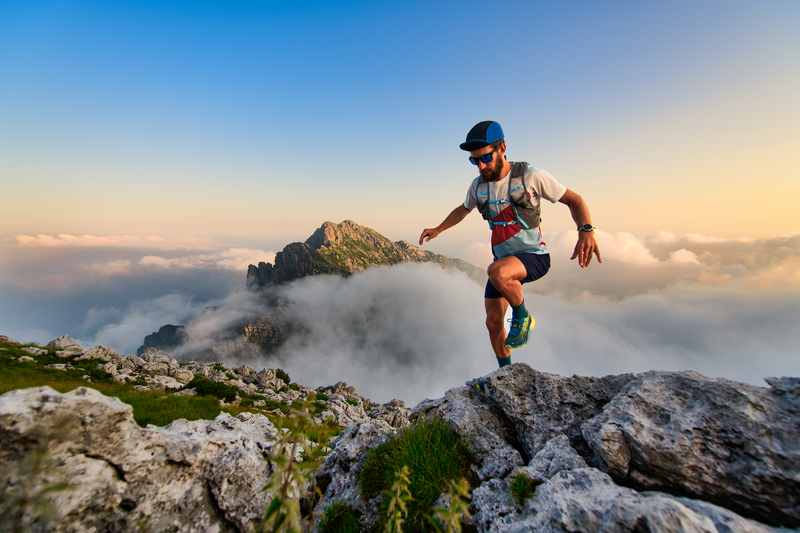
95% of researchers rate our articles as excellent or good
Learn more about the work of our research integrity team to safeguard the quality of each article we publish.
Find out more
ORIGINAL RESEARCH article
Front. Immunol. , 06 February 2020
Sec. Comparative Immunology
Volume 11 - 2020 | https://doi.org/10.3389/fimmu.2020.00100
This article is part of the Research Topic Recent Advances in Veterinary Immunology Concepts and Methodology View all 10 articles
Pigs with severe combined immunodeficiency (SCID) are an emerging biomedical animal model. Swine are anatomically and physiologically more similar to humans than mice, making them an invaluable tool for preclinical regenerative medicine and cancer research. One essential step in further developing this model is the immunological humanization of SCID pigs. In this work we have generated T− B− NK− SCID pigs through site directed CRISPR/Cas9 mutagenesis of IL2RG within a naturally occurring DCLRE1C (ARTEMIS)−/− genetic background. We confirmed ART−/− IL2RG−/Y pigs lacked T, B, and NK cells in both peripheral blood and lymphoid tissues. Additionally, we successfully performed a bone marrow transplant on one ART−/− IL2RG−/Y male SCID pig with bone marrow from a complete swine leukocyte antigen (SLA) matched donor without conditioning to reconstitute porcine T and NK cells. Next, we performed in utero injections of cultured human CD34+ selected cord blood cells into the fetal ART−/− IL2RG−/Y SCID pigs. At birth, human CD45+ CD3ε+ cells were detected in cord and peripheral blood of in utero injected SCID piglets. Human leukocytes were also detected within the bone marrow, spleen, liver, thymus, and mesenteric lymph nodes of these animals. Taken together, we describe critical steps forwards the development of an immunologically humanized SCID pig model.
Animals with severe combined immunodeficiency (SCID) are invaluable to biomedical researchers because they are permissive to engraftment of human cells, allowing one to study developmental processes within an in vivo environment. In 2012, we discovered the first naturally occurring SCID pigs (1, 2), caused by mutations within the ARTEMIS gene, resulting in a T− B− NK+ SCID phenotype (3, 4). Since then, pigs with mutations in RAG1 (5, 6), RAG2 (7, 8), IL2RG (9–11), and RAG2/IL2RG (12) have also been generated through different mutagenic approaches. Within the past few years, such SCID pigs are now being utilized by cancer (13), disease model (12), and stem cell therapy (7) researchers. Biocontainment facilities (14), isolators (12), and Cesarean section (15) techniques have allowed survival of animals, enabling longer term studies. An important step in further developing the SCID pig model is to immunologically humanize these animals through the introduction of human CD34+ hematopoietic stem cells. Similarities between human and porcine immune genes (16) suggest that human immune development would be supported in vivo within the pig (17). Development of such a model could provide researchers with a larger humanized animal for use in cancer (13, 17), HIV, and vaccine development research.
The first SCID mouse, described in 1983 (18), is capable of being humanized by either injection of human peripheral blood leukocytes (19) or by implantation of human fetal liver, thymus, and/or lymph node tissue (20). Reconstitution of human immune cell subsets in SCID mice often requires addition of human cytokine genes, humanization of resident mouse immune genes, or administration of developmental cytokines to the mice (21–24). However, limitations of mouse models include differences in size, drug metabolism, and disease pathology compared to humans (25, 26). Thus, one major goal of the SCID pig community is to create an immunologically humanized SCID pig, which would provide a valuable and unique tool for preclinical research, in a more anatomically and/or physiologically relevant animal model.
The most commonly used strain for humanization is the non-obese diabetic (NOD)-SCID- IL2RG (NSG) mouse (27). The NOD mouse background contains polymorphisms within the SIRPA (signal regulatory protein alpha) gene, allowing it to bind to human CD47 to transduce a “don't eat me” signal in mouse myeloid cells to inhibit phagocytosis (28–30). We have demonstrated that porcine SIRPA also binds to human CD47 to inhibit phagocytosis of human cells (31), indicating pigs may be permissive to human xenografts, similar to NOD mice. In addition to the SIRPA polymorphism, NSG mice also have a T− B− NK− cellular phenotype. This cellular phenotype can be generated through mutagenesis of genes required for VDJ recombination (i.e., ARTEMIS or RAG1/2), in addition to IL2RG. Previous reports show that mouse NK cells negatively impact human cell engraftment in SCID mice (27). NK cells in ART−/− SCID pigs are functional in vitro (4), and thus we anticipated swine NK cells could also negatively impact human cell engraftment. To deplete NK cells in our current ART−/− SCID pig model, we mutagenized IL2RG in an ART−/− mutant cell line. The resulting pigs are similar to NSG mice in cellular phenotype and are expected to be similar in SIRPA/CD47 dependent phagocytic tolerance (31).
Here we describe the generation of ART−/− IL2RG−/Y SCID pigs derived by site-directed CRISPR/Cas9 mutagenesis of IL2RG in an ART−/− fetal fibroblast cell line. Modified ART−/− IL2RG−/Y embryos, derived from somatic cell nuclear transfer, were implanted in gilts via surgical embryo transfer. Piglets were born at full term and confirmed to have the expected T− B− NK− cellular phenotype based on flow cytometry and immunohistochemical (IHC) analysis of blood and lymphoid organs. We next determined if these double mutant pigs could be humanized via the introduction of human CD34+ cord blood stem cells. Gestational day 41 ART−/− IL2RG−/Y fetuses were injected with human CD34+ cells within the intraperitoneal space by ultrasound guidance and piglets were delivered via Cesarean section at gestational day 119. We probed for human myeloid, lymphoid, and erythroid cells in peripheral blood and lymphoid organs in piglets for up to 7 days of age. We found evidence of human CD45+ cell engraftment in several tissues in the ART−/− IL2RG−/− pigs. Specifically, we detected CD3ε+ T and Pax5+ B lymphocytes in blood and lymphoid organs. Taken together, we successfully established the first steps toward the generation of a humanized SCID pig model.
Our study was designed to develop a T− B− NK− SCID pig model by generating ART−/− IL2RG−/Y pigs by CRISPR/Cas9 site directed mutagenesis of our existing ART−/− pig line, which was discovered in 2012 (1, 2, 32). We aimed to generate these pigs as a large animal biomedical model for human cell and tissue xenotransplantation. Once we successfully created the ART−/− IL2RG−/Y fibroblast cell line, we performed a total of eight embryo transfer surgeries to generate piglets. Of these transfers, five females became pregnant, and a total of three litters were born; one of which we performed in utero injections of human CD34+ cells. Once piglets were born, we confirmed their T− B− NK− phenotype. We performed a pig to pig bone marrow transplant on one ART−/− IL2RG−/Y boar, which would allow us to eventually collect semen for future breeding and use of this genetic line. We performed in utero injections of human cord blood selected CD34+ cells on ART−/− IL2RG−/Y fetuses from one pregnant female. Three piglets were born from this litter, with two piglets showing evidence of human immune cell engraftment. The low number of animals in this study are a result of small litter sizes of cloned piglets, as well as low pregnancy rates of embryo transfer procedures.
All animal protocols were approved by Iowa State University's Institutional Animal Care and Use Committee. All animals were utilized in accordance with the Animal Welfare Act and the Guide for the Care and Use of Laboratory Animals. All human sample collection protocols were approved by Iowa State University's Institutional Review Board.
Our population of SCID pigs has two natural mutations in two separate ARTEMIS alleles, termed ART12 and ART16. The ART12 allele contains a nonsense mutation in exon 10, while the ART16 allele contains a splice site mutation in intron 8(3). Frozen semen from a bone marrow transplanted (BMT) rescued ART12/16 (33) boar was utilized to artificially inseminate two ART+/− carrier sows. The sows were sacrificed at day 35 of gestation and fetuses were collected in a sterile manner to obtain fetal fibroblasts (pFF), as described previously (34). Briefly, minced tissue from each fetus was digested in 20 mL of digestion media (Dulbecco-modified Eagle medium [DMEM] containing L-glutamine and 1 g/L D-glucose [Cellgro] supplemented with 200 units/mL collagenase and 25 Kunitz units/mL DNaseI) for 5 h at 38.5°C. After digestion, pFF cells were washed in sterile PBS and cultured in DMEM supplemented with 15% fetal bovine serum (FBS) and 40 μg/mL gentamicin (Sigma Aldrich). Upon reaching 100% confluence, the pFF cells were trypsinized, frozen in FBS with 10% dimethyl sulfoxide (DMSO) and stored long-term in liquid nitrogen. Simultaneously, cellular DNA was sent for swine leukocyte antigen (SLA) typing, as described in Powell et al. (33). SRY (sex determining region Y) and ARTEMIS primers (Supplemental Table 1) were utilized to identify pFF sex and ARTEMIS genotype (3).
Guide RNAs targeting exon 5 of IL2RG were designed utilizing software available from Zhang Lab (https://zlab.bio/guide-design-resources). The sequence of the designed sgRNA was:
5′-GGCCACTATCTATTCTCTGAAGG-3′; the bold font identifies the PAM site. The sgRNA oligos were annealed and ligated into the human codon-optimized SpCas9 expression plasmid (pX330; Addgene plasmid # 42230), as described previously (35). We only transfected male ART12/12 cell lines (herein referred to as ART−/−).
To identify putative off-target sequences for the CRISPR/Cas9 mutagenesis used in ART−/− IL2RG−/Y piglets, bioinformatics tools (http://www.rgenome.net/cas-offinder/) were used. Ten potential off-target sites were identified and primers for the off-target positions were designed. Genomic DNA samples obtained from ear notches of ART−/− IL2RG−/Y pigs were used as templates in PCR amplification of potential off-target regions. Primers and gene information for this purpose are in Supplemental Table 2. DNA sequencing results revealed no mutations had occurred in any of the potential off-target positions.
Male ART−/− pFFs were used for cell transfection, as described in Whitworth et al. (36). Briefly, pFFs were cultured in 75 cm2 flasks to reach 90% confluency, trypsinized, resuspended at a concentration of 1.0 × 106 cells/mL in Electroporation Buffer medium (25% Opti-MEM [Gibco, 319850070] and 75% cytosalts [120 mM KCl, 0.15 mM CaCl2, 10 mM K2HPO4; pH 7.6, 5 mM MgCl2]), and prepared for transfection. A mixture of 1 μg sgRNA ligated vector and 200 μL of cell suspension in electroporation buffer was then transferred into 2 mm gap cuvettes (Fisher Sci, 9104-6050) and exposed to three- 1 ms square-wave pulses at 250 V, using the BTX Electro Cell Manipulator (Harvard Apparatus). Transfected cells were then diluted, 80–200 cells were plated in 100 mm culture dishes to obtain distinct clonal cell colonies and maintained at 38.5°C in 5% CO2. After 10–12 days in culture, cell colonies were delineated using cloning cylinders and picked for clonal colony propagation and DNA sequencing. Primers (Supplemental Table 1) flanking the IL2RG exon 5 target region were utilized to test clonal colonies and PCR products thus obtained were purified by ExoSAP-IT PCR product Cleanup kit (Affymetrix Inc, Thermo Fisher Scientific). Mutant PCR products were cloned into PCR2.1 vectors (Life Technologies) and transformed into E.coli DH5-α maximum competent cells (Life Technologies). Ten colonies were chosen and DNA from these samples were sent to the Iowa State University DNA Facility for sequencing. Sequences were aligned by Bio-Edit software (Ibis Biosciences, Carlsbad, CA, USA) for comparison with wild-type alleles, to identify cell lines with the appropriate mutations in IL2RG on the ART−/− background.
Purchased pig oocytes (DeSoto Biosciences, Inc.) or those derived from aspirating ovaries collected from a local abattoir were utilized for in vitro maturation (IVM), as previously described (37, 38). Briefly, oocytes were matured in vitro with maturation medium (TCM-199 with 2.9 mM HEPES, 5 μg/mL insulin, 10 ng/mL epidermal growth factor, 0.5 μg/mL follicle stimulating hormone, 0.5 μg/mL luteinizing hormone, 0.91 mM pyruvate, 0.5 mM cysteine, 10% porcine follicular fluid, and 25 ng/mL gentamicin) (Sigma Aldrich), and transferred into fresh medium after 22 h. Following IVM, cumulus-oocyte-complexes (COC) were vortexed for 3 min in 0.1% hyaluronidase in TCM199 with HEPES to obtain denuded oocytes. Metaphase II (MII) oocytes, identified by the presence of an extruded polar body, were placed in manipulation medium (TCM199 with HEPES supplemented with 7 μg/mL cytochalasin B) and used thereafter for somatic cell nuclear transfer (SCNT). The extruded polar body, along with a portion of the adjacent cytoplasm, presumably containing the M II plate, were removed, and a donor nucleus of the appropriate ART−/− IL2RG−/Y genotype was placed in the perivitelline space by using a thin glass capillary. The reconstructed embryos were then placed in a fusion medium (0.3 M mannitol, 0.1 mM CaCl2, 0.1 mM MgCl2, and 0.5 mM HEPES) (Sigma Aldrich) and exposed to two DC pulses (1-s interval) at 1.2 kV/cm for 30 μs using a BTX Electro Cell Manipulator (Harvard Apparatus). After fusion, these embryos were activated in embryo activation medium (10 μg/mL cytochalasin B) for 4 h. After chemical activation, cloned zygotes were treated with 500 mM Scriptaid for 12–14 h and then cultured in porcine zygote medium 3 (PZM-3) (recipe per 100 mL: 0.6312 g NaCl, 0.2106 g NaHCO3, 0.0746 g KCl, 0.0048 g KH2PO4, 0.0022 g Na-pyruvate, 0.0146 g L-glutamate, 0.0546 g hypotaurine, 0.0617 g Ca-lactate, 0.001 g gentamicin, 2.0 mL BME essential amino acid, 1.0 mL MEM non-essential amino acid, and 0.3 g BSA). until embryo transfer. Embryos produced over 2 days were then surgically transferred into the ampullary-isthmic junction of the oviduct of the surrogate on day 1–2 post estrus. Pregnancies were confirmed by ultrasound ~30 days following embryo transfer.
At gestational day 119, pregnant gilts underwent Cesarean sections. We chose gestational day 119 instead of 114 (normal gestation) because piglets derived from somatic cell nuclear transfer typically requiring longer gestational period. Initial anesthesia was induced with either a lumbar epidural of propofol (0.83–1.66 mg/kg) (Zoetis) or intravenous injection of Ketamine (1–2 mg/kg) (Akorn) and Xylazine (1–2 mg/kg) (Akorn), and anesthesia was maintained on oxygen and isoflurane (Phoenix). An abdominal incision was made to expose and remove the uterus. After removal, the uterus was immediately rinsed in chlorohexidine and then surgically opened to remove the piglets. All piglets had their cords clamped before being immediately placed into sterile polystyrene boxes and delivered into biocontainment facilities (14). All piglets were fed ~250 mL of pasteurized porcine colostrum within the first 24 h of life. A total of eight Art−/− IL2RG−/Y SCID pigs were created and assessed within this study (animal IDs: 6401, 6402, 6403, 6701, 6702, 6901, 6902, and 6903). Piglets derived from the gilt that underwent laparotomy procedures for human stem cell injection (see below) did not receive colostrum. After birth, DNA was isolated from ear notch tissues and subjected to genotyping for ART and IL2RG status using primers and protocols described in Supplemental Table 1.
Whole blood or cord blood from newborn ART−/− IL2RG−/Y piglets was collected into an EDTA blood collection tube. Whole blood was stained for porcine CD3ε, CD8α, and CD172α to assess the presence of porcine T and NK cells. Cells were additionally stained for porcine CD21 to assess the presence of B cells. Blood from the ART−/− IL2RG−/Y BMT was also stained for CD79α to assess B cell reconstitution. Additional information about the antibodies used can be found in Table 1.
Blood was collected from the bone marrow transplanted boar approximately once a month after the BMT and subjected to either a complete blood count (CBC) at Iowa State University's Veterinary Diagnostic lab or by flow cytometry analysis using the above listed antibodies. All samples were run on a custom BD LSR II (BD Biosciences) and data were analyzed using Flowjo (Tree Star).
A complete SLA-matched female sow of ~4 years of age was euthanized and used as a bone marrow donor for one ART−/− IL2RG−/Y piglet. Briefly, sternum and ribs collected from the animal were dipped in 70% ethanol after collection. A sterilized Dremel tool was used to make holes halfway through the bone to expose bone marrow. Sterilized Spratt Brun bone curettes were used to scrape marrow from the bone. HBSS (without phenol red) was used to flush the bone marrow to collect cells; any other loosened marrow was also placed in HBSS. After marrow isolation, the suspension was washed in HBSS. The cells were resuspended in ACK (ammonium chloride potassium) lysing solution (Lonza) for 10 min at room temperature. The suspension was washed in HBSS and filtered through a 70 μm cell strainer. A total of 2.27 × 108 million unfractionated cells were isolated and resuspended in ~3 mL of HBSS for infusion.
To infuse bone marrow-derived cells into the ART−/− IL2RG−/Y recipient, the 5-day old SCID piglet was anesthetized with and maintained on isoflurane gas during the procedure. A catheter was placed in an ear vein and the cell suspension slowly infused. After infusion, personnel monitored the piglet until fully recovered.
Lymphoid organs were collected into 3.7% formaldehyde in 1X PBS for 24 hours. Tissues were then moved to 70% ethanol until processing. IHC staining for T and B lymphocyte markers (for ART−/− IL2RG−/Y immune characterization) was performed in paraffin-embedded tissue thin sections at the Kansas State Veterinary Diagnostic Laboratory (KSVDL). Briefly, deparaffinized slide-mounted thin sections were pre-treated for 5 min with a peroxide block, followed by incubation with primary antibody. A mouse monoclonal anti-CD3ε (clone LN10, Leica Biosystems) was used to stain for pig T cells, while a mouse monoclonal anti-CD79α (clone HM57, Abcam) was used to stain for B cells. Primary antibodies were incubated with PowerVision Poly-HRP anti-mouse IgG at room temperature for 25 min with DAB chromagen, and then counterstained with hematoxylin.
Lymphoid tissues from SCID pigs engrafted with human cells were treated similarly as above. Staining was performed at Michigan State University's Department of Pathobiology and Diagnostic Investigation. Tissues were stained for anti-CD3ε (Dako #A0452) and anti-Pax5 (Ventana clone 24) to assess for the presence of human T and B cells, respectively. Briefly, CD3ε staining was performed by antigen retrieval with standard ER1 retrieval for 20 min, and tissues were analyzed on a BondMax (Leica Biosystems) for the detection of DAB chromagen. For Pax5 staining, antigen retrieval was performed with standard CC1 retrieval for 64 min, and tissues were analyzed on a Discovery Ultra AP (Roche) for ultrared detection.
Human cord blood was collected at the Mary Greeley Medical Center in Ames, Iowa, into 50 mL conical tubes containing 8 mL of anticoagulant citrate dextrose solution (38 mM citric acid, 85.25 mM sodium citrate, 136 mM dextrose). Mononuclear cells (MNCs) were isolated from cord blood by diluting blood 1:2 in HBSS and then layering over Ficoll-Paque (GE Healthcare). Buffy coats were collected and washed in HBSS. Prior to stem cell isolation, MNCs were resuspended in an isolation HBSS (iHBSS) consisting of 0.5% FBS and 2 mM EDTA in HBSS.
To isolate human CD34+ cells, we used a CD34 MicroBead kit from Miltenyi Biotech. Briefly, MNCs were incubated in iHBSS with CD34 microbeads and FcR blocking reagent for 30 min at 4°C on a rocker. After the incubation period, cells were washed in iHBSS and then passed through a LS column in a Miltenyi magnet (Miltenyi Biotech) to capture human CD34+ cells. The column was then removed from the magnet and cells were flushed, washed once more in HBSS, and then frozen in 10% DMSO and 90% FBS at −80°C until use.
Human CD34+ cells were thawed by diluting into complete RPMI media (10% FBS, 2 mM glutamine, 50 μg/mL gentamicin, and 10 mM HEPES) (Gibco). Cells were cultured in Miltenyi StemMACS media containing Thrombopoietin (TPO), Stem Cell Factor (SCF), and Flt3-Ligand (FLT-3L), at starting concentrations of 38-42 × 103 cells/mL. Cells were left in culture for 7 days and expanded 184.5-fold. Cells were prepared for injection by washing three times in phosphate buffered saline and resuspended at a concentration of 26.6 × 106 cells/mL. A total of 150 μL of cell suspension with either 2 or 4 × 106 cells were administered to the fetuses in 0.9% saline.
Laparotomy procedures were performed as previously described in Boettcher et al. (39). Briefly, the gilt was started on 15 mg of Matrix (Merck Animal Health) orally 1 day before surgery and maintained on Matrix until gestational day 118. Immediately prior to sedation, the gilt was given 0.01 mg/kg Glycopyrrolate (West-Ward Pharmaceuticals) by intramuscular injection and then anesthetized with 2 mg/kg Xylazine (Akorn) and 5 mg/kg Telazol (Zoetis) by intramuscular injection. The gilt was then placed in dorsal recumbency, intubated, and started on isoflurane (Phoenix, St. Joseph, MO) (3–5%) and oxygen (2.5 L/min). Lactated Ringer's solution (Hospira) was given in an ear catheter at a constant rate infusion within 10 min of the first incision. The abdominal area was scrubbed with chlorohexidine and the surgical field was covered with sterile drapes and Ioban drapes.
A ventral midline incision was made from the caudal most nipple extending to the caudal aspect of the umbilicus through the linea alba into the peritoneal cavity. The left uterine horn was exposed and visualized with a ZONARE ultrasound with an L14-5sp intraoperative linear array transducer (10 MHz). Two live and one nonviable fetus were visualized in the left horn; one was injected with 4 million cultured human stem cells in a volume of 0.15 mL within the intraperitoneal space. The left horn was placed back into the abdominal cavity and the right horn was exposed and visualized, and three fetuses were observed. One fetus was injected with 2 million and another fetus with 4 million human stem cells within the intraperitoneal space. In total, three out of the five viable fetuses were injected. The abdominal cavity was lavaged with 500 mL of Lactated Ringer's solution and sutured closed. The gilt then received 0.18 mg/kg Buprenophine- Sustained Release (ZooPharm) subcutaneously, 5 mg/kg Ceftiofur Crystalline Free Acid (Excede) (Zoetis), and 0.3 mg/kg of meloxicam (Norbrook) and monitored by personnel until recovered from anesthesia. The gilt recovered from the laparotomy surgery with no issues and underwent a Cesarean section at day 119 of gestation, as described above.
At the time of Cesarean section, we observed two live piglets within the left horn and one live piglet in the right horn of the uterus. During the laparotomy, we observed two viable fetuses in the left horn, of which one was injected, and their relative position was recorded. After Cesarean delivery, by position in the uterus we were able to identify the two developed piglets in the left horn as injected (6901) and non-injected (6902). The right horn originally had three viable fetuses but only one piglet survived to term. After flow cytometric analysis, we confirmed that the one piglet on the right horn (6903) had been injected based on presence of human cells.
To collect lymphoid MNCs, tissue was collected and placed into Hanks Balanced Salt Solution (HBSS) with 10 μg/mL gentamicin. Tissues were minced in a digestion solution of HBSS with 300 mg/mL of collagenase, 3% FBS, and 2 mM HEPES (referred to as d-HBSS). The tissue incubated for 1 h at 37°C with vortexing every 15 min, and then strained over a 70 μm cell strainer. Cells were washed once and counted with a BD counting kit, and then were stained as described below.
Peripheral or cord blood was collected from piglets into EDTA blood containers (BD Biosciences). Whole blood or MNC from tissues were stained with antibodies against human and pig cell subset markers (Table 1). Each staining step was incubated for 15 min at 4°C and washed with 1X PBS with 0.1% sodium azide. Red blood cells were lysed with ammonium chloride lysing solution. Cells were fixed in 2% formaldehyde in 1X PBS and data were acquired on a FACs Canto II flow cytometer (BD Biosciences) and analyzed using FlowJo (Treestar).
We previously described the discovery of naturally occurring ART−/− SCID pigs (3), which we have been able to raise and breed (14, 33) for research purposes. We started with this genetic background for IL2RG site-directed mutagenesis. One goal of producing ART−/− IL2RG−/Y SCID pigs is to generate a breeding colony, such that IL2RG knockout piglets can be derived through natural birth rather than cloning procedures. In our breeding protocol, this would require bone marrow transplantation of a SCID boar, so he could be raised to sexual maturity and bred to ART−/+ carrier females. Mutagenizing our existing ART−/− line would facilitate producing SCID pigs with matching SLA to carrier animals within our colony. Therefore, we decided to utilize cloned ART−/− fibroblasts for IL2RG mutagenesis for somatic cell nuclear transfer (SCNT) to generate pigs with these desired genetics. Figure 1 shows a schematic for the process of generating ART−/− IL2RG−/Y piglets.
Figure 1. Use of CRISPR/Cas9 system to generate ART−/− IL2RG−/Y SCID pigs. (A) Semen from an ART−/− boar was used to inseminate ART−/+ carrier sows. Two pregnant sows were euthanized at 35 days of gestation and fetal fibroblasts were collected. Collected ART−/− fetal fibroblasts were transfected with PX330 plasmid with Cas9 and sgRNA against IL2RG target site, generating ART−/− IL2RG−/Y mutant fetal fibroblasts (pFF). Once the mutant pFFs lines were established, somatic cell nuclear transfer was performed, and embryos were transferred into surrogate gilts. Piglets born from these litters were delivered via Cesarean section at gestational day 119 into biocontainment facilities. (B) Sequence of IL2RG from wildtype and mutated line. Blue sequence is intron 4, and green sequence is exon 5. Mutation spans through intron 4 and exon 5. Underlined sequence indicates the designed sRNA with the letters in red showing the protospacer adjacent motif (PAM) sequence (NGG).
ART−/− pFFs for gene editing were derived from an ART−/− male by ART−/+ female mating (see Materials and Methods for information on ARTEMIS genotypes) (Figure 1A). Gestational day 35 fibroblasts were collected and underwent SLA typing and were genotyped for ART status. Genotyping revealed that two male and two female cell lines were ART−/− mutants, while the other six male and three female cell lines were ART−/+ carriers (Supplemental Table 3A).
The first sentence should be split into two sentences in the figure legend:
To mutagenize IL2RG, a single guide RNA (sgRNA) was designed to target exon 5 of IL2RG (Figure 1B.) We selected a male ART−/− cell line (7707-FB1) that had a complete SLA-match (haplotype 26.6/68.19a) to a male carrier fibroblast line (7709-FB6) to transfect with a vector to express sgRNA and Cas9 protein (Supplemental Tables 3A,B). After transfection, a total of 202 individual clonal colonies from the 7707-FB1 cell line were screened using PCR and Sanger sequencing. Five (2.5%) clonal colonies were confirmed to be IL2RG−/Y (hemizygous) (Supplemental Table 4), with one cell line (7707-FB1-U23) carrying a 120 bp deletion in intron 4 and exon 5 of the IL2RG locus, which was expected to cause a frameshift leading to an premature stop codon (Figure 1B). A total of 920 IL2RG−/Y ART−/− (7707-FB1-U23) and 512 non-modified (7709-FB6) SCNT derived embryos were transferred surgically into seven recipient gilts. Both cell lines shared the same SLA haplotype of 26.6/68.19a. Among those transferred, four gilts were confirmed pregnant and two carried their piglets to full term and produced five live male piglets via Cesarean section that were reared in biocontainment facilities (14) (Supplemental Table 5). All five piglets were confirmed to have the 120 bp loss in IL2RG and the established mutation in exon 10 of ARTEMIS (3) (ART12 allele information found in Materials and Methods). None of the live born piglets were derived from non-modified, carrier embryos (7709-FB6) that would have been used as a bone marrow donor.
Once ART−/− IL2RG−/Y piglets were delivered, blood was collected and analyzed by flow cytometry to confirm the expected T− B− NK− cellular phenotype of these animals. A total of three ART−/− IL2RG−/Y pigs were born (6401, 6402, 6403) in this litter and were analyzed with a wildtype and ART−/− pigs. We assessed forward and side scatter (FSC/SSC) and stained cells for CD172α, CD16, CD3ε, and CD8α to assess for myeloid, T, and B cells (Figure 2). FSC/SSC plots show that the lymphocyte population is nearly absent in ART−/− IL2RG−/Y SCID pigs compared to ART−/− and wild type pigs. We measured CD172α and CD16 staining on monocytes as a staining positive control and show CD172α+ CD16+ monocytes. Compared to wildtype, T cells (CD3ε+) and NK cells (CD3ε− CD8α+) were absent in ART−/− IL2RG−/Y piglets. We also stained for pCD21 in a second litter (6701 and 6702) and confirmed there were no B cells in the circulation of ART−/− IL2RG−/Y pigs (Supplemental Figure 1). Lack of B cells in these pigs is consistent with our previous findings in ART−/− SCID pigs, the genetic background used to generate ART−/− IL2RG−/Y pigs (1, 32). Furthermore, ART−/− IL2RG−/Y pigs had atrophic and smaller thymus, spleen, and lymphoid tissue within the intestines compared to wildtype pigs (Supplemental Figure 2).
Figure 2. ART−/− IL2RG−/Y SCID pigs lack T and NK cells in peripheral blood. Flow cytometric analysis of major leukocyte populations from peripheral blood of wildtype, ART−/−, and ART−/− IL2RG−/Y pigs. Percentages for granulocytes (G, blue), monocytes (M, green), and lymphocytes (L, orange) are shown for each type of pig. The monocyte population was assessed for CD172α and CD16 expression. The lymphocyte population was assessed for CD3ε and CD8 for T cells (CD3ε+, CD8α−/+) and NK cells (CD3ε− CD8α+).
We then confirmed that T and B cells were absent from lymphoid tissues. Thymus, spleen, and Peyer's patches were collected from a wildtype and two ART−/− IL2RG−/Y pigs (6401 and 6402) and stained for CD3ε and CD79α to assess for the presence of T and B cells, respectively (Figure 3). Thymic tissue was assessed from only one ART−/− IL2RG−/Y pig. The ART−/− IL2RG−/Y pigs lacked normal T and B cells in all lymphoid tissues assessed.
Figure 3. ART−/− IL2RG−/Y SCID pigs lack T and B cells in lymphoid organs. Thymus, spleen, and Peyer's Patches were collected and assessed for (A) T cells (CD3ε) and (B) B cells in a wildtype and two ART−/− IL2RG−/Y SCID pigs.
An additional goal for generation of ART−/− IL2RG−/Y SCID pigs was to establish a male breeding population to maintain this line. Carrier ART−/+ females bred with an ART−/− IL2RG−/Y SCID boar would generate litters with a mix of males and females with different ART and IL2RG genotypes for future studies and breeding. The original intent of performing embryo transfers with non-modified carrier embryos was for them to provide a source of bone marrow for the ART−/− IL2RG−/Y pigs. In our two full-term pregnancies, one gilt was transferred with carrier and ART−/− IL2RG−/Y embryos (7707-FB1-U23 and 7709-FB6), while the other was transferred with only ART−/− IL2RG−/Y embryos. From both litters, only ART−/− IL2RG−/Y piglets were born, thus requiring an alternative source of bone marrow for a BMT.
We therefore performed a BMT on one ART−/− IL2RG−/Y male piglet using complete SLA matched (26.6/68.19a) bone marrow from a 4-year-old sow, which was raised on a conventional farm. A total of 2.27 × 108 million unfractionated bone marrow cells from the ribs and sternum were collected and administered to one ART−/− IL2RG−/Y SCID pig. The piglet was not conditioned prior to the BMT, based on our previous success with porcine T and B cell reconstitution without conditioning in ART−/− SCID pigs (33).
Peripheral blood was collected approximately once a month after the BMT and analyzed by either flow cytometric analysis (FACS) or a complete blood count (CBC) (Figure 4). FACS analysis using antibodies against CD3ε, CD172α, CD16, CD79α, and CD21 revealed circulating T (CD3ε+) and NK cells (CD3ε− CD172α− CD16+) (4), but very few B cells (CD79α+ CD21+/−) Of note, B cell reconstitution is variable in human SCIDs with mutations in ARTEMIS post BMT (40–42), which may be a function of conditioning regimens. CBC analysis showed that white blood cells and lymphocytes increased monthly after the transplant, to near normal levels by 4 months post BMT. Importantly, the ART−/− IL2RG−/Y boar post BMT has been maintained in the biocontainment bubble since birth and is sexually mature and healthy as of 1.5 years of age.
Figure 4. T and NK cells are reconstituted after pig to pig bone marrow transplantation. (A) One ART−/− IL2RG−/Y SCID piglet underwent a bone marrow transplant at 5 days of age. At five months post transplantation, mononuclear cells were stained for CD3ε, CD172α, CD16, CD79α, and CD21 to assess for donor T (CD3ε+), NK (CD3ε− CD16+ CD172α−), and B cell (CD79α+ CD21+/−) development. (B) Complete blood counts were also performed routinely, and white blood cell counts increased upon every collection point. CBC data shown for up to 4 months post BMT.
Once we confirmed the cellular phenotype of ART−/− IL2RG−/Y pigs, we investigated whether these pigs were capable of engrafting human CD34+ hematopoietic stem cells. We had previously attempted intravenous or intraosseous injection of human CD34+ cells into single mutant ART−/− piglets (within 1 week of age), with various cell doses and busulfan conditioning (Supplemental Table 6). We did not detect any evidence of engraftment in peripheral blood or lymphoid organs 15 weeks post-transplant. As an alternative approach, in utero injection of human stem cells into the intraperitoneal space of ART−/− IL2RG−/Y SCID pig fetuses was performed.
During mid-gestation (30–45 days), the fetal liver is the major site for hematopoiesis for many species (43–46), including swine (47). Previous reports show that injection of human CD34+ cells into the intraperitoneal space of fetal piglets and sheep leads to differentiation and engraftment of human immune cells (48–51). Engraftment of human cells into pig and sheep fetuses is facilitated by the fact that the cellular environment is immunologically privileged early in gestation. However, such injections have not been reported in SCID pigs. Approaches to inject fetal piglets require laparotomy procedures to expose the uterus to visualize fetuses via ultrasound imaging. Thus, laparotomy surgery with ultrasound guidance was used to inject human CD34+ cells into the intraperitoneal space of ART−/− IL2RG−/Y fetuses (39) (Figure 5A). Positively selected human CD34+ cells isolated from cord blood were cultured with thrombopoietin (TPO), FLT-3L, and stem cell factor (SCF) for the 7 days prior to injection to enhance expansion for the appropriate cell dose delivery. After culturing, cells were either CD45+CD34+ (23-28%) or CD45+ CD34− (71–76%) (Supplemental Figure 3).
Figure 5. Human leukocytes in ART−/− IL2RG−/Y SCID pig cord blood. (A) Somatic cell nuclear transfer derived ART−/− IL2RG−/Y embryos were surgically transferred into a surrogate gilt. At gestational day 41, the pregnant gilt underwent a laparotomy procedure to expose the uterus and ultrasound guidance was utilized to inject human CD34+ stem cells into the intraperitoneal space of fetal piglets. After surgery, piglets were delivered via Cesarean section at gestational day 119 into biocontainment facilities. (B) Blood was collected from a human (peripheral blood) and three neonatal (6901, 6902, and 6903) SCID pigs (cord blood) and stained for human and pig CD45, as well as human CD47. All cells in circulation of cord blood were gated from SCID pigs. Pigs 6901 and 6903 both had human cells in cord blood, as shown by presence of hCD45+ and hCD47+ cells.
To create fetuses for human cell injections, one surrogate gilt was transplanted with 320 ART−/− IL2RG−/Y mutant embryos (Supplemental Table 7). Pregnancy was confirmed at 28 days of gestation and laparotomy surgery for in utero injection was performed on gestational day 41. We injected half of the viable fetuses (n = 5 total; 3 injected) with 2–4 × 106 cultured human CD34+ stem cells. The position in the uterine horn was recorded for all fetuses. The dam fully recovered from the laparotomy surgery and three piglets (6901, 6902, 6903) were delivered via Cesarean section at gestational day 119. The uterine positions at Cesarean section were noted and allowed identification of injected (6901, 6903) and un-injected (6902) piglets (see details in Methods). Cord blood was immediately collected from the three piglets to assess for the presence of human immune cells. We have tested and determined panels of anti-human antibodies that are not cross reactive to swine leukocytes (Supplemental Figure 4). Whole cord blood was stained for pig and human CD45, as well as for human CD47. The two CD34+ cell injected pigs (6901 and 6903) were found to have human CD45+, as well as human CD47+ cells in cord blood (Figure 5B).
We next assessed if human cells were circulating in peripheral blood. One piglet (6901) was euthanized on day 0 due to a severe cleft palate. Peripheral blood was collected from 6901 at day 0 and at 1 day of age for 6902 and 6903. These blood samples were stained for human T cells (hCD3ε, hCD4α, and hCD8α) (Figure 6A), B cells (hCD20) (Figure 6B), and myeloid cells (hCD33) (Figure 6C). Red blood cells were also stained for human CD47 (Figure 6D). Nearly all human CD45+ cells circulating in 6901 and 6903 consisted of human CD3+ cells that were positive for hCD4α or hCD8α (Figure 6A).
Figure 6. Human CD3ε+ cells are the primary cell type in peripheral blood of ART−/− IL2RG−/Y SCID pigs. Peripheral blood was collected from 6901 (day 0), 6902, and 6903 (Day 1) and stained for various human immune cell markers. Whole human blood was stained as a control to show gating strategy. All cells in circulation were gated from SCID pigs, while only lymphocytes and monocytes were gated from the human sample for the analysis of T, B, and myeloid cells. RBC were also stained separately for human CD47. (A) Presence of human T cells were assessed by staining for human CD45, CD3ε, CD4α, and CD8α. Cells that were CD3ε+ (left) were then assessed for CD4α and CD8α expression (right). Human T cells were present in both 6901 and 6903. Single positive CD4α+ and CD8α+ cells were also present. (B) Human B cells were assessed by staining for hCD45 and hCD20. B cells were not present in any pigs. (C) Human myeloid cells were assessed by staining for hCD45 and hCD33. Myeloid cells were not present in any pigs. (D) RBCs were stained for human CD47 expression to determine if human erythroid lineage differentiated in SCID pigs. No RBCs from SCID pigs expressed hCD47.
Since we observed human cells in peripheral blood, we next evaluated whether human immune cells were present within lymphoid organs. Interestingly, during necropsy, we observed grossly visible mesenteric lymph nodes in 6901 and 6903, but not in 6902. Additionally, all three pigs had some remnant, immature thymic tissue present over the heart (Supplemental Figure 5), which was collected for analysis. Of the tissues collected, cells were isolated from bone marrow, liver, spleen, and thymic tissue for flow cytometric analysis. Whole cell suspensions from bone marrow and thymus were stained, while mononuclear cells were stained from liver and spleen. Human CD45+ cells were found in all four tissues assessed in 6901 and 6903, which both had human CD45+ cells in circulation (Figure 7). At least half of the isolated thymic tissue cells from these two animals were human cells. Animal 6902 did not have any human CD45+ cells in any lymphoid organs.
Figure 7. Human leukocyte engraftment in bone marrow, liver, spleen, and thymic tissue of in utero injected ART−/− IL2RG−/Y SCID pigs. (A) Lymphoid organs from 0-day old (6901) or 7-day old (6902 and 6903) SCID pigs were analyzed for the presence of human leukocytes by staining with human and pig CD45. Bone marrow liver, spleen, and thymic tissue from both 6901 and 6903 contained human CD45+ cells. All cells from isolated bone marrow and thymic tissue were stained, while mononuclear cells from spleen and liver were stained. (B) Human CD45+ cells in isolated cells from thymic tissue expressed human CD3ε. PBMCs from an adult human were stained as a gating control. Black histogram is gated on hCD45+ cells, while gray is hCD45− cells. Human CD4α and CD8α expression was assessed on human CD3ε+ cells within the Art−/− IL2RG−/Y thymic tissue and human PBMC.
Since a majority of the human cells we observed in these pigs were hCD3ε+, we assessed the expression of hCD4α and hCD8α within the thymic tissue cells. Early in development, T cells express both CD4α and CD8α. In animal 6901, we observed that the thymic hCD45+ hCD3ε+ cells were either hCD4α+ hCD8α− or hCD4α− hCD8α−, while in 6903 they appeared to be hCD4α− hCD8α− (Figure 7B).
In addition to flow cytometric analysis of cells within lymphoid tissues, we also analyzed tissues by IHC. Lymphoid tissues were collected and assessed for the presence of human T and B cells in the in utero injected piglets. Thymic tissue, spleen, ileum, and mesenteric lymph nodes from both 6901 and 6903 had CD3ε+ cells (Figure 8A). Spleens from both 6901 and 6903 also had punctate Pax5+ cells present, which is a marker for B cell development. A mesenteric lymph node from 6901 also had Pax5+ cells (Figure 8B). Tissues from 6902 did not stain positively for either CD3ε or Pax5, which is consistent with the lack of human CD45+ cells in blood. These histology results are consistent with the flow cytometric analyses demonstrating human leukocyte engraftment within ART−/− IL2RG−/Y SCID pigs injected with human CD34+ cells.
Figure 8. Human CD3ε+ and Pax5+ cells in lymphoid organs of in utero injected ART−/− IL2RG−/Y SCID pigs. Lymphoid tissues were collected and stained for (A) CD3ε and (B) Pax5. (A) Thymic tissues from 6901 and 6903 were robustly populated with CD3ε+ cells, and CD3ε+ cells were also present in periarteriolar sheaths within the spleen. The ileum tissue from both animals had punctate CD3ε+ cells (black arrows); CD3ε+ cells were also present within mesenteric lymph nodes (LN). Lymphoid tissues from 6902 did not contain CD3ε+ cells. (B) Spleen from both 6901 and 6903 had punctate Pax5+ cells. Only the mesenteric LN in 6901 had Pax5+ cells. No Pax5+ cells were found in tissues from 6902.
Herein we have described foundational steps toward the development of an immunologically humanized large animal SCID model. To create the model, we introduced a mutation into the IL2RG gene using the CRISPR/Cas9 system in a naturally occurring ART−/− SCID background to generate ART−/− IL2RG−/Y pigs that lacked T, B, and NK cells. We performed a pig to pig BMT procedure on one male ART−/− IL2RG−/Y pig, which led to successful reconstitution of graft T and NK cells, but very few B cells. Next, we utilized in utero injection procedures to introduce human hematopoietic stem cells into the intraperitoneal space of SCID pig fetuses. We observed that human CD3ε+ cells were present in bone marrow, spleen, liver, and thymic tissue in injected pigs after birth. Human Pax5+ cells were also present within the spleen and mesenteric lymph nodes of injected pigs. Intrahepatic injection of human CD34+ cells in newborn NOD Rag−/− IL2RG−/− mice has resulted in similar patterns of reconstitution (T and B cell development) as our ART−/− IL2RG−/Y pig (52).
One surprising finding was that we did not detect human myeloid cells, as has previously been reported in past in utero injections of human CD34+ cells in immunocompetent pig fetuses (48). In this initial humanization model, we had a pre-determined end point of 7 days to assess human cells in blood and tissues, and therefore we only probed for myeloid cells during this period. In previous studies, myeloid lineage development had been assessed at 40 days post injection (80 days of gestation) (48). It may be that human myeloid cells are transient during gestation in this fetal injection model. Further investigation is needed to improve human myeloid reconstitution in neonatal ART−/− IL2RG−/Y SCID pigs.
In the process of in vitro CD34+ cell culture with SCF, TPO, and FLT-3L, some cells may lose their stemness, which likely contributed lack of a variety of cells that differentiated (i.e., only T and B cells) within our SCID pig model. In mouse models genetic modifications have been required to attain human myeloid and NK cell engraftment, including human CSF-1, IL-15, GM-CSF, Flt-3L, IL-3, TPO, as human cells do not recognize mouse cytokines (21, 23, 53–56). An area to be investigated is how human cells respond to swine cytokines and if the swine bone marrow niche is supportive of human myeloid cells. Humanization of certain cytokine genes may be required in future humanization attempts in our pig model. In vitro culturing assays with human hematopoietic stem cells and porcine cytokines can be a first-line screen for assessing porcine cytokine cross-reactivity. Additionally, in future studies, we can assess cytokine secretion by developed human cells within the pigs.
In our model, we observed that a majority of human cells that developed were CD3ε+. We assessed the thymic tissue to better understand the development of human T cells. We interestingly did not observe CD4α+CD8α+ double positive cells within the thymus, which is an expected normal stage of T cell development. In future studies, it will be imperative that we perform deeper phenotyping of human cells that have differentiated within the pig. Previous reports by Kalscheuer et al. (57) and Ogle et al. (49) show that the swine thymus can support engraftment and differentiation of human T cells. The lack thymic development in a SCID pig fetus may negatively impact the ability of human cells to develop, which may warrant transplantation of human thymic tissue after birth.
Another potential method to increase engraftment is to condition the fetuses prior to human cell injection. Plerixafor, a drug that mobilizes stem cells out of bone marrow (58), has previously been utilized for in utero injections of human cells into sheep fetuses to improve engraftment of human cells (59). Plerixafor is an agonist for CXCL12 on stromal cells, which binds to CXCR4 on hematopoietic stem cells (HSC) (58). Administration of plerixafor mobilizes sheep HSC out of bone marrow, providing more available niches for human HSC to engraft. Goodrich et al. (59) described that administration of plerixafor along with injection of CD34+ CXCR4+ human stem cells and mesenchymal stem cells improved chimerism (in peripheral blood) 5 weeks after transplantation from 2.80 to 8.77%. Now that T− B− NK− SCID pigs and biocontainment facilities are available for extended postnatal follow-up, a similar regimen could be administered to SCID pig fetuses prior to in utero injection with human stem cells.
We show that human T cells differentiated and homed to lymphoid tissues in the ART−/− ILR2G−/Y SCID pigs. Human B cells also differentiated, but to a much lower extent. While the human cells that differentiated within the SCID pigs were not extensively characterized, the major aims of this study were to develop methodologies to humanize SCID pigs and to determine the feasibility of performing these methodologies on ART−/− ILR2G−/Y SCID fetuses. Moving forward, we expect that optimization of humanization methods would lead to increased levels of engraftment, and thus a higher number of human cells in any given tissue which could be used for different types of analyses.
Performing single cell RNAseq on isolated human cells form thymic and bone marrow tissues would be of particular interest to understand how human cells differentiate within the SCID pig primary lymphoid organs and the composition of the differentiating cell population within the graft. A comparison could be made between human cells that differentiated in NSG mice compared to ART−/− ILR2G−/Y SCID pigs. Additionally, since we have now established that human T and B cells differentiate and home to swine lymphoid organs, the functionality of these cells could be assessed either through in vivo vaccination studies or in vitro stimulation assays. Understanding the full extent of human cell differentiation and functionality will be critical as this model is developed further.
One issue in both pig to pig BMT, as well as in utero injection of human HSCs, was the failure of pig or human B cells to robustly develop. Historically, some human SCID patients that underwent BMT have also failed to develop graft derived B cells (40). In some cases, significant B cell reconstitution in human BMT can require up to 2 years (60, 61). One leading hypothesis regarding B cell development issues is due to differences in the B cell niche in the bone marrow. Single mutant IL2RG knock out pigs are capable of developing B cells, which can be detected in circulation (9, 10), however they are non-functional due to the absence of T helper cells. Mutations in ARTEMIS lead to a B cell block of differentiation at the pre-B cell phase (62). Together, an ART−/− Il2RG−/Y pig likely still has premature B cells present in the bone marrow, which would prevent further engraftment and differentiation of graft stem cells in this niche. Conditioning prior to stem cell transplantation has helped improve B cell reconstitution in some cases (63), although such conditioning procedures would be difficult prior to in utero cell transplantation. To our knowledge this is the first time a bone marrow transplantation has been performed on a double mutant SCID pig. Thus, further assessment of the bone marrow niches of ART−/− IL2RG−/Y pigs may be required to better understand conditioning regimens that may be needed for engraftment.
As the field of biomedical SCID pig research expands, new techniques will arise to optimize human cell engraftment within SCID pig models. We can draw from previous large animal in utero injection protocols (48–51, 59, 64), as well as humanization techniques performed in immunocompromised mice (65, 66). In our SCID pig model, we show that human T and B cells can develop. As we improve reconstitution of human cell subsets, the humanized SCID pig will be a critical alternative large animal model for researchers preclinical or co-clinical trials.
The raw data supporting the conclusions of this article will be made available by the authors, without undue reservation, to any qualified researcher.
The studies involving human participants were reviewed and approved by the Iowa State University Institutional Review Board. The patients/participants provided their written informed consent to participate in this study. The animal study was reviewed and approved by the Iowa State University Institutional Animal Care and Use Committee.
AB designed humanization experiments, isolated human stem cells, performed fetal injections, human immune cell flow cytometry, and wrote the draft of the manuscript. YL isolated fetal fibroblasts, performed CRISPR/Cas9 genome modification, performed somatic cell nuclear transfer and embryo transfers to generate double mutant piglets, and wrote the draft of the manuscript. BS was involved with double mutant pig generation. AA performed fetal injection laparotomy procedures on pregnant gilt. MK performed immunohistochemistry for human immune cells in lymphoid tissues. KB and CL performed flow cytometry for ART−/− IL2RG−/Y immunophenotyping and bone marrow transplantation monitoring. AC-O and JW performed immunohistochemistry for porcine immune cells in lymphoid tissues. EP performed IV injections of human stem cells in ART−/− pigs. JS collected cord blood for stem cell isolations that were used in fetal injections of ART−/− IL2RG−/Y fetuses. ES performed pig bone marrow isolation for bone marrow transplantation. C-SH performed MHC PCR analysis for bone marrow donors. JR performed bone marrow transplantation. SC genotyped fetal fibroblast cell lines for ART status. SC, ZK, and MA were involved in experimental planning for all facets of this project. JC was involved in experimental flow cytometry planning. SS performed numerous ultrasound pregnancy checks on surgically transferred gilts. GD'A and JJ assisted with fetal injection procedures. FG and EW provided human stem cells for IV injection procedures in ART−/− pigs. JD provided guidance in pig breeding to generate the litters for pFF collection and assisted in project planning discussions. JR and CT were involved in all aspects of procedures and experiments performed in this study and edited the manuscript.
This project was funded by the Office of The Director, the Office of Research Infrastructure Programs, the National Institutes of Health 1R24OD019813.
The authors declare that the research was conducted in the absence of any commercial or financial relationships that could be construed as a potential conflict of interest.
We thank Iowa State University's animal care staff for the care of SCID animals throughout the study. This manuscript has been released as a Pre-Print at BioRxiv (67).
The Supplementary Material for this article can be found online at: https://www.frontiersin.org/articles/10.3389/fimmu.2020.00100/full#supplementary-material
1. Ozuna AGC, Rowland RRR, Nietfeld JC, Kerrigan MA, Dekkers JCM, Wyatt CR. Preliminary findings of a previously unrecognized porcine primary immunodeficiency disorder. Vet Pathol. (2013) 50:144–146. doi: 10.1177/0300985812457790
2. Basel MT, Balivada S, Beck AP, Kerrigan MA, Pyle MM, Dekkers JCM, et al. Human xenografts are not rejected in a naturally occurring immunodeficient porcine line: a human tumor model in pigs. Biores Open Access. (2012) 1:63–8. doi: 10.1089/biores.2012.9902
3. Waide EH, Dekkers JCM, Ross JW, Rowland RRR, Wyatt CR, Ewen CL, et al. Not all SCID pigs are created equally: two independent mutations in the artemis gene cause SCID in Pigs. J Immunol. (2015) 195:3171–9. doi: 10.4049/jimmunol.1501132
4. Powell EJ, Cunnick JE, Knetter SM, Loving CL, Waide EH, Dekkers JCM, et al. NK cells are intrinsically functional in pigs with Severe Combined Immunodeficiency (SCID) caused by spontaneous mutations in the Artemis gene. Vet Immunol Immunopathol. (2016) 175:1–6. doi: 10.1016/j.vetimm.2016.04.008
5. Huang J, Guo X, Fan N, Song J, Zhao B, Ouyang Z, et al. RAG1/2 knockout pigs with severe combined immunodeficiency. J Immunol. (2014) 193:1496–503. doi: 10.4049/jimmunol.1400915
6. Ito T, Sendai Y, Yamazaki S, Seki-Soma M, Hirose K, Watanabe M, et al. Generation of recombination activating gene-1-deficient neonatal piglets: a model of T and B cell deficient severe combined immune deficiency. PLoS ONE. (2014) 9:e113833. doi: 10.1371/journal.pone.0113833
7. Lee K, Kwon D-N, Ezashi T, Choi Y-J, Park C, Ericsson AC, et al. Engraftment of human iPS cells and allogeneic porcine cells into pigs with inactivated RAG2 and accompanying severe combined immunodeficiency. Proc Natl Acad Sci USA. (2014) 111:7260–5. doi: 10.1073/pnas.1406376111
8. Suzuki S, Iwamoto M, Hashimoto M, Suzuki M, Nakai M, Fuchimoto D, et al. Generation and characterization of RAG2 knockout pigs as animal model for severe combined immunodeficiency. Vet Immunol Immunopathol. (2016) 178:37–49. doi: 10.1016/j.vetimm.2016.06.011
9. Suzuki S, Iwamoto M, Saito Y, Fuchimoto D, Sembon S, Suzuki M, et al. Il2rg gene-targeted severe combined immunodeficiency pigs. Cell Stem Cell. (2012) 10:753–8. doi: 10.1016/j.stem.2012.04.021
10. Kang J-T, Cho B, Ryu J, Ray C, Lee E-J, Yun Y-J, et al. Biallelic modification of IL2RG leads to severe combined immunodeficiency in pigs. Reprod Biol Endocrinol. (2016) 14:74. doi: 10.1186/s12958-016-0206-5
11. Watanabe M, Nakano K, Matsunari H, Matsuda T, Maehara M, Kanai T, et al. Generation of interleukin-2 receptor gamma gene knockout pigs from somatic cells genetically modified by zinc finger nuclease-encoding mRNA. PLoS ONE. (2013) 8:e76478. doi: 10.1371/journal.pone.0076478
12. Lei S, Ryu J, Wen K, Twitchell E, Bui T, Ramesh A, et al. Increased and prolonged human norovirus infection in RAG2/IL2RG deficient gnotobiotic pigs with severe combined immunodeficiency. Sci Rep. (2016) 6:25222. doi: 10.1038/srep25222
13. Boettcher AN, Kiupel M, Adur MK, Cocco E, Santin AD, Bellone S, et al. Human Ovarian Cancer Tumor Formation in Severe Combined Immunodeficient (SCID) Pigs. Front Oncol. (2019) 9:9. doi: 10.3389/fonc.2019.00009
14. Powell EJ, Charley S, Boettcher AN, Varley L, Brown J, Schroyen M, et al. Creating effective biocontainment facilities and maintenance protocols for raising specific pathogen-free, severe combined immunodeficient (SCID) pigs. Lab Anim. (2018) 52:402–12. doi: 10.1177/0023677217750691
15. Hara H, Shibata H, Nakano K, Abe T, Uosaki H, Ohnuki T, et al. Production and rearing of germ-free X-SCID pigs. Exp Anim. (2018) 67:139–46. doi: 10.1538/expanim.17-0095
16. Dawson HD, Loveland JE, Pascal G, Gilbert JGR, Uenishi H, Mann KM, et al. Structural and functional annotation of the porcine immunome. BMC Genomics. (2013) 14:332. doi: 10.1186/1471-2164-14-332
17. Boettcher AN, Loving CL, Cunnick JE, Tuggle CK. Development of Severe Combined Immunodeficient (SCID) pig models for translational cancer modeling: future insights on how humanized SCID pigs can improve preclinical cancer research. Front Oncol. (2018) 8:559. doi: 10.3389/fonc.2018.00559
18. Bosma GC, Custer RP, Bosma MJ. A severe combined immunodeficiency mutation in the mouse. Nature. (1983) 301:527. doi: 10.1038/301527a0
19. Mosier DE, Gulizia RJ, Baird SM, Wilson DB. Transfer of a functional human immune system to mice with severe combined immunodeficiency. Nature. (1988) 335:256. doi: 10.1038/335256a0
20. McCune JM, Namikawa R, Kaneshima H, Shultz LD, Lieberman M, Weissman IL. The SCID-hu mouse: murine model for the analysis of human hematolymphoid differentiation and function. Science. (1988) 241:1632–9. doi: 10.1126/science.2971269
21. Rongvaux A, Willinger T, Martinek J, Strowig T, Gearty SV, Teichmann LL, et al. Development and function of human innate immune cells in a humanized mouse model. Nat Biotechnol. (2014) 32:364–72. doi: 10.1038/nbt.2858
22. Brehm MA, Aryee K-E, Bruzenksi L, Greiner DL, Shultz LD, Keck J. Transgenic expression of human IL15 in NOD-scid IL2rgnull (NSG) mice enhances the development and survival of functional human NK cells. J Immunol. (2018) 200:103.20 LP-103.20.
23. Iwabuchi R, Ikeno S, Kobayashi-Ishihara M, Takeyama H, Ato M, Tsunetsugu-Yokota Y, et al. Introduction of Human Flt3-L and GM-CSF into Humanized Mice Enhances the Reconstitution and Maturation of Myeloid Dendritic Cells and the Development of Foxp3(+)CD4(+) T Cells. Front Immunol. (2018) 9:1042. doi: 10.3389/fimmu.2018.01042
24. Strowig T, Rongvaux A, Rathinam C, Takizawa H, Borsotti C, Philbrick W, et al. Transgenic expression of human signal regulatory protein alpha in Rag2-/-gamma(c)-/- mice improves engraftment of human hematopoietic cells in humanized mice. Proc Natl Acad Sci USA. (2011) 108:13218–23. doi: 10.1073/pnas.1109769108
25. Perlman RL. Mouse models of human disease: an evolutionary perspective. Evol Med Public Heal. (2016) 2016:170–6. doi: 10.1093/emph/eow014
26. Elsea SH, Lucas RE. The Mousetrap: what we can learn when the mouse model does not mimic the human Disease. ILAR J. (2002) 43:66–79. doi: 10.1093/ilar.43.2.66
27. McDermott SP, Eppert K, Lechman ER, Doedens M, Dick JE. Comparison of human cord blood engraftment between immunocompromised mouse strains. Blood. (2010) 116:193–200. doi: 10.1182/blood-2010-02-271841
28. Takenaka K, Prasolava TK, Wang JCY, Mortin-Toth SM, Khalouei S, Gan OI, et al. Polymorphism in Sirpa modulates engraftment of human hematopoietic stem cells. Nat Immunol. (2007) 8:1313–23. doi: 10.1038/ni1527
29. Yamauchi T, Takenaka K, Urata S, Shima T, Kikushige Y, Tokuyama T, et al. Polymorphic Sirpa is the genetic determinant for NOD-based mouse lines to achieve efficient human cell engraftment. Blood. (2013) 121:1316–25. doi: 10.1182/blood-2012-06-440354
30. Kwong LS, Brown MH, Barclay AN, Hatherley D. Signal-regulatory protein alpha from the NOD mouse binds human CD47 with an exceptionally high affinity– implications for engraftment of human cells. Immunology. (2014) 143:61–7. doi: 10.1111/imm.12290
31. Boettcher AN, Cunnick JE, Powell EJ, Egner TK, Charley SE, Loving CL, et al. Porcine signal regulatory protein alpha binds to human CD47 to inhibit phagocytosis: Implications for human hematopoietic stem cell transplantation into severe combined immunodeficient pigs. Xenotransplantation. (2018) 26:e12466. doi: 10.1111/xen.12466
32. Ewen CL, Cino-Ozuna AG, He H, Kerrigan MA, Dekkers JCM, Tuggle CK, et al. Analysis of blood leukocytes in a naturally occurring immunodeficiency of pigs shows the defect is localized to B and T cells. Vet Immunol Immunopathol. (2014) 162:174–9. doi: 10.1016/j.vetimm.2014.10.003
33. Powell EJ, Graham J, Ellinwood NM, Hostetter J, Yaeger M, Ho C-S, et al. T Cell Lymphoma and leukemia in severe combined immunodeficiency pigs following bone marrow transplantation: a case report. Front Immunol. (2017) 8:813. doi: 10.3389/fimmu.2017.00813
34. Ross JW, Whyte JJ, Zhao J, Samuel M, Wells KD, Prather RS. Optimization of square-wave electroporation for transfection of porcine fetal fibroblasts. Transgenic Res. (2010) 19:611–20. doi: 10.1007/s11248-009-9345-1
35. Cong L, Ran FA, Cox D, Lin S, Barretto R, Habib N, et al. Multiplex genome engineering using CRISPR/Cas systems. Science. (2013) 339:819–23. doi: 10.1126/science.1231143
36. Whitworth KM, Lee K, Benne JA, Beaton BP, Spate LD, Murphy SL, et al. Use of the CRISPR/Cas9 system to produce genetically engineered pigs from in vitro-derived oocytes and embryos. Biol Reprod. (2014) 91:78. doi: 10.1095/biolreprod.114.121723
37. Yang C-X, Du Z-Q, Wright EC, Rothschild MF, Prather RS, Ross JW. Small RNA profile of the cumulus-oocyte complex and early embryos in the pig. Biol Reprod. (2012) 87:117. doi: 10.1095/biolreprod.111.096669
38. Wright EC, Hale BJ, Yang C-X, Njoka JG, Ross JW. MicroRNA-21 and PDCD4 expression during in vitro oocyte maturation in pigs. Reprod Biol Endocrinol. (2016) 14:21. doi: 10.1186/s12958-016-0152-2
39. Boettcher AN, Ahrens AP, Charley SE, Tuggle CK. A comprehensive protocol for laparotomy in swine to facilitate ultrasound-guided injection into the fetal intraperitoneal space. Comp Med. (2019) 69:123–9. doi: 10.30802/AALAS-CM-18-000098
40. Buckley RH, Win CM, Moser BK, Parrott RE, Sajaroff E, Sarzotti-Kelsoe M. Post-transplantation B cell function in different molecular types of SCID. J Clin Immunol. (2013) 33:96–110. doi: 10.1007/s10875-012-9797-6
41. Pannicke U, Honig M, Schulze I, Rohr J, Heinz GA, Braun S, et al. The most frequent DCLRE1C (ARTEMIS) mutations are based on homologous recombination events. Hum Mutat. (2010) 31:197–207. doi: 10.1002/humu.21168
42. Schuetz C, Neven B, Dvorak CC, Leroy S, Ege MJ, Pannicke U, et al. SCID patients with ARTEMIS vs RAG deficiencies following HCT: increased risk of late toxicity in ARTEMIS-deficient SCID. Blood. (2014) 123:281–9. doi: 10.1182/blood-2013-01-476432
43. Yong KSM, Keng CT, Tan SQ, Loh E, Chang KTE, Tan TC, et al. Human CD34(lo)CD133(lo) fetal liver cells support the expansion of human CD34(hi)CD133(hi) hematopoietic stem cells. Cell Mol Immunol. (2016) 13:605–14. doi: 10.1038/cmi.2015.40
44. Battista JM, Tallmadge RL, Stokol T, Felippe MJB. Hematopoiesis in the equine fetal liver suggests immune preparedness. Immunogenetics. (2014) 66:635–49. doi: 10.1007/s00251-014-0799-9
45. Sinkora M, Butler JE, Holtmeier W, Sinkorova J. Lymphocyte development in fetal piglets: facts and surprises. Vet Immunol Immunopathol. (2005) 108:177–84. doi: 10.1016/j.vetimm.2005.08.013
46. Sinkora M, Butler JE. The ontogeny of the porcine immune system. Dev Comp Immunol. (2009) 33:273–83. doi: 10.1016/j.dci.2008.07.011
47. Sinkora M, Sinkorova J, Butler JE. B cell development and VDJ rearrangement in the fetal pig. Vet Immunol Immunopathol. (2002) 87:341–6. doi: 10.1016/S0165-2427(02)00062-4
48. Fujiki Y, Fukawa K, Kameyama K, Kudo O, Onodera M, Nakamura Y, et al. Successful multilineage engraftment of human cord blood cells in pigs after in utero transplantation. Transplantation. (2003) 75:916–22. doi: 10.1097/01.TP.0000057243.12110.7C
49. Ogle BM, Knudsen BE, Nishitai R, Ogata K, Platt JL. Toward development and production of human T cells in swine for potential use in adoptive T cell immunotherapy. Tissue Eng Part A. (2009) 15:1031–40. doi: 10.1089/ten.tea.2008.0117
50. Ogle BM, Butters KA, Plummer TB, Ring KR, Knudsen BE, Litzow MR, et al. Spontaneous fusion of cells between species yields transdifferentiation and retroviral transfer in vivo. FASEB J. (2004) 18:548–50. doi: 10.1096/fj.03-0962fje
51. McConico A, Butters K, Lien K, Knudsen B, Wu X, Platt JL, et al. In utero cell transfer between porcine littermates. Reprod Fertil Dev. (2011) 23:297–302. doi: 10.1071/RD10165
52. Dykstra C, Lee AJ, Lusty EJ, Shenouda MM, Shafai M, Vahedi F, et al. Reconstitution of immune cell in liver and lymph node of adult- and newborn-engrafted humanized mice. BMC Immunol. (2016) 17:18. doi: 10.1186/s12865-016-0157-9
53. Rathinam C, Poueymirou WT, Rojas J, Murphy AJ, Valenzuela DM, Yancopoulos GD, et al. Efficient differentiation and function of human macrophages in humanized CSF-1 mice. Blood. (2011) 118:3119–28. doi: 10.1182/blood-2010-12-326926
54. Willinger T, Rongvaux A, Takizawa H, Yancopoulos GD, Valenzuela DM, Murphy AJ, et al. Human IL-3/GM-CSF knock-in mice support human alveolar macrophage development and human immune responses in the lung. Proc Natl Acad Sci USA. (2011) 108:2390–5. doi: 10.1073/pnas.1019682108
55. Rongvaux A, Willinger T, Takizawa H, Rathinam C, Auerbach W, Murphy AJ, et al. Human thrombopoietin knockin mice efficiently support human hematopoiesis in vivo. Proc Natl Acad Sci USA. (2011) 108:2378–83. doi: 10.1073/pnas.1019524108
56. Chen Q, Khoury M, Chen J. Expression of human cytokines dramatically improves reconstitution of specific human-blood lineage cells in humanized mice. Proc Natl Acad Sci USA. (2009) 106:21783–8. doi: 10.1073/pnas.0912274106
57. Kalscheuer H, Onoe T, Dahmani A, Li H-W, Holzl M, Yamada K, et al. Xenograft tolerance and immune function of human T cells developing in pig thymus xenografts. J Immunol. (2014) 192:3442–50. doi: 10.4049/jimmunol.1302886
58. Uy GL, Rettig MP, Cashen AF. Plerixafor, a CXCR4 antagonist for the mobilization of hematopoietic stem cells. Expert Opin Biol Ther. (2008) 8:1797–804. doi: 10.1517/14712598.8.11.1797
59. Goodrich AD, Varain NM, Jeanblanc CM, Colon DM, Kim J, Zanjani ED, et al. Influence of a dual-injection regimen, plerixafor and CXCR4 on in utero hematopoietic stem cell transplantation and engraftment with use of the sheep model. Cytotherapy. (2014) 16:1280–93. doi: 10.1016/j.jcyt.2014.05.025
60. Marie-Cardine A, Divay F, Dutot I, Green A, Perdrix A, Boyer O, et al. Transitional B cells in humans: characterization and insight from B lymphocyte reconstitution after hematopoietic stem cell transplantation. Clin Immunol. (2008) 127:14–25. doi: 10.1016/j.clim.2007.11.013
61. Ogonek J, Kralj Juric M, Ghimire S, Varanasi PR, Holler E, Greinix H, et al. Immune Reconstitution after allogeneic hematopoietic stem cell transplantation. Front Immunol. (2016) 7:507. doi: 10.3389/fimmu.2016.00507
62. Noordzij JG, Verkaik NS, van der Burg M, van Veelen LR, de Bruin-Versteeg S, Wiegant W, et al. Radiosensitive SCID patients with Artemis gene mutations show a complete B-cell differentiation arrest at the pre-B-cell receptor checkpoint in bone marrow. Blood. (2003) 101:1446–52. doi: 10.1182/blood-2002-01-0187
63. van der Burg M, Weemaes CMR, Preijers F, Brons P, Barendregt BH, van Tol MJD, et al. B-cell recovery after stem cell transplantation of Artemis-deficient SCID requires elimination of autologous bone marrow precursor-B-cells. Haematologica. (2006) 91:1705–9.
64. Kim J, Zanjani ED, Jeanblanc CM, Goodrich AD, Hematti P. Generation of CD34+ cells from human embryonic stem cells using a clinically applicable methodology and engraftment in the fetal sheep model. Exp Hematol. (2013) 41:749–58.e5. doi: 10.1016/j.exphem.2013.04.003
65. Shultz LD, Brehm MA, Garcia-Martinez JV, Greiner DL. Humanized mice for immune system investigation: progress, promise and challenges. Nat Rev Immunol. (2012) 12:786–98. doi: 10.1038/nri3311
66. Lepus CM, Gibson TF, Gerber SA, Kawikova I, Szczepanik M, Hossain J, et al. Comparison of human fetal liver, umbilical cord blood, and adult blood hematopoietic stem cell engraftment in NOD-scid/γc−/−, Balb/c-Rag1−/− γc−/−, and C.B-17-scid/bg immunodeficient mice. Hum Immunol. (2009) 70:790–802. doi: 10.1016/j.humimm.2009.06.005
Keywords: severe combined immunodeficiency, SCID, swine, biomedical model, humanization
Citation: Boettcher AN, Li Y, Ahrens AP, Kiupel M, Byrne KA, Loving CL, Cino-Ozuna AG, Wiarda JE, Adur M, Schultz B, Swanson JJ, Snella EM, Ho C-S, Charley SE, Kiefer ZE, Cunnick JE, Putz EJ, Dell'Anna G, Jens J, Sathe S, Goldman F, Westin ER, Dekkers JCM, Ross JW and Tuggle CK (2020) Novel Engraftment and T Cell Differentiation of Human Hematopoietic Cells in ART−/− IL2RG−/Y SCID Pigs. Front. Immunol. 11:100. doi: 10.3389/fimmu.2020.00100
Received: 23 August 2019; Accepted: 15 January 2020;
Published: 06 February 2020.
Edited by:
Armin Saalmueller, University of Veterinary Medicine Vienna, AustriaReviewed by:
Maria Montoya, Spanish National Research Council (CSIC), SpainCopyright © 2020 Boettcher, Li, Ahrens, Kiupel, Byrne, Loving, Cino-Ozuna, Wiarda, Adur, Schultz, Swanson, Snella, Ho, Charley, Kiefer, Cunnick, Putz, Dell'Anna, Jens, Sathe, Goldman, Westin, Dekkers, Ross and Tuggle. This is an open-access article distributed under the terms of the Creative Commons Attribution License (CC BY). The use, distribution or reproduction in other forums is permitted, provided the original author(s) and the copyright owner(s) are credited and that the original publication in this journal is cited, in accordance with accepted academic practice. No use, distribution or reproduction is permitted which does not comply with these terms.
*Correspondence: Jason W. Ross, andyb3NzQGlhc3RhdGUuZWR1; Christopher K. Tuggle, Y2t0dWdnbGVAaWFzdGF0ZS5lZHU=
†These authors have contributed equally to this work
Disclaimer: All claims expressed in this article are solely those of the authors and do not necessarily represent those of their affiliated organizations, or those of the publisher, the editors and the reviewers. Any product that may be evaluated in this article or claim that may be made by its manufacturer is not guaranteed or endorsed by the publisher.
Research integrity at Frontiers
Learn more about the work of our research integrity team to safeguard the quality of each article we publish.