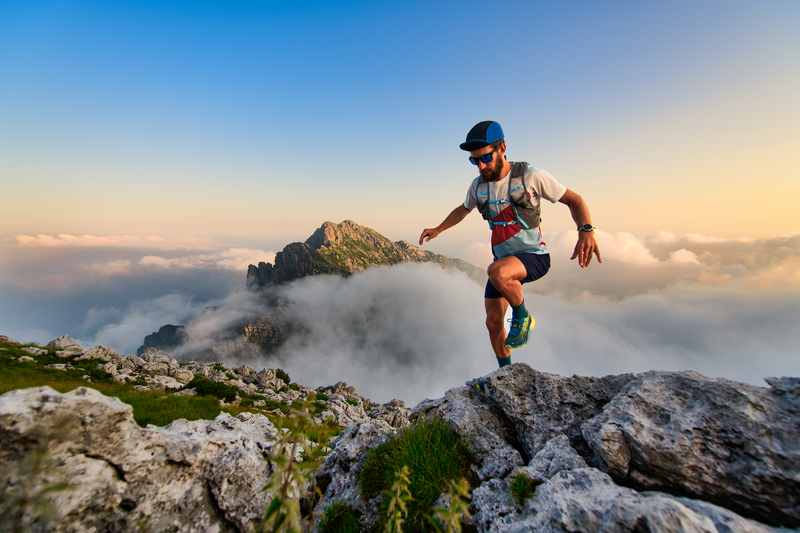
94% of researchers rate our articles as excellent or good
Learn more about the work of our research integrity team to safeguard the quality of each article we publish.
Find out more
REVIEW article
Front. Immunol. , 07 February 2020
Sec. Antigen Presenting Cell Biology
Volume 11 - 2020 | https://doi.org/10.3389/fimmu.2020.00134
This article is part of the Research Topic Sentinel CLECs at Immunological Decision Nodes View all 9 articles
C-type lectin receptors (CLRs) are important in several immune regulatory processes. These receptors recognize glycans expressed by host cells or by pathogens. Whereas pathogens are recognized through their glycans, which leads to protective immunity, aberrant cellular glycans are now increasingly recognized as disease-driving factors in cancer, auto-immunity, and allergy. The vast variety of glycan structures translates into a wide spectrum of effects on the immune system ranging from immune suppression to hyper-inflammatory responses. CLRs have distinct expression patterns on antigen presenting cells (APCs) controlling their role in immunity. CLRs can also be exploited to selectively target specific APCs, modulate immune responses and enhance antigen presentation. Here we will discuss the role of glycans and their receptors in immunity as well as potential strategies for immune modulation. A special focus will be given to different dendritic cell subsets as these APCs are crucial orchestrators of immune responses in infections, cancer, auto-immunity and allergies. Furthermore, we will highlight the potential use of nanoscale lipid bi-layer structures (liposomes) in targeted immunotherapy.
The immune system faces a plethora of challenges every single day, ranging from contact with pathogens to the identification of malignant cells. It is becoming evident that the glycome has a crucial role in immunology with glycans and glycan-binding proteins, such as C-type lectin receptors (CLRs), being important regulators in the balancing act between disease and homeostasis. Prominent examples are the recognition of specific glycan signatures by CLRs on immune cells and subsequent immune responses toward pathogens and cancer cells as well as aberrant reactions toward specific foreign proteins leading to the development of allergies. Hence, one of the key parameters that supports effective defense mechanisms is protein and lipid glycosylation. Protein glycosylation is defined by the covalent linkage of carbohydrates to proteins. With more than half of the cellular proteins showing attachment of sugar chains in various length and structure (1), glycosylation increases proteomic diversity more than any other post-translational modification and has a broad impact on protein functions. The development and extent of glycosylation is a coordinated, enzyme-driven process in which several 100 enzymes, such as glycosidases and glycosyl transferases, determine the specific glycan signature of a cell together with the availability of activated sugar donor substrates and the accessibility to glycan modification sites (1–3). Hence, glycobiology is a complex field to study given that a much greater amount of variables has to be considered than embedded in genetic coding. However, the past years yielded important breakthroughs in methods of synthetizing glycans and knowledge on glycans in immunity. Why glycosylation has a highlighted role in the field of immunology and how these glycans can be used in clinical applications will be discussed in this review.
Every cell is covered with a dense coat of glycans (4, 5) with typical glycosylation patterns helping to distinguish between self and foreign proteins of invading pathogens. Notably, all key proteins involved in the recognition of antigen and downstream effector functions are glycosylated (6, 7), which points toward a central role of glycobiology in immune responses. Alterations in glycosylation can occur in response to environmental or genetic stimuli. Well-known examples are changes of glycan patterns during tumorigenesis, where N-glycan structures are altered on tumors together with a higher presence of mucins or sialic acids in the glycan shield of malignant cells (8–11).
With respect to humoral immunity, glycosylation of the immunoglobulin (Ig) Fc domain influences the biological activity of antibodies by interaction with complement and Fcγ receptors (FcγR), but might also affect CLR recognition (12–14). Alterations in glycosylation of the variable domain may contribute to the pathogenicity of autoantibodies, which has been shown in the case of anti-citrullinated protein antibodies (ACPA) and may lead to a breach in tolerance or persistence of inflammation in rheumatoid arthritis (15, 16). Thus, it is important to understand the role of CLRs in maintaining homeostasis, affecting anti-tumor responses or how these receptors induce protective immunity to infections.
Dendritic cells (DCs) are the professional APC of the human innate immune system, and therefore instrumental in determining T cell polarization by the secretion of certain cytokines and chemokines. DCs reside in mucosal tissues and sample their environment for pathogens and inflammation. Pathogens express pathogen-associated molecular patterns (PAMPS), whereas inflammation is recognized by sensing damage associated molecular patterns (DAMPS) (17). Some of these signals are sugars or glycosylated structures, and therefore can be recognized by a specific group of pattern recognition receptors (PRRs) on the surface of APCs. CLRs are one type of PRRs which are equipped with a carbohydrate recognition domain that specifically recognizes glycan moieties on host cells, tumor cells as well as pathogens. CLRs are expressed at high density on the surface of DCs and have been shown to be important instructors of T cell immunity (5).
Myeloid CLRs are predominantly surface transmembrane proteins that sense endogenous and/or exogenous ligands (18–20). The C subclass refers to the necessity of calcium needed for the binding of a diverse repertoire of carbohydrate ligands, including various fucose-, mannose- and galactose-carrying structures (21, 22). CLRs are structures with diverse functions that, next to their role as adhesion molecules and endocytosis receptors, dictate immunity toward various pathogens, cellular proteins and lipids (21–26). CLR signaling is a complex phenomenon by itself, but can also interfere in signaling pathways induced by various other myeloid PRRs (18, 27, 28) and hence provides a variety of possibilities in immunological responses and potential applications in the field of immunotherapy. Several CLRs are linked to canonical cytoplasmic signaling motifs, which shift signaling routes into either activating or inhibitory directions. An activating signal is generally associated with the presence of an immunoreceptor tyrosine-based activation motif (ITAM) as intracellular domain (18, 26, 29). The ITAM domain, which leads to Syk kinase activation (Figure 1), is either embedded within the cytoplasmic tail of the receptor or acquired via engagement with ITAM-bearing FcRγ adaptor molecules (29, 30). The latter includes the CLRs Mincle, Dectin-2, and BDCA-2, where Syk activation induces transcription of pro-inflammatory cytokines by activating subunits of the transcription factor NF-κB complex (18, 20); Several other CLRs, such as Dectin-1 and CLEC9A carry a hemITAM motif, which likewise involves signaling via Syk (18, 31–34). Dectin-1, a CLR that recognizes β-glucans on the surface of a variety of bacteria and fungi (35, 36), triggers an activating immune response upon ligand binding where Syk induces both canonical and non-canoncical NF-κB signaling via canonical subunits p65 and c-Rel and the non-canonical subunit RelB. Activation of both NF-κB pathways promotes the transcription of pro-inflammatory cytokines, such as TNF, IL-6, and IL-23 (27, 37). Nevertheless, although Syk signaling is generally associated with an activating signal, not all ITAM- or hemITAM-bearing CLRs result in the same transcriptional program and can hence also lead to inhibitory signaling outcomes. As such, CLEC9A interacts with endogenous ligands, in particular danger associated molecular patterns (DAMPs). CLEC9A senses tissue damage and reduces excessive inflammatory responses to delimit host tissue damage and hence preserves homeostasis (20, 38). A third group of CLRs is coupled to an intracellular immunoreceptor tyrosine-based inhibition motif (ITIM) domain (20, 26, 39). ITIM signaling can modulate the immune response induced by kinase-associated heterologous receptors via the recruitment of phosphatases, which counteract kinase-associated receptors like the Syk-coupled CLRs mentioned above (Figure 1). Within this group, DCIR senses self-associated patterns and acts to maintain homeostasis of the immune system (18). However, there is also a group of CLRs that carries none of the above-mentioned motifs. Members of this group include MR, Dec-205, langerin, and DC-SIGN. DC-SIGN is expressed in large quantities on the surface of DCs and macrophages. This receptor binds to mannosylated and fucosylated residues (40) and leads to the internalization of the bound ligands into the lysosomal pathway resulting in MHC-II presentation. DC-SIGN represents a prime example of CLRs that tailor immune responses toward specific self and foreign structures in interaction with the signaling of other PRRs. It has been reported that DC-SIGN recognition of mannose-carrying PAMPs expressed by Mycobacterium tuberculosis and HIV-1 initiates the activation of the kinase Raf-1. Raf-1 modulates Toll-like receptor (TLR)-induced NF-κB activation to enhance the production of pro-inflammatory cytokines, such as IL-6 and IL-12 (27, 28). However, upon recognition of fucose-carrying PAMPs being expressed by Schistosoma mansoni and Helicobacter pylori, DC-SIGN signaling leads to a suppression of the TLR-4-induced pro-inflammatory responses via inhibition of IL-12, enhanced secretion of IL-10 and the TH2- attracting chemokines CCL17 and CCL22. Fucose recognition by DC-SIGN abrogates TH1 and TH17 responses and hereby favors a TH2 outcome (27, 28). Thus, CLRs also have carbohydrate-specific signaling properties, which adds another level of immunomodulatory flexibility to this family of PRRs.
Figure 1. Canonical signaling motives and pathways of C-type lectin receptors. C-type lectin receptors (CLRs) are a group of carbohydrate-recognizing surface receptors, being expressed at high density on myeloid cells, such as DCs. The immunoreceptor tyrosine-based activating motif (ITAM), hemITAM, and immunoreceptor tyrosine-based inhibitory motif (ITIM) are canonical signaling motifs downstream of the CLR domain that influence immune response upon ligand binding by either activating kinases (e.g., Syk) or phosphatases (SHP-1, SHP-2).
As reviewed by Iborra and Sancho (18), not only the cytoplasmic signaling motif, but also the nature of the ligand plays an important role in the immunological outcome of CLR signaling. Ligand affinity and avidity can modify quantity and duration of signaling via the ITAM domain (18, 41, 42): while high-avidity ligands induce an activating signal, ligands of lower avidity result in hypophosphorylation of the ITAM domain, referred to as “inhibitory ITAM” (20). The aggregated state of the ligand modifies its affinity with the respective CLR, as soluble and particulate ligands are differentially sensed by their receptors, with the soluble version generally being a poor trigger of activating signaling (18, 20). A further important determinant for CLR signaling is particle size, as the presentation of CLRs on particulate structures rather than in soluble form may promote delayed phagocytosis. Smaller particles are endocytosed rapidly, which leads to a faster signal attenuation, while delayed phagocytosis results in an enhanced cytokine production (18, 36, 43, 44). Furthermore, the location of the respective CLR can be decisive, as different cell types may express different isotypes of the receptor resulting in different cellular locations (20).
Taken together, CLRs read specific carbohydrate signatures, initiate individually tailored immune responses, or modulate responses initiated by other PRRs. Accordingly, DCs initiate the expression of specific cytokines that lead to the polarization of naïve T cells into specialized subsets (Figure 2). As CLRs are differentially expressed on different DC subsets, targeting of these subsets via CLRs could harness their power to achieve a very specific immune modulatory effect in future innovations.
Figure 2. C-type lectin receptor signaling shapes immune responses by steering T cell polarization. Upon binding of glycosylated proteins (GP) to C-type lectin receptors (CLR) on dendritic cells (DC), a downstream signaling cascade is becoming activated and can remodel signaling pathways induced by heterologous receptors, such as Toll-like receptors (TLR). The signal initiates the transcription of specific cytokines and chemokines, followed by the secretion of the expressed mediators by the DC. Furthermore, the CLR acts as an endocytosis receptor facilitating the uptake of the GP by the DC. The endocytosed GP is degraded into peptide fragments within compartments of the endocytic processing pathway. These antigenic peptides bind to MHC-II molecules, which are produced in the rough endoplasmic reticulum (RER) and fuse into the endocytic processing pathway. Upon binding of antigenic peptides to MHC-II, the formed complex is transported to the plasma membrane of the DC, where it allows for presentation of antigenic peptides to naïve CD4+ T helper cells (TH) being equipped with the cognate T cell receptor (TCR). This activating signal together with the pro- or anti-inflammatory character of the secreted cytokines determines the polarization of naïve CD4+ T helper cells into specialized subsets. In the context of cross-presentation, ingested antigen can furthermore be presented to cytotoxic CD8+ T cells, which is mediated via MHC-I molecules.
Several DC subtypes have been distinguished based on crucial cell surface markers, cytokine production as well as genetic imprint (45). Detailed analyses of expression of surface markers and the transcriptome reveal four major subtypes of human DCs: CD141+ DC, CD1c+ DC, plasmacytoid DC (pDC) and monocyte-derived DC (moDC). CD141+ DCs appear as major producers of type III interferons and play a role in anti-viral as well as anti-tumor responses via the induction of cytotoxic T lymphocytes. CD1c+ DCs can efficiently induce TH17 cells, and produce IL-12, making them proficient in immune responses against intracellular bacteria and fungi (46). The CD1c+ subset appears to be more sensitive to stimuli from the local microenvironment and may display differing functionality in different tissues (47). pDCs are known for their pro-inflammatory IFN type I production which implicates them in various auto-immune diseases ranging from psoriasis to lupus (48–50), yet have also been connected to immunosuppression in transplants and anti-allergic responses (51–53). In contrast to the previously listed subtypes, moDCs have a CD14+ monocyte-like imprint and stem from distinct bone marrow precursor cells (54). Infiltrating DCs in tissue from several autoimmune diseases are related to in vitro generated moDCs (55, 56). Tissues rich in immune cells, such as lung, liver, gut and skin tissue, harbor a range of the different DC subsets mentioned above, all adapted to their specific niche, whereas some tissues harbor distinct DC subtypes. Human skin hosts two other important DC subtypes: Langerhans cells (LCs) and CD14+ dermal DCs (45). LCs express langerin and E-cadherin on their surface and can induce CD4+ T cells of a TH2 phenotype as well as activate CD8+ T cells via cross-presentation (57–61). The effect of LCs on the induction of distinct T cell phenotypes might depend on the antigen as measles virus and HIV-1 capture by LCs does not lead to cross-presentation (62–64). In contrast, LCs are able to transfer antigens to other DCs, facilitating cross-presentation (63). Dermal DCs are able to induce Tregs but this also depends on the activation signal (59, 65). Studies analyzing the transcriptome of dermal DCs have shown that these cells, just like moDCs, stem from monocytes, suggesting that dermal DCs are macrophages (66). Even though there is plasticity between different DC subtypes and functional distinctions are often less clear, one could hypothesize that different DC functions might depend on the repertoire of CLRs and other PRRs that are expressed by the respective subset. Thus, the possible CLR-induced signaling routes can be exploited by targeting specific CLRs to induce or silence immune responses.
Functional characteristics of DC subsets can be addressed by using subset-specific CLR targeting strategies and help guide the immune response in desired directions. Shifting the focus to targeted immunotherapy for systemic applications led to the emergence of cellular vaccines. The first FDA-approved product from this category was Sipuleucel-T, an anti-cancer vaccine in which autologous monocytes are differentiated ex vivo to DCs and incubated with a fusion protein that consists of a common prostate cancer antigen linked to an adjuvant. The loaded DCs are subsequently infused into the patient (67, 68). The technology of personalized cellular immunotherapy quickly spread over to other clinical fields, such as autoimmune disease (69, 70). However, this approach is very expensive and laborious. Moreover, DC progenitors are used and differentiation into DCs ex vivo might fail to provide all the required signals. Current technologies aim to circumvent the isolation of monocytes and their in vitro differentiation to DCs with subsequent antigen loading and activation. Therefore, major focus arose for in vivo targeting of DCs. However, the use of undirected drug delivery systems carries high risks that the drug will be taken up by by-stander cells in the injection niche rather than by DCs. This led to the emergence of delivery techniques that specifically target DCs, and hence allow to manipulate DC-dependent orchestration of immune responses.
One of these strategies includes targeting DCs via antibodies. This concept was pioneered by Steinmann and colleagues, who targeted Dec-205 on DCs for antigen delivery with a recombinant IgG construct (12, 71). Inspired by the new possibilities several other groups drove the field of in vivo targeting by antibodies forward and expanded it to other CLRs, including DC-SIGN, langerin, Dectin-1 and MR (72–75). A detailed collection of conducted studies is provided by Lehman et al. (76). Different approaches have been used to create antibody-linked antigens. Next to the recombinant expression of an antibody-antigen fusion construct, another possibility is to chemically link the antibody to the antigen (76). Recombinant production has the advantage that the targeting antibody can be genetically modified within the cloning process. This allows for humanization, improved stability, and modifications of the Fc region (13, 76, 77). The latter is frequently applied to minimize unwanted interaction with FcRs that are present on several immune cell types. In the same way, mutations in the IgG Fc region could also allow to modulate DC signaling triggered via the FcR. Downscaling the targeting antibody to only the Fab or scFv region displays a further way to avoid unwanted FcR binding (78, 79). Antibody glycosylation itself also displays a conceivable route to bypass unwanted FcR interactions. A highly conserved glycosylation site (Asn297) is found at the IgG Fc domain, which carries complex N-glycan structures (80, 81). Glycan substitution could lead to reduced binding to respective receptors: A lack of IgG core fucosylation was shown to increase affinity for FcγRIIIA (82), while terminal sialylation generally appears to decrease affinity to FcγRs (83). Another targeting approach includes the interaction of biotinylated antibodies with streptavidin-coupled antigens (84). When using an antibody-based targeting strategy, the choice of specific targeting epitopes within the CLR showed to be meaningful as well: Targeting DC-SIGN with different antibodies against the carbohydrate recognition domain (CRD) or neck region lead to differential levels of internalization, routing and T cell presentation. While anti-CRD-antibodies are preferentially routed to lysosomal compartments, anti-neck-antibodies reside prolonged in early endosomal compartments, which is generally associated with increased cross-presentation (85). This suggests that the neck domain of DC-SIGN represents an interesting target for DC-specific vaccination approaches, especially in the field of cancer vaccines. Nevertheless, the intracellular trafficking mechanisms of cross-presentation have not been fully elucidated yet. Hence, despite being routed to lysosomal compartments, antigens targeted to the CRD of DC-SIGN can also be cross-presented. Therefore, targeting antigens to distinct endosomal compartments via different receptor epitopes can, due to different protease activities in different compartments, result in the presentation of diverse epitopes, which broadens the peptide repertoire presented to T cells. However, it is important to consider that antibodies have the potential to activate or block several receptors, including CLRs, and this could result in unwanted or unknown immune responses. Still, the signaling inducing capacity of antibodies could also be used as an advantage to act as the adjuvant or enhance the adjuvant activity. Hence, the usage as targeting strategy has many opportunities.
Targeting DCs with antibodies against DC-specific markers allows high selectivity, sometimes to the depth of reaching only one particular DC subset due to the specific expression of a CLR. The CLR CLEC9A is highly expressed by CD141+ DCs. Using a chimeric anti-CLEC9A antibody fused to a cytomegalovirus (CMV) antigen, the CD141+ DCs were specifically targeted, which resulted in significantly more robust cross-presentation to antigen-specific CD8+ T cells compared to non-targeted antigen (86). Although not superior to non-targeted constructs in terms of cross-presentation in vivo, injections of a fluorescent variant of the anti-CLEC9A antibody into mice with a humanized immune system showed CD141+ DC-subset specific uptake, which yields another piece of proof for subset-specific targeting.
Injection of antibodies against langerin or Dec-205 in human skin biopsies resulted in highly specific uptake of anti-langerin by LCs, but less specific uptake by anti-Dec-205 targeted CD1a+ dermal DCs (87). This finding demonstrates that LCs can be targeted effectively, while Dec-205 is also expressed in various types of leukocytes, which reduces selectivity (88). These results highlight the importance of subset markers that are less redundantly expressed by other APCs. However, many DC subset defining markers are also expressed on other APC, which complicates the picture of reaching one subset only. Nevertheless, targeting a more ubiquitously expressed APC marker does not necessarily result in a functional disadvantage as long as it provokes a synergistic immune effect. When epidermal mouse LCs were targeted with ovalbumin (OVA)-coupled antibodies against Dec-205 or langerin via intradermal injection, a successful uptake of the antigens by the LCs was observed. However, only Dec-205-targeting resulted in potent antigen presentation to CD4+ and CD8+ T cells. LCs targeted by anti-langerin were also unable to trigger T cell proliferation (72). Thus, even though langerin is LC-specific, targeting to the more ubiquitous CLR leads to functional immune activation. Moreover, a more broadly expressed CLR also allows for a broader response and reaches different DC subsets. Targeting of the CLR DCIR on monocytes, CD1c+ DCs and pDCs with antibodies coupled to liposomes containing a TLR7 agonist, enhanced uptake by monocytes and CD1c+ DCs over 10-fold compared to controls and activated both pDCs and DCs (89). Thus, using CLRs that show more ubiquitous expression patterns on APC can lead to synergistic immune modulation.
A second strategy for CLR targeting exploits the potential of natural or artificial glycans as CLR ligands. This strategy allows for fine tuning of the CLR-ligand interaction by adjusting the multivalency and spatial orientation of the ligand, which leads to higher targeting efficiency and CLR clustering (90). Several studies targeting DC-SIGN, MR, and langerin confirm a preferential uptake and presentation of antigens when being guided by specific glycan structures (90–93). In the field of allergen immunotherapy, the creation of neo-glycoconjugates was applied as a ligand-based strategy for targeting DCs. Non-oxidized, yeast-derived mannan structures were coupled to allergoids and these neo-glycoconjugates were reported to enhance allergen uptake via MR, DC-SIGN, and Dectin-2 by human moDCs (94, 95). Using sugars to target CLRs on DCs has the drawback that CLRs are often expressed by different subsets and different CLRs have similar carbohydrate specificities. Targeting more ubiquitously expressed CLRs or using more universal sugar ligands could also be a benefit as more DC subtypes are targeted, as long as the immune response induced by the different DCs is similar. Still, a potential solution for improving specificity could be the synthesis of glycans that are highly specific for one CLR. Recently, a glycomimetic ligand was synthesized that binds specifically langerin but not DC-SIGN (93). Thus, chemical engineering of sugars as CLR ligands could be very valuable to enhance targeting specificity and might also be used to induce specific signaling via the CLRs.
As knowledge and attention for glycan-based targeting strategies is rapidly growing, CLR-ligands offer more targeting approaches that are frequently applied in practice, especially in combination with nanoparticle carrier systems. Tri-mannose ligands anchored to liposomes showed an increased liposome uptake in human moDCs compared to unmodified or simpler mannosylated liposomes (96). Making use of the difucosylated oligosaccharide Lewis Y (LeY) as an antigen for targeting langerin on LCs and DC-SIGN on moDCs led to efficient internalization by DC-SIGN+ moDCs and antigen presentation to tumor antigen specific CD4+ and CD8+ T cell lines in vitro (97). Hereby, LeY-loaded liposomes were used as drug carriers. Interestingly, these liposomes were readily internalized by DC-SIGN+ moDCs but in spite of binding to langerin, were not endocytosed by LCs. This study sheds light on further aspects such as particle size and conformation that have to be considered for subset specific targeting and therefore displays another variable that should be considered when designing nanoparticle-based drug carrier systems.
Commonly used carrier systems for therapeutic components are liposomes, which are spherical nanoscale structures that consist of a lipid bi-layer. These nanostructures allow for the packaging of all important components of a cellular vaccine into one spatial compartment, with a possibility to anchor a targeting label together with simultaneous delivery of antigens and adjuvant to the targeted cellular components (98, 99). Furthermore, liposomes shield the packaged content from premature degradation and can be manufactured in different shapes and sizes, which in turn allows for the accommodation of differing uptake requirements of different APC. Both antibodies and glycan structures can be anchored to liposomes for mediating immune cell type-specific uptake, enhancing targeting efficiency and immune modulation.
The following examples illustrate how the usage of liposomes as drug delivery systems became a popular approach in the development of novel vaccines. Broecker et al. used the cancer-associated glycan-α-N-acetylgalactosamine coupled to a glycosphingolipid as a vaccine model antigen. This model antigen elicited a more robust antigen specific humoral response after in vivo immunization of mice when administered in a liposomal formulation compared to injection of model antigen in its naked form (100). Interestingly, the size of the liposomes had an effect on the induced IgG subtype. Liposomes around 400 nm in size provoked IgG2a, thus more TH1-primed antibody responses, while 120 nm sized liposomes led to an induction of IgG1 antibodies, pointing toward TH2 priming. Liposome-based delivery can also be modulated by considering liposomal characteristics such as shape, rigidity and electric charges (98). For example, sialic acid residues are anionic glycans often present on proteins of tumor cells, contributing to a survival advantage of tumor cells via the engagement of sialic acid receptors (Siglecs) (101, 102). These anionic sugar moieties attract positively charged structures. To block anionic sialic acid sites on cancer cells, a study reported on a cationic liposomal formulation that efficiently inhibited the growth of lung cancer cells in vitro, whereas using the naked inhibitor did not result in significant blockage of proliferation, indicating a beneficial effect of oppositely charged liposome structures for relaying the drug of choice to its proper therapeutic target (103). While formulations with a cationic charge can enhance cellular uptake due to a favorable interaction with the negatively charged cell membrane, this effect reduces cell-specificity. Depending on the desired effect- reaching a broad set of cells or specific immune cells- electric charge of the liposomes has to be taken into account (98). Thus, the possibility to control size and composition of the liposomes themselves might open interesting ways for immune modulation.
Most importantly, bringing the antigens and adjuvants into close proximity allows for a superior uptake and immune effect compared to the use of non-targeted soluble compounds. Liposomes loaded with the Neisseria meningitidis antigen PorA and mannose were taken up more efficiently by bone marrow derived mouse dendritic cells (BMDCs) compared to non-targeted PorA liposomes, and unlike the non-glycosylated liposomes, induced IL-12 production, which points to a superior immune activating potential of BMDC-targeted liposomes (104).
Liposomes coupled to a neoglycolipid containing mannotriose residues targeted human mononuclear phagocytes and induced co-stimulatory molecules as well as pro-inflammatory cytokines. This effect was observed without the use of TLR agonists or pro-inflammatory cytokines pointing to an immune modulating effect of the liposomes and the sugar ligand without the need for additional adjuvants (105). This points to the possibility that certain liposomal formulations are immunogenic by themselves and could contribute to immune modulation. Glycoliposomes targeted to DC-SIGN via the glycan Lewis X were taken up efficiently by moDCs via DC-SIGN (106). Inclusion of a TLR4 ligand induced more efficient antigen presentation to CD8+ T cells, compared to the soluble TLR4 ligand alone. Hence, it appears logical to combine glycan-based CLR targeting with suitable adjuvants in liposomal formulations, or with specific liposomes that have an immune modulatory effect, to skew the immune response in a desired direction.
As mentioned previously, the problem of off-targeting might not be that relevant as long as this results in a uni-directional immune response. Battling infectious diseases and cancer requires immune activation without exhaustion and inflammation, whereas allergy and auto-immune diseases require the attenuation of inflammation and the induction of tolerogenic immunity. This directionality can be aided tremendously by using the proper adjuvant in cellular vaccine formulations and immune therapy. Next to TLR ligands, more and more interest arises for exploring the immunomodulatory qualities of glycans as adjuvants, albeit evidence on their adjuvant properties is still scarce.
In the anti-cancer field breaking tolerance and anergy in the tumor microenvironment poses the biggest challenge and potent adjuvants are needed to break the tolerance. Therefore, it appears logical to assess sugars that are ligands for (hem)ITAM-associated CLRs. One of these is β-glucan, a polysaccharide that is currently investigated in the field of cancer research. β-glucan acts on Dectin-1, leading to the activation of DCs, TH1 cytokine production and the expansion of tumor antigen specific T cells [reviewed in (107)]. In the case of breast cancer, tumor-infiltrating DCs promote an inflammatory TH2 response. The β-glucan Curdlan has been reported to reprogram tumor-infiltrating DCs by inducing the expression of IL-12p70 according to Dectin-1 signaling, thereby favoring a TH1 response. A human mouse model for breast cancer demonstrated that these Dectin-1-activated DCs enhance anti-tumor CD8+ T cells and inhibit tumor growth (108). Currently, β-glucan is tested in clinical trials for safety and efficacy with IV administration, as a booster for chemotherapies, and future studies are expected with this component as a therapeutic vaccine adjuvant.
For infectious diseases, one of the biggest challenges of developing effective vaccines is the induction of potent antigen-specific T cell responses. Glycosylated adjuvants can also help further this field. A glycoprotein from Lactobacillus kefiri was shown to boost LPS-dependent macrophage activation in vitro, a mechanism demonstrated to be dependent on the ITAM-bearing CLR Mincle, together with its cytoplasmic modulator CARD9 (109). This shows the importance of the identification of novel adjuvants derived from non-pathogenic microorganisms. However, as glycosylation can also affect lipids, glycolipids can also be exploited as immunomodulatory adjuvants. A synthetically modified version of the glycolipid lipomannan, derived from M. tuberculosis, was used as a conjugate to tetanus toxoid in mice, which resulted in a synergistic boosting of IFN-γ, IL-2, IP-10, and TNF-α levels in splenocytes of immunized mice (110).
Less is known about the use of glyco-conjugate adjuvants for the induction of tolerogenic immunity. Most popular adjuvant compounds in studies that aim for the induction of tolerogenic DCs include Vitamin D, Vitamin A, dexamethasone and the widely-used canonical NF-κB inhibitor BAY-117082 (111). The underlying principle of using these adjuvants is the inhibition of pro-inflammatory TH1 and TH17 responses. For the treatment of autoimmune diseases like rheumatoid arthritis, two vaccine strategies are commonly applied: One strategy involves immune silencing using a self-antigen coupled to a tolerogenic cytokine, whereas the other strategy is immunogenic in nature and employs an adjuvant capable of shifting the cellular response in a TH2 direction (112). Especially the latter strategy can be supported by using glycan-based derivatives that mimic PAMPs on parasites (113, 114). Several glycans derived from the parasite S. mansoni have been shown to interact with CLRs on human macrophages and DCs in vitro leading to suppressive immunity and a TH2 bias. Moreover, it has been demonstrated that engagement of the parasite-derived glycans on CLRs results in crosstalk with TLR-induced signaling, a crosstalk which can override the pro-inflammatory effect of LPS on the TLRs and leads to a TH2, or Treg immune signature (115).
An alternative approach to inducing disease specific tolerance is the use of strong tolerogenic adjuvants, such as Vitamin D3, which can have a general immune overriding effect, by an induction of tolerogenic DC that stimulate IL-10 or FoxP3+ Tregs and silence the activating TH1-TH17 cell response (116). This approach is especially relevant for allergies, where tailoring a desired tolerogenic immune response poses even more challenges as it is limited to immune silencing strategies which do not support TH2 immunity. One of the biggest challenges of treating allergy via immunotherapy is that induction of long-lasting memory for an allergen-specific anti-inflammatory immune response, i.e., for sustained efficacy, requires long-term administration of high allergen doses (3–5 years). Appropriate adjuvants, such as Vitamin D3, could help overcoming this barrier by inducing protective anti-inflammatory responses more effectively, rapidly and stably, allowing for lower-dose treatment (116–118). Similarly to Vitamin D3, glycans might be applicable as tolerogenic adjuvants. Current innovations of allergen immunotherapy make use of the previously mentioned mannan-based neo-glycoconjugate platform (94, 95). The benefits of this approach are not only limited to a superior targeting of DCs and antigen uptake. Coupling non-oxidized mannan to allergoids also induced Treg cells via upregulation of PD-L1 on human DCs and proved efficacy by showing in vivo hypoallergenicity and induction of blocking antibodies (95). The potential of this approach is currently investigated in clinical trials. Another potential strategy for the induction of Tregs adopts one mechanism of tumor cells for creating immune suppression in their microenvironment through the use of sialylated antigens, which can tolerize DCs via the interaction with Siglec-E. Loading of DCs with sialylated antigens resulted in the antigen-specific induction of de novo Tregs and the inhibiton of auto-reactive T cells in mice (119). Thus, growing evidence suggests glycans harboring immunomodulatory potential, a potential that possibly can revolutionize future immunotherapies.
The wheel around glycosylation keeps spinning and therefore many groups are exploring further possibilities to make use of carbohydrates for immunological applications. Glycoconjugate drugs gain popularity, but a major problem might be that these drugs will also be recognized by different CLRs possibly resulting in less desired responses. A recent study reports on a synthetic glyco-adjuvant named p(Man–TLR7), which is composed of a TLR7 agonist together with mannose in a co-polymer nanostructure (120). p(Man-TLR7) modulates immunity by binding both TLR7 and mannan-recognizing CLRs on DCs. The glyco-adjuvant was conjugated to model antigens via a self-immolative linkage, which facilitates the release of chemically unmodified antigen after endocytosis and thereby amplifies antigen presentation to T cells. This antigen-p(Man-TLR7) platform was demonstrated to achieve superior humoral and cellular immunity and hence presents a new strategy to enhance the immunogenicity of future vaccines. It is becoming clear that CLRs carry the potential to boost vaccine effectiveness and may also be used to target vaccines selectively to certain cell-types. Consequently, new drug formats are in constant development. As described previously, antibodies are a common way to target CLRs. However, antibodies also carry glycosylation structures and therefore can bind GBPs, which makes them not only interesting for targeting purposes but also for immune modulation. Massoud et al. investigated the interaction of IVIg with CD11c+ DCs, aiming for the identification of novel receptors for intravenous immunoglobulin (IVIg), a preparation of pooled human polyclonal IgG that is used as immune-modulatory therapy in autoimmune and inflammatory disease (121). They demonstrated the requirement for IgG sialylation for the induction of tolerogenic DCs and identified DCIR as a novel receptor for sialylated IgG. DCIR is an ITIM-linked CLR that induces inhibitory signals via phosphatase activation. Furthermore, DCIR facilitates the internalization of ligands, which is crucial for the induction of Tregs. The identification of this receptor in the context of tolerance induction opens up new possibilities for future innovations in the field of tolerogenic immunotherapies. Another interesting approach is the co-delivery of several components encapsulated in nanoparticle structures, such as liposomes. Co-delivery of antigens together with suitable adjuvants can potentially prime DCs to induce a wanted T cell reaction toward the antigen. Furthermore, these liposomes could be modified to target specific CLRs via one of the previously mentioned strategies (Figure 3). By shedding light on various adjuvants and the respective effects of CLR signaling one could build a platform, which is not only limited to one disease but can be extended further to several immunological disorders. Hence, combining the knowledge of glycobiology and immunology leads to endless possibilities for immune modulatory therapies. Therefore, it will remain crucial to investigate the identification of novel DC subsets, receptors, and sugars. Existing studies focusing on glycan identification serve as proof of concept. Parameswarappa et al. report about the identification of immunogenic glycotopes to boost efficacy of S. pneumoniae vaccines and identified a promising tetrasachharide that induced superior immunological protection against pneumonia, as observed in mice. Hence, using this glycan array screening, the weakest immunogenic serotype of the 13-valent pneumococcus vaccine could be improved (122). A further interesting cell-based glycan array was described to probe glycan-GBP interactions (123). This platform's fundament is the lectin-resistant Chinese hamster ovary (CHO) cell mutant Lec2 that expresses a narrow and relatively homogenous repertoire of glycoforms. By using recombinant glycosyltransferases, several carbohydrate structures can be installed on the cell surface. Probing these surface glycan epitopes with fluorescently labeled GBPs allows for high-throughput profiling for strong interactions directly on the cell surface. These examples illustrate a rising interest in glycobiology, a field that was long time neglected and might enter its blossom phase in the coming years. Also beyond the borders of immunology, the role of glycosylation is no illusion anymore, and therefore we are expecting glycobiology to drive further key-insights into several aspects of health and disease.
Figure 3. Potential CLR-targeting drug-delivery applications. Liposomes are nano-scale particles, surrounded by a membranous phospholipid-bi-layer and can as such be applied as a drug delivery vehicle to target antigens to DCs. Surface modifications, for instance by antibodies targeting CLRs (e.g., DC-SIGN) or glycoproteins as CLR ligands allow for in vivo targeting of specific DC subsets via their CLR repertoire and may also interfere with downstream signaling. The aqueous phase of the liposome can harbor several molecules, and by this manipulate immunological signaling. With the given advantage of liposomes bringing the involved components into close proximity, novel immunotherapeutic drugs can be designed that make use of co-delivery, e.g., by combining antigens with immunomodulatory adjuvants and by this manipulate TH cell skewing.
The role of glycans in immunity is multi-faceted and overarches both the innate and adaptive branches of the immune system. Antigen presentation by DCs to T cells as well as T cell polarization are processes shaped by glycans and CLRs, highlighting the central influence of the glycome on the innate and adaptive immune system. DCs fulfill a unique role within the human immune system by orchestrating innate and adaptive immune responses. Recognizing this potential, it is worth investing future research efforts to delineate the expression pattern of CLRs in DC subsets, their function for the respective DC subset and their applicability for future innovations, including targeting of (nano)vaccines to specific cell types. Current knowledge gives evidence for immunomodulatory effects of triggering CLRs. Hence, glycosylated structures could be applied as glyco-adjuvants to improve efficacy of immunotherapies. This might provide us with a toolbox to fine-tune and improve future treatment options for cancer, infectious disease, allergy, and autoimmunity. Nevertheless, caution is advised with interpreting the immunomodulatory effects, as the role of CLRs in cellular vaccines is not fully elucidated yet. Hence, the glycome universe is a relatively novel but ever more highlighted field and its exploration is a must for the future of immune therapy.
SB and NN performed the literature search, wrote the manuscript, and created all figures. TG critically read and carefully revised all versions of the manuscript providing valuable guidance and insight. RR, ST, and EJ critically read the manuscript and provided valuable additions with regard to their field of immunological expertise.
This project is embedded in the DC4Balance consortium, which was supported by Health Holland. TBHG was supported by the European Research Council, Advanced grant (670424).
The authors declare that the research was conducted in the absence of any commercial or financial relationships that could be construed as a potential conflict of interest.
We thank the members of the Experimental Immunology Department of the Amsterdam UMC for their valuable input.
1. Cummings RD. The repertoire of glycan determinants in the human glycome. Mol Biosyst. (2009) 5:1087–104. doi: 10.1039/b907931a
2. Nairn AV, Moremen KW. Handbook of Glycomics. London: Academic Press (2009). p. 95–136. doi: 10.1016/B978-0-12-373600-0.00005-6
3. Nairn AV, York WS, Harris K, Hall EM, Pierce JM, Moremen KW. Regulation of glycan structures in animal tissues: transcript profiling of glycan-related genes. J Biol Chem. (2008) 283:17298–313. doi: 10.1074/jbc.M801964200
4. Colley KJ, Varki A, Kinoshita T. Chapter 4: cellular organization of glycosylation. In: Varki A, Cummings RD, Esko JD, Freeze HH, Stanley P, Bertozzi CR, Hart GW, Etzler ME, editors. Essentials of Glycobiology. Cold Spring Harbor, NY: Cold Spring Harbor Laboratory Press (2015).
5. Pereira MS, Alves I, Vicente M, Campar A, Silva MC, Padrão NA, et al. Glycans as key checkpoints of T cell activity and function. Front Immunol. (2018) 9:1–13. doi: 10.3389/fimmu.2018.02754
6. Marth JD, Grewal PK. Mammalian glycosylation in immunity. Nat Rev Immunol. (2008) 8:874–87. doi: 10.1038/nri2417
7. Ryan SO, Cobb BA. Host glycans and antigen presentation. Microbes Infect. (2012) 14:894–903. doi: 10.1016/j.micinf.2012.04.010
8. Borsig L. Selectins in cancer immunity. Glycobiology. (2018) 28:648–55. doi: 10.1093/glycob/cwx105
9. Monzavi-Karbassi B, Jousheghany F, Kieber-Emmons T. Tumor-associated glycans and tregs in immunogenicity of an autologous cell-based vaccine. Immunol Invest. (2016) 45:746–58. doi: 10.1080/08820139.2016.1219865
10. Ninkovic T, Hanisch F-G. O-glycosylated human MUC1 repeats are processed in vitro by immunoproteasomes. J Immunol. (2014) 179:2380–8. doi: 10.4049/jimmunol.179.4.2380
11. Rabinovich GA, van Kooyk Y, Cobb BA. Glycobiology of immune responses. Ann N Y Acad Sci. (2012) 1253:1–15. doi: 10.1111/j.1749-6632.2012.06492.x
12. Hawiger D, Inaba K, Dorsett Y, Guo M, Mahnke K, Rivera M, et al. Dendritic cells induce peripheral T cell unresponsiveness under steady state conditions in vivo. J Exp Med. (2001) 194:769–79. doi: 10.1084/jem.194.6.769
13. Li F, Ravetch JV. Inhibitory Fcγ receptor engagement drives adjuvant and anti-tumor activities of agonistic CD40 antibodies. Science. (2011) 333:1030–4. doi: 10.1126/science.1206954
14. Li T, DiLillo DJ, Bournazos S, Giddens JP, Ravetch JV, Wang LX. Modulating IgG effector function by Fc glycan engineering. Proc Natl Acad Sci USA. (2017) 114:3485–90. doi: 10.1073/pnas.1702173114
15. Hafkenscheid L, Moel E, Smolik I, Tanner S, Meng X, Jansen BC, et al. N-linked glycans in the variable domain of IgG anti-citrullinated protein antibodies predict the development of rheumatoid arthritis. Arthr Rheumatol. (2019) 71:1–8. doi: 10.1002/art.40920
16. Scherer HU, Huizinga TWJ, Krönke G, Schett G, Toes REM. The B cell response to citrullinated antigens in the development of rheumatoid arthritis. Nat Rev Rheumatol. (2018) 14:157–69. doi: 10.1038/nrrheum.2018.10
17. Tang D, Kang R, Coyne CB, Zeh HJ, Lotze MT. PAMPs and DAMPs: signal 0s that spur autophagy and immunity. Immunol Rev. (2012) 249:158–75. doi: 10.1111/j.1600-065X.2012.01146.x
18. Iborra S, Sancho D. Signalling versatility following self and non-self sensing by myeloid C-type lectin receptors. Immunobiology. (2015) 220:175–84. doi: 10.1016/j.imbio.2014.09.013
19. Johnson LL, Jones MB, Ryan SO, Cobb BA. The regulatory power of glycans and their binding partners in immunity. Trends Immunol. (2013) 34:290–8. doi: 10.1016/j.it.2013.01.006
20. del Fresno C, Iborra S, Saz-Leal P, Martínez-López M, Sancho D. Flexible signaling of Myeloid C-type lectin receptors in immunity and inflammation. Front Immunol. (2018) 9:1–13. doi: 10.3389/fimmu.2018.00804
21. Wolfert MA, Boons GJ. Adaptive immune activation: glycosylation does matter. Nat Chem Biol. (2013) 9:776–84. doi: 10.1038/nchembio.1403
22. Zelensky AN, Gready JE. The C-type lectin-like domain superfamily. FEBS J. (2005) 272:6179–217. doi: 10.1111/j.1742-4658.2005.05031.x
23. García-Vallejo JJ, Van Kooyk Y. Endogenous ligands for C-type lectin receptors: The true regulators of immune homeostasis. Immunol Rev. (2009) 230:22–37. doi: 10.1111/j.1600-065X.2009.00786.x
24. Osorio F, Reis e Sousa C. Myeloid C-type lectin receptors in pathogen recognition and host defense. Immunity. (2011) 34:651–64. doi: 10.1016/j.immuni.2011.05.001
25. Rabinovich GA, Croci DO. Regulatory circuits mediated by lectin-glycan interactions in autoimmunity and cancer. Immunity. (2012) 36:322–35. doi: 10.1016/j.immuni.2012.03.004
26. Sancho D, Reis e Sousa C. Signaling by myeloid C-type lectin receptors in immunity and homeostasis. Annu Rev Immunol. (2012) 30:491–529. doi: 10.1146/annurev-immunol-031210-101352
27. Gringhuis S, den Dunnen J, Litjens M, van der Vlist M, Wevers B, Bruijns S, et al. Dectin-1 directs T helper cell differentiation by controlling noncanonical NF-kappaB activation through Raf-1 and Syk. Nat Immunol. (2009) 10:203–13. doi: 10.1038/ni.1692
28. Gringhuis SI, Kaptein TM, Wevers BA, Mesman AW, Geijtenbeek TBH. Fucose-specific DC-SIGN signalling directs T helper cell type-2 responses via IKKε-and CYLD-dependent Bcl3 activation. Nat Commun. (2014) 5:1–13. doi: 10.1038/ncomms4898
29. Hoving JC, Wilson GJ, Brown GD. Signalling C-type lectin receptors, microbial recognition and immunity. Cell Microbiol. (2014) 16:185–94. doi: 10.1111/cmi.12249
30. Dambuza IM, Brown GD. C-type lectins in immunity: Recent developments. Curr Opin Immunol. (2015) 32:21–7. doi: 10.1016/j.coi.2014.12.002
31. Bauer B, Steinle A. HemITAM: a single tyrosine motif that packs a punch. Sci Signal. (2017) 10:1–10. doi: 10.1126/scisignal.aan3676
32. Fuller GLJ, Williams JAE, Tomlinson MG, Eble JA, Hanna SL, Pöhlmann S, et al. The C-type lectin receptors CLEC-2 and Dectin-1, but not DC-SIGN, signal via a novel YXXL-dependent signalling cascade. J Biol Chem. (2007) 282:12397–409. doi: 10.1074/jbc.M609558200
33. Robinson MJ, Sancho D, Slack EC, LeibundGut-Landmann S, Sousa CR. Myeloid C-type lectins in innate immunity. Nat Immunol. (2006) 7:1258–65. doi: 10.1038/ni1417
34. Rogers NC, Slack EC, Edwards AD, Nolte MA, Schulz O, Schweighoffer E, et al. Syk-dependent cytokine induction by dectin-1 reveals a novel pattern recognition pathway for C type lectins. Immunity. (2005) 22:507–17. doi: 10.1016/j.immuni.2005.03.004
35. Brown G. Dectin-1: a signalling non-TLR pattern-recognition receptor. Nat Rev Immunol. (2006) 6:33–43. doi: 10.1038/nri1745
36. Goodridge HS, Reyes CN, Becker CA, Katsumoto TR, Ma J, Wolf AJ, et al. Activation of the innate immune receptor Dectin-1 upon formation of a “phagocytic synapse.” Nature. (2011) 472:471–5. doi: 10.1038/nature10071
37. LeibundGut-Landmann S, Gross O, Robinson MJ, Osorio F, Slack EC, Tsoni SV, et al. Syk- and CARD9-dependent coupling of innate immunity to the induction of T helper cells that produce interleukin 17. Nat Immunol. (2007) 8:630–8. doi: 10.1038/ni1460
38. del Fresno C, Saz-Leal P, Enamorado M, Wculek SK, Martínez-Cano S, Blanco-Menéndez N, et al. DNGR-1 in dendritic cells limits tissue damage by dampening neutrophil recruitment. Science. (2018) 362:351–6. doi: 10.1126/science.aan8423
39. Kanazawa N, Okazaki T, Nishimura H, Tashiro K, Inaba K, Miyachi Y. DCIR acts as an inhibitory receptor depending on its immunoreceptor tyrosine-based inhibitory motif. J Invest Dermatol. (2002) 118:261–6. doi: 10.1046/j.0022-202x.2001.01633.x
40. Švajger U, Anderluh M, Jeras M, Obermajer N. C-type lectin DC-SIGN: An adhesion, signalling and antigen-uptake molecule that guides dendritic cells in immunity. Cell Signal. (2010) 22:1397–405. doi: 10.1016/j.cellsig.2010.03.018
41. Lowell A. The expanding roles of ITAM adaptors FcRgamma and DAP12 in myeloid Cells. Immunol Rev. (2011) 232:42–58. doi: 10.1111/j.1600-065X.2009.00841.x
42. Yamasaki S, Ishikawa E, Kohno M, Saito T. The quantity and duration of FcRγ signals determine mast cell degranulation and survival. Blood. (2004) 103:3093–101. doi: 10.1182/blood-2003-08-2944
43. Hernanz-Falcón P, Joffre O, Williams DL, Reis e Sousa C. Internalization of Dectin-1 terminates induction of inflammatory responses. Eur J Immunol. (2009) 39:507–13. doi: 10.1002/eji.200838687
44. Rosas M, Liddiard K, Kimberg M, Faro-Trindade I, McDonald JU, Williams DL, et al. The induction of inflammation by Dectin-1 in vivo is dependent on myeloid cell programming and the progression of phagocytosis. J Immunol. (2014) 181:3549–57. doi: 10.4049/jimmunol.181.5.3549
45. O'Keeffe M, Mok WH, Radford KJ. Human dendritic cell subsets and function in health and disease. Cell Mol Life Sci. (2015) 72:4309–25. doi: 10.1007/s00018-015-2005-0
46. Carenza C, Calcaterra F, Oriolo F, Di Vito C, Ubezio M, Della Porta MG, et al. Costimulatory molecules and immune checkpoints are differentially expressed on different subsets of dendritic cells. Front Immunol. (2019) 10:1325. doi: 10.3389/fimmu.2019.01325
47. Watchmaker PB, Lahl K, Lee M, Baumjohann D, Morton J, Kim SJ, et al. Comparative transcriptional and functional profiling defines conserved programs of intestinal DC differentiation in humans and mice. Nat Immunol. (2014) 15:98–108. doi: 10.1038/ni.2768
48. Döring Y, Manthey HD, Drechsler M, Lievens D, Megens RTA, Soehnlein O, et al. Auto-antigenic protein-DNA complexes stimulate plasmacytoid dendritic cells to promote atherosclerosis. Circulation. (2012) 125:1673–83. doi: 10.1161/CIRCULATIONAHA.111.046755
49. Lande R, Chamilos G, Ganguly D, Demaria O, Frasca L, Durr S, et al. Cationic antimicrobial peptides in psoriatic skin cooperate to break innate tolerance to self-DNA. Eur J Immunol. (2015) 45:203–13. doi: 10.1002/eji.201344277
50. Rönnblom L, Pascual V. The innate immune system in SLE: type I interferons and dendritic cells. Lupus. (2008) 17:394–9. doi: 10.1177/0961203308090020
51. Goubier A, Dubois B, Gheit H, Joubert G, Villard-Truc F, Asselin-Paturel C, et al. Plasmacytoid dendritic cells mediate oral tolerance. Immunity. (2008) 29:464–75. doi: 10.1016/j.immuni.2008.06.017
52. Lambrecht BN. Lung dendritic cells: targets for therapy in allergic disease. Curr Mol Med. (2008) 8:393–400. doi: 10.2174/156652408785160916
53. Rogers NM, Isenberg JS, Thomson AW. Plasmacytoid dendritic cells: No longer an enigma and now key to transplant tolerance? Am J Transpl. (2013) 13:1125–33. doi: 10.1111/ajt.12229
54. Lee J, Breton G, Oliveira TYK, Zhou YJ, Aljoufi A, Puhr S, et al. Restricted dendritic cell and monocyte progenitors in human cord blood and bone marrow. J Exp Med. (2015) 212:385–99. doi: 10.1084/jem.20141442
55. Jongbloed SL, Lebre MC, Fraser AR, Gracie JA, Sturrock RD, Tak PP, et al. Enumeration and phenotypical analysis of distinct dendritic cell subsets in psoriatic arthritis and rheumatoid arthritis. Arthritis Res Ther. (2006) 8:R15. doi: 10.1186/ar1864
56. Segura E, Touzot M, Bohineust A, Cappuccio A, Chiocchia G, Hosmalin A, et al. Human inflammatory dendritic cells induce Th17 cell differentiation. Immunity. (2013) 38:336–48. doi: 10.1016/j.immuni.2012.10.018
57. Cao T, Ueno H, Glaser C, Fay JW, Palucka AK, Banchereau J. Both Langerhans cells and interstitial DC cross-present melanoma antigens and efficiently activate antigen-specific CTL. Eur J Immunol. (2007) 37:2657–67. doi: 10.1002/eji.200636499
58. Cunningham AL, Abendroth A, Jones C, Nasr N, Turville S. Viruses and langerhans cells. Immunol Cell Biol. (2010) 88:416–23. doi: 10.1038/icb.2010.42
59. Klechevsky E. Human dendritic cells - stars in the skin. Eur J Immunol. (2013) 43:3147–55. doi: 10.1002/eji.201343790
60. Stoitzner P, Tripp CH, Eberhart A, Price KM, Jung JY, Bursch L, et al. Langerhans cells cross-present antigen derived from skin. Proc Natl Acad Sci USA. (2006) 103:7783–8. doi: 10.1073/pnas.0509307103
61. Klechevsky E, Morita R, Liu M, Cao Y, Thompson-Snipes L, Briere F, et al. Functional specializations of human epidermal Langerhans cells and CD14+ dermal dendritic Cells. Immunity. (2008) 29:497–510. doi: 10.1016/j.immuni.2008.07.013
62. Rodriguez-Plata MT, Urrutia A, Cardinaud S, Buzón MJ, Izquierdo-Useros N, Prado JG, et al. HIV-1 capture and antigen presentation by dendritic cells: enhanced viral capture does not correlate with better T cell activation. J Immunol. (2012) 188:6036–45. doi: 10.4049/jimmunol.1200267
63. van den Berg LM, Cardinaud S, van der Aar AMG, Sprokholt JK, de Jong MAWP, Zijlstra-Willems EM, et al. Langerhans cell–dendritic cell cross-talk via langerin and hyaluronic acid mediates antigen transfer and cross-presentation of HIV-1. J Immunol. (2015) 195:1763–73. doi: 10.4049/jimmunol.1402356
64. Van Der Vlist M, De Witte L, De Vries RD, Litjens M, De Jong MAWP, Fluitsma D, et al. Human Langerhans cells capture measles virus through langerin and present viral antigens to CD4 + T cells but are incapable of cross-presentation. Eur J Immunol. (2011) 41:2619–31. doi: 10.1002/eji.201041305
65. Zaba LC, Krueger JG, Lowes MA. Resident and “inflammatory” dendritic cells in human skin. J Invest Dermatol. (2009) 129:302–8. doi: 10.1038/jid.2008.225
66. McGovern N, Schlitzer A, Gunawan M, Jardine L, Shin A, Poyner E, et al. Human dermal CD14+ cells are a transient population of monocyte-derived macrophages. Immunity. (2014) 41:465–77. doi: 10.1016/j.immuni.2014.08.006
67. Hammerstrom AE, Cauley DH, Atkinson BJ, Sharma P. Cancer immunotherapy: sipuleucel-T and beyond. Pharmacotherapy. (2011) 8:813–28. doi: 10.1592/phco.31.8.813
68. Kantoff PW, Higano CS, Shore ND, Berger ER, Small EJ, Penson DF, et al. Sipuleucel-T immunotherapy for castration-resistant prostate cancer. N Engl J Med. (2010) 363:411–22. doi: 10.1056/NEJMoa1001294
69. Bell GM, Anderson AE, Diboll J, Reece R, Eltherington O, Harry RA, et al. Autologous tolerogenic dendritic cells for rheumatoid and inflammatory arthritis. Ann Rheum Dis. (2017) 76:227–34. doi: 10.1136/annrheumdis-2015-208456
70. Benham H, Nel HJ, Law SC, Mehdi AM, Street S, Ramnoruth N, et al. Citrullinated peptide dendritic cell immunotherapy in HLA-risk genotype-positive rheumatoid arthritis patients. Sci Transl Med. (2015) 7:290ra87. doi: 10.1126/scitranslmed.aaa9301
71. Mahnke K, Guo M, Lee S, Sepulveda H, Swain SL, Nussenzweig M, et al. The dendritic cell receptor for endocytosis, DEC-205, can recycle and enhance antigen presentation via major histocompatibility complex class II-positive lysosomal compartments. J Cell Biol. (2000) 151:673–84. doi: 10.1083/jcb.151.3.673
72. Flacher V, Tripp CH, Stoitzner P, Haid B, Koch F, Park CG, et al. Epidermal Langerhans cells rapidly capture and present antigens from C-type lectin-targeting antibodies deposited in the dermis. J Invest Dermatol. (2010) 130:755–62. doi: 10.1038/jid.2009.343
73. Ni L, Gayet I, Zurawski S, Duluc D, Flamar A-L, Li X-H, et al. Concomitant activation and antigen uptake via human Dectin-1 results in potent antigen-specific CD8 + T cell responses. J Immunol. (2010) 185:3504–13. doi: 10.4049/jimmunol.1000999
74. Pereira CF, Torensma R, Hebeda K, Kretz-Rommel A, Faas SJ, Figdor CG, et al. In vivo targeting of DC-SIGN-positive antigen-presenting cells in a nonhuman primate model. J Immunother. (2007) 30:705–14. doi: 10.1097/CJI.0b013e31812e6256
75. Tsuji T, Matsuzaki J, Kelly MP, Ramakrishna V, Vitale L, He L-Z, et al. Antibody-targeted NY-ESO-1 to mannose receptor or DEC-205 in vitro elicits dual human CD8+ and CD4+ T cell responses with broad antigen specificity. J Immunol. (2011) 186:1218–27. doi: 10.4049/jimmunol.1000808
76. Lehmann C, Heger L, Heidkamp G, Baranska A, Lühr J, Hoffmann A, et al. Direct delivery of antigens to dendritic cells via antibodies specific for endocytic receptors as a promising strategy for future therapies. Vaccines. (2016) 4:8. doi: 10.3390/vaccines4020008
77. Ducancel F, Muller BH. Molecular engineering of antibodies for therapeutic and diagnostic purposes. MAbs. (2012) 4:445–57. doi: 10.4161/mabs.20776
78. Demangel C, Zhou J, Choo ABH, Shoebridge G, Halliday GM, Britton WJ. Single chain antibody fragments for the selective targeting of antigens to dendritic cells. Mol Immunol. (2005) 42:979–85. doi: 10.1016/j.molimm.2004.09.034
79. Ejaz A, Ammann CG, Werner R, Huber G, Oberhauser V, Hörl S, et al. Targeting viral antigens to CD11c on dendritic cells induces retrovirus-specific T cell responses. PLoS ONE. (2012) 7:1–14. doi: 10.1371/journal.pone.0045102
80. Arnold JN, Saldova R, Abd Hamid UM, Rudd PM. Evaluation of the serum N-linked glycome for the diagnosis of cancer and chronic inflammation. Proteomics. (2008) 8:3284–93. doi: 10.1002/pmic.200800163
81. Einarsdottir HK, Selman MHJ, Kapur R, Scherjon S, Koeleman CAM, Deelder AM, et al. Comparison of the Fc glycosylation of fetal and maternal immunoglobulin G. Glycoconj J. (2013) 30:147–57. doi: 10.1007/s10719-012-9381-6
82. Shields RL, Lai J, Keck R, O'Connell LY, Hong K, Gloria Meng Y, et al. Lack of fucose on human IgG1 N-linked oligosaccharide improves binding to human FcγRIII and antibody-dependent cellular toxicity. J Biol Chem. (2002) 277:26733–40. doi: 10.1074/jbc.M202069200
83. Scallon BJ, Tam SH, McCarthy SG, Cai AN, Raju TS. Higher levels of sialylated Fc glycans in immunoglobulin G molecules can adversely impact functionality. Mol Immunol. (2007) 44:1524–34. doi: 10.1016/j.molimm.2006.09.005
84. Dong H, Stanek O, Rudilla Salvador F, Länger U, Morillon E, Ung C, et al. Induction of protective immunity against Mycobacterium tuberculosis by delivery of ESX antigens into airway dendritic cells. Mucosal Immunol. (2013) 6:522–34. doi: 10.1038/mi.2012.92
85. Tacken PJ, Ginter W, Berod L, Cruz LJ, Joosten B, Sparwasser T, et al. Targeting DC-SIGN via its neck region leads to prolonged antigen residence in early endosomes, delayed lysosomal degradation and cross-presentation. Blood. (2011) 118:4111–9. doi: 10.1182/blood-2011-04-346957
86. Tullett KM, Leal Rojas IM, Minoda Y, Tan PS, Zhang J-G, Smith C, et al. Targeting CLEC9A delivers antigen to human CD141+ DC for CD4+ and CD8+ T cell recognition. JCI Insight. (2016) 1:1–12. doi: 10.1172/jci.insight.87102
87. Stoitzner P, Schaffenrath S, Tripp CH, Reider D, Komenda K, Del Frari B, et al. Human skin dendritic cells can be targeted in situ by intradermal injection of antibodies against lectin receptors. Exp Dermatol. (2014) 23:909–15. doi: 10.1111/exd.12573
88. Kato M, McDonald KJ, Khan S, Ross IL, Vuckovic S, Chen K, et al. Expression of human DEC-205 (CD205) multilectin receptor on leukocytes. Int Immunol. (2006) 18:857–69. doi: 10.1093/intimm/dxl022
89. Klauber TCB, Laursen JM, Zucker D, Brix S, Jensen SS, Andresen TL. Delivery of TLR7 agonist to monocytes and dendritic cells by DCIR targeted liposomes induces robust production of anti-cancer cytokines. Acta Biomater. (2017) 53:367–77. doi: 10.1016/j.actbio.2017.01.072
90. van Dinther D, Stolk DA, van de Ven R, van Kooyk Y, de Gruijl TD, and den Haan JMM. Targeting C-type lectin receptors: a high-carbohydrate diet for dendritic cells to improve cancer vaccines. J Leukoc Biol. (2017) 102:1017–34. doi: 10.1189/jlb.5MR0217-059RR
91. Singh SK, Streng-Ouwehand I, Litjens M, Kalay H, Burgdorf S, Saeland E, et al. Design of neo-glycoconjugates that target the mannose receptor and enhance TLR-independent cross-presentation and Th1 polarization. Eur J Immunol. (2011) 41:916–25. doi: 10.1002/eji.201040762
92. Unger WWJ, Van Beelen AJ, Bruijns SC, Joshi M, Fehres CM, Van Bloois L, et al. Glycan-modified liposomes boost CD4 + and CD8 + T-cell responses by targeting DC-SIGN on dendritic cells. J Control Release. (2012) 160:88–95. doi: 10.1016/j.jconrel.2012.02.007
93. Wamhoff E.-C., Schulze J, Bellmann L, Rentzsch M, Bachem G, Fuchsberger FF, et al. A specific, glycomimetic langerin ligand for human Langerhans cell targeting. ACS Central Sci. (2019) 5:808–20. doi: 10.1021/acscentsci.9b00093
94. Benito-Villalvilla C, Soria I, Subiza JL, Palomares O. Novel vaccines targeting dendritic cells by coupling allergoids to mannan. Aller J Int. (2018) 27:256–62. doi: 10.1007/s40629-018-0069-8
95. Sirvent S, Soria I, Cirauqui C, Cases B, Manzano AI, Diez-Rivero CM, et al. Novel vaccines targeting dendritic cells by coupling allergoids to nonoxidized mannan enhance allergen uptake and induce functional regulatory T cells through programmed death ligand 1. J Allergy Clin Immunol. (2016) 138:558–567.e11. doi: 10.1016/j.jaci.2016.02.029
96. White KL, Rades T, Furneaux RH, Tyler PC, Hook S. Mannosylated liposomes as antigen delivery vehicles for targeting to dendritic cells. J Pharm Pharmacol. (2006) 58:729–37. doi: 10.1211/jpp.58.6.0003
97. Fehres CM, Kalay H, Bruijns SCM, Musaafir SAM, Ambrosini M, Van Bloois L, et al. Cross-presentation through langerin and DC-SIGN targeting requires different formulations of glycan-modified antigens. J Control Release. (2015) 203:67–76. doi: 10.1016/j.jconrel.2015.01.040
98. Benne N, van Duijn J, Kuiper J, Jiskoot W, Slütter B. Orchestrating immune responses: How size, shape and rigidity affect the immunogenicity of particulate vaccines. J Control Release. (2016) 234:124–34. doi: 10.1016/j.jconrel.2016.05.033
99. Frenz T, Grabski E, Durán V, Hozsa C, Stepczynska A, Furch M, et al. Antigen presenting cell-selective drug delivery by glycan-decorated nanocarriers. European J Pharm Biopharm. (2015) 95:13–7. doi: 10.1016/j.ejpb.2015.02.008
100. Broecker F, Götze S, Hudon J, Rathwell DCK, Pereira CL, Stallforth P, et al. Synthesis, liposomal formulation, and immunological evaluation of a minimalistic carbohydrate-α-GalCer vaccine candidate. J Med Chem. (2018) 61:4918–27. doi: 10.1021/acs.jmedchem.8b00312
102. Rodrigues E, Macauley MS. Hypersialylation in cancer: Modulation of inflammation and therapeutic opportunities. Cancers. (2018) 10:1–19. doi: 10.3390/cancers10060207
103. Cai G, Wang K, Qu N, Qiu P, Vlahakis JZ, Szarek WA, et al. Antitumor effect of a liposome-encapsulated β1,4-galactosyltransferase inhibitor. Int J Pharm. (2018) 552:388–93. doi: 10.1016/j.ijpharm.2018.10.010
104. Arigita C, Bevaart L, Everse LA, Koning GA, Hennink WE, Crommelin DJA, et al. Liposomal meningococcal B vaccination: role of dendritic cell targeting in the development of a protective immune response. Infect Immun. (2003) 71:5210–8. doi: 10.1128/IAI.71.9.5210-5218.2003
105. Matsuoka Y, Kawauchi Y, Kawauchi K, Takiyama A, Kojima S, Kuroda Y, et al. In vitro activation and maturation of human mononuclear phagocytes by stimulation with liposomes coated with a neoglycolipid containing α1–3, α1–6-mannotriose. Glycoconj J. (2019) 36:185–97. doi: 10.1007/s10719-019-09870-6
106. Boks MA, Ambrosini M, Bruijns SC, Kalay H, Van Bloois L, Storm G, et al. MPLA incorporation into DC-targeting glycoliposomes favours anti-tumour T cell responses. J Control Release. (2015) 216:37–46. doi: 10.1016/j.jconrel.2015.06.033
107. Geller A, Shrestha R, Yan J. Yeast-derived β-glucan in cancer: novel uses of a traditional therapeutic. Int J Mol Sci. (2019) 20:3618. doi: 10.3390/ijms20153618
108. Wu TC, Xu K, Banchereau R, Marches F, Yu CI, Martinek J, et al. Reprogramming tumor-infiltrating dendritic cells for CD103+CD8+ Mucosal T-cell differentiation and breast cancer rejection. Cancer Immunol Res. (2014) 2:487–500. doi: 10.1158/2326-6066.CIR-13-0217
109. Malamud M, Carasi P, Assandri MH, Freire T, Lepenies B, De Los Ángeles Serradell M. S-Layer glycoprotein from Lactobacillus kefiri exerts its immunostimulatory activity through glycan recognition by Mincle. Front Immunol. (2019) 10:1–11. doi: 10.3389/fimmu.2019.01422
110. Bunthitsakda W, Leelayuwapan H, Paha J, Kangwanrangsan N, Chawengkirttikul R, Ponpuak M, et al. Controlled rapid synthesis and in vivo immunomodulatory effects of LM α(1,6)mannan with an amine linker. Carbohydr Polym. (2018) 195:420–31. doi: 10.1016/j.carbpol.2018.04.045
111. Phillips BE, Garciafigueroa Y, Engman C, Trucco M, and Giannoukakis N. Tolerogenic dendritic cells and T-regulatory cells at the clinical trials crossroad for the treatment of autoimmune disease; emphasis on type 1 diabetes therapy. Front Immunol. (2019) 10:148. doi: 10.3389/fimmu.2019.00148
112. Marciani DJ. Effects of immunomodulators on the response induced by vaccines against autoimmune diseases. Autoimmunity. (2017) 50:393–402. doi: 10.1080/08916934.2017.1373766
113. Keijzer C, Van der Zee R, Van Eden W, Broere F. Treg inducing adjuvants for therapeutic vaccination against chronic inflammatory diseases. Front Immunol. (2013) 4:1–10. doi: 10.3389/fimmu.2013.00245
114. Wu Z, Wang L, Tang Y, Sun X. Parasite-derived proteins for the treatment of allergies and autoimmune diseases. Front Microbiol. (2017) 8:1–13. doi: 10.3389/fmicb.2017.02164
115. Prasanphanich NS, Mickum ML, Heimburg-Molinaro J, Cummings RD. Glycoconjugates in host-helminth interactions. Front Immunol. (2013) 4:1–22. doi: 10.3389/fimmu.2013.00240
116. Van Der Aar AMG, Sibiryak DS, Bakdash G, Van Capel TMM, Van Der Kleij HPM, Opstelten DJE, et al. Vitamin D3 targets epidermal and dermal dendritic cells for induction of distinct regulatory T cells. J Allergy Clin Immunol. (2011) 127:1532–40.e7. doi: 10.1016/j.jaci.2011.01.068
117. Heijink IH, Van Oosterhout AJM. Strategies for targeting T-cells in allergic diseases and asthma. Pharmacol Therap. (2006) 112:489–500. doi: 10.1016/j.pharmthera.2006.05.005
118. Schneider LP, Schoonderwoerd AJ, Moutaftsi M, Howard RF, Reed SG, de Jong EC, et al. Intradermally administered TLR4 agonist GLA-SE enhances the capacity of human skin DCs to activate T cells and promotes emigration of Langerhans cells. Vaccine. (2012) 30:4216–24. doi: 10.1016/j.vaccine.2012.04.051
119. Perdicchio M, Ilarregui JM, Verstege MI, Cornelissen LAM, Schetters STT, Engels S, et al. Sialic acid-modified antigens impose tolerance via inhibition of T-cell proliferation and de novo induction of regulatory T cells. Proc Natl Acad Sci USA. (2016) 113:3329–34. doi: 10.1073/pnas.1507706113
120. Wilson DS, Hirosue S, Raczy MM, Bonilla-Ramirez L, Jeanbart L, Wang R, et al. Antigens reversibly conjugated to a polymeric glyco-adjuvant induce protective humoral and cellular immunity. Nat Mater. (2019) 18:175–85. doi: 10.1038/s41563-018-0256-5
121. Massoud AH, Yona M, Xue D, Chouiali F, Alturaihi H, Ablona A, et al. Dendritic cell immunoreceptor: a novel receptor for intravenous immunoglobulin mediates induction of regulatory T cells. J Allergy Clin Immunol. (2014) 133:853–63.e5. doi: 10.1016/j.jaci.2013.09.029
122. Parameswarappa SG, Reppe K, Geissner A, Ménová P, Govindan S, Calow ADJ, et al. A semi-synthetic oligosaccharide conjugate vaccine candidate confers protection against Streptococcus pneumoniae serotype 3 infection. Cell Chem Biol. (2016) 23:1407–16. doi: 10.1016/j.chembiol.2016.09.016
Keywords: protein glycosylation in immunology, C-type lectin receptors, in vivo targeting dendritic cells, immunotherapy, immunomodulation, glycan vaccines
Citation: Busold S, Nagy NA, Tas SW, van Ree R, de Jong EC and Geijtenbeek TBH (2020) Various Tastes of Sugar: The Potential of Glycosylation in Targeting and Modulating Human Immunity via C-Type Lectin Receptors. Front. Immunol. 11:134. doi: 10.3389/fimmu.2020.00134
Received: 11 November 2019; Accepted: 20 January 2020;
Published: 07 February 2020.
Edited by:
John Kenneth Hoober, Susavion Biosciences, Inc., United StatesReviewed by:
Maureen Taylor, Imperial College London, United KingdomCopyright © 2020 Busold, Nagy, Tas, van Ree, de Jong and Geijtenbeek. This is an open-access article distributed under the terms of the Creative Commons Attribution License (CC BY). The use, distribution or reproduction in other forums is permitted, provided the original author(s) and the copyright owner(s) are credited and that the original publication in this journal is cited, in accordance with accepted academic practice. No use, distribution or reproduction is permitted which does not comply with these terms.
*Correspondence: Teunis B. H. Geijtenbeek, dC5iLmdlaWp0ZW5iZWVrQGFtc3RlcmRhbXVtYy5ubA==
†These authors have contributed equally to this work
Disclaimer: All claims expressed in this article are solely those of the authors and do not necessarily represent those of their affiliated organizations, or those of the publisher, the editors and the reviewers. Any product that may be evaluated in this article or claim that may be made by its manufacturer is not guaranteed or endorsed by the publisher.
Research integrity at Frontiers
Learn more about the work of our research integrity team to safeguard the quality of each article we publish.