- Experimental Obstetrics and Gynecology, Medical Faculty, Otto-von-Guericke University Magdeburg, Magdeburg, Germany
Human chorionic gonadotropin (hCG) serves as one of the first signals provided by the embryo to the mother. Exactly at the time when the first step of the implantation process is initiated and the blastocyst adheres to the maternal endometrium, the embryonic tissue starts to actively secrete hCG. Shortly thereafter, the hormone can be detected in the maternal circulation where its concentration steadily increases throughout early pregnancy as it is continuously released by the forming placenta. Accumulating evidence underlines the critical function of hCG for embryo implantation and placentation. hCG not only regulates biological aspects of these early pregnancy events but also supports maternal immune cells in their function as helpers in the establishment of an adequate embryo-endometrial relationship. In view of its early presence in the maternal circulation, hCG has the potential to influence both local uterine immune cell populations as well as peripheral ones. The current review aims to summarize recent literature on the participation of innate and adaptive immune cells in embryo implantation and placentation with a specific focus on their regulation by hCG.
Introduction
Within a few days, after fertilization of the oocyte took place in the fallopian tube, the early embryo in its morula stage enters the uterine cavity. Here, after becoming a blastocyst, the embryo starts to implant into the maternal endometrium (Figure 1). The implantation process is initiated by an apposition reaction followed by the adhesion of the trophoblast cells of the blastocyst to the epithelial layer of the endometrium. Subsequently, trophoblast cells begin to proliferate, differentiate, cross the epithelial basement membrane and invade into the endometrial stroma to form the placenta (1). Uterine spiral arteries (uSA) located within the stroma are targeted by invasive trophoblast cells that replace the endothelial cell layer and provoke alterations in extracellular matrix proteins. Consequently, maternal SA are remodeled from high-resistance into low-resistance vessels with the aim to guarantee a high blood flow from the mother to the fetus by the mid-second trimester and ascertain the fetus to be supplied with sufficient nutrients for an adequate fetal development (2). Impairments in placentation due to shallow trophoblast invasion is associated with late-onset adverse pregnancy outcomes such as intrauterine growth restriction (IUGR) and early-onset preeclampsia (PE) (3) (Figure 1). Moreover, on the maternal side, endometrial stromal cells differentiate into a specialized cell type called decidua cells, via a process called decidualization (4). This structural and functional remodeling of the uterine bed is strongly mediated by the steroid hormones progesterone (P4) and estrogen (E2) (5). In addition, a variety of growth factors, cytokines, prostaglandins, matrix degrading enzymes and their inhibitors as well as adhesion molecules orchestrate the fetal-maternal dialogue and ensure a timely well-defined progression of the highly complex implantation process (6). However, embryo implantation and placentation do not only depend on the availability of individual molecules but also rely on the presence of distinct immune cell populations. These immune cell populations reside in the decidual tissue and are often highly specialized compared to their peripheral counterparts. Unique phenotypic and functional features allow them not only to tolerate the foreign paternal antigens expressed by the fetal tissue but also to actively participate in the different steps of the implantation procedure. Immunological dysregulations are often made responsible for cases of idiopathic infertility and miscarriage underlying the meaning of the maternal immune system for healthy pregnancy progression.
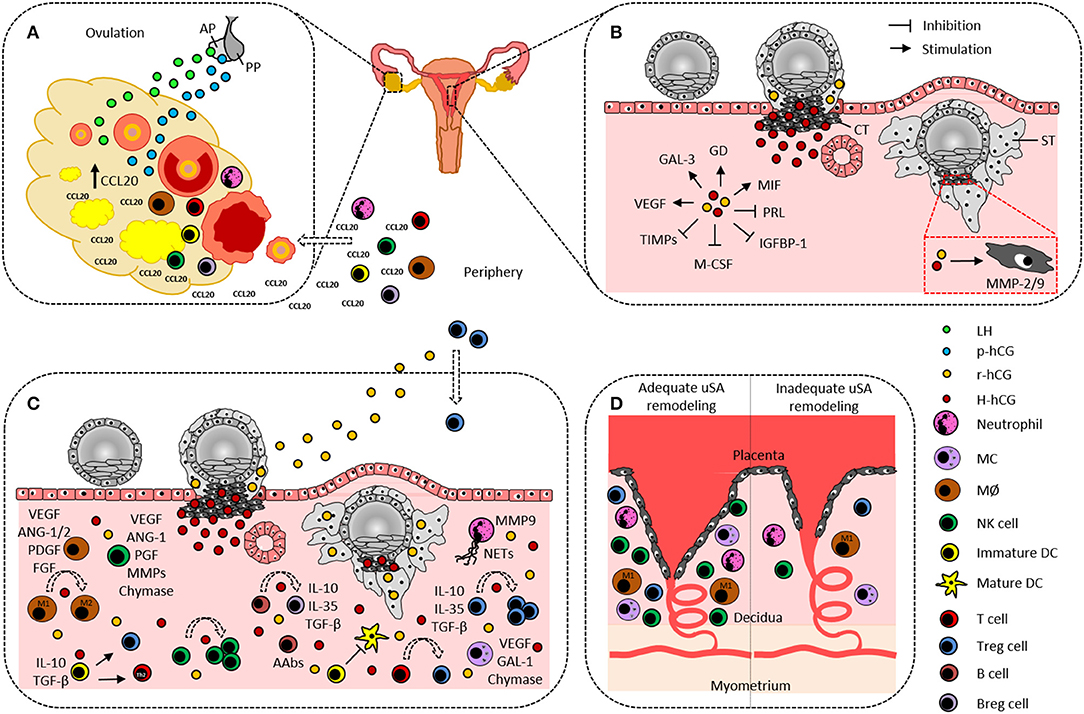
Figure 1. Hypothetical scenario on the participation of human chorionic gonadotropin and immune cells in ovulation and embryo implantation. (A) Pituitary gland-produced LH and p-hCG induce ovarian CCL20 secretion that in turn promotes leukocyte influx from the periphery into the ovary. Ovarian DCs and MØ are suggested to actively contribute to the ovulation process. (B) Cytotrophoblast-derived H-hCG and syncytiotrophoblast-derived r-hCG promote angiogenesis, trophoblast invasion, and tissue-remodeling by inducing endometrial expression of GAL-3, GD, MIF, and VEGF as well as trophoblastic expression of MMP-2 and−9, and by inhibiting endometrial expression of PRL, IGFBP-1, M-CSF, and TIMPs. (C) Decidual innate and adaptive immune cells support embryo implantation by producing and secreting a variety of factors that are indicated aside each immune cell type and are partially induced by hCG. Additionally, hCG confers fetal tolerance by enhancing uterine NK cells, M2 cells, tolerogenic DCs, Th2 cells, Treg, and Breg, by promoting NETs formation by neutrophils, and by inducing fetal-protective AAbs in B cells. (D) Neutrophils, MØ, MCs, NK cells, and Treg cells are proposed to support uSA remodeling and fetal nourishment. A lack or dysfunctionality of these immune cells may result in improper uSA remodeling followed by fetal undernourishment and fetal growth restriction. AAbs, asymmetric antibodies; ANG-1/2, angiopoietin-1/2; AP, anterior pituitary gland; Breg, regulatory B cell; CCL20, CC-chemokine ligand 20; CT, cytotrophoblast; DC, dendritic cell; PDGF, platelet derived growth factor; FGF, fibroblast growth factor; GAL-1/3, galectin-1/3; GD, glycodelin; IGFBP-1, insulin-like growth factor binding protein-1; H-hCG, hyperglycosylated human chorionic gonadotropin; IL, interleukin; LH, luteinizing hormone; MØ, macrophage; MC, mast cell; M-CSF, macrophage colony stimulating factor; MIF, migration inhibitory factor; MMP-2/9, matrix metalloproteinase-2/9; NETs, neutrophil extracellular traps; NK cell, natural killer cell; p-hCG, pituitary human chorionic gonadotropin; PGF, placental growth factor; PP, posterior pituitary gland; PRL, prolactin; r-hCG, regular human chorionic gonadotropin; ST, syncytiotrophoblast; TGF-β, transforming growth factor-β; TIMPs, tissue inhibitors of matrix metalloproteinases; Treg, regulatory T cell; uSA, uterine spiral artery; VEGF, vascular endothelial growth factor.
This review will discuss the state-of-the art for the involvement of innate and adaptive immune cell populations in embryo implantation and placentation and will particularly emphasize the role of the hormone human chorionic gonadotropin (hCG) as immune-modulating factor in these processes.
hCG in Embryo Implantation and Placentation
hCG represents one of the first molecular messages send out by the pre-implanting embryo to modulate the implantation site and to ensure a timely initiation of the nidation process. Despite CGB gene expression was proven already in the 8-cell stage embryo (7), active secretion of the hormone starts at the blastocyst stage (8) and enables hCG detection in the maternal circulation 10 days after fertilization. Later on, hCG is produced in high amounts by trophoblast cells (9) resulting in the highest hCG values between the 10th and 11th week of pregnancy. By the end of the first trimester, hCG levels decrease but remain elevated compared to non-pregnant individuals. Notably, a drop of hCG seems to be required for normal pregnancy progression. A recent meta-analysis provided evidence that elevated hCG levels can be detected already at the end of the first trimester in women developing preterm PE (10) and hCG was suggested as a useful predictor for the development and severity of PE (11, 12).
Five different hCG isoforms have been described so far: regular hCG (r-hCG), free-β hCG (hCGβ), hyperglycosylated hCG (H-hCG), hyperglycosylated free-β hCG (H-hCGβ), and pituitary hCG (p-hCG) (13), all of them with distinct biological functions.
r-hCG, produced by syncytiotrophoblast cells is best known for its function to rescue the corpus luteum and to maintain P4 production during early pregnancy (14). However, although often neglected, r-hCG has a broader influence on fetal and maternal pathways allowing proper implantation and placentation. This includes the fusion of cytotrophoblast cells into the multinuclear structure of the syncytiotrophoblast (15), the formation of the umbilical circulation in villous tissue and the formation of the umbilical cord (16, 17), the growth of fetal organs (18), the contribution to angiogenesis by forcing the development and growth of uSA (19–21) and the suppression of myometrial contractions (22). Thereby, hCG targets several molecules that are involved in decidualization, implantation, vascularization and tissue remodeling such as prolactin, insulin-like growth factor binding protein-1, macrophage colony stimulating factor, leukemia inhibitory factor (LIF), vascular endothelial growth factor (VEGF), matrix metalloproteinase (MMP)-9, tissue inhibitors of MMPs (TIMPs), galectin-3, and glycodelin (23–26) (Figure 1B).
H-hCG is produced by cytotrophoblast cells and is the most abundant hCG isoform around implantation (27). Its major function is to induce proliferation and invasion of cytotrophoblast cells and it has been reported that H-hCG proportions higher than 50% of total hCG are required for successful embryo implantation (28) (Figure 1B). Whereas, tissue growth factors and collagenases positively modulate H-hCG expression, endothelin-1 and prostaglandin F2α are negative modulators of H-hCG expression (29).
High hCGβ and H-hCGβ levels are also indicative for highly invasive processes as both hCG isoforms support tumor cell growth and survival and their presence is associated with poor prognosis for the patients (30). Finally, p-hCG in collaboration with the luteinizing hormone (LH) promotes ovulation and corpus luteum formation during the menstrual cycle (31).
Clinical Application of hCG in Artificial Reproductive Techniques (ART)—Advantage or Disadvantage?
An increasing number of unintentionally childless couples is seeking help in medical reproduction centers to fulfill their wish of having a child of their own. After several in vitro fertilization (IVF)/intracytoplasmic sperm injection (ICSI) cycles using the common clinical protocols after which the patients failed to become or stay pregnant, the demand for unconventional treatment options increases. However, for most of these treatment options there is still no clear evidence for an overall higher success rate or only specific patient groups benefit from these interventions (32). Thus, personalized medicine and the development of new treatment strategies for infertile and miscarriage patients are strongly desired and hCG may represent a promising target in this regard.
hCG is usually applied in two different preparations, either as urine-derived preparation (uhCG) or as recombinant preparation (rhCG) in gonadotropin-releasing hormone agonist or antagonist protocols (33). As a standard procedure, hCG is applied after ovarian stimulation to induce final oocyte maturation. Additionally, some patients receive an intrauterine hCG injection prior to embryo transfer with the aim to improve implantation rates (IR) and live birth rates (LBR). In the majority of recently published studies, uhCG or rhCG is injected into the uterine cavity using an insemination catheter after the cervical mucus is wiped out with a cotton swab or a syringe (34–36). Some study designs also include a flushing step with saline prior to hCG infusion (37). hCG is infused in different doses of 500 up to 1,000 IU solved in either medium or saline and application time points differ between <5 min and days before embryo transfer (38).
As clearly pointed out by Makrigiannakis et al. in their review article in 2017, the efficacy of hCG via the intrauterine hCG application route is controversially discussed (39). Since 2011, several meta-analyses reported different outcomes with regard to IR, clinical pregnancy rates (CPR), ongoing-pregnancy rates (OPR), miscarriage rates (MR), and LBR. The heterogeneity among the different meta-analyses might be attributed to the timing of intrauterine hCG administration meaning the exact time-point of hCG injection prior to embryo transfer as well as the time-point when the embryo is transferred (early cleavage stage or blastocyst stage), the choice of the hCG preparation and the hCG dosage applied. Supportive evidence for improved clinical parameters after intrauterine hCG administration arose from a meta-analysis published by Ye et al. (40) and a potential explanation for the clinical benefit is provided by Strug et al. (41). The latter suggested that hCG counteracts endometrial dyssynchrony resulting from ovarian stimulation and promotes expression of markers essential for the survival of the endometrial stroma (41). On the contrary, Osman et al. (42) and Hou et al. (43) found no beneficial effects on all clinical parameters or showed improvements on only some of them. Notably, Volovsky et al. (44) even demonstrated negative effects on CPR after intrauterine hCG application and this was particularly true for patients without defined repeated implantation failure (RIF).
In a recent Cochrane review from Craciunas et al. (45), it is proposed that in particular patients undergoing cleavage-stage embryo transfer receiving an hCG dosage above 500 IU benefit from intrauterine hCG injection but not patients undergoing blastocyst transfer. The last meta-analysis from 2019 concluded that intrauterine hCG administration before embryo transfer could significantly improve IR, CPR, OPR, and LBR and significantly lowered the MR. These authors suggested that patients treated with 500 IU hCG within 15 min prior to embryo transfer can achieve optimal outcomes (46). Moreover, a recent study by Huang et al. (37) implied that the number of previous implantation failures may influence the efficacy of intrauterine hCG treatment.
Altogether, it can be stated that an overall beneficial effect of intrauterine hCG injection before embryo transfer is not definitely proven and more research has to be done in this field.
Beneficial Role of hCG in Pregnancy and in ART—Is the Immune System Involved?
There is no doubt that hCG possesses a variety of immune-modulating properties (47, 48). However, how hCG supports immune cells in their function in controlling embryo implantation and placentation is far from being understood. The participation of immune cells in pregnancy-related processes is not restricted to pregnancy itself but begins already during the menstrual cycle before ovulation takes place. Upon the LH surge, an inflammatory reaction is induced where leukocytes are actively recruited to the ovaries through a mechanism involving the leukocyte chemoattractant CCL20. Among these leukocytes are neutrophils, monocytes, natural killer (NK) cells, dendritic cells (DCs), B cells, and T cells and for some of them, a critical role in the ovulation process has been proven (49, 50). Notably, hCG also augments CCL20 expression in the ovaries and thereby stimulates a CCL20-driven leukocyte influx (51). Most likely, this function is attributed to the p-hCG isoform (Figure 1A). Moreover, p-hCG may induce the expression of the macrophage migration inhibitory factor (MIF) in human endometrial stromal cells during the menstrual cycle (Figure 1B). MIF is known to modulate immune responses on his part and to act as a growth and angiogenic factor (52), features that are highly relevant to support remodeling of the endometrial bed.
Later on, during the pre-implantation period local immune cell populations seem to take over critical functions in preparing the implantation site. Approaches where RIF patients received autologous peripheral blood mononuclear cells (PBMCs) before embryo transfer resulted in significantly increased IR and CPR (53, 54). PBMCs were isolated from peripheral blood by using density-gradient centrifugation either on the day of ovulation in fresh embryo transfer cycles or 5 days prior scheduled frozen-thawed embryo transfer cycles. PBMCs were then cultured and monitored for up to 3 days as quality of PBMC cultures was shown to be predictive for the efficiency of PBMC transfers. In each case, 1 × 106 or 4 × 107 PBMCs were transferred into the uterine cavity 2 days before embryo transfer (53, 54).
Markedly, PBMCs that have been activated by hCG prior to their transfer into RIF patients also significantly improved IR, CPR, and LBR irrespective whether the patients underwent fresh or frozen/thawed embryo transfer or whether early cleavage stage embryos or blastocysts were transferred (55–57). Moreover, it became evident that patients with more than three implantation failures may be the group that particularly benefits from this immunotherapy. Several studies were conducted to estimate the underlying mechanisms of an implantation-regulation by hCG-activated PBMCs. One study involved pure mouse material and found that adoptive transfer of PBMCs from non-pregnant mice into mice suffering from an implantation dysfunction elevated the pregnancy rate and increased the endometrial expression of VEGF and LIF during the implantation window (58). Another study combined human PBMCs and mouse embryos. The authors showed that PBMCs from early pregnant women enhanced spreading and invasion of mouse embryos to a greater extent than PBMCs from non-pregnant women. Interestingly, when PBMCs from non-pregnant women were previously exposed to hCG, they possessed a higher capability to promote embryo outgrowth compared to untreated PBMCs (59). Additionally, two studies focusing on human sample material depicted that PBMCs obtained from early pregnant women increased invasion of human trophoblast cells in vitro, while PBMCs from non-pregnant women did not. After PBMCs from non-pregnant women were treated with hCG they showed comparable effects on human trophoblast cells as PBMCs from pregnant women (60, 61) suggesting that previous exposure to hCG is required for PBMC-driven trophoblast invasion. Yu et al. (61) further indicated that hCG-activated PBMCs significantly augmented MMP-2, MMP-9, and VEGF and decreased TIMP-1 and TIMP-2 expression in human trophoblast cells.
These findings let assume that hCG administration to patients undergoing ART will support pregnancy in two steps that involve the maternal immune system. First, hCG injection will provoke an active immigration of immune cells into reproductive tissues and thereby direct those cells to the place of action. Second, hCG will activate immigrated and residual immune cell populations in the pre-implantation phase to support these cells in promoting embryo attachment and invasion as well as in forcing the decidualization process.
hCG-Mediated Immune Regulation Favoring Embryo Implantation and Placentation
Innate and adaptive immune cell types play a pivotal role in the early and late steps of embryo implantation as well as in placentation. hCG is recognized as a key factor in this immune-mediated regulation. However, it remains to be elucidated which specific immune cell populations are targeted by hCG and how they are regulated.
Neutrophils
Neutrophils are in the first line of innate defense against pathogens to protect the mother and her unborn child. Their functional repertoire includes: phagocytosis, production of granules with potent proteolytic activity and of microbicidal peptides, synthesis of reactive oxygen species, formation of neutrophil extracellular traps (NETs) (Figure 1C), and secretion of pro-inflammatory cytokines and chemokines. Furthermore, they directly interact with macrophages, DCs, NK cells, B cells, and T cells (62). During pregnancy, NETs formation with microbicidal impact is considered as another defense mechanism to protect fetal tissues from infections (63) and hCG was reported to stimulate this pathway (64). In non-pregnant individuals, neutrophils can be found in the cervix, endometrium and fallopian tubes (65, 66) while in pregnant women neutrophils additionally invade the decidua, placenta, and fetal membranes (67, 68). At insemination neutrophils specifically migrate and accumulate around the uterine epithelium (69). Here, they overtake various critical functions associated with angiogenesis, uSA remodeling and trophoblast invasion (70, 71) (Figure 1D). One of the major molecules involved in neutrophil activity with regard to tissue remodeling processes is MMP-9 (72) (Figure 1C). Moreover, human neutrophils under the influence of P4 and E2 induce a specific subpopulation of regulatory T (Treg) cells with pro-angiogenic properties (73). Whether hCG affects neutrophils in a similar way is not known. However, it was described that low doses of hCG inhibit proliferation and induce apoptosis in human neutrophils (74, 75). By doing so, hCG may control neutrophil function as their excessive activation was proven in adverse pregnancy outcomes such as fetal loss and PE (67, 76).
Monocytes and Macrophages
After their generation in the bone marrow, monocytes typically circulate in the peripheral bloodstream for some days before they enter into tissues and differentiate into tissue-specific macrophages. Here, macrophages fulfill a plethora of different functions including the removal of dead cells and cell debris as well as the presentation of foreign antigens during inflammatory processes (77). Two major subtypes of macrophages have been described: pro-inflammatory M1 cells and anti-inflammatory M2 cells (78). As embryo implantation is a state of controlled inflammation, M1 cells are the main macrophage subtype during this period. Immediately after fertilization, macrophages are actively recruited into the endometrium, myometrium and the decidua and represent the second most abundant immune cell population (79). They support angiogenesis by secreting pro-angiogenic factors like VEGF-A, fibroblast growth factor, platelet derived growth factor, and angiopoietin-1 and−2 (Figure 1C). Moreover, although not directly affecting trophoblast invasion or smooth muscle cell organization, M1 cells promote uSA remodeling by inducing extracellular matrix breakdown and phagocyte apoptotic vascular smooth muscle cells (80). After implantation, macrophages have to switch into the immunomodulatory M2 phenotype to ensure tolerance toward the increasing levels of foreign fetal antigens. This M1 to M2 cell shift seems to be partially fostered by hCG as indicated in human and mouse experimental settings (81, 82) (Figure 1C). On the other hand, hCG stimulates pro-inflammatory functions in human monocytes and macrophages (83, 84) suggesting that in case of an infection, hCG helps to protect the fetus from being attacked. Notably, human placental macrophages are able to produce high amounts of the pro-inflammatory cytokine IL-1 which in turn stimulates hCG secretion by human trophoblast cells (85) proposing a reciprocal interrelationship between macrophages and hCG during pregnancy.
Equally important are fetal macrophages, called Hofbauer cells that infiltrate the villous stroma and reside close to the fetal capillaries (86). Hofbauer cells represent a M2 phenotype and mainly produce IL-10 and TGF-β (87). They promote placental angiogenesis, villous tree growth and branching and protect the fetus from being immunological rejected (88, 89). Likewise human decidual macrophages (90), Hofbauer cells express the LH/CG receptor (91) allowing them to bind and incorporate hCG. This ability seems of fundamental importance in adjusting hCG levels at the fetal-maternal interface and to prevent an aberrant genital differentiation in early pregnancy (92).
Mast Cells
The contribution of mast cells (MCs) for pregnancy establishment, maintenance, and termination was longtime controversially discussed (93–96). However, nowadays there is accumulating evidence that MCs are indeed critically involved in pregnancy success and in particular regulate fundamental processes during early pregnancy. MCs are present in human (97) and rodent uteri (98, 99) exhibiting a mixed population of different MC types (97, 100). We showed that female mice devoid of MCs were either completely incapable to implant or implanted but showed insufficient uSA remodeling and abnormal placentation (100) resulting in IUGR (101) (Figure 1D). Our and other data further revealed that factors like VEGF (102), galectin-1 (100), and chymases (103) are involved in MC-mediated activities (Figure 1C). As human and mouse MCs express steroid receptors (97, 104) it is suggested that their functionality is affected by the local hormonal environment. In fact, E2 and P4 upregulate chemokine receptor expression on MCs and promote their immigration into the fetal-maternal interface, induce the production of MC mediators and their release by degranulation (104). However, whether hCG possesses similar effects on MCs is unknown as research activities in this field are very limited.
Natural Killer Cells
Uterine NK cells display a unique profile that differentiates these cells fundamentally from their peripheral counterparts. A high cytokine and angiogenic secretory profile as well as a poor cytotoxic potential are characteristic for uterine NK cells (105). During the first trimester of pregnancy NK cells represent the most abundant immune cell population in decidual tissue (105). However, whether decidual NK cells are derived from peripheral NK cells that enter the fetal-maternal interface and convert into the decidual phenotype and/or expand from residual NK cells is not finally resolved (106, 107). Recently, it was suggested that under the influence of P4 and IL-15, peripheral NK cells start to proliferate, migrate into the implantation site along chemotactic and hormonal gradients provided by trophoblast and endometrial cells and finally convert into decidual NK cells due to high levels of local TGF-β and IL-11 (106).
hCG seems to influence peripheral and endometrial NK cells in several ways although hCG effects on peripheral NK cells are rather inconclusive. While studies from the 80s showed an inhibitory effect of hCG on human peripheral NK cell activity (108, 109), more recent studies suggested a stimulatory effect of hCG on NK cell activity and number (110). Shirshev et al. proposed that hCG levels representative for the first trimester stimulated the expression of specific miRNAs within peripheral NK cells known as positive regulators of NK cell survival, cytolytic activity and production of pro-inflammatory cytokines (111). Notably, higher KIR2DL4+ peripheral NK cell numbers and lower implantation rates have been found in patients undergoing IVF and receiving hCG for final oocyte maturation suggesting that in those patients hCG treatment may compromise pregnancy success (112). By contrast, hCG elevates the number of endometrial NK cells through the mannose receptor and may therefore positively influence embryo implantation (113) (Figure 1C).
In line with macrophages and MCs, uterine NK cells are key regulators of embryo implantation and placentation. In both humans and mice, uterine NK cells control trophoblast invasion, support angiogenesis and contribute to proper uSA remodeling (Figure 1D). More specifically, human uterine NK cells either directly force remodeling of the endometrial vascular bed through secretion of MMPs (114) or indirectly by regulating invasive trophoblast cells that in turn promote vascular transformation (115). Human uterine NK cells also produce and secrete a variety of pro-angiogenic factors including VEGF, placental growth factor, angiopoietin-1, and angiogenin-2 (116) (Figure 1C). In mice, uterine NK cells are subdivided in two major subsets based on the expression Dolichos biflorus agglutinin (DBA). Whereas, DBA− uNK cells secrete high amounts of IFN-γ and thereby assist in vascular remodeling (117), DBA+ uNK cells predominantly produce pro-angiogenic factors (118). In contrast to human uterine NK cells suggested to either enhance or inhibit trophoblast invasion, mouse uNK cells seem to primarily suppress trophoblast motility (119).
Our own research studies identified the heme catabolizing enzyme heme oxygenase-1 (HO-1) as a regulator of uterine NK cell numbers and functionality. HO-1 deficient female mice showed fewer uterine NK cells and lower expression of uterine NK cell-associated angiogenic factors. Moreover, pregnant HO-1 deficient female mice displayed insufficient remodeled uSAs, IUGR fetuses and gestational hypertension (120, 121). The administration of gaseous carbon monoxide, a HO-1 degradation product, normalized uNK numbers and restored uSA remodeling, suggesting that uNKs cells are responsible for SA remodeling. However, fetal impairments are not only attributable to NK absence as their depletion, although interfering with uSA remodeling, did not result in fetal growth restriction (103). This finding, however, contradicts observations made by other research groups (122, 123). Intriguingly, in our NK cell-deficient mouse model, females increase their number of uterine MCs that can compensate for the effects of NK cells and avert major damage to the fetus (124). Accordingly, pregnant females lacking both innate immune cell populations show markedly impaired uSA remodeling and high vascular resistance resulting in disturbed fetal development and growth restricted neonates (101). This was attributable to the chymase Mcpt5, secreted by both cell types (103). Consequently, mice without Mcpt5+ cells present a similar phenotype of impaired uSA remodeling and IUGR but not hypertension (103).
Dendritic Cells
DCs link the innate and the adaptive immune system and are therefore decisive for the induction of late immune responses. Although they are detectable in the uterine tissue before and during pregnancy, compared to macrophages and NK cells, DCs represent a minor immune cell population. DCs accumulate in the uterine tissue when the embryo establishes its first contacts with the maternal endometrium (125). At this time, uterine DCs are critically involved in endometrial changes that are indispensable for further pregnancy progression. In female mice lacking decidual DCs it has been shown that decidual proliferation and differentiation was markedly impaired. Moreover, these females showed perturbed angiogenesis characterized by reduced vascular expansion and attenuated maturation (125). In agreement, impaired homing of CXCR4+ DCs into decidual tissue led to a disorganized vasculature with improper uSA remodeling later on (126). As a result, decidual DC-deficient females were unable to implant (125).
DCs also participate in the ovulation process. In follicular fluid, these cells make up a major fraction of all ovarian immune cells and were proven to possess an anti-inflammatory capacity that likely serves to restrict the ovulatory-associated inflammation (127). Furthermore, ovarian DCs are essential for expansion of the cumulus-oocyte complex, release of the ovum from the ovarian follicle, corpus luteum formation and enhanced lymphangiogenesis (49). Gonadotropins including hCG induce immigration of DCs into ovaries, thus supporting DC-mediated ovulation control (49) (Figure 1A).
Additionally to their non-immunological activities during pregnancy, DCs are naturally involved in decisions whether fetal tissues are immunologically tolerated or rejected. Typically, DCs take up antigens in peripheral tissues, undergo maturation, immigrate into lymphoid organs and finally present antigen-derived peptides to T cells. By doing so, DCs efficiently prime T cell responses either with a pro- or an anti-inflammatory profile. As presentation of fetal alloantigens may provoke an overwhelming alloreactive T cell response leading to fetal rejection, phenotype, and functions of DCs are modulated during normal pregnancy to restrict detrimental effects for the fetus. This accounts in particular for decidual DCs that in contrast to their peripheral counterparts possess a tolerant, immune regulatory phenotype characterized by a reduced T cell stimulatory capacity (128) and high expression of IL-10 and TGF-β (129). Decidual DCs are able to induce Treg cells and Th2 responses contributing to an overall anti-inflammatory fetal environment (130) (Figure 1C). Both decidua- and trophoblast-derived factors such as E2, soluble HLA-G, glycoprotein 1a, thymic stromal lymphopoietin and galectin-1 instruct DCs to adopt a tolerogenic phenotype (131–135). Moreover, several human and mouse studies identified hCG as a regulator of DCs during pregnancy, however with contradicting results (131, 136–138). Our own analyses revealed that the application of hCG as well as adoptive transfer of hCG-treated tolerogenic DCs into abortion-prone females significantly reduced peripheral and local frequencies of mature DCs, increased Treg cells and protected fetuses from rejection (139, 140). Notably, tolerogenic DCs have to be present at fecundation to confer protection (140) emphasizing their role at very early pregnancy stages. In humans, we proposed hCG as a factor maintaining mature peripheral DCs, and particular myeloid DCs type 1, at low levels but not as a general regulator of DC frequencies during pregnancy (141, 142).
B Cells
B cells are one of the two major immune cell types belonging to the adaptive branch of the immune system. Best known for their ability to produce immunoglobulins, B cells also participate in antigen presentation and in cytokine production. Early studies from the 70s and 80s investigated the development of an anti-paternal humoral immune response during murine pregnancy and found that this response was restricted to specific allogeneic mating combinations and only became evident after repeated mating cycles. Bell and Billingham (143) further identified the placenta as the tissue provoking the anti-paternal humoral immune response. Nowadays, the participation of B cells in fundamental pregnancy processes is increasingly becoming the focus and it is to be assumed that these cells are also involved in embryo implantation and placentation. We recently observed that pregnant female mice lacking B cells were more susceptible to lipopolysaccharide meaning their fetuses died at doses compatible with fetuses from B cell-competent female mice. This implies a critical role of B cells in the control of bacterial infections during pregnancy (144). Under steady state conditions, mouse studies from the 80s proposed that B cell deficiency during pregnancy does not affect resorption frequencies, litter sizes and neonatal survival (145–147). Our own previous findings show that fetuses of B cell-deficient female mice were smaller compared to fetuses from B cell-competent female mice already within the first half of pregnancy (144). This implies that a lack of B cells during pregnancy, although not leading to complete fetal loss, compromises fetal growth and may thus affect the health of the progeny in long term.
In humans, a B cell deficit evoked by medical intervention before or during pregnancy resulted in an elevated rate of pregnancy-associated complications such as spontaneous abortions, PE, IUGR, and preterm deliveries (148–150) suggesting that B cells are involved in early pregnancy pathways determining pregnancy outcome. However, the study of phenotypic and functional characteristics of uterine B cell populations is impeded by their scarcity at the fetal-maternal interface (151). Analyses of B cell frequencies during mouse and human pregnancy revealed around 1% of mouse B cells and <5% of human B cells in decidual tissue (152, 153). Furthermore, pregnancy-driven changes in B cell numbers of all developmental stages in bone marrow, blood, spleen and lymph nodes (154–160) may affect local B cell frequencies and if deregulated may cause adverse pregnancy outcomes. Indeed, there is evidence that not only a lack but also an overrepresentation of distinct B cell populations at different gestational ages can harm the fetus. For instance, reduced frequencies of regulatory B (Breg) cells during early pregnancy in humans and mice are associated with spontaneous abortions (161, 162). Breg cells comprise all B cells that negatively regulate immune responses and are able to secrete high amounts of immune suppressive cytokines such as IL-10, IL-35, and TGF-β (Figure 1C). We and other research groups demonstrated that hCG not only suppressed the proliferation of mouse splenic B cells but also induced the generation of mouse and human IL-10-producing Breg cells as well as the production of pregnancy-protective asymmetric antibodies (163, 164) (Figure 1C). By doing so, hCG supports the immune suppressive characteristics of B cells and fosters maternal immune tolerance during early pregnancy.
Yet, there are two sides of the medal. In mice, Breg cells are often reported to be part of the CD19+ CD5+ B1a cell population (165), however B1a B cells depending on their phenotype seem to play an ambivalent role during pregnancy. We found that mouse peritoneal B1a B cells expressing high levels of plasma cell alloantigen 1 and IL-10 support fetal survival, whereas B1a B cells expressing low levels of both marker molecules can induce fetal rejection (166). Moreover, increased frequencies of human B1a B cells during the third trimester of pregnancy were detected in PE patients and were associated with pathologic elevated hCG levels proposing a rather detrimental effect of hCG in this regard. As B1a B cells possess the capacity to produce autoantibodies against the angiotensin II type 1 receptor they were suggested to promote PE-associated symptoms (12). Likewise, production of autoantibodies by increased numbers of plasma cells present in endometrial lesions of endometriosis patients was associated with infertility (167).
Altogether, physiological elevated hCG levels during early pregnancy assist in fetal tolerance induction by promoting the generation and function of Breg cells, whereas high hCG levels at later pregnancy stages may compromise fetal well-being by enhancing autoreactive B cells. Whether hCG encourages B cells in promoting embryo implantation and placentation remains to be elucidated.
T Cells
T cells being the second major adaptive immune cell type are more abundant at the fetal-maternal interface than B cells and are much better studied. Approximately 10–20% of all decidual leukocytes are T cells, being CD8+ T cells more than CD4+ T cells including classical as well as regulatory subsets (168). In mice, CD4+ T helper (Th) and CD8+ T cells represent up to 2% of decidual leukocytes during early and mid-gestation (169). It is suggested that human decidual T cells are highly differentiated, express a broad range of cytokines and cytotoxic markers and exhibit a unique transcriptional profile characterized by strong expression of genes involved in interferon signaling (170). Moreover, although capable to recognize the foreign trophoblastic antigens, decidual T cells do not attack trophoblast cells but rather support their growth and invasiveness (171). Among all decidual T cells, the best described are pro-inflammatory Th1 and Th17 cells as well as anti-inflammatory Th2 and Foxp3+ Treg cells and dysregulations in these Th subsets are indicative for several pregnancy complications (172, 173). Nevertheless, the functional properties of decidual T cells are still poorly defined.
Normal pregnancy progression is the result of timely-regulated local shifts between pro- and anti-inflammatory immune responses allowing implantation and placentation in a pro-inflammatory environment, fetal growth in an anti-inflammatory environment and finally the induction of labor and delivery again under pro-inflammatory conditions (174). hCG was shown to affect Th cells in several ways and it is suggested that the hormone advance the passage from a Th1-dominated into a Th2-dominated local environment at the end of the first trimester. hCG is suggested to impair proliferation and to induce apoptosis of conventional T cells (175–179), thus reducing the number of alloreactive T cells that may harm the fetus. Moreover, hCG helps T cells to adopt a suppressor phenotype and to secrete preferentially immune regulatory cytokines such as IL-10 and TGF-β (139, 175, 180, 181). This is true for both human and mouse T cells as both express highly conserved LH/CG receptor molecules (139, 182) and are susceptible to hCG signaling. hCG signaling in turn was proposed to act through the signaling molecules AKT and ERK (183).
Remarkably, in vivo hCG administration into mice and humans could significantly improve pregnancy outcomes. We showed that hCG injection during the peri-implantation period into abortion-prone mice increased Treg cell frequencies and significantly reduced the fetal rejection rate (139). In agreement, IVF patients receiving hCG exhibited significantly elevated peripheral Treg cell levels associated with improved IR and LBR when compared to non-hCG-treated controls (36). Likewise, spontaneous abortion patients showed lower peripheral numbers of Th17 cells and elevated Treg cell frequencies after hCG exposure (184, 185), indicating that hCG is able to correct immunological dysbalances associated with adverse pregnancy outcomes. It is worthy to speculate that hCG not only increases peripheral Treg cells but also augments the local Treg cell pool. By this means, hCG may act through three different pathways: (A) active recruitment of peripheral Treg cells into the fetal-maternal interface (186), (B) local expansion of decidual Treg cells (82), and (C) conversion of conventional T cells into Treg cells (181, 183) (Figure 1C).
Recently, Robertson and colleagues provided novel insights into the functionality of decidual Treg cells. The authors proposed a role of decidual Treg cells in preventing excessive inflammatory responses evoked by local effector T cells, a supportive function for other leukocytes and non-hematopoietic cells in the implantation process and a direct involvement of decidual Treg cells in adaptions of the maternal vascular bed (187) (Figure 1D). All these mechanisms are pivotal for the embryo to successfully pass the first steps of its development and because of their modulation by hCG, it can be stated that this unique hormone is critically involved.
Concluding Remarks
During early pregnancy, innate and adaptive immune cells participate in several fundamental physiological processes including trophoblast invasion, decidualization, angiogenesis, and placentation, all of them allowing proper fetal development and growth. Hereby, some of the immune cells work in close relationships as suggested for MCs, NK cells, and DCs. Moreover, as emphasized throughout this review, hCG is a molecule with a multitude of immunological properties. This hormone does not only regulate local immune cell numbers but also forces these cells to adopt a unique phenotype with the aim to support and protect the fetus. Taken together, the literature discussed here may at least partially provide an explanation for the success rates after hCG treatments in ART or miscarriage patients.
Author Contributions
AS and AZ wrote and revised the manuscript.
Conflict of Interest
The authors declare that the research was conducted in the absence of any commercial or financial relationships that could be construed as a potential conflict of interest.
References
1. Bischof P, Campana A. Trophoblast differentiation and invasion: its significance for human embryo implantation. Early Pregnancy. (1997) 3:81–95.
2. Whitley GS, Cartwright JE. Cellular and molecular regulation of spiral artery remodelling: lessons from the cardiovascular field. Placenta. (2010) 31:465–74. doi: 10.1016/j.placenta.2010.03.002
3. Huppertz B. The critical role of abnormal trophoblast development in the etiology of preeclampsia. Curr Pharm Biotechnol. (2018) 19:771–80. doi: 10.2174/1389201019666180427110547
4. Ramathal CY, Bagchi IC, Taylor RN, Bagchi MK. Endometrial decidualization: of mice and men. Semin Reprod Med. (2010) 28:17–26. doi: 10.1055/s-0029-1242989
5. Marquardt RM, Kim TH, Shin JH, Jeong JW. Progesterone and estrogen signaling in the endometrium: what goes wrong in endometriosis? Int J Mol Sci. (2019) 20:E3822. doi: 10.3390/ijms20153822
6. Kim SM, Kim JS. A review of mechanisms of implantation. Dev Reprod. (2017) 21:351–9. doi: 10.12717/DR.2017.21.4.351
7. Bonduelle ML, Dodd R, Liebaers I, Van Steirteghem A, Williamson R, Akhurst R. Chorionic gonadotrophin-beta mRNA, a trophoblast marker, is expressed in human 8-cell embryos derived from tripronucleate zygotes. Hum Reprod. (1988) 3:909–14. doi: 10.1093/oxfordjournals.humrep.a136808
8. Lopata A, Hay DL. The potential of early human embryos to form blastocysts, hatch from their zona and secrete HCG in culture. Hum Reprod. (1989) 4(8 Suppl.):87–94. doi: 10.1093/humrep/4.suppl_1.87
9. Jeschke U, Karsten U, Reimer T, Richter DU, Bergemann C, Briese V, et al. Stimulation of hCG protein and mRNA in first trimester villous cytotrophoblast cells in vitro by glycodelin A. J Perinat Med. (2005) 33:212–8. doi: 10.1515/JPM.2005.039
10. Than NG, Romero R, Tarca AL, Kekesi KA, Xu Y, Xu Z, et al. Integrated systems biology approach identifies novel maternal and placental pathways of preeclampsia. Front Immunol. (2018) 9:1661. doi: 10.3389/fimmu.2018.01661
11. Panwar M, Kumari A, Hp A, Arora R, Singh V, Bansiwal R. Raised neutrophil lymphocyte ratio and serum beta hCG level in early second trimester of pregnancy as predictors for development and severity of preeclampsia. Drug Discov Ther. (2019) 13:34–7. doi: 10.5582/ddt.2019.01006
12. Jensen F, Wallukat G, Herse F, Budner O, El-Mousleh T, Costa SD, et al. CD19+CD5+ cells as indicators of preeclampsia. Hypertension. (2012) 59:861–8. doi: 10.1161/HYPERTENSIONAHA.111.188276
13. Cole LA. hCG, five independent molecules. Clin Chim Acta. (2012) 413:48–65. doi: 10.1016/j.cca.2011.09.037
14. Jarvela IY, Ruokonen A, Tekay A. Effect of rising hCG levels on the human corpus luteum during early pregnancy. Hum Reprod. (2008) 23:2775–81. doi: 10.1093/humrep/den299
15. Shi QJ, Lei ZM, Rao CV, Lin J. Novel role of human chorionic gonadotropin in differentiation of human cytotrophoblasts. Endocrinology. (1993) 132:1387–95. doi: 10.1210/endo.132.3.7679981
16. Rao CV, Li X, Toth P, Lei ZM, Cook VD. Novel expression of functional human chorionic gonadotropin/luteinizing hormone receptor gene in human umbilical cords. J Clin Endocrinol Metab. (1993) 77:1706–14. doi: 10.1210/jc.77.6.1706
17. Rao CV, Lei ZM. The past, present and future of nongonadal LH/hCG actions in reproductive biology and medicine. Mol Cell Endocrinol. (2007) 269:2–8. doi: 10.1016/j.mce.2006.07.007
18. Goldsmith PC, McGregor WG, Raymoure WJ, Kuhn RW, Jaffe RB. Cellular localization of chorionic gonadotropin in human fetal kidney and liver. J Clin Endocrinol Metab. (1983) 57:654–61. doi: 10.1210/jcem-57-3-654
19. Berndt S, Blacher S, Perrier d'Hauterive S, Thiry M, Tsampalas M, Cruz A, et al. Chorionic gonadotropin stimulation of angiogenesis and pericyte recruitment. J Clin Endocrinol Metab. (2009) 94:4567–74. doi: 10.1210/jc.2009-0443
20. Zygmunt M, Herr F, Keller-Schoenwetter S, Kunzi-Rapp K, Munstedt K, Rao CV, et al. Characterization of human chorionic gonadotropin as a novel angiogenic factor. J Clin Endocrinol Metab. (2002) 87:5290–6. doi: 10.1210/jc.2002-020642
21. Herr F, Baal N, Reisinger K, Lorenz A, McKinnon T, Preissner KT, et al. HCG in the regulation of placental angiogenesis. Results of an in vitro study. Placenta. (2007) 28(Suppl. A):S85–93. doi: 10.1016/j.placenta.2007.02.002
22. Eta E, Ambrus G, Rao CV. Direct regulation of human myometrial contractions by human chorionic gonadotropin. J Clin Endocrinol Metab. (1994) 79:1582–6. doi: 10.1210/jcem.79.6.7989459
23. Licht P, Losch A, Dittrich R, Neuwinger J, Siebzehnrubl E, Wildt L. Novel insights into human endometrial paracrinology and embryo-maternal communication by intrauterine microdialysis. Hum Reprod Update. (1998) 4:532–8. doi: 10.1093/humupd/4.5.532
24. Fluhr H, Bischof-Islami D, Krenzer S, Licht P, Bischof P, Zygmunt M. Human chorionic gonadotropin stimulates matrix metalloproteinases-2 and−9 in cytotrophoblastic cells and decreases tissue inhibitor of metalloproteinases-1,−2, and−3 in decidualized endometrial stromal cells. Fertil Steril. (2008) 90(4 Suppl.):1390–5. doi: 10.1016/j.fertnstert.2007.08.023
25. Fogle RH, Li A, Paulson RJ. Modulation of HOXA10 and other markers of endometrial receptivity by age and human chorionic gonadotropin in an endometrial explant model. Fertil Steril. (2010) 93:1255–9. doi: 10.1016/j.fertnstert.2008.11.002
26. Yang H, Lei CX, Zhang W. Human chorionic gonadotropin (hCG) regulation of galectin-3 expression in endometrial epithelial cells and endometrial stromal cells. Acta Histochem. (2013) 115:3–7. doi: 10.1016/j.acthis.2011.05.002
27. Sasaki Y, Ladner DG, Cole LA. Hyperglycosylated human chorionic gonadotropin and the source of pregnancy failures. Fertil Steril. (2008) 89:1781–6. doi: 10.1016/j.fertnstert.2007.03.010
28. Cole LA. Hyperglycosylated hCG, a review. Placenta. (2010) 31:653–64. doi: 10.1016/j.placenta.2010.06.005
29. Sunder S, Lenton EA. Endocrinology of the peri-implantation period. Baillieres Best Pract Res Clin Obstet Gynaecol. (2000) 14:789–800. doi: 10.1053/beog.2000.0119
30. Cole LA. hCG, the wonder of today's science. Reprod Biol Endocrinol. (2012) 10:24. doi: 10.1186/1477-7827-10-24
31. Cole LA, Gutierrez JM. Production of human chorionic gonadotropin during the normal menstrual cycle. J Reprod Med. (2009) 54:245–50.
32. Bashiri A, Halper KI, Orvieto R. Recurrent implantation failure-update overview on etiology, diagnosis, treatment and future directions. Reprod Biol Endocrinol. (2018) 16:121. doi: 10.1186/s12958-018-0414-2
33. Chen Y, Zhang Y, Hu M, Liu X, Qi H. Timing of human chorionic gonadotropin (hCG) hormone administration in IVF/ICSI protocols using GnRH agonist or antagonists: a systematic review and meta-analysis. Gynecol Endocrinol. (2014) 30:431–7. doi: 10.3109/09513590.2014.895984
34. Xu Z, Chen W, Chen C, Xiao Y, Chen X. Effect of intrauterine injection of human chorionic gonadotropin before frozen-thawed embryo transfer on pregnancy outcomes in women with endometriosis. J Int Med Res. (2019) 47:2873–80. doi: 10.1177/0300060519848928
35. Mostajeran F, Godazandeh F, Ahmadi SM, Movahedi M, Jabalamelian SA. Effect of intrauterine injection of human chorionic gonadotropin before embryo transfer on pregnancy rate: a prospective randomized study. J Res Med Sci. (2017) 22:6. doi: 10.4103/1735-1995.199096
36. Liu X, Ma D, Wang W, Qu Q, Zhang N, Wang X, et al. Intrauterine administration of human chorionic gonadotropin improves the live birth rates of patients with repeated implantation failure in frozen-thawed blastocyst transfer cycles by increasing the percentage of peripheral regulatory T cells. Arch Gynecol Obstet. (2019) 299:1165–72. doi: 10.1007/s00404-019-05047-6
37. Huang P, Wei L, Li X, Qin A. Effects of intrauterine perfusion of human chorionic gonadotropin in women with different implantation failure numbers. Am J Reprod Immunol. (2018) 79:e12809. doi: 10.1111/aji.12809
38. Simopoulou M, Sfakianoudis K, Maziotis E, Tsioulou P, Giannelou P, Grigoriadis S, et al. Investigating the optimal time for intrauterine human chorionic gonadotropin infusion in order to improve IVF outcome: a systematic review and meta-analysis. In Vivo. (2019) 33:1737–49. doi: 10.21873/invivo.11664
39. Makrigiannakis A, Vrekoussis T, Zoumakis E, Kalantaridou SN, Jeschke U. The role of HCG in implantation: a mini-review of molecular and clinical evidence. Int J Mol Sci. (2017) 18:E1305. doi: 10.3390/ijms18061305
40. Ye H, Hu J, He W, Zhang Y, Li C. The efficacy of intrauterine injection of human chorionic gonadotropin before embryo transfer in assisted reproductive cycles: meta-analysis. J Int Med Res. (2015) 43:738–46. doi: 10.1177/0300060515592903
41. Strug MR, Su R, Young JE, Dodds WG, Shavell VI, Diaz-Gimeno P, et al. Intrauterine human chorionic gonadotropin infusion in oocyte donors promotes endometrial synchrony and induction of early decidual markers for stromal survival: a randomized clinical trial. Hum Reprod. (2016) 31:1552–61. doi: 10.1093/humrep/dew080
42. Osman A, Pundir J, Elsherbini M, Dave S, El-Toukhy T, Khalaf Y. The effect of intrauterine HCG injection on IVF outcome: a systematic review and meta-analysis. Reprod Biomed Online. (2016) 33:350–9. doi: 10.1016/j.rbmo.2016.05.010
43. Hou W, Shi G, Cai B, Ding C, Song J, Zhang X, et al. Effect of intrauterine injection of human chorionic gonadotropin before fresh embryo transfer on IVF and ICSI outcomes: a meta-analysis. Arch Gynecol Obstet. (2018) 298:1061–9. doi: 10.1007/s00404-018-4923-1
44. Volovsky M, Healey M, MacLachlan V, Vollenhoven BJ. Should intrauterine human chorionic gonadotropin infusions ever be used prior to embryo transfer? J Assist Reprod Genet. (2018) 35:273–8. doi: 10.1007/s10815-017-1049-5
45. Craciunas L, Tsampras N, Raine-Fenning N, Coomarasamy A. Intrauterine administration of human chorionic gonadotropin (hCG) for subfertile women undergoing assisted reproduction. Cochrane Database Syst Rev. (2018) 10:CD011537. doi: 10.1002/14651858.CD011537.pub3
46. Gao M, Jiang X, Li B, Li L, Duan M, Zhang X, et al. Intrauterine injection of human chorionic gonadotropin before embryo transfer can improve in vitro fertilization-embryo transfer outcomes: a meta-analysis of randomized controlled trials. Fertil Steril. (2019) 112:89–97 e1. doi: 10.1016/j.fertnstert.2019.02.027
47. Schumacher A, Costa SD, Zenclussen AC. Endocrine factors modulating immune responses in pregnancy. Front Immunol. (2014) 5:196. doi: 10.3389/fimmu.2014.00196
48. Tsampalas M, Gridelet V, Berndt S, Foidart JM, Geenen V, Perrier d'Hauterive S. Human chorionic gonadotropin: a hormone with immunological and angiogenic properties. J Reprod Immunol. (2010) 85:93–8. doi: 10.1016/j.jri.2009.11.008
49. Cohen-Fredarow A, Tadmor A, Raz T, Meterani N, Addadi Y, Nevo N, et al. Ovarian dendritic cells act as a double-edged pro-ovulatory and anti-inflammatory sword. Mol Endocrinol. (2014) 28:1039–54. doi: 10.1210/me.2013-1400
50. Turner EC, Hughes J, Wilson H, Clay M, Mylonas KJ, Kipari T, et al. Conditional ablation of macrophages disrupts ovarian vasculature. Reproduction. (2011) 141:821–31. doi: 10.1530/REP-10-0327
51. Al-Alem L, Puttabyatappa M, Rosewell K, Brannstrom M, Akin J, Boldt J, et al. Chemokine ligand 20: a signal for leukocyte recruitment during human ovulation? Endocrinology. (2015) 156:3358–69. doi: 10.1210/en.2014-1874
52. Akoum A, Metz CN, Morin M. Marked increase in macrophage migration inhibitory factor synthesis and secretion in human endometrial cells in response to human chorionic gonadotropin hormone. J Clin Endocrinol Metab. (2005) 90:2904–10. doi: 10.1210/jc.2004-1900
53. Madkour A, Bouamoud N, Louanjli N, Kaarouch I, Copin H, Benkhalifa M, et al. Intrauterine insemination of cultured peripheral blood mononuclear cells prior to embryo transfer improves clinical outcome for patients with repeated implantation failures. Zygote. (2016) 24:58–69. doi: 10.1017/S0967199414000719
54. Nobijari FF, Arefi SS, Moini A, Taheripanah R, Fazeli E, Kharazi H, et al. Endometrium immunomodulation by intrauterine insemination administration of treated peripheral blood mononuclear cell prior frozen/thawed embryos in patients with repeated implantation failure. Zygote. (2019) 27:214–8. doi: 10.1017/S0967199419000145
55. Yoshioka S, Fujiwara H, Nakayama T, Kosaka K, Mori T, Fujii S. Intrauterine administration of autologous peripheral blood mononuclear cells promotes implantation rates in patients with repeated failure of IVF-embryo transfer. Hum Reprod. (2006) 21:3290–4. doi: 10.1093/humrep/del312
56. Okitsu O, Kiyokawa M, Oda T, Miyake K, Sato Y, Fujiwara H. Intrauterine administration of autologous peripheral blood mononuclear cells increases clinical pregnancy rates in frozen/thawed embryo transfer cycles of patients with repeated implantation failure. J Reprod Immunol. (2011) 92:82–7. doi: 10.1016/j.jri.2011.07.001
57. Li S, Wang J, Cheng Y, Zhou D, Yin T, Xu W, et al. Intrauterine administration of hCG-activated autologous human peripheral blood mononuclear cells (PBMC) promotes live birth rates in frozen/thawed embryo transfer cycles of patients with repeated implantation failure. J Reprod Immunol. (2017) 119:15–22. doi: 10.1016/j.jri.2016.11.006
58. Yu N, Yang J, Guo Y, Fang J, Yin T, Luo J, et al. Intrauterine administration of peripheral blood mononuclear cells (PBMCs) improves endometrial receptivity in mice with embryonic implantation dysfunction. Am J Reprod Immunol. (2014) 71:24–33. doi: 10.1111/aji.12150
59. Nakayama T, Fujiwara H, Maeda M, Inoue T, Yoshioka S, Mori T, et al. Human peripheral blood mononuclear cells (PBMC) in early pregnancy promote embryo invasion in vitro: HCG enhances the effects of PBMC. Hum Reprod. (2002) 17:207–12. doi: 10.1093/humrep/17.1.207
60. Egawa H, Fujiwara H, Hirano T, Nakayama T, Higuchi T, Tatsumi K, et al. Peripheral blood mononuclear cells in early pregnancy promote invasion of human choriocarcinoma cell line, BeWo cells. Hum Reprod. (2002) 17:473–80. doi: 10.1093/humrep/17.2.473
61. Yu N, Yan W, Yin T, Wang Y, Guo Y, Zhou D, et al. HCG-activated human peripheral blood mononuclear cells (PBMC) promote trophoblast cell invasion. PLoS ONE. (2015) 10:e0125589. doi: 10.1371/journal.pone.0125589
62. Yang F, Feng C, Zhang X, Lu J, Zhao Y. The diverse biological functions of neutrophils, beyond the defense against infections. Inflammation. (2017) 40:311–23. doi: 10.1007/s10753-016-0458-4
63. Gomez-Lopez N, Romero R, Xu Y, Miller D, Unkel R, Shaman M, et al. Neutrophil extracellular traps in the amniotic cavity of women with intra-amniotic infection: a new mechanism of host defense. Reprod Sci. (2017) 24:1139–53. doi: 10.1177/1933719116678690
64. Giaglis S, Stoikou M, Sur Chowdhury C, Schaefer G, Grimolizzi F, Rossi SW, et al. Multimodal regulation of NET formation in pregnancy: progesterone antagonizes the pro-NETotic effect of estrogen and G-CSF. Front Immunol. (2016) 7:565. doi: 10.3389/fimmu.2016.00565
65. Givan AL, White HD, Stern JE, Colby E, Gosselin EJ, Guyre PM, et al. Flow cytometric analysis of leukocytes in the human female reproductive tract: comparison of fallopian tube, uterus, cervix, and vagina. Am J Reprod Immunol. (1997) 38:350–9. doi: 10.1111/j.1600-0897.1997.tb00311.x
66. King AE, Critchley HO, Kelly RW. Innate immune defences in the human endometrium. Reprod Biol Endocrinol. (2003) 1:116. doi: 10.1186/1477-7827-1-116
67. Giaglis S, Stoikou M, Grimolizzi F, Subramanian BY, van Breda SV, Hoesli I, et al. Neutrophil migration into the placenta: good, bad or deadly? Cell Adh Migr. (2016) 10:208–25. doi: 10.1080/19336918.2016.1148866
68. Osman I, Young A, Ledingham MA, Thomson AJ, Jordan F, Greer IA, et al. Leukocyte density and pro-inflammatory cytokine expression in human fetal membranes, decidua, cervix and myometrium before and during labour at term. Mol Hum Reprod. (2003) 9:41–5. doi: 10.1093/molehr/gag001
69. Song ZH, Li ZY, Li DD, Fang WN, Liu HY, Yang DD, et al. Seminal plasma induces inflammation in the uterus through the gammadelta T/IL-17 pathway. Sci Rep. (2016) 6:25118. doi: 10.1038/srep25118
70. Kliman HJ, Sammar M, Grimpel YI, Lynch SK, Milano KM, Pick E, et al. Placental protein 13 and decidual zones of necrosis: an immunologic diversion that may be linked to preeclampsia. Reprod Sci. (2012) 19:16–30. doi: 10.1177/1933719111424445
71. Kobara H, Miyamoto T, Suzuki A, Asaka R, Yamada Y, Ishikawa K, et al. Lipocalin2 enhances the matrix metalloproteinase-9 activity and invasion of extravillous trophoblasts under hypoxia. Placenta. (2013) 34:1036–43. doi: 10.1016/j.placenta.2013.08.004
72. Daimon E, Wada Y. Role of neutrophils in matrix metalloproteinase activity in the preimplantation mouse uterus. Biol Reprod. (2005) 73:163–71. doi: 10.1095/biolreprod.104.038539
73. Nadkarni S, Smith J, Sferruzzi-Perri AN, Ledwozyw A, Kishore M, Haas R, et al. Neutrophils induce proangiogenic T cells with a regulatory phenotype in pregnancy. Proc Natl Acad Sci USA. (2016) 113:E8415–24. doi: 10.1073/pnas.1611944114
74. Shirshev SV, Kuklina EM. Role of cAMP and neutrophil cyclooxygenase in gonadotropin-dependent regulation of T lymphocyte proliferation. Biochemistry. (2001) 66:994–8. doi: 10.1023/A:1012321609879
75. Shirshev SV, Kuklina EM, Yarilin AA. Reproductive hormones in the regulation of apoptosis of neutrophils. Biochemistry. (2003) 68:688–95. doi: 10.1023/A:1024626128286
76. Hahn S, Giaglis S, Hoesli I, Hasler P. Neutrophil NETs in reproduction: from infertility to preeclampsia and the possibility of fetal loss. Front Immunol. (2012) 3:362. doi: 10.3389/fimmu.2012.00362
77. Gordon S, Taylor PR. Monocyte and macrophage heterogeneity. Nat Rev Immunol. (2005) 5:953–64. doi: 10.1038/nri1733
78. Brown MB, von Chamier M, Allam AB, Reyes L. M1/M2 macrophage polarity in normal and complicated pregnancy. Front Immunol. (2014) 5:606. doi: 10.3389/fimmu.2014.00606
79. Ramhorst R, Grasso E, Paparini D, Hauk V, Gallino L, Calo G, et al. Decoding the chemokine network that links leukocytes with decidual cells and the trophoblast during early implantation. Cell Adh Migr. (2016) 10:197–207. doi: 10.1080/19336918.2015.1135285
80. Lash GE, Pitman H, Morgan HL, Innes BA, Agwu CN, Bulmer JN. Decidual macrophages: key regulators of vascular remodeling in human pregnancy. J Leukoc Biol. (2016) 100:315–25. doi: 10.1189/jlb.1A0815-351R
81. Rami D, La Bianca M, Agostinis C, Zauli G, Radillo O, Bulla R. The first trimester gravid serum regulates procalcitonin expression in human macrophages skewing their phenotype in vitro. Mediators Inflamm. (2014) 2014:248963. doi: 10.1155/2014/248963
82. Furcron AE, Romero R, Mial TN, Balancio A, Panaitescu B, Hassan SS, et al. Human chorionic gonadotropin has anti-inflammatory effects at the maternal-fetal interface and prevents endotoxin-induced preterm birth, but causes dystocia and fetal compromise in mice. Biol Reprod. (2016) 94:136. doi: 10.1095/biolreprod.116.139345
83. Abu Alshamat E, Al-Okla S, Soukkarieh CH, Kweider M. Human chorionic gonadotrophin (hCG) enhances immunity against L. tropica by stimulating human macrophage functions. Parasite Immunol. (2012) 34:449–54. doi: 10.1111/j.1365-3024.2012.01368.x
84. Wan H, van Helden-Meeuwsen CG, Garlanda C, Leijten LM, Maina V, Khan NA, et al. Chorionic gonadotropin up-regulates long pentraxin 3 expression in myeloid cells. J Leukoc Biol. (2008) 84:1346–52. doi: 10.1189/jlb.0108067
85. Yagel S, Lala PK, Powell WA, Casper RF. Interleukin-1 stimulates human chorionic gonadotropin secretion by first trimester human trophoblast. J Clin Endocrinol Metab. (1989) 68:992–5. doi: 10.1210/jcem-68-5-992
86. Young OM, Tang Z, Niven-Fairchild T, Tadesse S, Krikun G, Norwitz ER, et al. Toll-like receptor-mediated responses by placental Hofbauer cells (HBCs): a potential pro-inflammatory role for fetal M2 macrophages. Am J Reprod Immunol. (2015) 73:22–35. doi: 10.1111/aji.12336
87. Johnson EL, Chakraborty R. Placental Hofbauer cells limit HIV-1 replication and potentially offset mother to child transmission (MTCT) by induction of immunoregulatory cytokines. Retrovirology. (2012) 9:101. doi: 10.1186/1742-4690-9-101
88. Loegl J, Hiden U, Nussbaumer E, Schliefsteiner C, Cvitic S, Lang I, et al. Hofbauer cells of M2a, M2b and M2c polarization may regulate feto-placental angiogenesis. Reproduction. (2016) 152:447–55. doi: 10.1530/REP-16-0159
89. Anteby EY, Natanson-Yaron S, Greenfield C, Goldman-Wohl D, Haimov-Kochman R, Holzer H, et al. Human placental Hofbauer cells express sprouty proteins: a possible modulating mechanism of villous branching. Placenta. (2005) 26:476–83. doi: 10.1016/j.placenta.2004.08.008
90. Zhang YM, Rao Ch V, Lei ZM. Macrophages in human reproductive tissues contain luteinizing hormone/chorionic gonadotropin receptors. Am J Reprod Immunol. (2003) 49:93–100. doi: 10.1034/j.1600-0897.2003.00013.x
91. Sonoda N, Katabuchi H, Tashiro H, Ohba T, Nishimura R, Minegishi T, et al. Expression of variant luteinizing hormone/chorionic gonadotropin receptors and degradation of chorionic gonadotropin in human chorionic villous macrophages. Placenta. (2005) 26:298–307. doi: 10.1016/j.placenta.2004.07.001
92. Yamaguchi M, Ohba T, Tashiro H, Yamada G, Katabuchi H. Human chorionic gonadotropin induces human macrophages to form intracytoplasmic vacuoles mimicking Hofbauer cells in human chorionic villi. Cells Tissues Organs. (2013) 197:127–35. doi: 10.1159/000342806
93. Salamonsen LA, Jeziorska M, Newlands GF, Dey SK, Woolley DE. Evidence against a significant role for mast cells in blastocyst implantation in the rat and mouse. Reprod Fertil Dev. (1996) 8:1157–64. doi: 10.1071/RD9961157
94. Wordinger RJ, Jackson FL, Morrill A. Implantation, deciduoma formation and live births in mast cell-deficient mice (W/Wv). J Reprod Fertil. (1986) 77:471–6. doi: 10.1530/jrf.0.0770471
95. Szelag A, Merwid-Lad A, Trocha M. Histamine receptors in the female reproductive system. Part I. Role of the mast cells and histamine in female reproductive system. Ginekol Pol. (2002) 73:627–35.
96. Menzies FM, Higgins CA, Shepherd MC, Nibbs RJ, Nelson SM. Mast cells reside in myometrium and cervix, but are dispensable in mice for successful pregnancy and labor. Immunol Cell Biol. (2012) 90:321–9. doi: 10.1038/icb.2011.40
97. De Leo B, Esnal-Zufiaurre A, Collins F, Critchley HOD, Saunders PTK. Immunoprofiling of human uterine mast cells identifies three phenotypes and expression of ERbeta and glucocorticoid receptor. F1000Res. (2017) 6:667. doi: 10.12688/f1000research.11432.1
98. Schmerse F, Woidacki K, Riek-Burchardt M, Reichardt P, Roers A, Tadokoro C, et al. In vivo visualization of uterine mast cells by two-photon microscopy. Reproduction. (2014) 147:781–8. doi: 10.1530/REP-13-0570
99. Cocchiara R, Albeggiani G, Di Trapani G, Azzolina A, Lampiasi N, Rizzo F, et al. Oestradiol enhances in vitro the histamine release induced by embryonic histamine-releasing factor (EHRF) from uterine mast cells. Hum Reprod. (1992) 7:1036–41. doi: 10.1093/oxfordjournals.humrep.a137790
100. Woidacki K, Popovic M, Metz M, Schumacher A, Linzke N, Teles A, et al. Mast cells rescue implantation defects caused by c-kit deficiency. Cell Death Dis. (2013) 4:e462. doi: 10.1038/cddis.2012.214
101. Meyer N, Schuler T, Zenclussen AC. Simultaneous ablation of uterine natural killer cells and uterine mast cells in mice leads to poor vascularization and abnormal doppler measurements that compromise fetal well-being. Front Immunol. (2017) 8:1913. doi: 10.3389/fimmu.2017.01913
102. Bosquiazzo VL, Ramos JG, Varayoud J, Munoz-de-Toro M, Luque EH. Mast cell degranulation in rat uterine cervix during pregnancy correlates with expression of vascular endothelial growth factor mRNA and angiogenesis. Reproduction. (2007) 133:1045–55. doi: 10.1530/REP-06-0168
103. Meyer N, Woidacki K, Knofler M, Meinhardt G, Nowak D, Velicky P, et al. Chymase-producing cells of the innate immune system are required for decidual vascular remodeling and fetal growth. Sci Rep. (2017) 7:45106. doi: 10.1038/srep45106
104. Jensen F, Woudwyk M, Teles A, Woidacki K, Taran F, Costa S, et al. Estradiol and progesterone regulate the migration of mast cells from the periphery to the uterus and induce their maturation and degranulation. PLoS ONE. (2010) 5:e14409. doi: 10.1371/journal.pone.0014409
105. Gaynor LM, Colucci F. Uterine Natural killer cells: functional distinctions and influence on pregnancy in humans and mice. Front Immunol. (2017) 8:467. doi: 10.3389/fimmu.2017.00467
106. Olmos-Ortiz A, Flores-Espinosa P, Mancilla-Herrera I, Vega-Sanchez R, Diaz L, Zaga-Clavellina V. Innate immune cells and toll-like receptor-dependent responses at the maternal-fetal interface. Int J Mol Sci. (2019) 20:E3654. doi: 10.3390/ijms20153654
107. Manaster I, Mizrahi S, Goldman-Wohl D, Sela HY, Stern-Ginossar N, Lankry D, et al. Endometrial NK cells are special immature cells that await pregnancy. J Immunol. (2008) 181:1869–76. doi: 10.4049/jimmunol.181.3.1869
108. Sulke AN, Jones DB, Wood PJ. Hormonal modulation of human natural killer cell activity in vitro. J Reprod Immunol. (1985) 7:105–10. doi: 10.1016/0165-0378(85)90064-6
109. Kurashige T, Morita H, Ogura H, Kurashige M, Kitamura I, Kamimura O. The effects of hormone and protein increases during pregnancy on natural killer (NK) cell activity. Asia Oceania J Obstet Gynaecol. (1986) 12:403–7. doi: 10.1111/j.1447-0756.1986.tb00211.x
110. Zamorina SA, Shirshev SV, Gorbunova OL. Regulation of phenotypic maturation of intact and interleukin-2-activated NK and NKT cells by chorionic gonadotropin. Dokl Biol Sci. (2010) 435:384–6. doi: 10.1134/S0012496610060037
111. Shirshev SV, Nekrasova IV, Gorbunova OL, Orlova EG, Maslennikova IL. MicroRNA in hormonal mechanisms of regulation of NK cell function. Dokl Biochem Biophys. (2017) 474:168–72. doi: 10.1134/S160767291703005X
112. Svoboda J, Ruzickova Z, Cuchalova L, Kralickova M, Rezacova J, Vrana M, et al. Ovulation stimulation protocols utilizing GnRH-antagonist/hCG, promote cytotoxic cell populations, predominant in patients with embryo implantation complications. Neuro Endocrinol Lett. (2013) 34:249–7.
113. Kane N, Kelly R, Saunders PT, Critchley HO. Proliferation of uterine natural killer cells is induced by human chorionic gonadotropin and mediated via the mannose receptor. Endocrinology. (2009) 150:2882–8. doi: 10.1210/en.2008-1309
114. Anacker J, Segerer SE, Hagemann C, Feix S, Kapp M, Bausch R, et al. Human decidua and invasive trophoblasts are rich sources of nearly all human matrix metalloproteinases. Mol Hum Reprod. (2011) 17:637–52. doi: 10.1093/molehr/gar033
115. Pijnenborg R, Bland JM, Robertson WB, Brosens I. Uteroplacental arterial changes related to interstitial trophoblast migration in early human pregnancy. Placenta. (1983) 4:397–413. doi: 10.1016/S0143-4004(83)80043-5
116. Lash GE, Schiessl B, Kirkley M, Innes BA, Cooper A, Searle RF, et al. Expression of angiogenic growth factors by uterine natural killer cells during early pregnancy. J Leukoc Biol. (2006) 80:572–80. doi: 10.1189/jlb.0406250
117. Ashkar AA, Di Santo JP, Croy BA. Interferon gamma contributes to initiation of uterine vascular modification, decidual integrity, and uterine natural killer cell maturation during normal murine pregnancy. J Exp Med. (2000) 192:259–70. doi: 10.1084/jem.192.2.259
118. Chen Z, Zhang J, Hatta K, Lima PD, Yadi H, Colucci F, et al. DBA-lectin reactivity defines mouse uterine natural killer cell subsets with biased gene expression. Biol Reprod. (2012) 87:81. doi: 10.1095/biolreprod.112.102293
119. Ain R, Canham LN, Soares MJ. Gestation stage-dependent intrauterine trophoblast cell invasion in the rat and mouse: novel endocrine phenotype and regulation. Dev Biol. (2003) 260:176–90. doi: 10.1016/S0012-1606(03)00210-0
120. Linzke N, Schumacher A, Woidacki K, Croy BA, Zenclussen AC. Carbon monoxide promotes proliferation of uterine natural killer cells and remodeling of spiral arteries in pregnant hypertensive heme oxygenase-1 mutant mice. Hypertension. (2014) 63:580–8. doi: 10.1161/HYPERTENSIONAHA.113.02403
121. Zenclussen ML, Casalis PA, El-Mousleh T, Rebelo S, Langwisch S, Linzke N, et al. Haem oxygenase-1 dictates intrauterine fetal survival in mice via carbon monoxide. J Pathol. (2011) 225:293–304. doi: 10.1002/path.2946
122. Freitag N, Zwier MV, Barrientos G, Tirado-Gonzalez I, Conrad ML, Rose M, et al. Influence of relative NK-DC abundance on placentation and its relation to epigenetic programming in the offspring. Cell Death Dis. (2014) 5:e1392. doi: 10.1038/cddis.2014.353
123. Fu B, Zhou Y, Ni X, Tong X, Xu X, Dong Z, et al. Natural killer cells promote fetal development through the secretion of growth-promoting factors. Immunity. (2017) 47:1100–13.e6. doi: 10.1016/j.immuni.2017.11.018
124. Meyer N, Woidacki K, Maurer M, Zenclussen AC. Safeguarding of fetal growth by mast cells and natural killer cells: deficiency of one is counterbalanced by the other. Front Immunol. (2017) 8:711. doi: 10.3389/fimmu.2017.00711
125. Plaks V, Birnberg T, Berkutzki T, Sela S, BenYashar A, Kalchenko V, et al. Uterine DCs are crucial for decidua formation during embryo implantation in mice. J Clin Invest. (2008) 118:3954–65. doi: 10.1172/JCI36682
126. Barrientos G, Tirado-Gonzalez I, Freitag N, Kobelt P, Moschansky P, Klapp BF, et al. CXCR4(+) dendritic cells promote angiogenesis during embryo implantation in mice. Angiogenesis. (2013) 16:417–27. doi: 10.1007/s10456-012-9325-6
127. Fainaru O, Hantisteanu S, Rotfarb N, Michaeli M, Hallak M, Ellenbogen A. CD11c+HLADR+ dendritic cells are present in human ovarian follicular fluid, and their maturity correlates with serum estradiol levels in response to gonadotropins. Fertil Steril. (2012) 97:702–6. doi: 10.1016/j.fertnstert.2011.12.030
128. Kammerer U, Eggert AO, Kapp M, McLellan AD, Geijtenbeek TB, Dietl J, et al. Unique appearance of proliferating antigen-presenting cells expressing DC-SIGN (CD209) in the decidua of early human pregnancy. Am J Pathol. (2003) 162:887–96. doi: 10.1016/S0002-9440(10)63884-9
129. Salamone G, Fraccaroli L, Gori S, Grasso E, Paparini D, Geffner J, et al. Trophoblast cells induce a tolerogenic profile in dendritic cells. Hum Reprod. (2012) 27:2598–606. doi: 10.1093/humrep/des208
130. Du MR, Guo PF, Piao HL, Wang SC, Sun C, Jin LP, et al. Embryonic trophoblasts induce decidual regulatory T cell differentiation and maternal-fetal tolerance through thymic stromal lymphopoietin instructing dendritic cells. J Immunol. (2014) 192:1502–11. doi: 10.4049/jimmunol.1203425
131. Segerer SE, Muller N, van den Brandt J, Kapp M, Dietl J, Reichardt HM, et al. Impact of female sex hormones on the maturation and function of human dendritic cells. Am J Reprod Immunol. (2009) 62:165–73. doi: 10.1111/j.1600-0897.2009.00726.x
132. Huang J, Burke P, Yang Y, Seiss K, Beamon J, Cung T, et al. Soluble HLA-G inhibits myeloid dendritic cell function in HIV-1 infection by interacting with leukocyte immunoglobulin-like receptor B2. J Virol. (2010) 84:10784–91. doi: 10.1128/JVI.01292-10
133. Martinez FF, Knubel CP, Sanchez MC, Cervi L, Motran CC. Pregnancy-specific glycoprotein 1a activates dendritic cells to provide signals for Th17-, Th2-, and Treg-cell polarization. Eur J Immunol. (2012) 42:1573–84. doi: 10.1002/eji.201142140
134. Guo PF, Du MR, Wu HX, Lin Y, Jin LP, Li DJ. Thymic stromal lymphopoietin from trophoblasts induces dendritic cell-mediated regulatory TH2 bias in the decidua during early gestation in humans. Blood. (2010) 116:2061–9. doi: 10.1182/blood-2009-11-252940
135. Blois SM, Ilarregui JM, Tometten M, Garcia M, Orsal AS, Cordo-Russo R, et al. A pivotal role for galectin-1 in fetomaternal tolerance. Nat Med. (2007) 13:1450–7. doi: 10.1038/nm1680
136. Wan H, Versnel MA, Leijten LM, van Helden-Meeuwsen CG, Fekkes D, Leenen PJ, et al. Chorionic gonadotropin induces dendritic cells to express a tolerogenic phenotype. J Leukoc Biol. (2008) 83:894–901. doi: 10.1189/jlb.0407258
137. Yoshimura T, Inaba M, Sugiura K, Nakajima T, Ito T, Nakamura K, et al. Analyses of dendritic cell subsets in pregnancy. Am J Reprod Immunol. (2003) 50:137–45. doi: 10.1034/j.1600-0897.2003.00063.x
138. Shirshev SV, Orlova EG, Loginova OA, Nekrasova IV, Gorbunova OL, Maslennikova IL. Hormonal regulation of dendritic cell differentiation in the thymus. Bull Exp Biol Med. (2018) 165:230–4. doi: 10.1007/s10517-018-4136-4
139. Schumacher A, Heinze K, Witte J, Poloski E, Linzke N, Woidacki K, et al. Human chorionic gonadotropin as a central regulator of pregnancy immune tolerance. J Immunol. (2013) 190:2650–8. doi: 10.4049/jimmunol.1202698
140. Dauven D, Ehrentraut S, Langwisch S, Zenclussen AC, Schumacher A. Immune modulatory effects of human chorionic gonadotropin on dendritic cells supporting fetal survival in murine pregnancy. Front Endocrinol. (2016) 7:146. doi: 10.3389/fendo.2016.00146
141. Sauss K, Ehrentraut S, Zenclussen AC, Schumacher A. The pregnancy hormone human chorionic gonadotropin differentially regulates plasmacytoid and myeloid blood dendritic cell subsets. Am J Reprod Immunol. (2018) 79:e12837. doi: 10.1111/aji.12837
142. Ehrentraut S, Sauss K, Neumeister R, Luley L, Oettel A, Fettke F, et al. Human miscarriage is associated with dysregulations in peripheral blood-derived myeloid dendritic cell subsets. Front Immunol. (2019) 10:2440. doi: 10.3389/fimmu.2019.02440
143. Bell SC, Billington WD. Humoral immune responses in murine pregnancy. V. Relationship to the differential immunogenicity of placental and fetal tissues. J Reprod Immunol. (1986) 9:289–302. doi: 10.1016/0165-0378(86)90030-6
144. Busse M, Campe KJ, Nowak D, Schumacher A, Plenagl S, Langwisch S, et al. IL-10 producing B cells rescue mouse fetuses from inflammation-driven fetal death and are able to modulate T cell immune responses. Sci Rep. (2019) 9:9335. doi: 10.1038/s41598-019-45860-2
145. Mattsson R, Mattsson A, Sulila P. Allogeneic pregnancy in B-lymphocyte deprived CBA/Ca mice—effects on maternal lymphoid organs and fetal survival. Dev Comp Immunol. (1985) 9:709–17. doi: 10.1016/0145-305X(85)90035-7
146. Mattsson R, Sulila P, Bernadotte F, Mattsson A. Allopregnancy in B-cell deprived C57/BL mice—an investigation focusing on the relationship between survival of the fetuses and anti-paternal immune activity of the mothers. Dev Comp Immunol. (1988) 12:167–76. doi: 10.1016/0145-305X(88)90034-1
147. Rodger JC. Lack of a requirement for a maternal humoral immune response to establish or maintain successful allogeneic pregnancy. Transplantation. (1985) 40:372–5. doi: 10.1097/00007890-198510000-00006
148. Chakravarty EF, Murray ER, Kelman A, Farmer P. Pregnancy outcomes after maternal exposure to rituximab. Blood. (2011) 117:1499–506. doi: 10.1182/blood-2010-07-295444
149. Gotestam Skorpen C, Hoeltzenbein M, Tincani A, Fischer-Betz R, Elefant E, Chambers C, et al. The EULAR points to consider for use of antirheumatic drugs before pregnancy, and during pregnancy and lactation. Ann Rheum Dis. (2016) 75:795–810. doi: 10.1136/annrheumdis-2015-208840
150. Das G, Damotte V, Gelfand JM, Bevan C, Cree BAC, Do L, et al. Rituximab before and during pregnancy: a systematic review, and a case series in MS and NMOSD. Neurol Neuroimmunol Neuroinflamm. (2018) 5:e453. doi: 10.1212/NXI.0000000000000453
151. Rieger L, Segerer S, Bernar T, Kapp M, Majic M, Morr AK, et al. Specific subsets of immune cells in human decidua differ between normal pregnancy and preeclampsia–a prospective observational study. Reprod Biol Endocrinol. (2009) 7:132. doi: 10.1186/1477-7827-7-132
152. Arenas-Hernandez M, Sanchez-Rodriguez EN, Mial TN, Robertson SA, Gomez-Lopez N. Isolation of leukocytes from the murine tissues at the maternal-fetal interface. J Vis Exp. (2015) 99:e52866. doi: 10.3791/52866
153. Feyaerts D, Benner M, van Cranenbroek B, van der Heijden OWH, Joosten I, van der Molen RG. Human uterine lymphocytes acquire a more experienced and tolerogenic phenotype during pregnancy. Sci Rep. (2017) 7:2884. doi: 10.1038/s41598-017-03191-0
154. Medina KL, Smithson G, Kincade PW. Suppression of B lymphopoiesis during normal pregnancy. J Exp Med. (1993) 178:1507–15. doi: 10.1084/jem.178.5.1507
155. Muzzio DO, Soldati R, Ehrhardt J, Utpatel K, Evert M, Zenclussen AC, et al. B cell development undergoes profound modifications and adaptations during pregnancy in mice. Biol Reprod. (2014) 91:115. doi: 10.1095/biolreprod.114.122366
156. Muzzio DO, Ziegler KB, Ehrhardt J, Zygmunt M, Jensen F. Marginal zone B cells emerge as a critical component of pregnancy well-being. Reproduction. (2016) 151:29–37. doi: 10.1530/REP-15-0274
157. Watanabe M, Iwatani Y, Kaneda T, Hidaka Y, Mitsuda N, Morimoto Y, et al. Changes in T, B, and NK lymphocyte subsets during and after normal pregnancy. Am J Reprod Immunol. (1997) 37:368–77. doi: 10.1111/j.1600-0897.1997.tb00246.x
158. Mahmoud F, Abul H, Omu A, Al-Rayes S, Haines D, Whaley K. Pregnancy-associated changes in peripheral blood lymphocyte subpopulations in normal Kuwaiti women. Gynecol Obstet Invest. (2001) 52:232–6. doi: 10.1159/000052981
159. Lima J, Martins C, Leandro MJ, Nunes G, Sousa MJ, Branco JC, et al. Characterization of B cells in healthy pregnant women from late pregnancy to post-partum: a prospective observational study. BMC Pregnancy Childb. (2016) 16:139. doi: 10.1186/s12884-016-0927-7
160. Zimmer JP, Garza C, Butte NF, Goldman AS. Maternal blood B-cell (CD19+) percentages and serum immunoglobulin concentrations correlate with breast-feeding behavior and serum prolactin concentration. Am J Reprod Immunol. (1998) 40:57–62. doi: 10.1111/j.1600-0897.1998.tb00389.x
161. Jensen F, Muzzio D, Soldati R, Fest S, Zenclussen AC. Regulatory B10 cells restore pregnancy tolerance in a mouse model. Biol Reprod. (2013) 89:90. doi: 10.1095/biolreprod.113.110791
162. Rolle L, Memarzadeh Tehran M, Morell-Garcia A, Raeva Y, Schumacher A, Hartig R, et al. Cutting edge: IL-10-producing regulatory B cells in early human pregnancy. Am J Reprod Immunol. (2013) 70:448–53. doi: 10.1111/aji.12157
163. Fettke F, Schumacher A, Canellada A, Toledo N, Bekeredjian-Ding I, Bondt A, et al. Maternal and fetal mechanisms of B cell regulation during pregnancy: human chorionic gonadotropin stimulates B cells to produce IL-10 while alpha-fetoprotein drives them into apoptosis. Front Immunol. (2016) 7:495. doi: 10.3389/fimmu.2016.00495
164. Liu J, Chen X, Hao S, Zhao H, Pang L, Wang L, et al. Human chorionic gonadotropin and IL-35 contribute to the maintenance of peripheral immune tolerance during pregnancy through mediating the generation of IL-10(+) or IL-35(+) Breg cells. Exp Cell Res. (2019) 383:111513. doi: 10.1016/j.yexcr.2019.111513
165. Yanaba K, Bouaziz JD, Haas KM, Poe JC, Fujimoto M, Tedder TF. A regulatory B cell subset with a unique CD1dhiCD5+ phenotype controls T cell-dependent inflammatory responses. Immunity. (2008) 28:639–50. doi: 10.1016/j.immuni.2008.03.017
166. Schumacher A, Ehrentraut S, Scharm M, Wang H, Hartig R, Morse HC 3rd, et al. Plasma cell alloantigen 1 and IL-10 secretion define two distinct peritoneal B1a B cell subsets with opposite functions, PC1(high) cells being protective and PC1(low) cells harmful for the growing fetus. Front Immunol. (2018) 9:1045. doi: 10.3389/fimmu.2018.01045
167. Hever A, Roth RB, Hevezi P, Marin ME, Acosta JA, Acosta H, et al. Human endometriosis is associated with plasma cells and overexpression of B lymphocyte stimulator. Proc Natl Acad Sci USA. (2007) 104:12451–6. doi: 10.1073/pnas.0703451104
168. Erlebacher A. Immunology of the maternal-fetal interface. Annu Rev Immunol. (2013) 31:387–411. doi: 10.1146/annurev-immunol-032712-100003
169. Croy BA, Chen Z, Hofmann AP, Lord EM, Sedlacek AL, Gerber SA. Imaging of vascular development in early mouse decidua and its association with leukocytes and trophoblasts. Biol Reprod. (2012) 87:125. doi: 10.1095/biolreprod.112.102830
170. Lissauer D, Kilby MD, Moss P. Maternal effector T cells within decidua: the adaptive immune response to pregnancy? Placenta. (2017) 60:140–4. doi: 10.1016/j.placenta.2017.09.003
171. Wang S, Qian J, Sun F, Li M, Ye J, Li M, et al. Bidirectional regulation between 1st trimester HTR8/SVneo trophoblast cells and in vitro differentiated Th17/Treg cells suggest a fetal-maternal regulatory loop in human pregnancy. Am J Reprod Immunol. (2019) 81:e13106. doi: 10.1111/aji.13106
172. Saito S, Nakashima A, Shima T, Ito M. Th1/Th2/Th17 and regulatory T-cell paradigm in pregnancy. Am J Reprod Immunol. (2010) 63:601–10. doi: 10.1111/j.1600-0897.2010.00852.x
173. Piccinni MP, Lombardelli L, Logiodice F, Kullolli O, Romagnani S, Le Bouteiller P. T helper cell mediated-tolerance towards fetal allograft in successful pregnancy. Clin Mol Allergy. (2015) 13:9. doi: 10.1186/s12948-015-0015-y
174. Mor G, Aldo P, Alvero AB. The unique immunological and microbial aspects of pregnancy. Nat Rev Immunol. (2017) 17:469–82. doi: 10.1038/nri.2017.64
175. Khare P, Bose A, Singh P, Singh S, Javed S, Jain SK, et al. Gonadotropin and tumorigenesis: direct and indirect effects on inflammatory and immunosuppressive mediators and invasion. Mol Carcinog. (2017) 56:359–70. doi: 10.1002/mc.22499
176. Rayev MB, Zamorina SA, Litvinova LS, Yurova KA, Khaziakhmatova OG, Timganova VP, et al. The influence of chorionic gonadotropin on phenotype conversion and hTERT gene expression by T-lymphocytes of different degrees of differentiation. Biomed Khim. (2017) 63:539–45. doi: 10.18097/PBMC20176306539
177. Carbone F, Procaccini C, De Rosa V, Alviggi C, De Placido G, Kramer D, et al. Divergent immunomodulatory effects of recombinant and urinary-derived FSH, LH, and hCG on human CD4+ T cells. J Reprod Immunol. (2010) 85:172–9. doi: 10.1016/j.jri.2010.02.009
178. Dong M, Ding G, Zhou J, Wang H, Zhao Y, Huang H. The effect of trophoblasts on T lymphocytes: possible regulatory effector molecules—a proteomic analysis. Cell Physiol Biochem. (2008) 21:463–72. doi: 10.1159/000129639
179. Kayisli UA, Selam B, Guzeloglu-Kayisli O, Demir R, Arici A. Human chorionic gonadotropin contributes to maternal immunotolerance and endometrial apoptosis by regulating Fas-Fas ligand system. J Immunol. (2003) 171:2305–13. doi: 10.4049/jimmunol.171.5.2305
180. Shirshev SV, Orlova EG, Zamorina SA, Nekrasova IV. Influence of reproductive hormones on the induction of CD4(+)CD25 (bright)Foxp (3+) regulatory T cells. Dokl Biol Sci. (2011) 440:343–6. doi: 10.1134/S0012496611050024
181. Poloski E, Oettel A, Ehrentraut S, Luley L, Costa SD, Zenclussen AC, et al. JEG-3 Trophoblast cells producing human chorionic gonadotropin promote conversion of human CD4+FOXP3- T cells into CD4+FOXP3+ regulatory T cells and foster T cell suppressive activity. Biol Reprod. (2016) 94:106. doi: 10.1095/biolreprod.115.135541
182. Lin J, Lojun S, Lei ZM, Wu WX, Peiner SC, Rao CV. Lymphocytes from pregnant women express human chorionic gonadotropin/luteinizing hormone receptor gene. Mol Cell Endocrinol. (1995) 111:R13–7. doi: 10.1016/0303-7207(95)03565-O
183. Diao LH, Li GG, Zhu YC, Tu WW, Huang CY, Lian RC, et al. Human chorionic gonadotropin potentially affects pregnancy outcome in women with recurrent implantation failure by regulating the homing preference of regulatory T cells. Am J Reprod Immunol. (2017) 77:e12618. doi: 10.1111/aji.12618
184. Sha J, Liu F, Zhai J, Liu X, Zhang Q, Zhang B. Alteration of Th17 and Foxp3(+) regulatory T cells in patients with unexplained recurrent spontaneous abortion before and after the therapy of hCG combined with immunoglobulin. Exp Ther Med. (2017) 14:1114–8. doi: 10.3892/etm.2017.4574
185. Koldehoff M, Katzorke T, Wisbrun NC, Propping D, Wohlers S, Bielfeld P, et al. Modulating impact of human chorionic gonadotropin hormone on the maturation and function of hematopoietic cells. J Leukoc Biol. (2011) 90:1017–26. doi: 10.1189/jlb.0910520
186. Schumacher A, Brachwitz N, Sohr S, Engeland K, Langwisch S, Dolaptchieva M, et al. Human chorionic gonadotropin attracts regulatory T cells into the fetal-maternal interface during early human pregnancy. J Immunol. (2009) 182:5488–97. doi: 10.4049/jimmunol.0803177
Keywords: human chorionic gonadotropin, uterine immune cells, embryo implantation, placentation, fetal tolerance, pregnancy
Citation: Schumacher A and Zenclussen AC (2019) Human Chorionic Gonadotropin-Mediated Immune Responses That Facilitate Embryo Implantation and Placentation. Front. Immunol. 10:2896. doi: 10.3389/fimmu.2019.02896
Received: 04 November 2019; Accepted: 26 November 2019;
Published: 10 December 2019.
Edited by:
Julia Szekeres-Bartho, Medical School, University of Pécs, HungaryReviewed by:
Nandor Gabor Than, Hungarian Academy of Sciences (MTA), HungaryGerard Chaouat, INSERM U976 Immunologie, Dermatologie, Oncologie, France
Copyright © 2019 Schumacher and Zenclussen. This is an open-access article distributed under the terms of the Creative Commons Attribution License (CC BY). The use, distribution or reproduction in other forums is permitted, provided the original author(s) and the copyright owner(s) are credited and that the original publication in this journal is cited, in accordance with accepted academic practice. No use, distribution or reproduction is permitted which does not comply with these terms.
*Correspondence: Anne Schumacher, YW5uZS5zY2h1bWFjaGVyQG1lZC5vdmd1LmRl