation of Foxp3 splice variants in human CD4+ and CD8+ T cells–identification of Foxp3Delta7 in human regulatory T cells. Mol Immunol. (2010) 48(1–3):321–32. doi: 10.1016/j.molimm.2010.07.008
49. Mailer RK, Joly AL, Liu S, Elias S, Tegner J, Andersson J. IL-1β promotes Th17 differentiation by inducing alternative splicing of FOXP3. Sci Rep. (2015) 5:14674. doi: 10.1038/srep14674
50. Ryder LR, Woetmann A, Madsen HO, Odum N, Ryder LP, Bliddal H, et al. Expression of full-length and splice forms of FoxP3 in rheumatoid arthritis. Scand J Rheumatol. (2010) 39:279–86. doi: 10.3109/03009740903555374
51. Sambucci M, Gargano F, De Rosa V, De Bardi M, Picozza M, Placido R, et al. FoxP3 isoforms and PD-1 expression by T regulatory cells in multiple sclerosis. Sci Rep. (2018) 8:3674. doi: 10.1038/s41598-018-21861-5
52. Joly AL, Seitz C, Liu S, Kuznetsov NV, Gertow K, Westerberg LS, et al. Alternative splicing of FOXP3 controls regulatory T cell effector functions and is associated with human atherosclerotic plaque stability. Circ Res. (2018) 122:1385–94. doi: 10.1161/CIRCRESAHA.117.312340
53. Joly AL, Andersson J. Alternative splicing, FOXP3 and cardiovascular disease. Aging. (2019) 11:1905–6. doi: 10.18632/aging.101897
54. Mailer RKW. Alternative splicing of FOXP3-virtue and vice. Front Immunol. (2018) 9:530. doi: 10.3389/fimmu.2018.00530
55. Cheng G, Yu A, Malek TR. T-cell tolerance and the multi-functional role of IL-2R signaling in T-regulatory cells. Immunol Rev. (2011) 241:63–76. doi: 10.1111/j.1600-065X.2011.01004.x
56. Tran DQ, Ramsey H, Shevach EM. Induction of FOXP3 expression in naive human CD4+FOXP3 T cells by T-cell receptor stimulation is transforming growth factor-beta dependent but does not confer a regulatory phenotype. Blood. (2007) 110:2983–90. doi: 10.1182/blood-2007-06-094656
57. Walker MR, Kasprowicz DJ, Gersuk VH, Benard A, Van Landeghen M, Buckner JH, et al. Induction of FoxP3 and acquisition of T regulatory activity by stimulated human CD4+CD25- T cells. J Clin Invest. (2003) 112:1437–43. doi: 10.1172/JCI19441
58. Sakaguchi S, Vignali DA, Rudensky AY, Niec RE, Waldmann H. The plasticity and stability of regulatory T cells. Nat Rev Immunol. (2013) 13:461–7. doi: 10.1038/nri3464
59. Hoeppli RE, Wu D, Cook L, Levings MK. The environment of regulatory T cell biology: cytokines, metabolites, and the microbiome. Front Immunol. (2015) 6:61. doi: 10.3389/fimmu.2015.00061
60. Francisco LM, Salinas VH, Brown KE, Vanguri VK, Freeman GJ, Kuchroo VK, et al. PD-L1 regulates the development, maintenance, and function of induced regulatory T cells. J Exp Med. (2009) 206:3015–29. doi: 10.1084/jem.20090847
61. Stathopoulou C, Gangaplara A, Mallett G, Flomerfelt FA, Liniany LP, Knight D, et al. PD-1 inhibitory receptor downregulates asparaginyl endopeptidase and maintains Foxp3 transcription factor stability in induced regulatory T cells. Immunity. (2018) 49:247–63 e7. doi: 10.1016/j.immuni.2018.05.006
62. Remedios KA, Zirak B, Sandoval PM, Lowe MM, Boda D, Henley E, et al. The TNFRSF members CD27 and OX40 coordinately limit TH17 differentiation in regulatory T cells. Sci Immunol. (2018) 3:eaau2042. doi: 10.1126/sciimmunol.aau2042
63. Sauer S, Bruno L, Hertweck A, Finlay D, Leleu M, Spivakov M, et al. T cell receptor signaling controls Foxp3 expression via PI3K, Akt, and mTOR. Proc Natl Acad Sci USA. (2008) 105:7797–802. doi: 10.1073/pnas.0800928105
64. Ohkura N, Hamaguchi M, Morikawa H, Sugimura K, Tanaka A, Ito Y, et al. T cell receptor stimulation-induced epigenetic changes and Foxp3 expression are independent and complementary events required for Treg cell development. Immunity. (2012) 37:785–99. doi: 10.1016/j.immuni.2012.09.010
65. Li MO, Rudensky AY. T cell receptor signalling in the control of regulatory T cell differentiation and function. Nat Rev Immunol. (2016) 16:220–33. doi: 10.1038/nri.2016.26
66. Oldenhove G, Bouladoux N, Wohlfert EA, Hall JA, Chou D, Dos Santos L, et al. Decrease of Foxp3+ Treg cell number and acquisition of effector cell phenotype during lethal infection. Immunity. (2009) 31:772–86. doi: 10.1016/j.immuni.2009.10.001
67. Tsuji M, Komatsu N, Kawamoto S, Suzuki K, Kanagawa O, Honjo T, et al. Preferential generation of follicular B helper T cells from Foxp3+ T cells in gut Peyer's patches. Science. (2009) 323:1488–92. doi: 10.1126/science.1169152
68. Kim HJ, Barnitz RA, Kreslavsky T, Brown FD, Moffett H, Lemieux ME, et al. Stable inhibitory activity of regulatory T cells requires the transcription factor Helios. Science. (2015) 350:334–9. doi: 10.1126/science.aad0616
69. Polesso F, Sarker M, Anderson A, Parker DC, Murray SE. Constitutive expression of NF-kappaB inducing kinase in regulatory T cells impairs suppressive function and promotes instability and pro-inflammatory cytokine production. Sci Rep. (2017) 7:14779. doi: 10.1038/s41598-017-14965-x
70. Yang XO, Nurieva R, Martinez GJ, Kang HS, Chung Y, Pappu BP, et al. Molecular antagonism and plasticity of regulatory and inflammatory T cell programs. Immunity. (2008) 29:44–56. doi: 10.1016/j.immuni.2008.05.007
71. Kleinewietfeld M, Hafler DA. The plasticity of human Treg and Th17 cells and its role in autoimmunity. Semin Immunol. (2013) 25:305–12. doi: 10.1016/j.smim.2013.10.009
72. Kleinewietfeld M, Hafler DA. Regulatory T cells in autoimmune neuroinflammation. Immunol Rev. (2014) 259:231–44. doi: 10.1111/imr.12169
73. Haringer B, Lozza L, Steckel B, Geginat J. Identification and characterization of IL-10/IFN-gamma-producing effector-like T cells with regulatory function in human blood. J Exp Med. (2009) 206:1009–17. doi: 10.1084/jem.20082238
74. Gandhi R, Kumar D, Burns EJ, Nadeau M, Dake B, Laroni A, et al. Activation of the aryl hydrocarbon receptor induces human type 1 regulatory T cell-like and Foxp3(+) regulatory T cells. Nat Immunol. (2010) 11:846–53. doi: 10.1038/ni.1915
75. Beriou G, Costantino CM, Ashley CW, Yang L, Kuchroo VK, Baecher-Allan C, et al. IL-17-producing human peripheral regulatory T cells retain suppressive function. Blood. (2009) 113:4240–9. doi: 10.1182/blood-2008-10-183251
76. Duhen T, Duhen R, Lanzavecchia A, Sallusto F, Campbell DJ. Functionally distinct subsets of human FOXP3+ Treg cells that phenotypically mirror effector Th cells. Blood. (2012) 119:4430–40. doi: 10.1182/blood-2011-11-392324
77. Arterbery AS, Osafo-Addo A, Avitzur Y, Ciarleglio M, Deng Y, Lobritto SJ, et al. Production of proinflammatory cytokines by monocytes in liver-transplanted recipients with de novo autoimmune hepatitis is enhanced and induces TH1-like regulatory T cells. J Immunol. (2016) 196:4040–51. doi: 10.4049/jimmunol.1502276
78. Voo KS, Wang YH, Santori FR, Boggiano C, Wang YH, Arima K, et al. Identification of IL-17-producing FOXP3+ regulatory T cells in humans. Proc Natl Acad Sci USA. (2009) 106:4793–8. doi: 10.1073/pnas.0900408106
79. Hovhannisyan Z, Treatman J, Littman DR, Mayer L. Characterization of interleukin-17-producing regulatory T cells in inflamed intestinal mucosa from patients with inflammatory bowel diseases. Gastroenterology. (2011) 140:957–65. doi: 10.1053/j.gastro.2010.12.002
80. Pesenacker AM, Bending D, Ursu S, Wu Q, Nistala K, Wedderburn LR. CD161 defines the subset of FoxP3+ T cells capable of producing proinflammatory cytokines. Blood. (2013) 121:2647–58. doi: 10.1182/blood-2012-08-443473
81. Bovenschen HJ, van de Kerkhof PC, van Erp PE, Woestenenk R, Joosten I, Koenen HJ. Foxp3+ regulatory T cells of psoriasis patients easily differentiate into IL-17A-producing cells and are found in lesional skin. J Invest Dermatol. (2011) 131:1853–60. doi: 10.1038/jid.2011.139
82. Sanchez Rodriguez R, Pauli ML, Neuhaus IM, Yu SS, Arron ST, Harris HW, et al. Memory regulatory T cells reside in human skin. J Clin Invest. (2014) 124:1027–36. doi: 10.1172/JCI72932
83. Li L, Boussiotis VA. The role of IL-17-producing Foxp3+ CD4+ T cells in inflammatory bowel disease and colon cancer. Clin Immunol. (2013) 148:246–53. doi: 10.1016/j.clim.2013.05.003
84. Panduro M, Benoist C, Mathis D. Tissue Tregs. Annu Rev Immunol. (2016) 34:609–33. doi: 10.1146/annurev-immunol-032712-095948
85. Burzyn D, Benoist C, Mathis D. Regulatory T cells in non-lymphoid tissues. Nat Immunol. (2013) 14:1007–13. doi: 10.1038/ni.2683
86. Cipolletta D. Adipose tissue-resident regulatory T cells: phenotypic specialization, functions and therapeutic potential. Immunology. (2014) 142:517–25. doi: 10.1111/imm.12262
87. Feuerer M, Herrero L, Cipolletta D, Naaz A, Wong J, Nayer A, et al. Lean, but not obese, fat is enriched for a unique population of regulatory T cells that affect metabolic parameters. Nat Med. (2009) 15:930–9. doi: 10.1038/nm.2002
88. Hall JA, Bouladoux N, Sun CM, Wohlfert EA, Blank RB, Zhu Q, et al. Commensal DNA limits regulatory T cell conversion and is a natural adjuvant of intestinal immune responses. Immunity. (2008) 29:637–49. doi: 10.1016/j.immuni.2008.08.009
89. Kolodin D, van Panhuys N, Li C, Magnuson AM, Cipolletta D, Miller CM, et al. Antigen- and cytokine-driven accumulation of regulatory T cells in visceral adipose tissue of lean mice. Cell Metab. (2015) 21:543–57. doi: 10.1016/j.cmet.2015.03.005
90. Bilate AM, Bousbaine D, Mesin L, Agudelo M, Leube J, Kratzert A, et al. Tissue-specific emergence of regulatory and intraepithelial T cells from a clonal T cell precursor. Sci Immunol. (2016) 1:eaaf7471. doi: 10.1126/sciimmunol.aaf7471
91. Fantuzzi G. Adipose tissue, adipokines, and inflammation. J Allergy Clin Immunol. (2005) 115:911–9. doi: 10.1016/j.jaci.2005.02.023
92. Hotamisligil GS, Shargill NS, Spiegelman BM. Adipose expression of tumor necrosis factor-alpha: direct role in obesity-linked insulin resistance. Science. (1993) 259:87–91. doi: 10.1126/science.7678183
93. Weisberg SP, McCann D, Desai M, Rosenbaum M, Leibel RL, Ferrante AW Jr. Obesity is associated with macrophage accumulation in adipose tissue. J Clin Invest. (2003) 112:1796–808. doi: 10.1172/JCI200319246
94. Lumeng CN, Bodzin JL, Saltiel AR. Obesity induces a phenotypic switch in adipose tissue macrophage polarization. J Clin Invest. (2007) 117:175–84. doi: 10.1172/JCI29881
95. Lumeng CN, Deyoung SM, Bodzin JL, Saltiel AR. Increased inflammatory properties of adipose tissue macrophages recruited during diet-induced obesity. Diabetes. (2007) 56:16–23. doi: 10.2337/db06-1076
96. Liu J, Divoux A, Sun J, Zhang J, Clement K, Glickman JN, et al. Genetic deficiency and pharmacological stabilization of mast cells reduce diet-induced obesity and diabetes in mice. Nat Med. (2009) 15:940–5. doi: 10.1038/nm.1994
97. Winer DA, Winer S, Shen L, Wadia PP, Yantha J, Paltser G, et al. B cells promote insulin resistance through modulation of T cells and production of pathogenic IgG antibodies. Nat Med. (2011) 17:610–7. doi: 10.1038/nm.2353
98. Schipper HS, Rakhshandehroo M, van de Graaf SF, Venken K, Koppen A, Stienstra R, et al. Natural killer T cells in adipose tissue prevent insulin resistance. J Clin Invest. (2012) 122:3343–54. doi: 10.1172/JCI62739
99. Nishimura S, Manabe I, Nagasaki M, Eto K, Yamashita H, Ohsugi M, et al. CD8+ effector T cells contribute to macrophage recruitment and adipose tissue inflammation in obesity. Nat Med. (2009) 15:914–20. doi: 10.1038/nm.1964
100. Cipolletta D, Kolodin D, Benoist C, Mathis D. Tissular T(regs): a unique population of adipose-tissue-resident Foxp3+CD4+ T cells that impacts organismal metabolism. Semin Immunol. (2011) 23:431–7. doi: 10.1016/j.smim.2011.06.002
101. Bastard JP, Jardel C, Bruckert E, Blondy P, Capeau J, Laville M, et al. Elevated levels of interleukin 6 are reduced in serum and subcutaneous adipose tissue of obese women after weight loss. J Clin Endocrinol Metab. (2000) 85:3338–42. doi: 10.1210/jcem.85.9.6839
102. Ahmed M, Gaffen SL. IL-17 in obesity and adipogenesis. Cytokine Growth Factor Rev. (2010) 21:449–53. doi: 10.1016/j.cytogfr.2010.10.005
103. Kimura A, Kishimoto T. IL-6: regulator of Treg/Th17 balance. Eur J Immunol. (2010) 40:1830–5. doi: 10.1002/eji.201040391
104. Winer S, Paltser G, Chan Y, Tsui H, Engleman E, Winer D, et al. Obesity predisposes to Th17 bias. Eur J Immunol. (2009) 39:2629–35. doi: 10.1002/eji.200838893
105. Lord GM, Matarese G, Howard JK, Baker RJ, Bloom SR, Lechler RI. Leptin modulates the T-cell immune response and reverses starvation-induced immunosuppression. Nature. (1998) 394:897–901. doi: 10.1038/29795
106. Fantuzzi G, Faggioni R. Leptin in the regulation of immunity, inflammation, and hematopoiesis. J Leukoc Biol. (2000) 68:437–46.
107. La Cava A, Alviggi C, Matarese G. Unraveling the multiple roles of leptin in inflammation and autoimmunity. J Mol Med. (2004) 82:4–11. doi: 10.1007/s00109-003-0492-1
108. Matarese G, Di Giacomo A, Sanna V, Lord GM, Howard JK, Di Tuoro A, et al. Requirement for leptin in the induction and progression of autoimmune encephalomyelitis. J Immunol. (2001) 166:5909–16. doi: 10.4049/jimmunol.166.10.5909
109. Matarese G, Sanna V, Di Giacomo A, Lord GM, Howard JK, Bloom SR, et al. Leptin potentiates experimental autoimmune encephalomyelitis in SJL female mice and confers susceptibility to males. Eur J Immunol. (2001) 31:1324–32. doi: 10.1002/1521-4141(200105)31:5<1324::AID-IMMU1324>3.0.CO;2-Y
110. Reis BS, Lee K, Fanok MH, Mascaraque C, Amoury M, Cohn LB, et al. Leptin receptor signaling in T cells is required for Th17 differentiation. J Immunol. (2015) 194:5253–60. doi: 10.4049/jimmunol.1402996
111. De Rosa V, Procaccini C, Cali G, Pirozzi G, Fontana S, Zappacosta S, et al. A key role of leptin in the control of regulatory T cell proliferation. Immunity. (2007) 26:241–55. doi: 10.1016/j.immuni.2007.01.011
112. Siegmund B, Lehr HA, Fantuzzi G. Leptin: a pivotal mediator of intestinal inflammation in mice. Gastroenterology. (2002) 122:2011–25. doi: 10.1053/gast.2002.33631
113. Busso N, So A, Chobaz-Peclat V, Morard C, Martinez-Soria E, Talabot-Ayer D, et al. Leptin signaling deficiency impairs humoral and cellular immune responses and attenuates experimental arthritis. J Immunol. (2002) 168:875–82. doi: 10.4049/jimmunol.168.2.875
114. Bernotiene E, Palmer G, Talabot-Ayer D, Szalay-Quinodoz I, Aubert ML, Gabay C. Delayed resolution of acute inflammation during zymosan-induced arthritis in leptin-deficient mice. Arthritis Res Ther. (2004) 6:R256–63. doi: 10.1186/ar1174
115. Matarese G, Carrieri PB, La Cava A, Perna F, Sanna V, De Rosa V, et al. Leptin increase in multiple sclerosis associates with reduced number of CD4(+)CD25+ regulatory T cells. Proc Natl Acad Sci USA. (2005) 102:5150–5. doi: 10.1073/pnas.0408995102
116. Cipolletta D, Cohen P, Spiegelman BM, Benoist C, Mathis D. Appearance and disappearance of the mRNA signature characteristic of Treg cells in visceral adipose tissue: age, diet, and PPARgamma effects. Proc Natl Acad Sci USA. (2015) 112:482–7. doi: 10.1073/pnas.1423486112
117. Matarese G, La Cava A. The intricate interface between immune system and metabolism. Trends Immunol. (2004) 25:193–200. doi: 10.1016/j.it.2004.02.009
118. Procaccini C, Galgani M, De Rosa V, Matarese G. Intracellular metabolic pathways control immune tolerance. Trends Immunol. (2012) 33:1–7. doi: 10.1016/j.it.2011.09.002
119. Pearce EL, Pearce EJ. Metabolic pathways in immune cell activation and quiescence. Immunity. (2013) 38:633–43. doi: 10.1016/j.immuni.2013.04.005
120. Binger KJ, Gebhardt M, Heinig M, Rintisch C, Schroeder A, Neuhofer W, et al. High salt reduces the activation of IL-4- and IL-13-stimulated macrophages. J Clin Invest. (2015) 125:4223–38. doi: 10.1172/JCI80919
121. Beier UH, Angelin A, Akimova T, Wang L, Liu Y, Xiao H, et al. Essential role of mitochondrial energy metabolism in Foxp3(+) T-regulatory cell function and allograft survival. FASEB J. (2015) 29:2315–26. doi: 10.1096/fj.14-268409
122. Gerriets VA, Kishton RJ, Nichols AG, Macintyre AN, Inoue M, Ilkayeva O, et al. Metabolic programming and PDHK1 control CD4+ T cell subsets and inflammation. J Clin Invest. (2015) 125:194–207. doi: 10.1172/JCI76012
123. Shi LZ, Wang R, Huang G, Vogel P, Neale G, Green DR, et al. HIF1alpha-dependent glycolytic pathway orchestrates a metabolic checkpoint for the differentiation of TH17 and Treg cells. J Exp Med. (2011) 208:1367–76. doi: 10.1084/jem.20110278
124. He N, Fan W, Henriquez B, Yu RT, Atkins AR, Liddle C, et al. Metabolic control of regulatory T cell (Treg) survival and function by Lkb1. Proc Natl Acad Sci USA. (2017) 114:12542–7. doi: 10.1073/pnas.1715363114
125. Binger KJ, Corte-Real BF, Kleinewietfeld M. Immunometabolic regulation of interleukin-17-producing T helper cells: uncoupling new targets for autoimmunity. Front Immunol. (2017) 8:311. doi: 10.3389/fimmu.2017.00311
126. Gerriets VA, Kishton RJ, Johnson MO, Cohen S, Siska PJ, Nichols AG, et al. Foxp3 and Toll-like receptor signaling balance Treg cell anabolic metabolism for suppression. Nat Immunol. (2016) 17:1459–66. doi: 10.1038/ni.3577
127. Thorens B, Mueckler M. Glucose transporters in the 21st Century. Am J Physiol Endocrinol Metab. (2010) 298:E141–5. doi: 10.1152/ajpendo.00712.2009
128. Delgoffe GM, Kole TP, Zheng Y, Zarek PE, Matthews KL, Xiao B, et al. The mTOR kinase differentially regulates effector and regulatory T cell lineage commitment. Immunity. (2009) 30:832–44. doi: 10.1016/j.immuni.2009.04.014
129. Delgoffe GM, Pollizzi KN, Waickman AT, Heikamp E, Meyers DJ, Horton MR, et al. The kinase mTOR regulates the differentiation of helper T cells through the selective activation of signaling by mTORC1 and mTORC2. Nat Immunol. (2011) 12:295–303. doi: 10.1038/ni.2005
130. Dang EV, Barbi J, Yang HY, Jinasena D, Yu H, Zheng Y, et al. Control of T(H)17/T(reg) balance by hypoxia-inducible factor 1. Cell. (2011) 146:772–84. doi: 10.1016/j.cell.2011.07.033
131. Procaccini C, De Rosa V, Galgani M, Abanni L, Cali G, Porcellini A, et al. An oscillatory switch in mTOR kinase activity sets regulatory T cell responsiveness. Immunity. (2010) 33:929–41. doi: 10.1016/j.immuni.2010.11.024
132. De Rosa V, La Cava A, Matarese G. Metabolic pressure and the breach of immunological self-tolerance. Nat Immunol. (2017) 18:1190–6. doi: 10.1038/ni.3851
133. Huynh A, DuPage M, Priyadharshini B, Sage PT, Quiros J, Borges CM, et al. Control of PI(3) kinase in Treg cells maintains homeostasis and lineage stability. Nat Immunol. (2015) 16:188–96. doi: 10.1038/ni.3077
134. Shrestha S, Yang K, Guy C, Vogel P, Neale G, Chi H. Treg cells require the phosphatase PTEN to restrain TH1 and TFH cell responses. Nat Immunol. (2015) 16:178–87. doi: 10.1038/ni.3076
135. Wu D, Luo Y, Guo W, Niu Q, Xue T, Yang F, et al. Lkb1 maintains Treg cell lineage identity. Nat Commun. (2017) 8:15876. doi: 10.1038/ncomms15876
136. O'Neill HM, Lally JS, Galic S, Thomas M, Azizi PD, Fullerton MD, et al. AMPK phosphorylation of ACC2 is required for skeletal muscle fatty acid oxidation and insulin sensitivity in mice. Diabetologia. (2014) 57:1693–702. doi: 10.1007/s00125-014-3273-1
137. Faubert B, Boily G, Izreig S, Griss T, Samborska B, Dong Z, et al. AMPK is a negative regulator of the Warburg effect and suppresses tumor growth in vivo. Cell Metab. (2013) 17:113–24. doi: 10.1016/j.cmet.2012.12.001
138. Berod L, Friedrich C, Nandan A, Freitag J, Hagemann S, Harmrolfs K, et al. De novo fatty acid synthesis controls the fate between regulatory T and T helper 17 cells. Nat Med. (2014) 20:1327–33. doi: 10.1038/nm.3704
139. Raverdeau M, Mills KH. Modulation of T cell and innate immune responses by retinoic Acid. J Immunol. (2014) 192:2953–8. doi: 10.4049/jimmunol.1303245
140. Lu L, Lan Q, Li Z, Zhou X, Gu J, Li Q, et al. Critical role of all-trans retinoic acid in stabilizing human natural regulatory T cells under inflammatory conditions. Proc Natl Acad Sci USA. (2014) 111:E3432–40. doi: 10.1073/pnas.1408780111
141. Urry Z, Chambers ES, Xystrakis E, Dimeloe S, Richards DF, Gabrysova L, et al. The role of 1α,25-dihydroxyvitamin D3 and cytokines in the promotion of distinct Foxp3+ and IL-10+ CD4+ T cells. Eur J Immunol. (2012) 42:2697–708. doi: 10.1002/eji.201242370
142. Chambers ES, Suwannasaen D, Mann EH, Urry Z, Richards DF, Lertmemongkolchai G, et al. 1α,25-dihydroxyvitamin D3 in combination with transforming growth factor-beta increases the frequency of Foxp3(+) regulatory T cells through preferential expansion and usage of interleukin-2. Immunology. (2014) 143:52–60. doi: 10.1111/imm.12289
143. Nikolouli E, Hardtke-Wolenski M, Hapke M, Beckstette M, Geffers R, Floess S, et al. Alloantigen-induced regulatory T cells generated in presence of vitamin C display enhanced stability of Foxp3 expression and promote skin allograft acceptance. Front Immunol. (2017) 8:748. doi: 10.3389/fimmu.2017.00748
144. Oyarce K, Campos-Mora M, Gajardo-Carrasco T, Pino-Lagos K. Vitamin C fosters the in vivo differentiation of peripheral CD4(+) Foxp3(-) T cells into CD4(+) Foxp3(+) regulatory T cells but impairs their ability to prolong skin allograft survival. Front Immunol. (2018) 9:112. doi: 10.3389/fimmu.2018.00112
145. Baban B, Chandler PR, Sharma MD, Pihkala J, Koni PA, Munn DH, et al. IDO activates regulatory T cells and blocks their conversion into Th17-like T cells. J Immunol. (2009) 183:2475–83. doi: 10.4049/jimmunol.0900986
146. Yan Y, Zhang GX, Gran B, Fallarino F, Yu S, Li H, et al. IDO upregulates regulatory T cells via tryptophan catabolite and suppresses encephalitogenic T cell responses in experimental autoimmune encephalomyelitis. J Immunol. (2010) 185:5953–61. doi: 10.4049/jimmunol.1001628
147. Rouse M, Singh NP, Nagarkatti PS, Nagarkatti M. Indoles mitigate the development of experimental autoimmune encephalomyelitis by induction of reciprocal differentiation of regulatory T cells and Th17 cells. Br J Pharmacol. (2013) 169:1305–21. doi: 10.1111/bph.12205
148. He FJ, MacGregor GA. A comprehensive review on salt and health and current experience of worldwide salt reduction programmes. J Hum Hypertens. (2009) 23:363–84. doi: 10.1038/jhh.2008.144
149. Farquhar WB, Edwards DG, Jurkovitz CT, Weintraub WS. Dietary sodium and health: more than just blood pressure. J Am Coll Cardiol. (2015) 65:1042–50. doi: 10.1016/j.jacc.2014.12.039
150. Farez MF, Fiol MP, Gaitan MI, Quintana FJ, Correale J. Sodium intake is associated with increased disease activity in multiple sclerosis. J Neurol Neurosurg Psychiatry. (2015) 86:26–31. doi: 10.1136/jnnp-2014-307928
151. Yang X, Yao G, Chen W, Tang X, Feng X, Sun L. Exacerbation of lupus nephritis by high sodium chloride related to activation of SGK1 pathway. Int Immunopharmacol. (2015) 29:568–73. doi: 10.1016/j.intimp.2015.09.027
152. Wu H, Huang X, Qiu H, Zhao M, Liao W, Yuan S, et al. High salt promotes autoimmunity by TET2-induced DNA demethylation and driving the differentiation of Tfh cells. Sci Rep. (2016) 6:28065. doi: 10.1038/srep28065
153. Monteleone I, Marafini I, Dinallo V, Di Fusco D, Troncone E, Zorzi F, et al. Sodium chloride-enriched diet enhanced inflammatory cytokine production and exacerbated experimental colitis in mice. J Crohns Colitis. (2017) 11:237–45. doi: 10.1093/ecco-jcc/jjw139
154. Muller DN, Wilck N, Haase S, Kleinewietfeld M, Linker RA. Sodium in the microenvironment regulates immune responses and tissue homeostasis. Nat Rev Immunol. (2019) 19:243–54. doi: 10.1038/s41577-018-0113-4
155. Wei Y, Lu C, Chen J, Cui G, Wang L, Yu T, et al. High salt diet stimulates gut Th17 response and exacerbates TNBS-induced colitis in mice. Oncotarget. (2017) 8:70–82. doi: 10.18632/oncotarget.13783
156. Wu C, Chen Z, Xiao S, Thalhamer T, Madi A, Han T, et al. SGK1 governs the reciprocal development of Th17 and regulatory T cells. Cell Rep. (2018) 22:653–65. doi: 10.1016/j.celrep.2017.12.068
157. Hernandez AL, Kitz A, Wu C, Lowther DE, Rodriguez DM, Vudattu N, et al. Sodium chloride inhibits the suppressive function of FOXP3+ regulatory T cells. J Clin Invest. (2015) 125:4212–22. doi: 10.1172/JCI81151
158. Sumida T, Lincoln MR, Ukeje CM, Rodriguez DM, Akazawa H, Noda T, et al. Activated beta-catenin in Foxp3(+) regulatory T cells links inflammatory environments to autoimmunity. Nat Immunol. (2018) 19:1391–402. doi: 10.1038/s41590-018-0236-6
159. Luo Y, Xue Y, Wang J, Dang J, Fang Q, Huang G, et al. Negligible effect of sodium chloride on the development and function of TGF-beta-induced CD4(+) Foxp3(+) regulatory T cells. Cell Rep. (2019) 26:1869–79 e3. doi: 10.1016/j.celrep.2019.01.066
160. Paling D, Solanky BS, Riemer F, Tozer DJ, Wheeler-Kingshott CA, Kapoor R, et al. Sodium accumulation is associated with disability and a progressive course in multiple sclerosis. Brain. (2013) 136:2305–17. doi: 10.1093/brain/awt149
161. Cortese M, Yuan C, Chitnis T, Ascherio A, Munger KL. No association between dietary sodium intake and the risk of multiple sclerosis. Neurology. (2017) 89:1322–9. doi: 10.1212/WNL.0000000000004417
162. Fitzgerald KC, Munger KL, Hartung HP, Freedman MS, Montalban X, Edan G, et al. Sodium intake and multiple sclerosis activity and progression in BENEFIT. Ann Neurol. (2017) 82:20–9. doi: 10.1002/ana.24965
163. Haase S, Wilck N, Kleinewietfeld M, Muller DN, Linker RA. Sodium chloride triggers Th17 mediated autoimmunity. J Neuroimmunol. (2019) 329:9–13. doi: 10.1016/j.jneuroim.2018.06.016
164. Lloyd-Price J, Abu-Ali G, Huttenhower C. The healthy human microbiome. Genome Med. (2016) 8:51. doi: 10.1186/s13073-016-0307-y
165. Macpherson AJ, Harris NL. Interactions between commensal intestinal bacteria and the immune system. Nat Rev Immunol. (2004) 4:478–85. doi: 10.1038/nri1373
166. Kamada N, Seo SU, Chen GY, Nunez G. Role of the gut microbiota in immunity and inflammatory disease. Nat Rev Immunol. (2013) 13:321–35. doi: 10.1038/nri3430
167. Belkaid Y, Hand TW. Role of the microbiota in immunity and inflammation. Cell. (2014) 157:121–41. doi: 10.1016/j.cell.2014.03.011
168. Lathrop SK, Bloom SM, Rao SM, Nutsch K, Lio CW, Santacruz N, et al. Peripheral education of the immune system by colonic commensal microbiota. Nature. (2011) 478:250–4. doi: 10.1038/nature10434
169. Atarashi K, Tanoue T, Oshima K, Suda W, Nagano Y, Nishikawa H, et al. Treg induction by a rationally selected mixture of Clostridia strains from the human microbiota. Nature. (2013) 500:232–6. doi: 10.1038/nature12331
170. Geuking MB, Cahenzli J, Lawson MA, Ng DC, Slack E, Hapfelmeier S, et al. Intestinal bacterial colonization induces mutualistic regulatory T cell responses. Immunity. (2011) 34:794–806. doi: 10.1016/j.immuni.2011.03.021
171. Arpaia N, Campbell C, Fan X, Dikiy S, van der Veeken J, deRoos P, et al. Metabolites produced by commensal bacteria promote peripheral regulatory T-cell generation. Nature. (2013) 504:451–5. doi: 10.1038/nature12726
172. Kim KS, Hong SW, Han D, Yi J, Jung J, Yang BG, et al. Dietary antigens limit mucosal immunity by inducing regulatory T cells in the small intestine. Science. (2016) 351:858–63. doi: 10.1126/science.aac5560
173. Haghikia A, Jorg S, Duscha A, Berg J, Manzel A, Waschbisch A, et al. Dietary fatty acids directly impact central nervous system autoimmunity via the small intestine. Immunity. (2015) 43:817–29. doi: 10.1016/j.immuni.2015.09.007
174. Poutahidis T, Kleinewietfeld M, Smillie C, Levkovich T, Perrotta A, Bhela S, et al. Microbial reprogramming inhibits Western diet-associated obesity. PLoS ONE. (2013) 8:e68596. doi: 10.1371/journal.pone.0068596
175. Smith PM, Howitt MR, Panikov N, Michaud M, Gallini CA, Bohlooly YM, et al. The microbial metabolites, short-chain fatty acids, regulate colonic Treg cell homeostasis. Science. (2013) 341:569–73. doi: 10.1126/science.1241165
176. Furusawa Y, Obata Y, Fukuda S, Endo TA, Nakato G, Takahashi D, et al. Commensal microbe-derived butyrate induces the differentiation of colonic regulatory T cells. Nature. (2013) 504:446–50. doi: 10.1038/nature12721
177. Atarashi K, Tanoue T, Shima T, Imaoka A, Kuwahara T, Momose Y, et al. Induction of colonic regulatory T cells by indigenous Clostridium species. Science. (2011) 331:337–41. doi: 10.1126/science.1198469
178. Wu HJ, Ivanov II, Darce J, Hattori K, Shima T, Umesaki Y, et al. Gut-residing segmented filamentous bacteria drive autoimmune arthritis via T helper 17 cells. Immunity. (2010) 32:815–27. doi: 10.1016/j.immuni.2010.06.001
179. Ivanov II Atarashi K, Manel N, Brodie EL, Shima T, Karaoz U, et al. Induction of intestinal Th17 cells by segmented filamentous bacteria. Cell. (2009) 139:485–98. doi: 10.1016/j.cell.2009.09.033
180. Gaboriau-Routhiau V, Rakotobe S, Lecuyer E, Mulder I, Lan A, Bridonneau C, et al. The key role of segmented filamentous bacteria in the coordinated maturation of gut helper T cell responses. Immunity. (2009) 31:677–89. doi: 10.1016/j.immuni.2009.08.020
181. Luu M, Pautz S, Kohl V, Singh R, Romero R, Lucas S, et al. The short-chain fatty acid pentanoate suppresses autoimmunity by modulating the metabolic-epigenetic crosstalk in lymphocytes. Nat Commun. (2019) 10:760. doi: 10.1038/s41467-019-08711-2
182. Häger J, Bang H, Hagen M, Frech M, Trager P, Sokolova MV, et al. The role of dietary fiber in rheumatoid arthritis patients: a feasibility study. Nutrients. (2019) 11:e2392. doi: 10.3390/nu11102392
183. Smits HH, Engering A, van der Kleij D, de Jong EC, Schipper K, van Capel TM, et al. Selective probiotic bacteria induce IL-10-producing regulatory T cells in vitro by modulating dendritic cell function through dendritic cell-specific intercellular adhesion molecule 3-grabbing non-integrin. J Allergy Clin Immunol. (2005) 115:1260–7. doi: 10.1016/j.jaci.2005.03.036
184. Kwon HK, Lee CG, So JS, Chae CS, Hwang JS, Sahoo A, et al. Generation of regulatory dendritic cells and CD4+Foxp3+ T cells by probiotics administration suppresses immune disorders. Proc Natl Acad Sci USA. (2010) 107:2159–64. doi: 10.1073/pnas.0904055107
185. David LA, Maurice CF, Carmody RN, Gootenberg DB, Button JE, Wolfe BE, et al. Diet rapidly and reproducibly alters the human gut microbiome. Nature. (2014) 505:559–63. doi: 10.1038/nature12820
186. De Filippo C, Cavalieri D, Di Paola M, Ramazzotti M, Poullet JB, Massart S, et al. Impact of diet in shaping gut microbiota revealed by a comparative study in children from Europe and rural Africa. Proc Natl Acad Sci USA. (2010) 107:14691–6. doi: 10.1073/pnas.1005963107
187. Wu GD, Chen J, Hoffmann C, Bittinger K, Chen YY, Keilbaugh SA, et al. Linking long-term dietary patterns with gut microbial enterotypes. Science. (2011) 334:105–8. doi: 10.1126/science.1208344
188. De Luca F, Shoenfeld Y. The microbiome in autoimmune diseases. Clin Exp Immunol. (2019) 195:74–85. doi: 10.1111/cei.13158
189. Nogueira AR, Shoenfeld Y. Microbiome and autoimmune diseases: cause and effect relationship. Curr Opin Rheumatol. (2019) 31:471–4. doi: 10.1097/BOR.0000000000000628
190. Cekanaviciute E, Yoo BB, Runia TF, Debelius JW, Singh S, Nelson CA, et al. Gut bacteria from multiple sclerosis patients modulate human T cells and exacerbate symptoms in mouse models. Proc Natl Acad Sci USA. (2017) 114:10713–8. doi: 10.1073/pnas.1711235114
191. Willebrand R, Hamad I, Van Zeebroeck L, Kiss M, Bruderek K, Geuzens A, et al. High salt inhibits tumor growth by enhancing anti-tumor immunity. Front Immunol. (2019) 10:1141. doi: 10.3389/fimmu.2019.01141
192. Cignarella F, Cantoni C, Ghezzi L, Salter A, Dorsett Y, Chen L, et al. Intermittent fasting confers protection in CNS autoimmunity by altering the gut microbiota. Cell Metab. (2018) 27:1222–35 e6. doi: 10.1016/j.cmet.2018.05.006
193. Morton AM, Sefik E, Upadhyay R, Weissleder R, Benoist C, Mathis D. Endoscopic photoconversion reveals unexpectedly broad leukocyte trafficking to and from the gut. Proc Natl Acad Sci USA. (2014) 111:6696–701. doi: 10.1073/pnas.1405634111
194. Esplugues E, Huber S, Gagliani N, Hauser AE, Town T, Wan YY, et al. Control of TH17 cells occurs in the small intestine. Nature. (2011) 475:514–8. doi: 10.1038/nature10228
Keywords: diet, microbiome, Treg—regulatory T cell, autoimmunity, environmental factors
Citation: Arroyo Hornero R, Hamad I, Côrte-Real B and Kleinewietfeld M (2020) The Impact of Dietary Components on Regulatory T Cells and Disease. Front. Immunol. 11:253. doi: 10.3389/fimmu.2020.00253
Received: 01 September 2019; Accepted: 30 January 2020;
Published: 21 February 2020.
Edited by:
Lucy S. K. Walker, University College London, United KingdomReviewed by:
Xing Chang, Westlake Institute for Advanced Study (WIAS), ChinaChristina E. Zielinski, Technical University of Munich, Germany
Copyright © 2020 Arroyo Hornero, Hamad, Côrte-Real and Kleinewietfeld. This is an open-access article distributed under the terms of the Creative Commons Attribution License (CC BY). The use, distribution or reproduction in other forums is permitted, provided the original author(s) and the copyright owner(s) are credited and that the original publication in this journal is cited, in accordance with accepted academic practice. No use, distribution or reproduction is permitted which does not comply with these terms.
*Correspondence: Markus Kleinewietfeld, bWFya3VzLmtsZWluZXdpZXRmZWxkJiN4MDAwNDA7dWhhc3NlbHQudmliLmJl