- Hematology and Clinical Immunology and Bone Marrow Transplant Program, Department of Medicine, University of Perugia, Perugia, Italy
Since their discovery CD4+FOXP3+ regulatory T cells (Tregs) represented a promising tool to induce tolerance in allogeneic hematopoietic cell transplantation. Preclinical models proved that adoptive transfer of Tregs or the use of compounds that can favor their function in vivo are effective for prevention and treatment of graft-vs.-host disease (GvHD). Following these findings, Treg-based therapies have been employed in clinical trials. Adoptive immunotherapy with Tregs effectively prevents GvHD induced by alloreactive T cells in the setting of one HLA haplotype mismatched hematopoietic transplantation. The absence of post transplant pharmacologic immunosuppression unleashes T-cell mediated graft-vs.-tumor (GvT) effect, which results in an unprecedented, almost complete control of leukemia relapse in this setting. In the present review, we will report preclinical studies and clinical trials that demonstrate Treg ability to promote donor engraftment, protect from GvHD and improve GvT effect. We will also discuss new strategies to further enhance in vivo efficacy of Treg-based therapies.
Introduction
CD4+FOXP3+ regulatory T cells (Tregs) are capable of suppressing the function of conventional CD4+ and CD8+ T cells (Tcons), B cells, NK cells and antigen presenting cells (APCs). They maintain tolerance to self and prevent autoimmune diseases, control excessive immune responses to allergens and pathogens, help maintain a balance with commensal microbial flora and the maternal tolerance to fetus. They have been shown to infiltrate tumors and suppress anti-tumor immunity (1–6). Recent studies have uncovered a role for bone marrow mouse Tregs in the maintenance of the hematopoietic stem cell (HSC) niche and in B cell lymphopoiesis (7, 8). Tregs develop in the thymus with a T cell receptor (TCR) repertoire that overlaps to some extent with that of Tcons (9–12). In addition, studies in mice reported that TGF-β and retinoic acid can induce differentiation of peripheral naïve CD4+ T cells into Tregs in response to antigenic stimulation (13–19). FOXP3 is a transcription factor of the forkhead winged helix family and is the lineage marker for both mouse and human Tregs (20–25), although human Tcons can express low levels of FOXP3 after activation (26, 27). FOXP3 deficiency causes lymphoproliferation and multi-organ autoimmunity in scurfy mice and a lethal X-linked syndrome with immune dysregulation, polyendocrinopathy, and entheropathy in humans (28–31). Recent studies in mouse models suggest that FOXP3 expression is not required to direct thymocyte development to the Treg cell lineage, but it is essential for Treg stability and function (32–34). TCR, IL-2, and TGF-β signaling can induce Foxp3 transcription and are responsible for the maintenance and the function of thymic Tregs and the differentiation of Tregs in the periphery (35–38). Among others, FOXP3 represses the transcription of genes for IL-2 and other inflammatory cytokines, while it activates the transcription of IL2RA, CTLA4, and its own gene (39, 40). The α chain of the IL-2 receptor (IL-2Rα, CD25) and CTLA-4 are constitutively expressed by Tregs, while they are expressed on Tcons upon activation (1, 2, 41–44). Due to the intranuclear localization of FOXP3, CD25 is currently used to select Tregs for functional in vitro studies and cellular therapy, although it is not an exclusive marker. As Tregs do not produce IL-2, they depend on IL-2 produced by other immune cells, mainly Tcons (45). Tregs constitutively express the high-affinity receptor for IL-2 (IL-2Rαβγc), thus they can efficiently compete for IL-2 with Tcons and NK cells (46–48). TCR stimulation activates Tregs that are capable of suppressing antigen-specific responses but can also exert bystander suppression (49). Tregs act through several mechanisms, including production of inhibitory cytokines such us IL-10 and TGF-β, cell-cell contacts, and cytolysis (5, 50). The inhibition of dendritic cell maturation and function is considered a core mechanism of Treg-mediated suppression. CTLA-4 on Tregs binds CD80 and CD86 on dendritic cells and inhibits maturation of APCs and co-stimulation of Tcons (51, 52).
Efforts have been made to exploit Treg function for the treatment of autoimmune and inflammatory diseases. Recently, clinical trials are evaluating Treg-based therapies in allogeneic hematopoietic transplantation (HCT) with promising results. Allogeneic HCT is a life-saving treatment for high-risk hematologic malignancies (53). After a conditioning regimen based on radiotherapy and/or chemotherapy, a bone marrow or a stem cell graft reconstitutes hematopoiesis and immunity of donor origin in the recipient. Residual host T cells that may have survived the conditioning regimen can recognize donor alloantigens and cause rejection. Similarly, donor alloreactive T cells recognize recipient alloantigens, eliminate malignant cells [graft-vs.-tumor (GvT) effect] and can prevent relapse. However, they also attack host normal tissues (mainly skin, gut and liver), causing graft-vs.-host disease (GvHD), that is a major cause of non-relapse mortality (NRM). Pharmacological immune suppression is widely used to prevent GvHD, but it also reduces the GvT effect. Separation of the GvT effect from GvHD is the main goal of the translational research in the field. In this setting, Tregs contribute to induction and maintenance of tolerance to alloantigens, facilitating engraftment and preventing the development of GvHD (54–56).
Induction of Tolerance by Tregs in Preclinical Models of Allogeneic HCT
Mouse models of allogeneic HCT have provided evidence that Tregs suppress T cell alloreactions and can promote engraftment and help control GvHD. The role of Tregs in GvHD has been mostly investigated in MHC mismatched bone marrow transplantation. Pioneering studies demonstrated that CD4+CD25+ T-cell depletion from the bone marrow graft exacerbated GvHD. When additional CD4+CD25+ T cells, either freshly isolated or ex-vivo expanded, were infused, GvHD onset was delayed and even prevented to various degrees (57–60). One study showed that Tregs also protected from GvHD in a minor histocompatibility antigen-disparate, MHC matched setting (60). In a mouse model of fully mismatched T-cell depleted bone marrow transplantation, infusion of donor Tcons killed all the mice within 30 days of acute GvHD. When donor CD4+CD25+ Tregs were co-infused at a 1:1 ratio with Tcons more than 70% of mice were protected from lethal acute GvHD. Co-infusion of Tregs reduced the number of Tcons that could be recovered in lymph nodes and GvHD target tissues such as skin and gut, thus limiting Tcon expansion (61). Importantly, when mice were co-injected with a leukemia or lymphoma cell line, transfer of Tregs did not inhibit Tcon-mediated GvT effect (60–62). Moreover, Treg transfer preserved thymic and lymph node architecture and even accelerated donor lymphoid reconstitution to such an extent that mice survived lethal mouse Cytomegalovirus (CMV) infection (63). Treg homing to lymph nodes and target tissue is an important variable in GvHD prevention. In mouse models of GvHD, bioluminescence analyses showed that Tregs localized to peripheral lymph nodes and spleen in the first 24–48 h with a peak on day 4 after infusion, then they migrated to peripheral tissues. When Tregs were infused 2 days before Tcons, an unfavorable 1:10 ratio with Tcons still protected from GvHD at some extent (64). Moreover, when Tregs were eliminated in vivo 2 days after their transfer and even before Tcon injection, mice survived to GvHD (65). Finally, CD62L− Tregs do not migrate to lymph nodes and are not capable of controlling GvHD (66, 67). Thus, Tregs migrate to lymph nodes and suppress Tcon proliferation early after transplant. However, Tregs could also suppress alloreactive Tcons in GvHD target tissues. One study showed that CCR5-deficient Tregs had reduced migration to mesenteric lymph nodes, liver, lung, and spleen and were less effective in preventing GvHD (68). In another study, CXCR3-transfected Tregs migrated better to liver, lung, and intestine and better controlled GvHD (69). Adoptively transferred third party Tregs also conferred protection from GVHD in mouse models of allogeneic transplantation, although they were less effective than donor Tregs. Third party Tregs survived for a shorter period, probably because they were rejected by donor Tcons. Thus, suppression of alloreactive T cells can also operate through MHC-independent mechanisms (65). Moreover, both radiation-resistant host Tregs and donor Tregs reduced the severity of chronic GvHD in a mouse model of bone marrow transplantation with a minor histocompatibility antigen mismatch (70). The capability of human donor Tregs to prevent and ameliorate GvHD caused by co-infused Tcons has been demonstrated in xenogeneic mouse models (71–73), and one of these studies also showed that the GvT effect was unaffected (73). Similar results were obtained with the infusion of third party human Tregs derived from umbilical cord blood and expanded before infusion (74). The same group also showed that fucosylation of expanded third party Tregs improved prevention of GvHD. In fact, the addition of a fucose formed the moiety found on P-selectin ligand on Treg cell surface, enhancing their persistence in vivo as a result of improved homing to the sites of inflammation (75).
Other studies focused more specifically on the role of Tregs in the engraftment of T-cell depleted bone marrow allografts. Joffre et al. demonstrated that when host Tregs were activated in vitro with donor allogeneic APCs, they inhibited host CD4+ and CD8+ T-cell mediated rejection of donor bone marrow graft, but not of a third-party bone marrow graft (76, 77). Another study showed that either donor or host CD62Lhi, but not CD62Llo, ex-vivo activated Tregs inhibited rejection of MHC-mismatched bone marrow in sublethally irradiated mice (66). In a similar model, donor Tregs promoted engraftment without being activated ex-vivo (78). Steiner et al. showed that third-party Tregs, either naïve or ex-vivo expanded, enhanced engraftment of bone marrow allografts (79). Adoptive transfer of host-type Tregs can also induce mixed chimerism and tolerance to a fully mismatched bone marrow graft after a conditioning with short-course costimulation blockade (with CTLA-4 Ig and anti-CD40L antibody) and rapamycin, in the absence of cytoreductive treatment (80). Rapamycin is used because it limits activation and expansion of Tcons while promoting the expansion of Tregs (81). The ability of Tregs to promote engraftment is consistent with their role in the maintenance of the HSC niche, where they protect HSCs from autologous and allogeneic immune attack (7, 8). Tregs are also critical for tolerance induction to allogeneic HCT after reduced intensity conditioning regimens with total lymphoid irradiation and anti-thymocyte globulin (TLI/ATG). These regimens kill host-type Tcons while partially sparing Tregs, which increase donor HSC engraftment, cell cycling and differentiation (82, 83). These results support the use of TLI/ATG to establish mixed chimerism after allogeneic HCT in patients with hematologic malignancies (84).
Treg-Based Therapies in One HLA Haplotype Mismatched Transplantation
In one HLA haplotype mismatched (haploidentical) hematopoietic transplantation, the high degree of HLA mismatch triggers strong host-vs.-graft and graft-vs.-host alloresponses. In the early 1990s, the combination of a myeloablative and immunosuppressive conditioning regimen with the infusion of a “mega-dose” of T-cell-depleted HSCs made haploidentical transplantation feasible and effective without the need for any post-transplant GvHD prophylaxis. The major limitation of this approach was delayed post-transplant immune recovery, which resulted in ~40% NRM, mainly due to infections (85–87). More recently, protocols of unmanipulated (T-cell replete) haploidentical transplantation have been developed. They are based on new strategies to control T-cell alloreactivity that also rely on Treg-induced tolerance (87, 88). A widespread and effective approach is the administration of high-dose cyclophosphamide following graft infusion (PTCy), which inhibits alloresponses while sparing donor HSCs (88–90). Donor Tregs are resistant to PTCy-induced cytotoxicity upon allogeneic HCT, because they express high levels of the enzyme aldehyde dehydrogenase, which is essential for in vivo detoxification of cyclophosphamide (91). Treg-mediated suppression plays an essential role in the prevention of GvHD in mouse models of allogeneic HCT with PTCy (91, 92). A recent study showed that PTCy induced functional impairment rather than elimination of alloreactive T cells and confirmed a role for Tregs in GvHD mouse models (93). Moreover, recovery of mature and functional natural killer (NK) cells is also impaired in patients undergoing haploidentical transplantation with PTCy (94, 95). Such effects could impair T cell- and NK cell-mediated leukemia killing after transplant. Importantly, clinical data suggest survival after haploidentical transplantation with PTCy is similar to that of patients undergoing HLA-matched sibling or unrelated HCT (96–102). Another trial used a rapamycin-based GvHD prophylaxis in order to promote in vivo expansion of Tregs and allow the infusion of unmanipulated haploidentical grafts (103).
In the setting of T-cell depleted haploidentical transplantation without post-transplant immunosuppression, the Perugia Bone Marrow Transplant Program is exploiting adoptive immunotherapy with freshly isolated donor Tcons and Tregs, in order to promote immune reconstitution while preventing GvHD (73, 87, 104, 105). CD4+CD25+ Tregs are isolated by a two-step immunomagnetic selection consisting of a negative CD19/CD8 selection, followed by a positive CD25 selection. The purity of CD4+FOXP3+ Tregs is around 70–80%. 2 × 106 Tregs/kg are infused 4 days before 1 × 106 Tcons/kg and about 10 × 106 CD34+ cells/Kg. No pharmacological GvHD prophylaxis is given. The first study reported results in 28 patients with hematologic malignancies (24 in any complete remission and 4 in relapse at transplant). They received a conditioning regimen with TBI, thiotepa, fludarabine, and cyclophosphamide. Twenty-six patients engrafted. Treg/Tcon adoptive immunotherapy was associated with rapid reconstitution of B cells and of T cells with a wide repertoire. Compared with standard T-cell depleted haploidentical transplantation, reconstitution of pathogen-specific CD4+ and CD8+ T cells, and of mature NK cells was faster. The incidence of CMV reactivation was markedly reduced, with no CMV-related deaths. Two patients developed ≥grade 2 acute GvHD and no patient developed chronic GvHD. One patient (in chemoresistant relapse at transplant) relapsed. Thirteen patients died of NRM, 4 of them because of extra-hematological toxicity (104). The second study extended the analysis to 43 patients with high-risk leukemia in any remission, including 24 reported before. In order to reduce NRM, a lower dose of cyclophosphamide or anti-T cell antibodies in the place of cyclophosphamide were used in the conditioning regimen. Forty-one patients engrafted. NRM was 40% and fell to 21% in patients who had received anti-T cell antibodies as a part of the conditioning. Incidence of ≥grade 2 acute GvHD was 15% and only one patient developed chronic GvHD. Incidence of leukemia relapse was 5% and it was significantly reduced compared to the standard protocol of T-cell depleted haploidentical transplantation (73). Finally, in an updated analysis of a total of 60 patients with acute leukemia in any remission at transplant, incidence of acute grade II-IV GvHD and chronic GvHD were 15 and 3%, respectively. Five-year incidence of NRM was 35%. Five-year relapse incidence was as low as 12%, confirming preclinical data that Treg adoptive transfer does not impair T-cell immune reconstitution and the GvT effect (87). More recently, this strategy of adoptive immunotherapy with Treg/Tcon in haploidentical transplantation has been combined with a low-toxicity conditioning regimen based on total marrow and lymphoid irradiation (TMLI). TMLI provides high-intensity irradiation to bone marrow, spleen, and major lymph node chains, while sparing other tissues (106). This transplant protocol has been tested in acute myeloid leukemia patients who were aged or unfit to receive total body irradiation. Preliminary data indicate this combination provides promising results in terms of control of leukemia relapse and chronic GvHD/leukemia-free survival (107). Despite acute GvHD still occurs in a relevant fraction of patients, Treg/Tcon adoptive immunotherapy strikingly improves outcomes of T-cell depleted haploidentical transplantation thanks to a better chronic GvHD/leukemia-free survival.
Treg-Based Therapies in HLA-Matched and Umbilical Cord Blood Transplantation
Since the number of freshly isolated Tregs to be used for cellular therapy is limited (5–10% of CD4+ T cells in peripheral blood), several protocols of expansion under good manufacturing practice have been developed and tested in clinical trials (108). Such expansion protocols preserve FOXP3+ Treg purity and suppressive function in vitro. Tregs can be isolated from peripheral blood or umbilical cord blood by immunomagnetic selection as described above, or by flow cytometric cell sorting. Tregs are usually incubated with anti-CD3/CD28 beads and high-dose IL-2 with or without rapamycin for around 2–3 weeks (108–112). Another option is the use of a modified K562 cell line, which expresses the Fc receptor CD64 to cross-link an anti-CD3 antibody, and CD86 to provide co-stimulation in the presence of IL-2 (113–115). Brunstein and colleagues used this approach to prevent GvHD after double-umbilical cord blood transplantation. Tregs were isolated by CD25 immunomagnetic selection from a third party cord blood unit that was 4–6/6 HLA matched with the patient, and expanded in vitro (114). In the last published clinical study, 3–100 × 106 Tregs/kg were infused in 11 patients with various hematologic malignancies, who also received post-transplant immune suppression. Expanded Tregs were detectable in patients for a maximum of 14 days after the infusion. The incidence of grade II-IV acute GvHD at 100 days was 9% compared with 45% in contemporary controls with the same transplant protocol without Treg infusion. Chronic GvHD was 0% compared with 14% in controls. Incidence of infections, NRM and relapse, and disease-free survival were similar in patients infused with Tregs and in controls (114). Such studies suggest third party Tregs can be an alternative to donor-derived Tregs. However, donor and third party Tregs have never been compared in clinical studies for their efficacy in GvHD prevention. The infusion of donor expanded Tregs has been also tested for the treatment of acute and chronic GvHD in small series of patients after HLA-matched transplantation, with a clinical response in some patients (109, 111).
A recent phase I/II study investigated the infusion of fresh donor Tregs and Tcons in the setting HLA-matched transplantation in 12 patients with various hematological malignancies (116). Tregs were purified by CD25+ immunomagnetic selection, followed by flow cytometric cell sorting of CD4+CD127loCD25+ cells (as Treg cells do not express the IL-7 receptor α subunit CD127 or express it at low intensity). Purity of CD4+FOXP3+ Tregs was over 90%. The first cohort of 5 patients received frozen Tregs but showed signs of GvHD, consistent with the reduced functionality of Tregs after cryopreservation and thawing (117). The other 7 patients received freshly isolated Tregs in combination with low-dose single-agent GvHD prophylaxis. No acute or chronic GvHD was observed (116).
Treatment With Low-Dose IL-2 in Allogeneic HCT
A promising alternative to Treg adoptive transfer is low-dose IL-2 therapy, that has been shown to selectively activate and expand Tregs in several autoimmune and inflammatory settings, due to the constitutive expression of CD25 and factors related to IL-2 receptor signaling (118, 119). The group from Dana-Faber Cancer Institute and Harvard University investigated in vivo stimulation of Tregs with low-dose IL-2 for the treatment of steroid-refractory chronic GvHD after allogeneic HCT. A phase I study established that the maximum tolerated dose of IL-2 was 1 × 106 IU/m2/day. During the 8 weeks of treatment, Treg counts and the Treg:Tcon ratio rose and 52% of patients had a clinical response (120). In a phase II study, 35 patients with steroid-refractory chronic GvHD were treated for 12 weeks. Two patients withdrew, 5 patients required dose reduction, and 61% of evaluable patients had a partial response to treatment. After a 4-week hiatus, low-dose IL-2 therapy could be extended for 2 years in patients with partial response or stable disease (121). The same group showed that low-dose IL-2 induced phosphorylation of signal transducer and activator of transcription 5 (STAT5) in Tregs but not in Tcons and preferentially expanded Tregs in vitro. Moreover, patients with severe chronic GvHD had constitutive phosphorylation of STAT5 in Tcons and high levels of IL-7 and IL-15. Treatment with low-dose IL-2 was associated with increased STAT5 phosphorylation in Tregs and decreased STAT5 phosphorylation in Tcons. This resulted in enhanced Treg proliferation, thymic export and resistance to apoptosis (122). Strategies to enhance efficacy of low-dose IL-2 therapy are under investigation and include combination with infusion of purified Tregs or extracorporeal photopheresis (123, 124). Another phase II study used ultra-low dose IL-2 for GvHD prophylaxis in 16 pediatric recipients of allogeneic HCT. Treatment started at a median of 28 days after transplant and consisted of 1–2 × 105 IU/m2 three times per week for 6 or 12 weeks. This treatment was safe and it was associated with expansion of Tregs in vivo. None of the patients developed II-IV GvHD, while the incidence of acute GvHD was 12% in a control group of patients who did not receive IL-2 treatment (125).
Perspectives
Recent clinical trials demonstrate that Treg-based therapies can effectively promote engraftment and prevent or treat GvHD after allogeneic HCT. However, these treatments fail to protect some patients from severe GvHD. Strategies to improve purity, enhance specificity, promote activation and control localization of Tregs are needed. Several experimental strategies are under investigation to achieve this goal.
Recent studies have focused on the generation of IL-2 compounds with enhanced selectivity for Tregs (126). One strategy is the use of anti-IL-2 antibodies in complex with IL-2 that allow for selective stimulation of T cell subsets. Boyman et al. showed that one anti-mouse IL-2 antibody prevented the binding of IL-2 to IL-2Rβγc, but not to CD25 (IL-2Rα). When complexed with IL-2, this antibody preferentially triggered the proliferation of Tregs (127). Subsequently, Trotta et al. reported the generation of a fully human anti-IL-2 antibody (F5111.2) that exerted the same effect on human Tregs when complexed with IL-2. Treatment with F5111.2-human IL-2 complexes was effective in preclinical models of autoimmune diseases and GvHD, and it did not affect immune response to mouse CMV (128). IL-2 can also be covalently linked to the antibody to generate a single-agent cytokine/antibody fusion that is more stable than the above immune complexes (129). Ward et al. designed a fusion protein of mouse IL-2 and CD25 that allows for a selective stimulation of Tregs in vivo. A treatment with this fusion protein delayed the development of diabetes in non-obese diabetic mice (130). An alternative strategy is engineering human IL-2 so that it preferentially binds the high affinity receptor IL-2Rαβγc and, consequently, preferentially activates Tregs (131, 132). Moreover, these IL-2 muteins are also designed to have a longer half-life and can provide a persistent stimulation of Tregs (130–132).
Another pleiotropic cytokine, TNF-α, has been recently shown to enhance Treg function. After the conditioning regimen, tissue macrophages release TNF-α, which activates donor alloreactive T cells that cause GvHD (133, 134). In fact, anti-TNF-α therapy with infliximab and etanercept is used to treat steroid-refractory chronic GvHD. However, some patients do not respond or even worsen (134–136). Tregs express higher levels of TNF receptor (TNFR) 2 compared with Tcons, and TNFR2+ Tregs are more suppressive than TNFR2− Tregs (137–140). While TNFR1−/− mice have defective immunity to infections and inflammatory response, TNFR2−/− mice are affected by exacerbated inflammation (141). Several reports suggest that TNF-α/TNFR2 signaling is required for effective mouse Treg development in the thymus and optimal function in vivo (142–145). In mouse models of allogeneic bone marrow transplantation, GvHD control was abrogated when mice were infused with TNFR2 deficient Tregs or with a TNFR2 blocking monoclonal antibody (143). Moreover, ex-vivo priming of Tregs with TNF-α enhanced the effectiveness of GvHD prevention (144). Finally, expansion of radiation resistant host Tregs with a TNFR2 agonist reduced GvHD severity and improved survival (145). Importantly, the GvT effect was unaffected by Treg stimulation in these studies. Ex-vivo priming or expansion of Tregs with TNF-α or a TNFR2 agonist before adoptive transfer could improve prevention and treatment of GvHD. TNFR2 agonists could also be used to expand Tregs in vivo (146, 147). As Tregs express higher levels of TNFR2, they could be preferentially activated. However, Tcons upregulate TNFR2 expression upon activation and become more resistant to Treg-mediated suppression (148). Thus, the effects of in vivo TNFR2 signaling stimulation should be carefully evaluated. Another option is treatment with agonists of the costimulatory receptor TNFR superfamily 25 (Death receptor 3, DR3), which strongly stimulate Treg proliferation while weakly affect CD4+ T cell proliferation (149, 150). Treatment of donor mice with DR3 agonists reduced severity of GvHD in recipient mice of MHC-mismatched bone marrow transplantation, preserving GvT effects (151). Moreover, when recipient mice received a prophylactic treatment with a DR3 agonist, recipient-derived Treg expanded and severity of GvHD was reduced. In contrast, treatment of recipient mice after transplant favored donor Tcon alloreactions and worsened GvHD (152). In other studies, Treg expansion was induced in donors with the combination of a DR3 agonist and low-dose IL-2. The infusion of donor expanded Tregs ameliorated GvHD but did not affect GvT activity in both MHC matched and MHC mismatched bone marrow transplantation models (153–155). Recently, Copsel et al. combined this approach with the administration of inhibitors of bromodomain and extra-terminal proteins (BETi), which suppress expression of pro-inflammatory cytokines and other genes involved in T cell activation. They found that BETi EP11313 spared Tregs and that GvHD severity was reduced in mice treated with EP11313 and low numbers of donor Tregs expanded with DR3 agonist and low-dose IL-2 (156).
While current protocols of adoptive transfer use polyclonal Tregs, antigen-specific Tregs could be more effective without exerting a broad suppression of immune responses. Alloantigen-specific Tregs can be generated in the presence of allogeneic APCs or by TCR gene transfer. An alternative is the generation of chimeric antigen receptors Tregs (CAR-Tregs) (157). MacDonald et al. generated human HLA-A2-specific CAR-Tregs that prevented GvHD caused by HLA-A2+ Tcons in a xenogeneic mouse model. They were more effective than Tregs expressing an irrelevant CAR (158). A similar strategy was also used to prevent rejection after xenogeneic transplantation (159). Another study used Tregs expressing a CAR that binds Fluorescein isothiocyanate-conjugated monoclonal antibodies (mAbCAR-Tregs). Using tissue-specific antibodies, mAbCAR-Tregs could be directed to different sites, where they exerted their suppressive function (160).
Finally, several compounds are under investigation for the prevention and treatment of GvHD, such as Janus-activated kinase inhibitors and others. They share the ability to preferentially inhibit Tcon function while sparing Treg suppressive activity to various degrees (161). Thus, Tregs play a key role in many of the current and newly developed strategies to induce tolerance in allogeneic HCT.
Conclusions
Treg-based therapies have provided promising clinical responses in allogeneic HCT. These treatments have proven to be safe and are not associated with the side effects that could have been anticipated, such as increased susceptibility to infections and leukemia relapse. Efforts are ongoing to improve the effectiveness of these approaches, whether they are based on adoptive transfer of freshly isolated, expanded or modified Tregs or on the induction of Treg expansion and function in vivo (Figure 1).
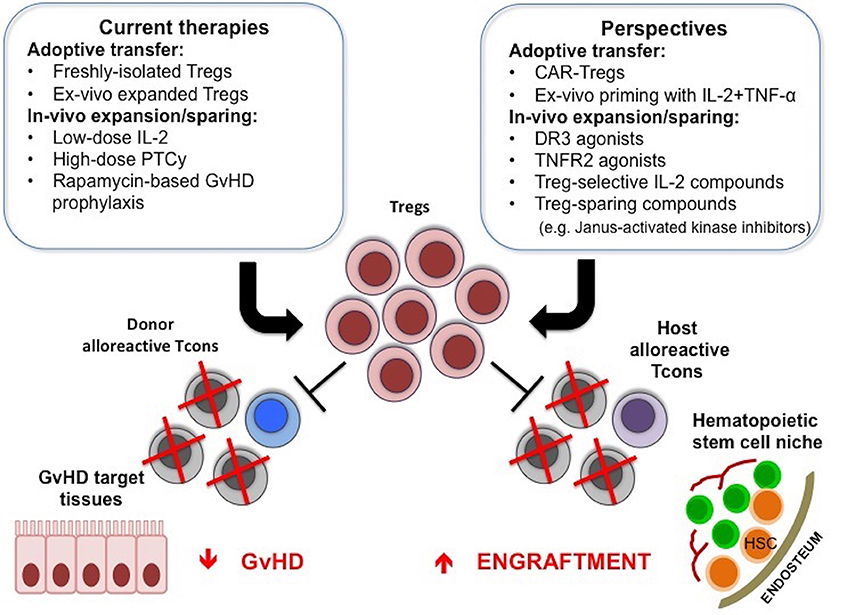
Figure 1. Treg-based therapies in allogeneic HCT. Adoptive transfer of Tregs or the use of compounds that can favor their function in vivo are used to promote donor engraftment and protect from GvHD after HCT. New strategies to further enhance in vivo efficacy of Treg-based therapies are under active investigation, and include CAR-Tregs, ex-vivo priming with IL-2 and TNF-α, TNFR2, or DR3 agonists, Treg-selective IL-2 compounds or compounds that inhibit Tcon function while sparing Treg suppressive activity.
To these days, treatment of patients with chemoresistant leukemia is still largely ineffective. In the present review, we discussed that Treg-adoptive transfer allows for a strong Tcon-mediated GvT effect while controlling GvHD. Although it requires further optimization, we believe this strategy is close to become an effective treatment for these patients.
Author Contributions
AM and SP wrote the manuscript. AV reviewed the manuscript. AP wrote and reviewed the manuscript.
Funding
The preparation of the article was funded by Associazione Italiana per la Ricerca sul Cancro (AIRC), Start-Up Grant 2017, project code: 20456.
Conflict of Interest
The authors declare that the research was conducted in the absence of any commercial or financial relationships that could be construed as a potential conflict of interest.
References
1. Sakaguchi S, Sakaguchi N, Asano M, Itoh M, Toda M. Immunologic self-tolerance maintained by activated T cells expressing IL-2 receptor alpha-chains (CD25). Breakdown of a single mechanism of self-tolerance causes various autoimmune diseases. J Immunol. (1995) 155:1151–64.
2. Takahashi T, Kuniyasu Y, Toda M, Sakaguchi N, Itoh M, Iwata M, et al. Immunologic self-tolerance maintained by CD25+CD4+ naturally anergic and suppressive T cells: induction of autoimmune disease by breaking their anergic/suppressive state. Int Immunol. (1998) 10:1969–80. doi: 10.1093/intimm/10.12.1969
3. Kim JM, Rasmussen JP, Rudensky AY. Regulatory T cells prevent catastrophic autoimmunity throughout the lifespan of mice. Nat Immunol. (2007) 8:191–7. doi: 10.1038/ni1428
4. Sakaguchi S, Yamaguchi T, Nomura T, Ono M. Regulatory T cells and immune tolerance. Cell. (2008) 133:775–87. doi: 10.1016/j.cell.2008.05.009
5. Sakaguchi S, Miyara M, Costantino CM, Hafler DA. FOXP3+ regulatory T cells in the human immune system. Nat Rev Immunol. (2010) 10:490–500. doi: 10.1038/nri2785
6. Wing JB, Tanaka A, Sakaguchi S. Human FOXP3+ regulatory T cell heterogeneity and function in autoimmunity and cancer. Immunity. (2019) 50:302–16. doi: 10.1016/j.immuni.2019.01.020
7. Fujisaki J, Wu J, Carlson AL, Silberstein L, Putheti P, Larocca R, et al. In vivo imaging of Treg cells providing immune privilege to the haematopoietic stem- cell niche. Nature. (2011) 474:216–9. doi: 10.1038/nature10160
8. Pierini A, Nishikii H, Baker J, Kimura T, Kwon HS, Pan Y, et al. Foxp3+ regulatory T cells maintain the bone marrow microenvironment for B cell lymphopoiesis. Nat Commun. (2017) 8:15068. doi: 10.1038/ncomms15068
9. Itoh M, Takahashi T, Sakaguchi N, Kuniyasu Y, Shimizu J, Otsuka F, et al. Thymus and autoimmunity: production of CD25+CD4+ naturally anergic and suppressive T cells as a key function of the thymus in maintaining immunologic self-tolerance. J Immunol. (1999) 162:5317–26.
10. Darrasse-Jèze G, Marodon G, Salomon BL, Catala M, Klatzmann D. Ontogeny of CD4+CD25+ regulatory/suppressor T cells in human fetuses. Blood. (2005) 105:4715–21. doi: 10.1182/blood-2004-10-4051
11. Jordan MS, Boesteanu A, Reed AJ, Petrone AL, Holenbeck AE, Lerman MA, et al. Thymic selection of CD4+CD25+ regulatory T cells induced by an agonist self-peptide. Nat Immunol. (2001) 2:301–6. doi: 10.1038/86302
12. Hsieh CS, Zheng Y, Liang Y, Fontenot JD, Rudensky AY. An intersection between the self-reactive regulatory and nonregulatory T cell receptor repertoires. Nat Immunol. (2006) 7:401–10. doi: 10.1038/ni1318
13. Chen W, Jin W, Hardegen N, Lei KJ, Li L, Marinos N, et al. Conversion of peripheral CD4+CD25− naive T cells to CD4+CD25+ regulatory T cells by TGF-beta induction of transcription factor Foxp3. J Exp Med. (2003) 198:1875–86. doi: 10.1084/jem.20030152
14. Apostolou I, von Boehmer H. In vivo instruction of suppressor commitment in naive T cells. J Exp Med. (2004) 199:1401–8. doi: 10.1084/jem.20040249
15. Kretschmer K, Apostolou I, Hawiger D, Khazaie K, Nussenzweig MC, von Boehmer H. Inducing and expanding regulatory T cell populations by foreign antigen. Nat Immunol. (2005) 6:1219–27. doi: 10.1038/ni1265
16. Benson MJ, Pino-Lagos K, Rosemblatt M, Noelle RJ. All-trans retinoic acid mediates enhanced T reg cell growth, differentiation, and gut homing in the face of high levels of co-stimulation. J Exp Med. (2007) 204:1765–74. doi: 10.1084/jem.20070719
17. Mucida D, Park Y, Kim G, Turovskaya O, Scott I, Kronenberg M, et al. Reciprocal TH17 and regulatory T cell differentiation mediated by retinoic acid. Science. (2007) 317:256–60. doi: 10.1126/science.1145697
18. Sun CM, Hall JA, Blank RB, Bouladoux N, Oukka M, Mora JR, et al. Small intestine lamina propria dendritic cells promote de novo generation of Foxp3 T reg cells via retinoic acid. J Exp Med. (2007) 204:1775–85. doi: 10.1084/jem.20070602
19. Coombes JL, Siddiqui KR, Arancibia-Cárcamo CV, Hall J, Sun CM, Belkaid Y, et al. A functionally specialized population of mucosal CD103+ DCs induces Foxp3+ regulatory T cells via a TGF-beta and retinoic acid-dependent mechanism. J Exp Med. (2007) 204:1757–64. doi: 10.1084/jem.20070590
20. Khattri R, Cox T, Yasayko SA, Ramsdell F. An essential role for Scurfin in CD4+CD25+ T regulatory cells. Nat Immunol. (2003) 4:337–42. doi: 10.1038/ni909
21. Fontenot JD, Gavin MA, Rudensky AY. Foxp3 programs the development and function of CD4+CD25+ regulatory T cells. Nat Immunol. (2003) 4:330–6. doi: 10.1038/ni904
22. Hori S, Nomura T, Sakaguchi S. Control of regulatory T cell development by the transcription factor Foxp3. Science. (2003) 299:1057–61. doi: 10.1126/science.1079490
23. Yagi H, Nomura T, Nakamura K, Yamazaki S, Kitawaki T, Hori S, et al. Crucial role of FOXP3 in the development and function of human CD25+CD4+ regulatory T cells. Int Immunol. (2004) 16:1643–56. doi: 10.1093/intimm/dxh165
24. Roncador G, Brown PJ, Maestre L, Hue S, Martínez-Torrecuadrada JL, Ling KL, et al. Analysis of FOXP3 protein expression in human CD4+CD25+ regulatory T cells at the single-cell level. Eur J Immunol. (2005) 35:1681–91. doi: 10.1002/eji.200526189
25. Miyara M, Yoshioka Y, Kitoh A, Shima T, Wing K, Niwa A, et al. Functional delineation and differentiation dynamics of human CD4+ T cells expressing the FoxP3 transcription factor. Immunity. (2009) 30:899–911. doi: 10.1016/j.immuni.2009.03.019
26. Gavin MA, Torgerson TR, Houston E, DeRoos P, Ho WY, Stray-Pedersen A, et al. Single-cell analysis of normal and FOXP3-mutant human T cells: FOXP3 expression without regulatory T cell development. Proc Natl Acad Sci USA. (2006) 103:6659–64. doi: 10.1073/pnas.0509484103
27. Wang J, Ioan-Facsinay A, van der Voort EI, Huizinga TW, Toes RE. Transient expression of FOXP3 in human activated nonregulatory CD4+ T cells. Eur J Immunol. (2007) 37:129–38. doi: 10.1002/eji.200636435
28. Brunkow ME, Jeffery EW, Hjerrild KA, Paeper B, Clark LB, Yasayko SA, et al. Disruption of a new forkhead/winged-helix protein, scurfin, results in the fatal lymphoproliferative disorder of the scurfy mouse. Nat Genet. (2001) 27:68–73. doi: 10.1038/83784
29. Wildin RS, Ramsdell F, Peake J, Faravelli F, Casanova JL, Buist N, et al. X-linked neonatal diabetes mellitus, enteropathy and endocrinopathy syndrome is the human equivalent of mouse scurfy. Nat Genet. (2001) 27:18–20. doi: 10.1038/83707
30. Bennett CL, Christie J, Ramsdell F, Brunkow ME, Ferguson PJ, Whitesell L, et al. The immune dysregulation, polyendocrinopathy, enteropathy, X-linked syndrome (IPEX) is caused by mutations of FOXP3. Nat Genet. (2001) 27:20–1. doi: 10.1038/83713
31. Chatila TA, Blaeser F, Ho N, Lederman HM, Voulgaropoulos C, Helms C, et al. JM2, encoding a fork head-related protein, is mutated in X-linked autoimmunity-allergic disregulation syndrome. J Clin Invest. (2000) 106:R75–81. doi: 10.1172/JCI11679
32. Gavin MA, Rasmussen JP, Fontenot JD, Vasta V, Manganiello VC, Beavo JA, et al. Foxp3-dependent programme of regulatory T-cell differentiation. Nature. (2007) 445:771–5. doi: 10.1038/nature05543
33. Lin W, Haribhai D, Relland LM, Truong N, Carlson MR, Williams CB, et al. Regulatory T cell development in the absence of functional Foxp3. Nat Immunol. (2007) 8:359–68. doi: 10.1038/ni1445
34. Lio CW, Hsieh CS. A two-step process for thymic regulatory T cell development. Immunity. (2008) 28:100–11. doi: 10.1016/j.immuni.2007.11.021
35. Zheng Y, Josefowicz S, Chaudhry A, Peng XP, Forbush K, Rudensky AY. Role of conserved non-coding DNA elements in the Foxp3 gene in regulatory T-cell fate. Nature. (2010) 463:808–12. doi: 10.1038/nature08750
36. Ohkura N, Hamaguchi M, Morikawa H, Sugimura K, Tanaka A, Ito Y, et al. T cell receptor stimulation-induced epigenetic changes and Foxp3 expression are independent and complementary events required for Treg cell development. Immunity. (2012) 37:785–99. doi: 10.1016/j.immuni.2012.09.010
37. Feng Y, van der Veeken J, Shugay M, Putintseva EV, Osmanbeyoglu HU, Dikiy S, et al. A mechanism for expansion of regulatory T-cell repertoire and its role in self-tolerance. Nature. (2015) 528:132–6. doi: 10.1038/nature16141
38. Iizuka-Koga M, Nakatsukasa H, Ito M, Akanuma T, Lu Q, Yoshimura A. Induction and maintenance of regulatory T cells by transcription factors and epigenetic modifications. J Autoimmun. (2017) 83:113–21. doi: 10.1016/j.jaut.2017.07.002
39. Bettelli E, Dastrange M, Oukka M. Foxp3 interacts with nuclear factor of activated T cells and NF-kappa B to repress cytokine gene expression and effector functions of T helper cells. Proc Natl Acad Sci USA. (2005) 102:5138–43. doi: 10.1073/pnas.0501675102
40. Wu Y, Borde M, Heissmeyer V, Feuerer M, Lapan AD, Stroud JC, et al. FOXP3 controls regulatory T cell function through cooperation with NFAT. Cell. (2006) 126:375–87. doi: 10.1016/j.cell.2006.05.042
41. Read S, Malmström V, Powrie F. Cytotoxic T lymphocyte-associated antigen 4 plays an essential role in the function of CD25(+)CD4(+) regulatory cells that control intestinal inflammation. J Exp Med. (2000) 192:295–302. doi: 10.1084/jem.192.2.295
42. Salomon B, Lenschow DJ, Rhee L, Ashourian N, Singh B, Sharpe A, et al. B7/CD28 costimulation is essential for the homeostasis of the CD4+CD25+ immunoregulatory T cells that control autoimmune diabetes. Immunity. (2000) 12:431–40. doi: 10.1016/S1074-7613(00)80195-8
43. Takahashi T, Tagami T, Yamazaki S, Uede T, Shimizu J, Sakaguchi N, et al. Immunologic self-tolerance maintained by CD25(+)CD4(+) regulatory T cells constitutively expressing cytotoxic T lymphocyte-associated antigen 4. J Exp Med. (2000) 192:303–10. doi: 10.1084/jem.192.2.303
44. Paust S, Lu L, McCarty N, Cantor H. Engagement of B7 on effector T cells by regulatory T cells prevents autoimmune disease. Proc Natl Acad Sci USA. (2004) 101:10398–403. doi: 10.1073/pnas.0403342101
45. Owen DL, Mahmud SA, Vang KB, Kelly RM, Blazar BR, Smith KA, et al. Identification of cellular sources of IL-2 needed for regulatory T cell development and homeostasis. J Immunol. (2018) 200:3926–33. doi: 10.4049/jimmunol.1800097
46. Pandiyan P, Zheng L, Ishihara S, Reed J, Lenardo MJ. CD4+CD25+Foxp3+ regulatory T cells induce cytokine deprivation-mediated apoptosis of effector CD4+ T cells. Nat Immunol. (2007) 8:1353–62. doi: 10.1038/ni1536
47. Gasteiger G, Hemmers S, Firth MA, Le Floc'h A, Huse M, Sun JC, et al. IL-2-dependent tuning of NK cell sensitivity for target cells is controlled by regulatory T cells. J Exp Med. (2013) 210:1167–78. doi: 10.1084/jem.20122462
48. Sitrin J, Ring A, Garcia KC, Benoist C, Mathis D. Regulatory T cells control NK cells in an insulitic lesion by depriving them of IL-2. J Exp Med. (2013) 210:1153–65. doi: 10.1084/jem.20122248
49. Thornton AM, Shevach EM. Suppressor effector function of CD4+CD25+ immunoregulatory T cells is antigen nonspecific. J Immunol. (2000) 164:183–90. doi: 10.4049/jimmunol.164.1.183
50. Shevach EM. Mechanisms of foxp3+ T regulatory cell-mediated suppression. Immunity. (2009) 30:636–45. doi: 10.1016/j.immuni.2009.04.010
51. Onishi Y, Fehervari Z, Yamaguchi T, Sakaguchi S. Foxp3+ natural regulatory T cells preferentially form aggregates on dendritic cells in vitro and actively inhibit their maturation. Proc Natl Acad Sci USA. (2008) 105:10113–8. doi: 10.1073/pnas.0711106105
52. Wing K, Onishi Y, Prieto-Martin P, Yamaguchi T, Miyara M, Fehervari Z, et al. CTLA-4 control over Foxp3+ regulatory T cell function. Science. (2008) 322:271–5. doi: 10.1126/science.1160062
53. Appelbaum FR, Forman SJ, Negrin RS, Blume KG. Thomas' Hematopoietic Cell Transplantation. Oxford: Wiley-Blackwell (2009). doi: 10.1002/9781444303537
54. Wood KJ, Sakaguchi S. Regulatory T cells in transplantation tolerance. Nat Rev Immunol. (2003) 3:199–210. doi: 10.1038/nri1027
55. Nguyen VH, Zeiser R, Negrin RS. Role of naturally arising regulatory T cells in hematopoietic cell transplantation. Biol Blood Marrow Transplant. (2006) 12:995–1009. doi: 10.1016/j.bbmt.2006.04.009
56. Pierini A, Alvarez M, Negrin RS. NK cell and CD4+FoxP3+ regulatory T cell based therapies for hematopoietic stem cell engraftment. Stem Cells Int. (2016) 2016:9025835. doi: 10.1155/2016/9025835
57. Cohen JL, Trenado A, Vasey D, Klatzmann D, Salomon BL. CD4(+)CD25(+) immunoregulatory T cells: new therapeutics for graft-versus-host disease. J Exp Med. (2002) 196:401–6. doi: 10.1084/jem.20020090
58. Hoffmann P, Ermann J, Edinger M, Fathman CG, Strober S. Donor-type CD4(+)CD25(+) regulatory T cells suppress lethal acute graft-versus-host disease after allogeneic bone marrow transplantation. J Exp Med. (2002) 196:389–99. doi: 10.1084/jem.20020399
59. Taylor PA, Lees CJ, Blazar BR. The infusion of ex vivo activated and expanded CD4(+)CD25(+) immune regulatory cells inhibits graft-versus-host disease lethality. Blood. (2002) 99:3493–9. doi: 10.1182/blood.V99.10.3493
60. Jones SC, Murphy GF, Korngold R. Post-hematopoietic cell transplantation control of graft-versus-host disease by donor CD425 T cells to allow an effective graft-versus-leukemia response. Biol Blood Marrow Transplant. (2003) 9:243–56. doi: 10.1053/bbmt.2003.50027
61. Edinger M, Hoffmann P, Ermann J, Drago K, Fathman CG, Strober S, et al. CD4+CD25+ regulatory T cells preserve graft-versus-tumor activity while inhibiting graft-versus-host disease after bone marrow transplantation. Nat Med. (2003) 9:1144–50. doi: 10.1038/nm915
62. Trenado A, Charlotte F, Fisson S, Yagello M, Klatzmann D, Salomon BL, et al. Recipient-type specific CD4+CD25+ regulatory T cells favor immune reconstitution and control graft-versus-host disease while maintaining graft-versus-leukemia. J Clin Invest. (2003) 112:1688–96. doi: 10.1172/JCI17702
63. Nguyen VH, Shashidhar S, Chang DS, Ho L, Kambham N, Bachmann M, et al. The impact of regulatory T cells on T-cell immunity following hematopoietic cell transplantation. Blood. (2008) 111:945–53. doi: 10.1182/blood-2007-07-103895
64. Nguyen VH, Zeiser R, Dasilva DL, Chang DS, Beilhack A, Contag CH, et al. In vivo dynamics of regulatory T-cell trafficking and survival predict effective strategies to control graft-versus-host disease following allogeneic transplantation. Blood. (2007) 109:2649–56. doi: 10.1182/blood-2006-08-044529
65. Pierini A, Colonna L, Alvarez M, Schneidawind D, Nishikii H, Baker J, et al. Donor requirements for regulatory T cell suppression of murine graft-versus-host disease. J Immunol. (2015) 195:347–55. doi: 10.4049/jimmunol.1402861
66. Taylor PA, Panoskaltsis-Mortari A, Swedin JM, Lucas PJ, Gress RE, Levine BL, et al. L-Selectin(hi) but not the L-selectin(lo) CD4+25+ T-regulatory cells are potent inhibitors of GVHD and BM graft rejection. Blood. (2004) 104:3804–12. doi: 10.1182/blood-2004-05-1850
67. Ermann J, Hoffmann P, Edinger M, Dutt S, Blankenberg FG, Higgins JP, et al. Only the CD62L+ subpopulation of CD4+CD25+ regulatory T cells protects from lethal acute GVHD. Blood. (2005) 105:2220–6. doi: 10.1182/blood-2004-05-2044
68. Wysocki CA, Jiang Q, Panoskaltsis-Mortari A, Taylor PA, McKinnon KP, Su L, et al. Critical role for CCR5 in the function of donor CD4+CD25+ regulatory T cells during acute graft-versus-host disease. Blood. (2005) 106:3300–7. doi: 10.1182/blood-2005-04-1632
69. Hasegawa H, Inoue A, Kohno M, Lei J, Miyazaki T, Yoshie O, et al. Therapeutic effect of CXCR3-expressing regulatory T cells on liver, lung and intestinal damages in a murine acute GVHD model. Gene Ther. (2008) 15:171–82. doi: 10.1038/sj.gt.3303051
70. Anderson BE, McNiff JM, Matte C, Athanasiadis I, Shlomchik WD, Shlomchik MJ. Recipient CD4+ T cells that survive irradiation regulate chronic graft-versus-host disease. Blood. (2004) 104:1565–73. doi: 10.1182/blood-2004-01-0328
71. Mutis T, van Rijn RS, Simonetti ER, Aarts-Riemens T, Emmelot ME, van Bloois L, et al. Human regulatory T cells control xenogeneic graft-versus-host disease induced by autologous T cells in RAG2−/−gammac−/− immunodeficient mice. Clin Cancer Res. (2006) 12:5520–5. doi: 10.1158/1078-0432.CCR-06-0035
72. Hippen KL, Harker-Murray P, Porter SB, Merkel SC, Londer A, Taylor DK, et al. Umbilical cord blood regulatory T-cell expansion and functional effects of tumor necrosis factor receptor family members OX40 and 4-1BB expressed on artificial antigen-presenting cells. Blood. (2008) 112:2847–57. doi: 10.1182/blood-2008-01-132951
73. Martelli MF, Di Ianni M, Ruggeri L, Falzetti F, Carotti A, Terenzi A, et al. HLA-haploidentical transplantation with regulatory and conventional T cell adoptive immunotherapy prevents acute leukemia relapse. Blood. (2014) 124:638–44. doi: 10.1182/blood-2014-03-564401
74. Parmar S, Liu X, Tung SS, Robinson SN, Rodriguez G, Cooper LJ, et al. Third-party umbilical cord blood-derived regulatory T cells prevent xenogenic graft-versus-host disease. Cytotherapy. (2014) 16:90–100. doi: 10.1016/j.jcyt.2013.07.009
75. Parmar S, Liu X, Najjar A, Shah N, Yang H, Yvon E, et al. Ex vivo fucosylation of third-party human regulatory T cells enhances anti-graft-versus-host disease potency in vivo. Blood. (2015) 125:1502–6. doi: 10.1182/blood-2014-10-603449
76. Joffre O, Gorsse N, Romagnoli P, Hudrisier D, van Meerwijk JP. Induction of antigen-specific tolerance to bone marrow allografts with CD4+CD25+ T lymphocytes. Blood. (2004) 103:4216–21. doi: 10.1182/blood-2004-01-0005
77. Joffre O, Santolaria T, Calise D, Al Saati T, Hudrisier D, Romagnoli P, et al. Prevention of acute and chronic allograft rejection with CD4+CD25+Foxp3+ regulatory T lymphocytes. Nat Med. (2008) 14:88–92. doi: 10.1038/nm1688
78. Hanash AM, Levy RB. Donor CD4+CD25+ T cells promote engraftment and tolerance following MHC-mismatched hematopoietic cell transplantation. Blood. (2005) 105:1828–36. doi: 10.1182/blood-2004-08-3213
79. Steiner D, Brunicki N, Blazar BR, Bachar-Lustig E, Reisner Y. Tolerance induction by third-party “off-the-shelf” CD4+CD25+ Treg cells. Exp Hematol. (2006) 34:66–71. doi: 10.1016/j.exphem.2005.10.011
80. Pilat N, Baranyi U, Klaus C, Jaeckel E, Mpofu N, Wrba F, et al. Treg-therapy allows mixed chimerism and transplantation tolerance without cytoreductive conditioning. Am J Transplant. (2010) 10:751–62. doi: 10.1111/j.1600-6143.2010.03018.x
81. Battaglia M, Stabilini A, Roncarolo MG. Rapamycin selectively expands CD4+CD25+FoxP3+ regulatory T cells. Blood. (2005) 105:4743–8. doi: 10.1182/blood-2004-10-3932
82. Hongo D, Tang X, Dutt S, Nador RG, Strober S. Interactions between NKT cells and Tregs are required for tolerance to combined bone marrow and organ transplants. Blood. (2012) 119:1581–9. doi: 10.1182/blood-2011-08-371948
83. Müller AM, Poyser J, Küpper NJ, Burnett C, Ko RM, Kohrt HE, et al. Donor hematopoiesis in mice following total lymphoid irradiation requires host T-regulatory cells for durable engraftment. Blood. (2014) 123:2882–92. doi: 10.1182/blood-2013-10-530212
84. Lowsky R, Takahashi T, Liu YP, Dejbakhsh-Jones S, Grumet FC, Shizuru JA, et al. Protective conditioning for acute graft-versus-host disease. N Engl J Med. (2005) 353:1321–31. doi: 10.1056/NEJMoa050642
85. Aversa F, Tabilio A, Velardi A, Cunningham I, Terenzi A, Falzetti F, et al. Treatment of high-risk acute leukemia with T-cell-depleted stem cells from related donors with one fully mismatched HLA haplotype. N Engl J Med. (1998) 339:1186–93. doi: 10.1056/NEJM199810223391702
86. Aversa F, Terenzi A, Tabilio A, Falzetti F, Carotti A, Ballanti S, et al. Full haplotype-mismatched hematopoietic stem-cell transplantation: a phase II study in patients with acute leukemia at high risk of relapse. J Clin Oncol. (2005) 23:3447–54. doi: 10.1200/JCO.2005.09.117
87. Mancusi A, Ruggeri L, Velardi A. Haploidentical hematopoietic transplantation for the cure of leukemia: from its biology to clinical translation. Blood. (2016) 128:2616–23. doi: 10.1182/blood-2016-07-730564
88. Kanakry CG, Fuchs EJ, Luznik L. Modern approaches to HLA-haploidentical blood or marrow transplantation. Nat Rev Clin Oncol. (2016) 13:132. doi: 10.1038/nrclinonc.2015.128
89. O'Donnell PV, Luznik L, Jones RJ, Vogelsang GB, Leffell MS, Phelps M, et al. Nonmyeloablative bone marrow transplantation from partially HLA-mismatched related donors using posttransplantation cyclophosphamide. Biol Blood Marrow Transplant. (2002) 8:377–86. doi: 10.1053/bbmt.2002.v8.pm12171484
90. Luznik L, O'Donnell PV, Symons HJ, Chen AR, Leffell MS, Zahurak M, et al. HLA-haploidentical bone marrow transplantation for hematologic malignancies using nonmyeloablative conditioning and high-dose, posttransplantation cyclophosphamide. Biol Blood Marrow Transplant. (2008) 14:641–50. doi: 10.1016/j.bbmt.2008.03.005
91. Kanakry CG, Ganguly S, Zahurak M, Bolaños-Meade J, Thoburn C, Perkins B, et al. Aldehyde dehydrogenase expression drives human regulatory T cell resistance to posttransplantation cyclophosphamide. Sci Transl Med. (2013) 5:211ra157. doi: 10.1126/scitranslmed.3006960
92. Ganguly S, Ross DB, Panoskaltsis-Mortari A, Kanakry CG, Blazar BR, Levy RB, et al. Donor CD4+ Foxp3+ regulatory T cells are necessary for posttransplantation cyclophosphamide-mediated protection against GVHD in mice. Blood. (2014) 124:2131–41. doi: 10.1182/blood-2013-10-525873
93. Wachsmuth LP, Patterson MT, Eckhaus MA, Venzon DJ, Gress RE, Kanakry CG. Post- transplantation cyclophosphamide prevents graft-versus-host disease by inducing alloreactive T cell dysfunction and suppression. J Clin Invest. (2019) 129:2357–73. doi: 10.1172/JCI124218
94. Russo A, Oliveira G, Berglund S, Greco R, Gambacorta V, Cieri N, et al. NK cell recovery after haploidentical HSCT with posttransplant cyclophosphamide: dynamics and clinical implications. Blood. (2018) 131:247–62. doi: 10.1182/blood-2017-05-780668
95. Roberto A, Di Vito C, Zaghi E, Mazza EMC, Capucetti A, Calvi M, et al. The early expansion of anergic NKG2Apos/CD56dim/CD16neg natural killer represents a therapeutic target in haploidentical hematopoietic stem cell transplantation. Haematologica. (2018) 103:1390–402. doi: 10.3324/haematol.2017.186619
96. Raiola AM, Dominietto A, di Grazia C, Lamparelli T, Gualandi F, Ibatici A, et al. Unmanipulated haploidentical transplants compared with other alternative donors and matched sibling grafts. Biol Blood Marrow Transplant. (2014) 20:1573–9. doi: 10.1016/j.bbmt.2014.05.029
97. Di Stasi A, Milton DR, Poon LM, Hamdi A, Rondon G, Chen J, et al. Similar transplantation outcomes for acute myeloid leukemia and myelodysplastic syndrome patients with haploidentical versus 10/10 human leukocyte antigen-matched unrelated and related donors. Biol Blood Marrow Transplant. (2014) 20:1975–81. doi: 10.1016/j.bbmt.2014.08.013
98. Ciurea SO, Zhang MJ, Bacigalupo AA, Bashey A, Appelbaum FR, Aljitawi OS, et al. Haploidentical transplant with posttransplant cyclophosphamide vs. matched unrelated donor transplant for acute myeloid leukemia. Blood. (2015) 126:1033–40. doi: 10.1182/blood-2015-04-639831
99. Kanate AS, Mussetti A, Kharfan-Dabaja MA, Ahn KW, DiGilio A, Beitinjaneh A, et al. Reduced-intensity transplantation for lymphomas using haploidentical related donors vs. HLA- matched unrelated donors. Blood. (2016) 127:938–47. doi: 10.1182/blood-2015-09-671834
100. Ghosh N, Karmali R, Rocha V, Ahn KW, DiGilio A, Hari PN, et al. Reduced-intensity transplantation for lymphomas using haploidentical related donors versus HLA-matched sibling donors: a center for international blood and marrow transplant research analysis. J Clin Oncol. (2016) 34:3141–9. doi: 10.1200/JCO.2015.66.3476
101. Bashey A, Zhang X, Jackson K, Brown S, Ridgeway M, Solh M, et al. Comparison of outcomes of hematopoietic cell transplants from T-replete haploidentical donors using post-transplantation cyclophosphamide with 10 of 10 HLA-A, -B, -C, -DRB1, and -DQB1 allele-matched unrelated donors and HLA-identical sibling donors: a multivariable analysis including disease risk index. Biol Blood Marrow Transplant. (2016) 22:125–33. doi: 10.1016/j.bbmt.2015.09.002
102. Dreger P, Sureda A, Ahn KW, Eapen M, Litovich C, Finel H, et al. PTCy-based haploidentical vs. matched related or unrelated donor reduced-intensity conditioning transplant for DLBCL. Blood Adv. (2019) 3:360–9. doi: 10.1182/bloodadvances.2018027748
103. Peccatori J, Forcina A, Clerici D, Crocchiolo R, Vago L, Stanghellini MT, et al. Sirolimus-based graft-versus-host disease prophylaxis promotes the in vivo expansion of regulatory T cells and permits peripheral blood stem cell transplantation from haploidentical donors. Leukemia. (2015) 29:396–405. doi: 10.1038/leu.2014.180
104. Di Ianni M, Falzetti F, Carotti A, Terenzi A, Castellino F, Bonifacio E, et al. Tregs prevent GVHD and promote immune reconstitution in HLA-haploidentical transplantation. Blood. (2011) 117:3921–8. doi: 10.1182/blood-2010-10-311894
105. Martelli MF, Di Ianni M, Ruggeri L, Pierini A, Falzetti F, Carotti A, et al. “Designed” grafts for HLA-haploidentical stem cell transplantation. Blood. (2014) 123:967–73. doi: 10.1182/blood-2013-10-531764
106. Rosenthal J, Wong J, Stein A, Qian D, Hitt D, Naeem H, et al. Phase 1/2 trial of total marrow and lymph node irradiation to augment reduced-intensity transplantation for advanced hematologic malignancies. Blood. (2011) 117:309–15. doi: 10.1182/blood-2010-06-288357
107. Pierini A, Ruggeri L, Carotti A, Falzetti F, Piccinelli S, Saldi S, et al. The “ultimate” haploidentical transplantation for the elderly with high-risk acute myeloid leukemia. Bone Marrow Transplant. (2019) 54(Suppl. 2):803–5. doi: 10.1038/s41409-019-0618-x
108. MacDonald KN, Piret JM, Levings MK. Methods to manufacture regulatory T cells for cell therapy. Clin Exp Immunol. (2019) 197:52–63. doi: 10.1111/cei.13297
109. Trzonkowski P, Bieniaszewska M, Juścinska J, Dobyszuk A, Krzystyniak A, Marek N, et al. First-in-man clinical results of the treatment of patients with graft versus host disease with human ex vivo expanded CD4+CD25+CD127- T regulatory cells. Clin Immunol. (2009) 133:22–6. doi: 10.1016/j.clim.2009.06.001
110. Brunstein CG, Miller JS, Cao Q, McKenna DH, Hippen KL, Curtsinger J, et al. Infusion of ex vivo expanded T regulatory cells in adults transplanted with umbilical cord blood: safety profile and detection kinetics. Blood. (2011) 117:1061–70. doi: 10.1182/blood-2010-07-293795
111. Theil A, Tuve S, Oelschlägel U, Maiwald A, Döhler D, Oßmann D, et al. Adoptive transfer of allogeneic regulatory T cells into patients with chronic graft-versus-host disease. Cytotherapy. (2015) 17:473–86. doi: 10.1016/j.jcyt.2014.11.005
112. Del Papa B, Ruggeri L, Urbani E, Baldoni S, Cecchini D, Zei T, et al. Clinical-grade-expanded regulatory T cells prevent graft-versus-host disease while allowing a powerful T cell-dependent graft-versus-leukemia effect in murine models. Biol Blood Marrow Transplant. (2017) 23:1847–51. doi: 10.1016/j.bbmt.2017.07.009
113. Hippen KL, Merkel SC, Schirm DK, Sieben CM, Sumstad D, Kadidlo DM, et al. Massive ex vivo expansion of human natural regulatory T cells (T(regs)) with minimal loss of in vivo functional activity. Sci Transl Med. (2011) 3:83ra41. doi: 10.1126/scitranslmed.3001809
114. Brunstein CG, Miller JS, McKenna DH, Hippen KL, DeFor TE, Sumstad D, et al. Umbilical cord blood-derived T regulatory cells to prevent GVHD: kinetics, toxicity profile, and clinical effect. Blood. (2016) 127:1044–51. doi: 10.1182/blood-2015-06-653667
115. McKenna DH Jr, Sumstad D, Kadidlo DM, Batdorf B, Lord CJ, Merkel SC, et al. Optimization of cGMP purification and expansion of umbilical cord blood-derived T-regulatory cells in support of first-in-human clinical trials. Cytotherapy. (2017) 19:250–62. doi: 10.1016/j.jcyt.2016.10.011
116. Meyer EH, Laport G, Xie BJ, MacDonald K, Heydari K, Sahaf B, et al. Transplantation of donor grafts with defined ratio of conventional and regulatory T cells in HLA-matched recipients. JCI Insight. (2019) 4:127244. doi: 10.1172/jci.insight.127244
117. Florek M, Schneidawind D, Pierini A, Baker J, Armstrong R, Pan Y, et al. Freeze and thaw of CD4+CD25+Foxp3+ regulatory T cells results in loss of CD62L expression and a reduced capacity to protect against graft-versus-host disease. PLoS ONE. (2015) 10:e0145763. doi: 10.1371/journal.pone.0145763
118. Saadoun D, Rosenzwajg M, Joly F, Six A, Carrat F, Thibault V, et al. Regulatory T-cell responses to low-dose interleukin-2 in HCV-induced vasculitis. N Engl J Med. (2011) 365:2067–77. doi: 10.1056/NEJMoa1105143
119. Klatzmann D, Abbas AK. The promise of low-dose interleukin-2 therapy for autoimmune and inflammatory diseases. Nat Rev Immunol. (2015) 15:283–94. doi: 10.1038/nri3823
120. Koreth J, Matsuoka K, Kim HT, McDonough SM, Bindra B, Alyea EP III, et al. Interleukin-2 and regulatory T cells in graft-versus-host disease. N Engl J Med. (2011) 365:2055–66. doi: 10.1056/NEJMoa1108188
121. Koreth J, Kim HT, Jones KT, Lange PB, Reynolds CG, Chammas MJ, et al. Efficacy, durability, and response predictors of low-dose interleukin-2 therapy for chronic graft-versus-host disease. Blood. (2016) 128:130–7. doi: 10.1182/blood-2016-02-702852
122. Matsuoka K, Koreth J, Kim HT, Bascug G, McDonough S, Kawano Y, et al. Low-dose interleukin-2 therapy restores regulatory T cell homeostasis in patients with chronic graft-versus-host disease. Sci Transl Med. (2013) 5:179ra43. doi: 10.1126/scitranslmed.3005265
123. Cutler CS, Koreth J, Ritz J. Mechanistic approaches for the prevention and treatment of chronic GVHD. Blood. (2017) 129:22–9. doi: 10.1182/blood-2016-08-686659
124. Belizaire R, Kim HT, Poryanda SJ, Mirkovic NV, Hipolito E, Savage WJ, et al. Efficacy and immunologic effects of extracorporeal photopheresis plus interleukin-2 in chronic graft-versus-host disease. Blood Adv. (2019) 3:969–79. doi: 10.1182/bloodadvances.2018029124
125. Kennedy-Nasser AA, Ku S, Castillo-Caro P, Hazrat Y, Wu MF, Liu H, et al. Ultra low-dose IL-2 for GVHD prophylaxis after allogeneic hematopoietic stem cell transplantation mediates expansion of regulatory T cells without diminishing antiviral and antileukemic activity. Clin Cancer Res. (2014) 20:2215–25. doi: 10.1158/1078-0432.CCR-13-3205
126. Abbas AK, Trotta E, Simeonov DR, Marson A, Bluestone JA. Revisiting IL-2: biology and therapeutic prospects. Sci Immunol. (2018) 3:eaat1482. doi: 10.1126/sciimmunol.aat1482
127. Boyman O, Kovar M, Rubinstein MP, Surh CD, Sprent J. Selective stimulation of T cell subsets with antibody-cytokine immune complexes. Science. (2006) 311:1924–7. doi: 10.1126/science.1122927
128. Trotta E, Bessette PH, Silveria SL, Ely LK, Jude KM, Le DT, et al. A human anti-IL-2 antibody that potentiates regulatory T cells by a structure-based mechanism. Nat Med. (2018) 24:1005–14. doi: 10.1038/s41591-018-0070-2
129. Spangler JB, Trotta E, Tomala J, Peck A, Young TA, Savvides CS, et al. Engineering a single-agent cytokine/antibody fusion that selectively expands regulatory T cells for autoimmune disease therapy. J Immunol. (2018) 201:2094–106. doi: 10.4049/jimmunol.1800578
130. Ward NC, Yu A, Moro A, Ban Y, Chen X, Hsiung S, et al. Review IL-2/CD25: a long-acting fusion protein that promotes immune tolerance by selectively targeting the IL-2 receptor on regulatory T cells. J Immunol. (2018) 201:2579–92. doi: 10.4049/jimmunol.1800907
131. Tchao N, Gorski KS, Yuraszeck T, Sohn SJ, Ishida K, Wong H, et al. Amg 592 is an investigational IL-2 mutein that induces highly selective expansion of regulatory T cells. Blood. (2017) 130 (Suppl. 1): 696. doi: 10.1182/blood.V126.23.919.919
132. Peterson LB, Bell CJM, Howlett SK, Pekalski ML, Brady K, Hinton H, et al. A long-lived IL-2 mutein that selectively activates and expands regulatory T cells as a therapy for autoimmune disease. J Autoimmun. (2018) 95:1–14. doi: 10.1016/j.jaut.2018.10.017
133. Ferrara JL, Levine JE, Reddy P, Holler E. Graft-versus-host disease. Lancet. (2009) 373:1550–61. doi: 10.1016/S0140-6736(09)60237-3
134. Levine JE. Implications of TNF-α in the pathogenesis and management of GVHD. Int J Hematol. (2011) 93:571–7. doi: 10.1007/s12185-011-0803-1
135. Yalniz FF, Hefazi M, McCullough K, Litzow MR, Hogan WJ, Wolf R, et al. Safety and efficacy of infliximab therapy in the setting of steroid-refractory acute graft-versus-host disease. Biol Blood Marrow Transplant. (2017) 23:1478–84. doi: 10.1016/j.bbmt.2017.05.001
136. Patriarca F, Sperotto A, Damiani D, Morreale G, Bonifazi F, Olivieri A, et al. Infliximab treatment for steroid-refractory acute graft-versus-host disease. Haematologica. (2004) 11:1352–9.
137. Chen X, Bäumel M, Männel DN, Howard OM, Oppenheim JJ. Interaction of TNF with TNF receptor type 2 promotes expansion and function of mouse CD4+CD25+ T regulatory cells. J Immunol. (2007) 179:154–61. doi: 10.4049/jimmunol.179.1.154
138. Chen X, Subleski JJ, Kopf H, Howard OM, Männel DN, Oppenheim JJ. Cutting edge: expression of TNFR2 defines a maximally suppressive subset of mouse CD4+CD25+FoxP3+ T regulatory cells: applicability to tumor-infiltrating T regulatory cells. J Immunol. (2008) 180:6467–71. doi: 10.4049/jimmunol.180.10.6467
139. Chen X, Subleski JJ, Hamano R, Howard OM, Wiltrout RH, Oppenheim JJ. Co-expression of TNFR2 and CD25 identifies more of the functional CD4+FOXP3+ regulatory T cells in human peripheral blood. Eur J Immunol. (2010) 40:1099–106. doi: 10.1002/eji.200940022
140. Okubo Y, Mera T, Wang L, Faustman DL. Homogeneous expansion of human T-regulatory cells via tumor necrosis factor receptor 2. Sci Rep. (2013) 3:3153. doi: 10.1038/srep03153
141. Peschon JJ, Torrance DS, Stocking KL, Glaccum MB, Otten C, Willis CR, et al. TNF receptor-deficient mice reveal divergent roles for p55 and p75 in several models of inflammation. J Immunol. (1998) 160:943–52.
142. Mahmud SA, Manlove LS, Schmitz HM, Xing Y, Wang Y, Owen DL, et al. Costimulation via the tumor-necrosis factor receptor superfamily couples TCR signal strength to the thymic differentiation of regulatory T cells. Nat Immunol. (2014) 15:473–81. doi: 10.1038/ni.2849
143. Leclerc M, Naserian S, Pilon C, Thiolat A, Martin GH, Pouchy C, et al. Control of GVHD by regulatory T cells depends on TNF produced by T cells and TNFR2 expressed by regulatory T cells. Blood. (2016) 128:1651–9. doi: 10.1182/blood-2016-02-700849
144. Pierini A, Strober W, Moffett C, Baker J, Nishikii H, Alvarez M, et al. TNF-α priming enhances CD4+FoxP3+ regulatory T-cell suppressive function in murine GVHD prevention and treatment. Blood. (2016) 128:866–71. doi: 10.1182/blood-2016-04-711275
145. Chopra M, Biehl M, Steinfatt T, Brandl A, Kums J, Amich J, et al. Exogenous TNFR2 activation protects from acute GvHD via host T reg cell expansion. J Exp Med. (2016) 213:1881–900. doi: 10.1084/jem.20151563
146. Mancusi A, Piccinelli S, Velardi A, Pierini A. The Effect of TNF-α on regulatory T cell function in graft-versus-host disease. Front Immunol. (2018) 9:356. doi: 10.3389/fimmu.2018.00356
147. Mancusi A, Alvarez M, Piccinelli S, Velardi A, Pierini A. TNFR2 signaling modulates immunity after allogeneic hematopoietic cell transplantation. Cytokine Growth Factor Rev. (2019) 47:54–61. doi: 10.1016/j.cytogfr.2019.05.001
148. Chen X, Hamano R, Jubleski JJ, Hurwitz AA, Howard OM, Oppenheim JJ. Expression of costimulatory TNFR2 induces resistance of CD4+FoxP3- conventional T cells to suppression by CD4+FoxP3+ regulatory T cells. J Immunol. (2010) 185:174–82. doi: 10.4049/jimmunol.0903548
149. Schreiber TH, Wolf D, Bodero M, Gonzalez L, Podack ER. T cell costimulation by TNFR superfamily (TNFRSF)4 and TNFRSF25 in the context of vaccination. J Immunol. (2012) 189:3311–8. doi: 10.4049/jimmunol.1200597
150. Khan SQ, Tsai MS, Schreiber TH, Wolf D, Deyev VV, Podack ER. Cloning, expression, and functional characterization of TL1A-Ig. J Immunol. (2013) 190:1540–50. doi: 10.4049/jimmunol.1201908
151. Kim BS, Nishikii H, Baker J, Pierini A, Schneidawind D, Pan Y, et al. Treatment with agonistic DR3 antibody results in expansion of donor Tregs and reduced graft-versus-host disease. Blood. (2015) 126:546–57. doi: 10.1182/blood-2015-04-637587
152. Nishikii H, Kim BS, Yokoyama Y, Chen Y, Baker J, Pierini A, et al. DR3 signaling modulates the function of Foxp3+ regulatory T cells and the severity of acute graft-versus-host disease. Blood. (2016) 128:2846–58. doi: 10.1182/blood-2016-06-723783
153. Wolf D, Barreras H, Bader CS, Copsel S, Lightbourn CO, Pfeiffer BJ, et al. Marked in vivo donor regulatory T cell expansion via interleukin-2 and TL1A-Ig stimulation ameliorates graft-versus-host disease but preserves graft-versus-leukemia in recipients after hematopoietic stem cell transplantation. Biol Blood Marrow Transplant. (2017) 23:757–66. doi: 10.1016/j.bbmt.2017.02.013
154. Copsel S, Wolf D, Kale B, Barreras H, Lightbourn CO, Bader CS, et al. Very low numbers of CD4+ FoxP3+ tregs expanded in donors via TL1A-Ig and low-dose IL-2 exhibit a distinct activation/functional profile and suppress GVHD in a preclinical model. Biol Blood Marrow Transplant. (2018) 24:1788–94. doi: 10.1016/j.bbmt.2018.04.026
155. Mavers M, Simonetta F, Nishikii H, Ribado JV, Maas-Bauer K, Alvarez M, et al. Activation of the DR3-TL1A axis in donor mice leads to regulatory T cell expansion and activation with reduction in graft-versus-host disease. Front Immunol. (2019) 10:1624. doi: 10.3389/fimmu.2019.01624
156. Copsel SN, Lightbourn CO, Barreras H, Lohse I, Wolf D, Bader CS, et al. BET Bromodomain inhibitors which permit Treg function enable a combinatorial strategy to suppress GVHD in pre-clinical allogeneic HSCT. Front Immunol. (2019) 9:3104. doi: 10.3389/fimmu.2018.03104
157. Dawson NAJ, Vent-Schmidt J, Levings MK. Engineered tolerance: tailoring development, function, and antigen-specificity of regulatory T cells. Front Immunol. (2017) 8:1460. doi: 10.3389/fimmu.2017.01460
158. MacDonald KG, Hoeppli RE, Huang Q, Gillies J, Luciani DS, Orban PC, et al. Alloantigen-specific regulatory T cells generated with a chimeric antigen receptor. J Clin Invest. (2016) 126:1413–24. doi: 10.1172/JCI82771
159. Noyan F, Zimmermann K, Hardtke-Wolenski M, Knoefel A, Schulde E, Geffers R, et al. Prevention of allograft rejection by use of regulatory T cells with an MHC-specific chimeric antigen receptor. Am J Transplant. (2017) 17:917–30. doi: 10.1111/ajt.14175
160. Pierini A, Iliopoulou BP, Peiris H, Pérez-Cruz M, Baker J, Hsu K, et al. T cells expressing chimeric antigen receptor promote immune tolerance. JCI Insight. (2017) 2:92865. doi: 10.1172/jci.insight.92865
Keywords: regulatory T cells, allogeneic hematopoietic transplantation, tolerance, engraftment, graft-vs.-host-disease, graft-vs.-tumor effect
Citation: Mancusi A, Piccinelli S, Velardi A and Pierini A (2019) CD4+FOXP3+ Regulatory T Cell Therapies in HLA Haploidentical Hematopoietic Transplantation. Front. Immunol. 10:2901. doi: 10.3389/fimmu.2019.02901
Received: 23 August 2019; Accepted: 26 November 2019;
Published: 17 December 2019.
Edited by:
Luca Vago, San Raffaele Scientific Institute (IRCCS), ItalyReviewed by:
Rosa Bacchetta, Stanford University, United StatesJohn Koreth, Dana-Farber Cancer Institute, United States
Copyright © 2019 Mancusi, Piccinelli, Velardi and Pierini. This is an open-access article distributed under the terms of the Creative Commons Attribution License (CC BY). The use, distribution or reproduction in other forums is permitted, provided the original author(s) and the copyright owner(s) are credited and that the original publication in this journal is cited, in accordance with accepted academic practice. No use, distribution or reproduction is permitted which does not comply with these terms.
*Correspondence: Antonio Pierini, YW50b25pby5waWVyaW5pJiN4MDAwNDA7dW5pcGcuaXQ=