- 1Millennium Institute on Immunology and Immunotherapy, Departamento de Genética Molecular y Microbiología, Facultad de Ciencias Biológicas, Pontificia Universidad Católica de Chile, Santiago, Chile
- 2Departamento de Biotecnología, Facultad de Ciencias del Mar y Recursos Biológicos, Universidad de Antofagasta, Antofagasta, Chile
- 3Sección de Biotecnología, Instituto de Salud Pública de Chile, Santiago, Chile
- 4Facultad de Medicina y Ciencia, Universidad San Sebastián, Providencia, Santiago, Chile
- 5Millennium Institute on Immunology and Immunotherapy, Departamento de Ciencias Biológicas, Facultad de Ciencias de la Vida, Universidad Andrés Bello, Santiago, Chile
- 6Departamento de Endocrinología, Escuela de Medicina, Facultad de Medicina, Pontificia Universidad Católica de Chile, Santiago, Chile
The Bacillus Calmette-Guérin (BCG) is a live attenuated tuberculosis vaccine that has the ability to induce non-specific cross-protection against pathogens that might be unrelated to the target disease. Vaccination with BCG reduces mortality in newborns and induces an improved innate immune response against microorganisms other than Mycobacterium tuberculosis, such as Candida albicans and Staphylococcus aureus. Innate immune cells, including monocytes and natural killer (NK) cells, contribute to this non-specific immune protection in a way that is independent of memory T or B cells. This phenomenon associated with a memory-like response in innate immune cells is known as “trained immunity.” Epigenetic reprogramming through histone modification in the regulatory elements of particular genes has been reported as one of the mechanisms associated with the induction of trained immunity in both, humans and mice. Indeed, it has been shown that BCG vaccination induces changes in the methylation pattern of histones associated with specific genes in circulating monocytes leading to a “trained” state. Importantly, these modifications can lead to the expression and/or repression of genes that are related to increased protection against secondary infections after vaccination, with improved pathogen recognition and faster inflammatory responses. In this review, we discuss BCG-induced cross-protection and acquisition of trained immunity and potential heterologous effects of recombinant BCG vaccines.
Introduction
One of the leading causes of human death worldwide is tuberculosis (TB), a bacterial infection caused by Mycobacterium (M.) tuberculosis. In 2017, 10 million people developed TB disease, causing 1.3 million deaths (1). To prevent TB, a vaccine was developed in 1921 by Albert Calmette and Camille Guérin, which is currently included in the immunization programs of most countries. This vaccine consists of an attenuated M. bovis bacillus that was repeatedly passaged in culture by Calmette and Guérin and which is known as the bacillus Calmette-Guérin (BCG) (2). This vaccine was developed from a virulent M. bovis strain that accumulated more than 14 genome deletions in different regions (3). In most countries, BCG is administered to newborns a few hours or days after birth and its protective effects against tuberculous meningitis and miliary tuberculosis (TB) have shown efficacy over 70%, while its protective effect against pulmonary TB displays an average of 52% of protection (4–7). Mathematical estimations suggest that BCG vaccination of 100.5 million children, out of the 132.8 million children born in the world, prevented nearly 30,000 cases of TB meningitis and 11,500 cases of miliary TB in the year 2002 (6). In adults, BCG vaccination fails to completely protect against pulmonary TB, showing a range of effectiveness between 0 and 80% (8–10), which explains why TB is one of the major causes of mortality worldwide (1). Despite this, in 2018 BCG was considered within the national vaccination program of 154 countries, including countries in America, Asia, Africa, and Europe, with coverage of over 90% (1). It was also administered to high-risk groups in additional countries, being one of the most widely used vaccines worldwide (1, 11). Besides protecting against TB, BCG vaccination also reduces mortality in children because of non-specific cross-protection induced by this vaccine against other unrelated pathogens (12, 13). Initial evidence for this phenomenon was described in Sweden in 1927 by the physician Carl Näslund, who found that during the first year of life, BCG-vaccinated newborns had a mortality rate that was three times lower than unvaccinated babies (14). This observation was also made by Albert Calmette, in 1931 (15). In Guinea-Bissau, a country with a high childhood mortality rate, the presence of a BCG-vaccination scar was associated with diminished mortality rates associated with malaria or unclassified fever (16). Besides, BCG-vaccinated children showed a reduced risk of developing acute lower respiratory tract infections (ALRI) as compared with non-vaccinated ones (17). Furthermore, several studies carried out in West Africa showed over a 40% reduction in mortality after BCG vaccination, preventing malaria, sepsis, respiratory infections, and leprosy (14, 16, 18–21). In Spain, BCG vaccination reduced hospitalizations due to respiratory infections unrelated to TB in children under 14 years of age (13). Also, reduced child mortality due to BCG vaccination has been observed in other places of the world, including Sweden, United Kingdom, South or Southeast Asia, India, and Haiti (22–24).
Another remarkable characteristic of BCG is that it can be used as an expression vector for recombinant antigens to develop novel vaccines for pathogenic bacteria and viruses (25–34), as well as for cancer diseases (35–43). BCG has been considered as a good vector given its safety shown in vaccinated neonates, children and adults for almost a 100 years and that BCG antigens may act as adjuvants, inducing innate and adaptive immune responses (11, 22–24, 44, 45).
Immune Response Induced by BCG Vaccination
The immune response elicited after BCG vaccination begins at the inoculation site after intradermal injection, where resident neutrophils, macrophages, and dendritic cells (DCs) interact with the bacillus (44, 46). The recognition of BCG by immune cells takes place through the interaction of different pattern recognition receptors (PRRs) with pathogen-associated molecular patterns (PAMPs), such as peptidoglycan, arabinogalactan, and mycolic acids located at the bacterium cell wall (44). Among the receptors involved in the recognition of BCG are toll-like receptors (TLRs) TLR2 and TLR4 present on the cell surface membrane (44). It has been shown that different proteins expressed by mycobacteria can work as TLR agonists, stimulating macrophage, and DC maturation and the secretion of pro-inflammatory cytokines (47). Likewise, complement receptors CR3 and CR4 are involved in the recognition of opsonized mycobacteria by DCs. Another group of cell receptors that recognize BCG PAMPs are nucleotide-binding oligomerization domain (NOD)-like receptors found in the cytosol of innate immune cells, such as NOD2, which interact with a specific component of the bacterial peptidoglycan (48). Besides, C-type lectins, such as DC-specific intercellular adhesion molecule-3-grabbing nonintegrin (DC-SIGN) interact with components of the bacterial wall and are involved in the recognition and internalization of BCG (48). After internalization by DCs, the mycobacterium can live up to 2 weeks inside these cells (49). This interaction induces DC maturation and migration that is characterized by an increase in the expression of co-stimulatory molecules, such as CD40, CD80, CD83, and CD86 (50). One of the antigens present in the cell wall of BCG corresponds to antigen (Ag) 85 (also present in M. tuberculosis), which stimulates the production of tumor necrosis factor-alpha (TNF-α), interleukin 1-beta (IL-1β) and IL-6 (51, 52), which are able to generate an pro-inflammatory state that promotes the activation of immune cells (50).
The development of an adaptive immune response starts when antigen-presenting cells (APCs, e.g., DCs, macrophages and B cells) present antigenic peptides on MHC molecules and prime T cells located at the nearest secondary lymphoid tissues or the spleen (53). In vitro and in vivo studies have shown that BCG-infected skin DCs migrate to the draining lymph nodes where they secrete TNF-α, IL-6, and IL-12 and activate both, CD4+ and CD8+ T cells (54–57) (Figure 1). Interestingly, it has been reported that BCG-infected human neutrophils cooperate with infected DCs to stimulate antigen-specific T cell responses (58).
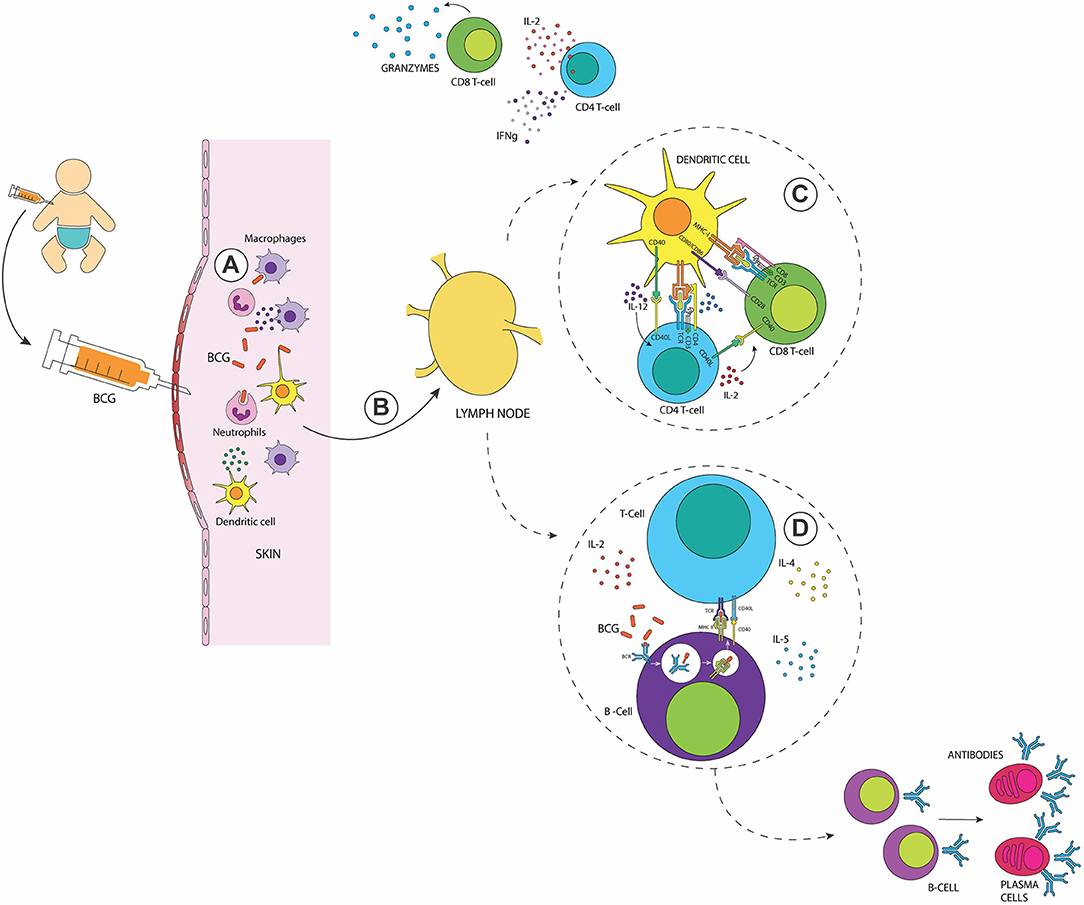
Figure 1. The immune response elicited after BCG vaccination in the newborn. (A) Recognition of the BCG at the inoculation site by neutrophils, macrophages, and DCs. (B) Activated skin DCs migrate to the draining lymph nodes to activate adaptive immune cells (C) Activation of Mycobacteria-specific CD4+ and CD8+ T cells with a TH1 profile, secreting elevated amounts of IFN-γ and granzymes (D) Activation of B cells leads to the generation of memory and plasma cells and the production of antigen-specific antibodies in response to the presence of antigens of BCG. After their activation, memory T and B cells reside in lymph nodes.
As summarized in Figure 1, the adaptive immune response induced after BCG vaccination involves the activation of both, CD4+ and CD8+ T cells (53, 59) with elevated production of IFN-γ, which increases the anti-mycobacterial activity of macrophages (52, 53). This cytokine also contributes to the activation of B cells and the subsequent generation of antigen-specific antibodies by plasma cells. In early stages after vaccination, a pool of mycobacteria-specific CD8+ T cells proliferates and is present in peripheral blood up to 10 weeks after BCG vaccination (60). These CD8+ T cells were able to secrete IFN-γ and express granzymes, as well as perforins supporting the cytotoxic potential for these cells (60, 61). Activated TH1 CD4+ T cells have also been detected (62, 63), which produce large amounts of IFN-γ, TNF-α, and IL-2 (55, 64). In newborns, BCG-specific CD4+ T cells could be detected in the peripheral blood 3 weeks after vaccination, with a peak at 10 weeks (60). Studies with T cells transferred from BCG-vaccinated mice into animals that are deficient for both B and T cells have shown that CD4+/CD8+ T cells are necessary for reducing and controlling bacterial dissemination (65). During the contraction phase, BCG-specific CD4+ and CD8+ T cells switch to a memory phenotype, with functional features of effector memory T cells secreting IFN-γ (64, 66). These memory T cells generate a strong lymphoproliferative response to TB antigens several months after vaccination in mice (66).
Between 4 and 8 weeks after BCG vaccination, there is an induction of a B cell response that increases the production of IgG (67) and induces long-lived memory B cells (68). These IgG molecules can opsonize BCG and M. tuberculosis, enhancing phagocytosis and the inhibition of intracellular bacterium growth (67). It was shown that mucosal BCG vaccination induces an airway-resident memory T cell population in the lungs, but immunoglobulin production was not measured in this study (69). Pulmonary immune response in mice infected with M. tuberculosis was improved when BCG was administered intranasally as compared to subcutaneous vaccination (70). This improved protective efficacy was concordant with an increased presence of IgA and CD4+IL-17A+ T cells in bronchoalveolar lavages of intranasally vaccinated mice (70). Although subcutaneous BCG vaccination enhances blood IgG levels, in the case of respiratory airway pathogens, IgA confers better protection against infections (70). This is due to the fact that IgA is constitutively found in serum and mucosa and corresponds to one of the first barriers of defense (71). These antibodies can neutralize, excrete pathogens and activate the immune response by the modulation of the secretion of cytokines, such as TNF-α and IL-1β (71).
BCG Vaccine As a Strategy for Modulating Immunity
Besides protection against TB, BCG has other clinical applications, especially in two main immunotherapy fields: treatments for cancer and autoimmune diseases, such as melanoma and type-1 diabetes (T1D), respectively (we summarize the contribution of BCG to immunotherapy in Figure 2). T1D is an autoimmune disease characterized by the destruction of pancreatic beta cells (72). This destruction leads to a lack of insulin production, which leads to the development of hyperglycemia, polyuria, and hypoinsulinemia (73). The effect of BCG vaccination in T1D is still controversial, as it was originally observed that BCG vaccination promoted a remission of the disease when patients were treated during the first month after diagnosis (74). On the other hand, a randomized clinical trial performed in 1999 where patients between 5 and 18 years old were vaccinated early after disease appearance showed no difference in glycated hemoglobin levels (HbA1c, an indicator of blood glucose levels) or endogenous insulin secretion compared to non-vaccinated ones (75). In a phase I trial performed in adults with long-term T1D, BCG vaccination in multiple doses was able to reduce HbA1c levels and increased the death of insulin-autoreactive T cells (76). Interestingly, BCG modulation of blood sugar was associated with a systemic shift toward a glycolytic pathway of glucose utilization (76). Another clinical trial performed with long-term T1D patients showed that vaccination with BCG stabilized HbA1c levels without producing hypoglycemia, an effect that could last up to 8 years after vaccination (77). In non-obese diabetic (NOD) mice, injection of BCG was shown to reduce insulitis and diabetes development (78). It has been demonstrated that the stimulation of TNF-α induced by BCG is involved in the destruction of insulin-autoreactive T cells (79). Despite this, the mechanism involved in the immunomodulatory effect induced by BCG in T1D patients has not yet been elucidated.
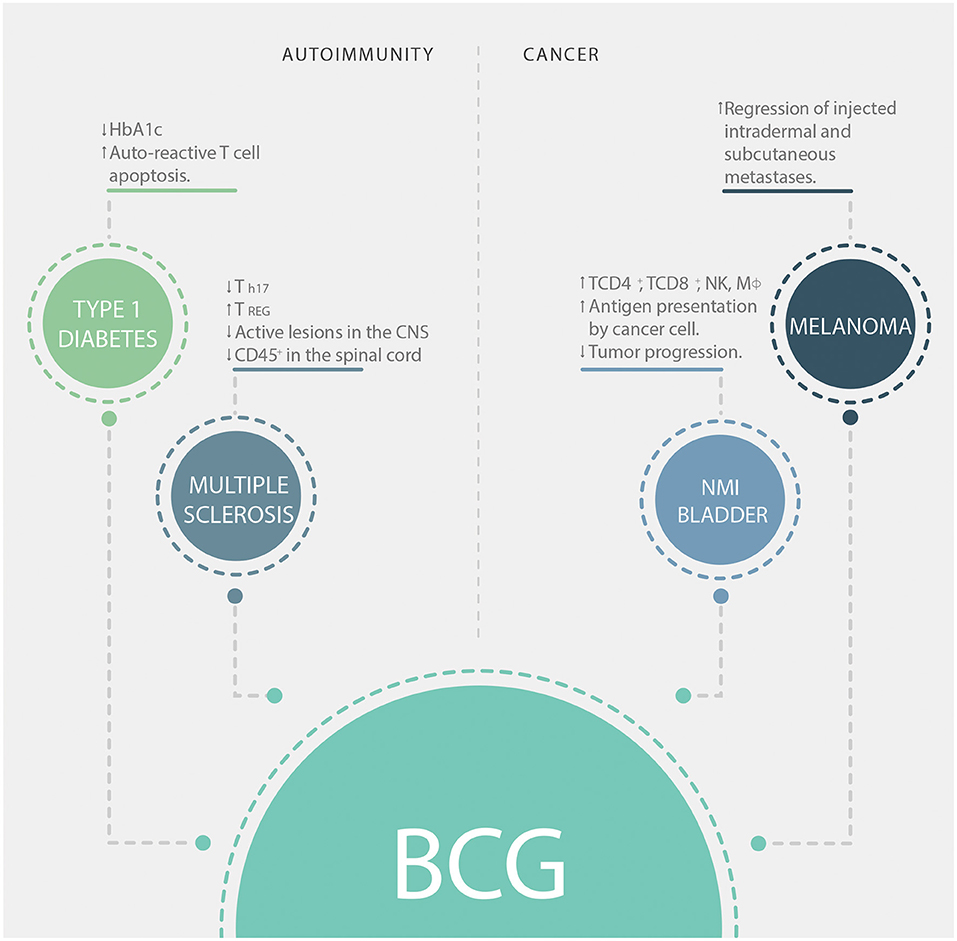
Figure 2. BCG applications in immunotherapy. Clinical applications of BCG in autoimmunity (left boxes) and cancer (right boxes). HbA1c, glycosylated hemoglobin; CNS, central nervous system; NK, natural killer cells; Mϕ, macrophages; NMI, non-muscle invasive.
Another important autoimmune disease is multiple sclerosis (MS), which is characterized by the development of neurological symptoms due to gradual demyelination of the central nervous system (CNS) as a consequence of an inflammatory-autoimmune response (80). At present, a specific treatment for this disease that is effective is not available. Nonetheless, clinical trials showed that BCG vaccination could reduce the frequency of active lesions in the CNS of 12 MS patients (81). Further, a phase II clinical trial showed that after the first demyelinating episode, BCG vaccination reduced the risk of developing clinically definite MS for 5 years (82). Moreover, in a widely used mouse model of MS named experimental autoimmune encephalomyelitis (EAE), the injection of subcutaneous extended freeze-dried (EFD) BCG attenuated the severity of EAE (83). Mice treated with EFD BCG showed significantly lower clinical scores and reduced infiltration of CD45+ cells in the spinal cords (83). Furthermore, this treatment was shown to reduce the frequency of TH17 cells and to increase the frequency of TREG cells in secondary lymph nodes. This consequence will help to limit the inflammation induced by EFD BCG in EAE (83).
Since several studies have demonstrated that BCG induces TH1/TH17 responses against TB and other unrelated pathogens (62, 84, 85), its capacity to exert a regulatory effect over autoimmune diseases, such as T1D and MS is very surprising. However, there are immune-metabolic pathways involved in the activation of the immune system after BCG vaccination that may account for explanations of these observations. Indeed, the activation of innate immune cells and T cells induced by this vaccine is partly mediated by the activation of cell glycolytic pathways (86). Besides, human Treg cells are highly glycolytic (87). Based on these findings, Ristori et al. proposed in 2018 that BCG induces a tolerogenic response via enhancement of glycolysis, contributing to the reduction of inflammation in autoimmune diseases (88). Another possible mechanism through which BCG can mediate protection in the context of autoimmune diseases relays on the immune response to the infection with the mycobacterium. After infection, it has been shown that activated, but not naïve, CD4+ T cells undergo apoptosis in an IFN-γ-dependent manner (89). Thus, apoptosis of activated T cells may have as a consequence the diminution of activated autoreactive cells, improving the health condition of the individual receiving vaccination. Also, TLR-signaling stimulated by mycobacterial components induces IL-10 secretion by B cells and consequent suppression of Th1 and Th17 activities, contributing to the suppression of autoimmune reactions (90).
On the other hand, there is increasing evidence for the use of BCG vaccination for the prevention and treatment of cancer (35–43). Vaccination of newborns with BCG reduces the risk of developing melanoma (35) and childhood leukemia (36). In the case of melanoma, direct vaccination with BCG into nodules of intradermal or subcutaneous metastases induced their regression in 90% of the injected lesions (37). As previously described, BCG exposure of tumoral macrophages induces transcriptional reprogramming of these cells, leading to an improved pro-inflammatory phenotype (91). BCG-treated macrophages can induce the activation of T cells infiltrating the tumor, thus improving antitumor immunity (91). Moreover, in patients with non-muscle invasive bladder cancer, it has been shown that BCG instillation after transurethral resection reduces tumor progression (38, 92). BCG impairs the tolerogenic milieu developed by carcinogenic cells, inducing local infiltration of macrophages, CD4+ T helper cells, CD8+ T cells, and NK cells, resulting in the development of local inflammation (40, 41, 93). Bladder tumor cells express antigen-presenting and co-stimulatory molecules after being infected with BCG, suggesting that these cells can function as antigen-presenting cells making them a good target to be destroyed by cytotoxic cells (42). The antitumor effects of BCG immunization could also be associated with the development of local inflammation that might overcome the tolerogenic environment induced by tumor cells (43).
The different effects observed after the administration of the BCG vaccine suggest that the activation of the immune system induced by it could vary depending on the environment in which it is found. BCG administration could exert a beneficial modulation of the immune system in the context of autoimmune diseases due to a redirection of the inflammatory response. In the case of cancer, the activation of the immune system in the presence of the bacteria within the tumor alters the tolerogenic environment induced by cancer cells, leading to a specific cytotoxic activity against the tumor cells.
Trained Immunity As a Consequence of BCG Vaccination
Netea et al. (94) were the first to propose the concept of “trained immunity,” which is defined as an increased non-specific response to a secondary infection mediated by the innate immune system, either to the same or different microorganisms (94). This type of immunity is characterized as being independent of T and B cell responses and is mediated by monocytes/macrophages and NK cells (95).
In humans, BCG vaccination of adults induces a trained phenotype in circulating monocytes, characterized by an increased capacity to produce proinflammatory cytokines, an effect that has translated to non-specific protection against unrelated pathogens, such as S. aureus and C. albicans (96, 97). Also, BCG vaccination of healthy human volunteers increased the capacity of NK cells to secrete proinflammatory cytokines, such as IL-1β and IL-6 after stimulation with M. tuberculosis or unrelated pathogens (S. aureus, C. albicans) (98). These observations were performed 3 months after vaccination, consistent with the fact that BCG reduces mortality in newborns during the first year of life, as mentioned above. Thus, BCG induces non-specific protection against unrelated pathogens (96).
Interestingly, innate immune cells mediate this nonspecific protection, independent of T and B cells. A comparison of systemic lethal candidiasis infection in severe combined immunodeficiency (SCID) mice, which lack T and B cells, and NOD/SCID/IL2Rγ (NSG) mice that lack T, B and, NK cells, showed that partial protection was mediated by NK cells in BCG-vaccinated mice (98). Specifically, mice were challenged with a lethal intravenous dose of C. albicans 2 weeks after BCG vaccination; while SCID vaccinated mice survived, NSG vaccinated mice were partially protected, suggesting a role for NK cells in the non-specific protective effect induced by BCG vaccination (98).
Besides, there is in vitro and in vivo evidence of BCG-related trained immunity effects in bovine monocytes (99). In vitro exposure of calf monocytes to BCG leads to enhanced TNF-α and IL-6 production after subsequent TLR agonist stimulation. Aerosol BCG vaccination of calves exerted a similar effect of PBMCs, boosting pro-inflammatory cytokine production, in association with a shift to anaerobic glycolysis (99). The trained immunity phenotype was also observed testing PBMCs obtained 3 months after vaccination (99), which further supports previous studies carried out in human cells.
It has been shown that one of the molecular mechanisms that induces the development of trained immunity is epigenetic reprogramming, specifically through histone modifications (95). Epigenetic modifications regulate gene expression in response to environmental signals (100). In the immune system, epigenetic modifications are involved in cell differentiation, inflammation and autoimmune diseases (96, 100–103). Different types of epigenetic modifications have been described, including DNA modifications, non-coding RNAs, histone modifications and chromatin remodeling (100). Histone modifications are highly dynamic and can change within minutes; there are different classes of modifications such as acetylation, methylation, phosphorylation, ubiquitylation, sumoylation, ADP ribosylation, deimination, and proline isomerization (104). Histones can be methylated at arginine or lysine residues. Lysine can accept up to three methyl groups, being mono-, di- or trimethylated. Arginine can be mono- or dimethylated (105). These modifications are involved in the activation or repression of the transcription of genes that they are associated with. While methylation of lysine 4 in histone 3 (H3K4), H3K36, and H3K79 are usually associated with transcription activation; methylation in H3K9, H3K27, and H4K20 are associated with gene silencing (105).
After BCG vaccination, peripheral blood monocytes show an increase in H3K4me3 histone modification associated with the promoters of the genes tnfα, il6, and tlr4 that lead to the transcriptional activation of these proinflammatory cytokines (96, 106). These responses are dependent on the nucleotide-binding oligomerization domain 2 (NOD2) receptor present in monocytes and receptor-interacting protein kinase 2 (Rip2) (96). These epigenetic modifications upregulated the expression of pattern recognition receptors (PPRs), namely TLRs, C-type lectins receptors, NOD-like receptors, and RIG-I-helicases that specifically recognize pathogen-associated molecular patterns (PAMPs) and modulated the accessibility of transcription factors to proinflammatory cytokine genes (96). Consequently, when these trained monocytes are exposed to a second infection, the pathogen is recognized by PPRs, leading to increased cytokine production (95).
In addition to epigenetic reprogramming, different cellular metabolic pathways are involved in the regulation and development of trained immunity in monocytes, macrophages and NK cells (86, 107–109). Indeed, glycolysis metabolism is increased in human monocytes after BCG vaccination, leading to a shift in the metabolic programming of the cell from oxidative phosphorylation to aerobic glycolysis (Warburg effect) (108). Additionally, it has been demonstrated that inhibition of the glycolytic pathway impairs the development of a trained immunity phenotype by preventing epigenetic rearrangements (86). Specifically, it was shown that glycolysis inhibits epigenetic modifications at the promoters of genes encoding for IL-6 and TNF-α in peripheral monocytes (86). Glutaminolysis and cholesterol synthesis have also been involved in the development of trained immunity in monocytes, being fumarate a key metabolite that can induce chromatin rearrangements. This metabolite induces an increase in H3K4me3 in the promoters of tnfa and il6 leading to elevated secretion of these cytokines upon re-stimulation with LPS (107). Additionally, accumulation of mevalonate, a metabolite of the cholesterol synthesis pathway, has also been shown to be able to induce trained immunity through the enrichment of H3K4me3 in the promoters of tnfa and il6 (109). All these reports support the notion that epigenetic regulation is intimately related and coordinated with the metabolic state of the cell.
Furthermore, all-trans retinoic acid (ATRA) is a vitamin A metabolite involved in the development of tolerogenic immunity (110). This metabolite regulates tolerogenic cytokine production and cell differentiation in monocytes, macrophages, DCs and T cells (111). In vitro stimulation of BCG trained monocytes with ATRA inhibits H3K4me3 and induces a strong repressive hallmark (H3K9me) in the promoters of proinflammatory genes such as tnfα, il6, il8, il10, and il1ra. Consequently, the transcription of these genes is silenced, inhibiting the “trained” phenotype (111).
BCG-induced epigenetic reprogramming of monocytes was shown to be able to protect humans against an experimental yellow fever virus (YFV) challenge (112). BCG-vaccinated subjects showed lower viremia after infection with an attenuated YFV vaccine strain. Interestingly, trained immunity induced by BCG was modulated by IL-1β treatment in vitro, and cytokine production was increased after vaccination (112). These changes in cytokine secretion were mediated by an increase in H3K4me3 and reduction of H3K9me3 in the promoter regions of the genes tnfα, il6, and il1β (112).
The genetic reprogramming described above could be implicated in the generation of memory-like innate immune cells (94). In this context, after BCG vaccination, innate immune cells, such as monocytes would undergo a series of chromatin modifications (84, 86, 96). These chromatin rearrangements would lead to a “trained phenotype” that would generate an enhanced innate response when exposed to any non-specific pathogen (Figure 3). Interestingly, chromatin rearrangements induced by BCG vaccination can reprogram bone marrow progenitors, stimulating myelopoiesis, and generating trained immune cells with a higher capacity to protect against a wide variety of pathogens (113, 114). These characteristics of trained immunity suggest that innate immune cells could be a different target for vaccination. As reviewed by Khader et al., vaccination against M. tuberculosis with BCG could be directed to the generation of trained hematopoietic progenitors and, in combination with classic vaccination for adaptive immunity generation, generate a greater and more effective immune response (115).
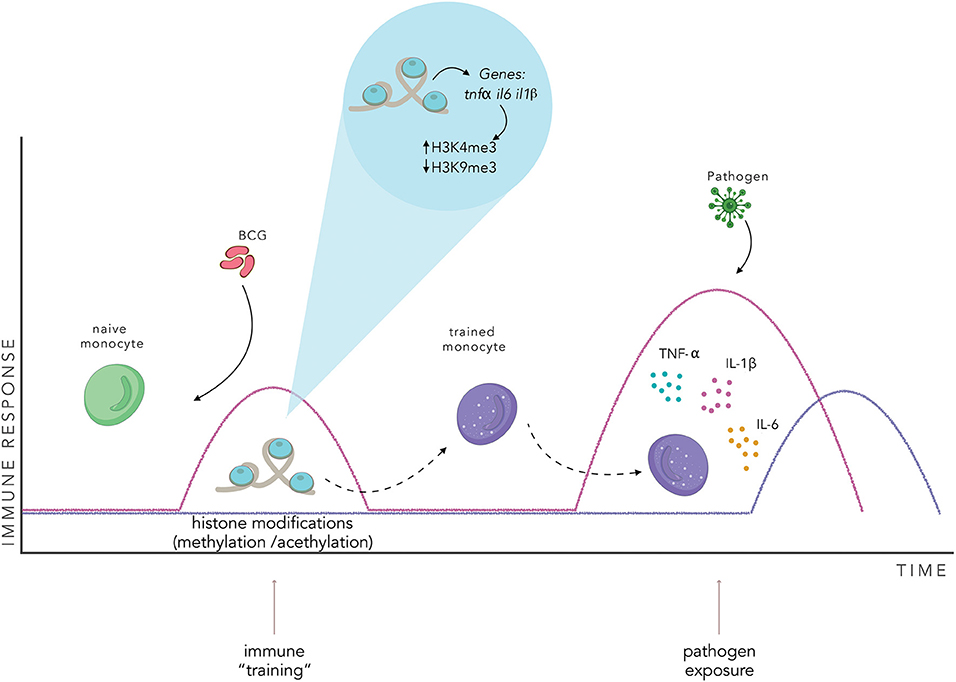
Figure 3. BCG vaccination induces an innate immune training. BCG vaccination activates the innate immune system and induces changes in the pattern of histone modifications of specific genes in innate immune cells. This chromatin rearrangement induces a “trained” state in the cell, enhancing the effectiveness of the innate immune response when exposed to a non-specific pathogen, inducing the secretion of proinflammatory cytokines, such as TNF-α, IL-1β, and IL-6. The pink line represents a trained immune response, the purple line represents a naïve innate immune response.
Recombinant BCG Vaccines
BCG has been considered a good expression vector for recombinant antigens due to several advantages. First, BCG administration is safe for neonates, infants, and adults. Second, BCG doses are relatively easy and non-expensive to produce, allowing for mass-production, and furthermore, it is temperature stable (45). Finally, BCG acts as auto-adjuvant and induces innate and adaptive immune responses (44). Several recombinant BCG (rBCG) strains that express heterologous antigens of different pathogens have been developed and tested since 1991 (25–34). These rBCGs formulations are excellent vaccine candidates, due to the auto-adjuvant characteristics conferred by BCG antigens.
In a mouse model of measles virus (MV) infection, rBCG expressing a nucleocapsid (N) protein of MV significantly reduced viral titers in brain homogenates and mortality due to measles-induced encephalitis (26). Splenocytes from vaccinated mice showed stronger proliferation of antigen-specific T cells in response to MV in vitro and an increase of serum MV-specific antibodies as compared to BCG-WT-vaccinated animals (26). This recombinant vaccine was later tested in infant rhesus macaques challenged with an intranasal inoculation of MV (116). Specifically, a total of eight newborn rhesus macaques received the rBCG-MV-N vaccine with no adverse effects, thereby demonstrating its safety. Moreover, rBCG-MV-N vaccination did not induce an increase in antigen-specific antibody titers or expansion of B cell follicles in lymph nodes. The determination of nasopharyngeal viral loads did not show significative differences in the vaccinated groups. Despite this, rBCG-MV-N vaccinated monkeys showed reduced lung pathology after viral challenge in comparison to those vaccinated with WT-BCG and paracortical hyperplasia in lymph nodes, suggesting that protection was mediated by specific T cells (116). Although vaccination with this rBCG was not able to prevent systemic infection, the reduction of lung pathology may prevent MV-associated deaths.
Toxoplasma gondii (T. gondii) is an intracellular protozoa parasite that infects a variety of warm-blooded animals and is one of the most prevalent human infections worldwide (117). Most immunocompetent humans do not develop clinical signs after acquired infection, but immunocompromised individuals are at risk of developing serious complications, including fatal ones (117). A rBCG expressing rhoptry protein 2 (ROP2), which is a protein of the T. gondii involved in host cell invasion induces antigen-specific immune responses in mice (27). Vaccination of mice with a rBCG expressing ROP2 induced the production of specific antibodies, which were detected in serum and induced cellular immune responses (27). Besides, delayed mortality was seen after infection with T. gondii in immunized mice (27).
Bordetella pertussis is a gram-negative coccobacillus that causes whooping cough (118). This disease causes serious complications including secondary bacterial pneumonia, apnea, bradycardia, pulmonary hypertension, and even death in infants younger than 6 months old (118). An rBCG expressing the S1 subunit of the B. pertussis toxin (dPT) was developed and tested as a vaccine in animals (119). Vaccination of mice showed that this vaccine was able to induce the secretion of antigen-specific antibodies and protect mice against a challenge with a lethal dose of B. pertussis (119–121). Stimulation of splenocytes of immunized mice with dPT antigen showed an increased capacity to secrete IFN-γ compared to the non-immunized group. Vaccination of 5-day old mice with the rBCG-S1PT vaccine protected them after a lethal dose challenge with B. pertussis, showing 100% survival, while non-vaccinated mice had 0% survival 8 days after challenge (121).
Human immunodeficiency virus (HIV) causes acquired immune deficiency syndrome (AIDS) in humans. According to the WHO, this syndrome caused 1.2 million deaths globally in 2016 (122). There is no effective vaccine preventing infection with this virus. Yet, there are many groups all over the world working on the development of an effective vaccine against this virus (28, 29, 31, 45, 123–127). The development of rBCGs expressing HIV antigens has been considered an interesting immunization strategy for a vaccine against HIV. An rBCG expressing the Env protein of the viral capsid of HIV has been shown to develop TH1 responses in mice (28). However, it was unable to induce the production of HIV-specific antibodies (28). An rBCG expressing viral antigens used in combination with a booster of viral vectors was also able to induce HIV-specific T cell responses in mice, which was characterized by the secretion of IFN-γ and a TH1 polarization (29). rBCG-HIVA, a recombinant BCG that expresses the H and P epitopes of the Env protein and viral polymerase respectively, has been shown to induce the activation of HIV-specific T cells in mice (30). This vaccine in combination with a recombinant viral vector induced robust T cell responses against HIV and M. tuberculosis (31). Although these are promising preclinical results, clinical trials must be done to evaluate their efficacy and protection in humans.
The human metapneumovirus (hMPV) is the second major cause of acute lower respiratory tract infections in children and the elderly. This viral infection induces inflammation and disruption of the lung architecture, causing bronchiolitis and pneumonia (128). There is no effective vaccine available to prevent infection with hMPV. However, a novel vaccine has been developed with a rBCG expressing the phosphoprotein (P) protein of hMPV (rBCG-P-hMPV) (32). This rBCG-P-hMPV formulation induces a humoral response against hMPV and can induce viral neutralization and confer protection against hMPV-infection, lowering the amounts of viral particles in the lungs in vaccinated mice (32, 129). Vaccination with rBCG-P-hMPV also reduced T cell infiltration and tissue damage in a mouse model of infection with hMPV, activating a TH1-type response and preventing the development of the disease (34).
Another relevant respiratory pathogen is the human orthopneumovirus (previously named human respiratory syncytial virus, hRSV), which is one of the leading causes of acute lower respiratory tract infections in the world (130). In 2015, hRSV caused over 33 million episodes of acute lower respiratory tract infections worldwide, being one of the major causes of hospitalization in children under 5 years of age (131). A rBCG vaccine has been developed expressing the nucleoprotein (N) of the hRSV (rBCG-N-hRSV) (32). In a mice preclinical model, the immunization with rBCG-N-hRSV confers protection against hRSV challenge, reducing both clinical pathology and neutrophil infiltration in the lungs (129). Noteworthy, this vaccine induces the secretion of viral-specific antibodies with neutralizing activity (32), which correlates with lower amounts of viral titers in the lungs in vaccinated mice (129). The rBCG-N-hRSV vaccine has been formulated under good manufacture practices (cGMP) and shown to maintain its promising results in pre-clinical trials. Immunization of mice with rBCG-N-hRSV induces a TH1/TH17 memory response that was capable of mediating virus clearance and avoiding lung damage (129).
Even though these rBCG strains have shown promising results and protection against their target pathogens, no reports of cross-protection against unrelated pathogens have been published yet. Also, it remains unclear if they maintain the benefits of cross-protection granted by the wild-type strain. Nevertheless, it appears that the trained immunity induced by BCG vaccination could represent additional protection against the pathogen, whose antigen must be expressed by the rBCG. Interestingly, several reports have shown that there is some degree of protection induced by WT-BCG vaccination against other pathogens (95, 96, 106, 112, 132). In the case of T. gondii, the determination of IFN-γ secretion by splenocytes stimulated with recombinant ROP2 showed an increased capacity of cells from mice vaccinated with WT-BCG compared to non-vaccinated animals (27), suggesting non-specific protection by WT-BCG against toxoplasmosis. For B. pertussis, it is worth noting that splenocytes obtained from mice vaccinated with WT-BCG showed increased IFN-γ secretion after stimulation with dPT as compared to the non-immunized group (121). Surprisingly, WT-BCG vaccinated mice exhibited 80% of survival after a lethal dose challenge, suggesting that the non-specific protection induced by this vaccine can exhibit a protective effect on B. pertussis infections and consequently against whooping cough (121). Also, WT-BCG decreases some disease parameters in the mouse models for hRSV and hMPV infection (32). However, the non-specific protection induced by the wild-type vaccine was lower compared to the protection induced by the rBCG expressing hRSV and hMPV antigens, respectively. Mice immunized with the WT-BCG showed intermediate values of viral gene copies and infiltrating neutrophils in the lungs between those that were not immunized and those immunized with the specific rBCG vaccines (rBCG-N-hRSV, and rBCG-P-hMPV) (32). These results support the existence of trained immunity as a consequence of BCG vaccination. The development of a trained innate immune response after rBCG vaccination might be an advantageous scenario for preventing infections, due to the enhanced immune response elicited after immunization against homologous and heterologous pathogens.
Discussion and Future Challenges
The BCG vaccine has been used in humans for almost a 100 years, proving its immunogenicity and safety. However, it is still unclear whether recombinant BCGs can induce trained immunity after vaccination. Furthermore, the use of the BCG vaccine as a vector for the development of novel recombinant vaccines displays a series of advantages. It is stable, has a low cost of production and acts as an auto-adjuvant, promoting the generation of TH1 phenotypes in CD4+ T cells that secrete high levels of IFN-γ and that are active against intracellular pathogens. Also, it generates CD8+ T cell activation that mediates a cytotoxic response. In addition to protection against M. tuberculosis, a characteristic that draws much attention to this vaccine is its effect on decreasing infant mortality in a non-specific manner. This characteristic gives this vaccine a great advantage over other vaccines that confer protection only against the pathogen for which they were developed. Related to this, it was recently described that BCG induces a memory phenotype in innate immune cells, a phenomenon that is known as “trained immunity.” Trained immunity confers non-specific protection against different pathogens, inducing upregulation of PPRs and the secretion of pro-inflammatory cytokines through epigenetic and metabolic reprogramming. This non-specific protection represents a great advantage when used in newborns since they still do not have a complete development of the adaptive immune system and have not been exposed to a wide range of harmful pathogens. Due to this, the training of innate immune cells conferred by BCG could be playing fundamental roles in helping the vaccinated individuals to respond to a wide variety of pathogens.
Trained immunity induced by BCG vaccination can be considered as a potential approach for improving vaccine development and effectiveness, due to the capacity to promote non-specific stimulation of PRRs in innate immune cells. Activation of these cells may contribute to the protection against different pathogens for which no specific vaccine yet exists. As for the case of the influenza A virus, the high mutation rate of this pathogen can impair the effectiveness of highly specific vaccines, thus the broad spectrum of pathogens to which trained immunity response could be a great advantage for the protection to such infectious agents (133, 134). Furthermore, viral infections such as hRSV have been shown to increase the host susceptibility to bacterial infections (135). Thus, vaccines capable of inducing trained immunity could potentially decrease the occurrence of coinfections. Besides, innate immune training strategies could also be applied to children, elderly or immunocompromised individuals that are unable to developing T or B cell-based specific immune responses (136–141). For these cases, the priming of the innate immune response could lead to a better immune response in cases of infections with a broad spectrum of pathogens, as trained immunity has been shown to respond well to viral, bacterial and fungal infections (96, 112, 114).
Even though BCG has been considered a good immunogenic vector, some factors need to be considered when using this bacterium to develop recombinant vaccines. One issue associated with recombinant BCG vaccines is the level of expression of the heterologous antigen (142). The selection of the vector is crucial, given that expression of the heterologous antigen can be mediated by integrative or replicative vectors. In the case of replicative vectors, as there can be more than one copy in a single mycobacterium, increasing the expression level of the antigen of interest (143). Despite this, integrative vectors are more stable and can promote a more stable expression of the antigen in time, which can, in turn, lead to a longer-lasting immune response (143). However, replicative vectors may have a risk of horizontal transfer to other bacteria present in the host, reducing the safety of the vaccine (144). As for the case of viral proteins, these pathogens use the host transcription and translation machinery to produce their proteins (145). Therefore, a potential drawback for using rBCG vaccines for viruses is the possibility that the expression of viral proteins by prokaryotic cells could proteins with variant conformations or altered epitopes (145). To address this problem, the most immunogenic peptides of the protein of interest could be identified and then cloned into a BCG to express only these peptides. In this way, the efficacy of the recombinant vaccine may be improved.
If recombinant BCGs can induce trained immunity after vaccination, we could think that trained immunity induced by rBCG could, in combination with the specific response, induce robust protection against the pathogen of interest. Determination of the development of trained immunity as a consequence of vaccination with recombinant BCG could represent another good advantage for this type of vaccine. Indeed, improving innate immunity represents an ideal complement for the cellular and/or humoral responses developed by rBCG.
Author Contributions
All authors listed have made a substantial, direct and intellectual contribution to the work, and approved it for publication.
Funding
This research was funded by FONDECYT grant number 1190830, 1170694, 1190864, and 1191300 from CONICYT Chile, doctorate grant N-21170620 from CONICYT Chile, as well as the Millennium Institute on Immunology and Immunotherapy grant number P09/016-F. AK was a Helen C. Levitt Visiting Professor at the Department of Microbiology and Immunology of the University of Iowa. This work was also supported by Regional Government of Antofagasta through the Innovation Fund for Competitiveness FIC-R 2017 (BIP Code: 30488811-0).
Conflict of Interest
The authors declare that the research was conducted in the absence of any commercial or financial relationships that could be construed as a potential conflict of interest.
References
1. WHO. Global World Health Organization. (2018). Available online at: https://www.who.int/tb/publications/global_report/en/
2. Herr HW, Morales A. History of bacillus calmette-guerin and bladder cancer: an immunotherapy success story. J Urol. (2008) 179:53–6. doi: 10.1016/j.juro.2007.08.122
3. Brosch R, Gordon SV, Pym A, Eiglmeier K, Garnier T, Cole ST. Comparative genomics of the mycobacteria. Int J Med Microbiol. (2000) 290:143–52. doi: 10.1016/S1438-4221(00)80083-1
4. Colditz GA, Berkey CS, Mosteller F, Brewer TF, Wilson ME, Burdick E, et al. The efficacy of Bacillus Calmette-Guérin vaccination of newborns and infants in the prevention of tuberculosis: meta-analyses of the published literature. Pediatrics. (1995) 96:29–35.
5. Miceli I, de Kantor IN, Colaiácovo D, Peluffo G, Cutillo I, Gorra R, et al. Evaluation of the effectiveness of BCG vaccination using the case-control method in Buenos Aires, Argentina. Int J Epidemiol. (1988) 17:629–34. doi: 10.1093/ije/17.3.629
6. Trunz BB, Fine PEM, Dye C. Effect of BCG vaccination on childhood tuberculous meningitis and miliary tuberculosis worldwide: a meta-analysis and assessment of cost-effectiveness. Lancet. (2006) 367:1173–80. doi: 10.1016/S0140-6736(06)68507-3
7. Bonifachich E, Chort M, Astigarraga A, Diaz N, Brunet B, Pezzotto SM, et al. Protective effect of Bacillus Calmette-Guerin (BCG) vaccination in children with extra-pulmonary tuberculosis, but not the pulmonary disease: a case-control study in Rosario, Argentina. Vaccine. (2006) 24:2894–9. doi: 10.1016/j.vaccine.2005.12.044
8. Colditz GA, Brewer TF, Berkey CS, Wilson ME, Burdick E, Fineberg HV, et al. Efficacy of BCG Vaccine in the Prevention of Tuberculosis: Meta-analysis of the Published Literature. J Am Med Assoc. (1994) 271:698–702. doi: 10.1001/jama.271.9.698
9. Fine PEM. Variation in protection by BCG: implications of and for heterologous immunity. Lancet. (1995) 346:1339–45. doi: 10.1016/S0140-6736(95)92348-9
10. Brewer TF. Preventing tuberculosis with Bacillus Calmette-Guérin vaccine: a meta-analysis of the literature. Clin Infect Dis. (2000) 31:S64–7. doi: 10.1086/314072
11. Rey-Jurado E, Tapia F, Muñoz-Durango N, Lay MK, Carreño LJ, Riedel CA, et al. Assessing the importance of domestic vaccine manufacturing centers: an overview of immunization programs, vaccine manufacture, and distribution. Front Immunol. (2018) 9:26. doi: 10.3389/fimmu.2018.00026
12. De Bree LCJ, Koeken VACM, Joosten LAB, Aaby P, Benn CS, van Crevel R, et al. Non-specific effects of vaccines: Current evidence and potential implications. Semin Immunol. (2018) 39:35–43. doi: 10.1016/j.smim.2018.06.002
13. De Castro MJ, Pardo-Seco J, Martinón-Torres F. Nonspecific (heterologous) protection of neonatal BCG vaccination against hospitalization due to respiratory infection and sepsis. Clin Infect Dis. (2015) 60:1611–9. doi: 10.1093/cid/civ144
14. Aaby P, Benn CS. Saving lives by training innate immunity with Bacille Calmette-Guérin vaccine. Proc Natl Acad Sci USA. (2012) 109:17317–8. doi: 10.1073/pnas.1215761109
15. Calmette A. Preventive Vaccination against tuberculosis with BCG. J R Soc Med. (1931) 24:1481–90. doi: 10.1177/003591573102401109
16. Roth A, Gustafson P, Nhaga A, Djana Q, Poulsen A, Garly M, et al. BCG vaccination scar associated with better childhood survival in Guinea-Bissau. Int J Epidemiol. (2005) 34:540–7. doi: 10.1093/ije/dyh392
17. Stensballe LG, Nante E, Jensen IP, Kofoed PE, Poulsen A, Jensen H, et al. Acute lower respiratory tract infections and respiratory syncytial virus in infants in Guinea-Bissau: a beneficial effect of BCG vaccination for girls: Community based case-control study. Vaccine. (2005) 23:1251–7. doi: 10.1016/j.vaccine.2004.09.006
18. Biering-Sørensen S, Aaby P, Napirna BM, Roth A, Ravn H, Rodrigues A, et al. Small randomized trial among low-birth-weight children receiving Bacillus Calmette-Guérin vaccination at first health center contact. Pediatr Infect Dis J. (2012) 31:306–8. doi: 10.1097/INF.0b013e3182458289
19. Aaby P, Roth A, Ravn H, Napirna BM, Rodrigues A, Lisse IM, et al. Randomized trial of BCG vaccination at birth to low-birth-weight children : beneficial nonspecific effects in the neonatal period? J Infect Dis. (2011) 204:245–52. doi: 10.1093/infdis/jir240
20. Ponnighaus JM, Msosa E, Gruer PJK, Liomba NG, Fine PEM, Sterne JAC, et al. Efficacy of BCG vaccine against leprosy and tuberculosis in northern Malawi. Lancet. (1992) 339:636–9. doi: 10.1016/0140-6736(92)90794-4
21. Garly M-L, Martins CL, Balé C, Baldé MA, Hedegaard KL, Gustafson P, et al. BCG scar and positive tuberculin reaction associated with reduced child mortality in West Africa. A non-specific beneficial effect of BCG? Vaccine. (2003) 21:2782–90. doi: 10.1016/S0264-410X(03)00181-6
22. Shann F. The non-specific effects of vaccines. Arch Dis Child. (2010) 95:662–7. doi: 10.1136/adc.2009.157537
23. Shann F. Nonspecific effects of vaccines and the reduction of mortality in children. Clin Ther. (2013) 35:109–14. doi: 10.1016/j.clinthera.2013.01.007
24. Higgins JPT, Soares-Weiser K, López-López JA, Kakourou A, Chaplin K, Christensen H, et al. Association of BCG, DTP, and measles containing vaccines with childhood mortality: systematic review. BMJ. (2016) 355:i5170. doi: 10.1136/bmj.i5170
25. Stover CK, de la Cruz VF, Fuerst TR, Burlein JE, Benson LA, Bennett LT, et al. New use of BCG for recombinant vaccines. Nature. (1991) 351:456–60. doi: 10.1038/351456a0
26. Fennelly GJ, Flynn JL, Meulen V, Liebert UG, Bloom BR. Recombinant bacille calmette-guréin priming against measles. J Infect Dis. (1995) 172:698–705. doi: 10.1093/infdis/172.3.698
27. Wang H, Liu Q, Liu K, Zhong W, Gao S, Jiang L, et al. Immune response induced by recombinant Mycobacterium bovis BCG expressing ROP2 gene of Toxoplasma gondii. Parasitol Int. (2007) 56:263–8. doi: 10.1016/j.parint.2007.04.003
28. Yu JS, Peacock JW, Jacobs WR, Frothingham R, Letvin NL, Liao HX, et al. Recombinant Mycobacterium bovis Bacillus Calmette-Guérin elicits human immunodeficiency virus type 1 envelope-specific T lymphocytes at mucosal sites. Clin Vaccine Immunol. (2007) 14:886–93. doi: 10.1128/CVI.00407-06
29. Chapman R, Stutz H, Jacobs W, Shephard E, Williamson AL. Priming with recombinant auxotrophic BCG expressing HIV-1 Gag, RT and Gp120 and boosting with recombinant MVA induces a robust T cell response in mice. PLoS ONE. (2013) 8:e71601. doi: 10.1371/annotation/4f08219c-2d7b-4309-8351-d3fe2378993f
30. Hopkins R, Bridgeman A, Bourne C, Mbewe-Mvula A, Sadoff JC, Both GW, et al. Optimizing HIV-1-specific CD8 + T-cell induction by recombinant BCG in prime-boost regimens with heterologous viral vectors. Eur J Immunol. (2011) 41:3542–52. doi: 10.1002/eji.201141962
31. Hopkins R, Bridgeman A, Joseph J, Gilbert SC, McShane H, Hanke T. Dual neonate vaccine platform against HIV-1 and M. tuberculosis. PLoS ONE. (2011) 6:e20067. doi: 10.1371/journal.pone.0020067
32. Soto JA, Gálvez NMS, Rivera CA, Palavecino CE, Céspedes PF, Rey-Jurado E, et al. Recombinant BCG vaccines reduce pneumovirus-caused airway pathology by inducing protective humoral immunity. Front Immunol. (2018) 9:2875. doi: 10.3389/fimmu.2018.02875
33. Céspedes PF, Gonzalez PA, Kalergis AM. Human metapneumovirus keeps dendritic cells from priming antigen-specific naive T cells. Immunology. (2013) 139:366–76. doi: 10.1111/imm.12083
34. Palavecino CE, Cespedes PF, Gomez RS, Kalergis AM, Bueno SM. Immunization with a recombinant bacillus calmette-guerin strain confers protective Th1 immunity against the human metapneumovirus. J Immunol. (2014) 192:214–23. doi: 10.4049/jimmunol.1300118
35. Pfahlberg A, Kölmel KF, Grange JM, Mastrangelo G, Krone B, Botev IN, et al. Inverse association between melanoma and previous vaccinations against tuberculosis and smallpox: results of the FEBIM study. J Invest Dermatol. (2002) 119:570–5. doi: 10.1046/j.1523-1747.2002.00643.x
36. Morra ME, Kien ND, Elmaraezy A, Abdelaziz OAM, Elsayed AL, Halhouli O, et al. Early vaccination protects against childhood leukemia: a systematic review and meta-analysis. Sci Rep. (2017) 7:1–9. doi: 10.1038/s41598-017-16067-0
37. Morton DL, Eilber FR, Holmes EC, Hunt JS, Ketcham AS, Silverstein MJ, et al. BCG immunotherapy of malignant melanoma: Summary of a seven year experience. Ann Surg. (1974) 180:635–43. doi: 10.1097/00000658-197410000-00029
38. Herr HW, Laudone VP, Badalament RA, Oettgen HF, Sogani PC, Freedman BD, et al. Bacillus Calmette-Guerin therapy alters the progression of superficial bladder cancer. J Clin Oncol. (1988) 6:1450–5. doi: 10.1200/JCO.1988.6.9.1450
39. Böhle A, Brandau S. Immune mechanisms in bacillus calmette-guerin immunotherapy for superficial bladder cancer. J Urol. (2003) 170:964–9. doi: 10.1097/01.ju.0000073852.24341.4a
40. Prescott S, James K, Hargreave TB, Chisholm GD, Smyth JF. Intravesical evans strain BCG therapy: quantitative immunohistochemical analysis of the immune response within the bladder wall. J Urol. (1992) 147:163642. doi: 10.1016/S0022-5347(17)37668-1
41. de Boer EC, de Jong WH, van der Meijden APM, Steerenberg PA, Witjes F, Vegt PDJ, et al. Leukocytes in the urine after intravesical BCG treatment for superficial bladder cancer. Urol Res. (1991) 19:45–50. doi: 10.1007/BF00294021
42. Ikeda N, Toida I, Iwasaki A, Kawai K, Akaza H. Surface antigen expression on bladder tumor cells induced by Bacillus Calmette-Guérin (BCG): a role of BCG internalization into tumor cells. Int J Urol. (2002) 9:29–35. doi: 10.1046/j.1442-2042.2002.00415.x
43. Binnewies M, Roberts EW, Kersten K, Chan V, Fearon DF, Merad M, Coussens LM, et al. Understanding the tumor immune microenvironment (TIME) for effective therapy. Nat Med. (2018) 24:541–50. doi: 10.1038/s41591-018-0014-x
44. Dockrell HM, Smith SG. What have we learnt about BCG vaccination in the last 20 years? Front Immunol. (2017) 8:1134. doi: 10.3389/fimmu.2017.01134
45. Kilpeläinen A, Maya-Hoyos M, Saubí N, Soto CY, Joseph Munne J. Advances and challenges in recombinant Mycobacterium bovis BCG-based HIV vaccine development: lessons learned. Expert Rev Vaccines. (2018) 17:1005–20. doi: 10.1080/14760584.2018.1534588
46. Moliva JI, Turner J, Torrelles JB. Immune responses to bacillus calmette–guérin vaccination: why do they fail to protect against Mycobacterium tuberculosis?. Front Immunol. (2017) 8:407. doi: 10.3389/fimmu.2017.00407
47. Kumar S, Sunagar R, Gosselin E. Bacterial protein toll-like-receptor agonists: a novel perspective on vaccine adjuvants. Front Immunol. (2019) 10:1144. doi: 10.3389/fimmu.2019.01144
48. Gagliardi MC, Teloni R, Giannoni F, Pardini M, Sargentini V, Brunori L, et al. Mycobacterium bovis bacillus calmette-guerin infects DC-SIGN- dendritic cell and causes the inhibition of IL-12 and the enhancement of IL-10 production. J Leukoc Biol. (2005) 78:106–13. doi: 10.1189/jlb.0105037
49. Jiao X, Lo-Man R, Guermonprez P, Fiette L, Dériaud E, Burgaud S, et al. Dendritic cells are host cells for mycobacteria in vivo that trigger innate and acquired immunity. J Immunol. (2002) 168:1294–301. doi: 10.4049/jimmunol.168.3.1294
50. Tsuji S, Matsumoto M, Takeuchi O, Akira S, Azuma I, Hayashi A, et al. Maturation of human dendritic cells by cell wall skeleton of Mycobacterium bovis bacillus calmette-guérin : involvement of toll-like receptors. Infect Immun. (2000) 68:6883–90. doi: 10.1128/IAI.68.12.6883-6890.2000
51. Joosten SA, van Meijgaarden KE, Arend SM, Prins C, Oftung F, Korsvold GE, et al. Mycobacterial growth inhibition is associated with trained innate immunity. J Clin Invest. (2018) 128:1837–51. doi: 10.1172/JCI97508
52. Bertholet S, Ireton GC, Kahn M, Guderian J, Mohamath R, Stride N, et al. Identification of human T cell antigens for the development of vaccines against Mycobacterium tuberculosis. J Immunol. (2008) 181:7948–57. doi: 10.4049/jimmunol.181.11.7948
53. Kaufmann SHE. Tuberculosis vaccines: time to think about the next generation. Semin Immunol. (2013) 25:172–81. doi: 10.1016/j.smim.2013.04.006
54. Bollampalli VP, Yamashiro LH, Feng X, Bierschenk D, Gao Y, Blom H, et al. BCG skin infection triggers IL-1R-MyD88- dependent migration of EpCAM low CD11b high skin dendritic cells to draining lymph node during CD4 + T-cell priming. PLoS Pathog. (2015) 11:e1005206. doi: 10.1371/journal.ppat.1005206
55. Su H, Peng B, Zhang Z, Liu Z, Zhang Z. The Mycobacterium tuberculosis glycoprotein Rv1016c protein inhibits dendritic cell maturation, and impairs Th1 /Th17 responses during mycobacteria infection. Mol Immunol. (2019) 109:58–70. doi: 10.1016/j.molimm.2019.02.021
56. Bizzell E, Sia JK, Quezada M, Enriquez A, Georgieva M, Rengarajan J. Deletion of BCG Hip1 protease enhances dendritic cell and CD4 T cell responses. J Leukoc Biol. (2018) 103:739–48. doi: 10.1002/JLB.4A0917-363RR
57. Humphreys IR, Stewart GR, Turner DJ, Patel J, Karamanou D, Snelgrove RJ, et al. A role for dendritic cells in the dissemination of mycobacterial infection. Microbes Infect. (2006) 8:1339–46. doi: 10.1016/j.micinf.2005.12.023
58. Morel C, Badell E, Abadie V, Robledo M, Setterblad N, Gluckman JC, et al. Mycobacterium bovis BCG-infected neutrophils and dendritic cells cooperate to induce specific T cell responses in humans and mice. Eur J Immunol. (2008) 38:437–47. doi: 10.1002/eji.200737905
59. Andersen P, Kaufmann SHE. Novel vaccination strategies against tuberculosis. Cold Spring Harb Perspect Med. (2014) 4:a018523. doi: 10.1101/cshperspect.a018523
60. Hanekom WA. The immune response to BCG vaccination of newborns. Ann New York Acad Sci. (2005) 1062:69–78. doi: 10.1196/annals.1358.010
61. Murray RA, Mansoor N, Harbacheuski R, Soler J, Davids V, Soares A, et al. Bacillus calmette guerin vaccination of human newborns induces a specific, functional CD8+ T cell response. J Immunol. (2014) 177:5647–51. doi: 10.4049/jimmunol.177.8.5647
62. Soares AP, Scriba TJ, Joseph S, Harbacheuski R, Ann R, Gelderbloem SJ, et al. Bacille calmette guerin vaccination of human newborns induces T cells with complex cytokine and phenotypic profiles. J Immunol. (2010) 180:3569–77. doi: 10.4049/jimmunol.180.5.3569
63. Ravn P, Boesen H, Pedersen BK, Andersen P. Human T cell responses induced by vaccination with Mycobacterium bovis bacillus calmette-Guérin. J Immunol. (1997) 158:1949–55.
64. Soares AP, Kwong Chung CKC, Choice T, Hughes EJ, Jacobs G, Van Rensburg EJ, et al. Longitudinal changes in CD4+ T-cell memory responses induced by BCG vaccination of newborns. J Infect Dis. (2013) 207:1084–94. doi: 10.1093/infdis/jis941
65. Feng CG, Britton WJ. CD4 + and CD8 + T cells mediate adoptive immunity to aerosol infection of Mycobacterium bovis bacillus calmette-Guérin. J Infect Dis. (2000) 181:1846–9. doi: 10.1086/315466
66. Silva CL, Bonato VLD, Lima VMF, Faccioli LH, Leão SC. Characterization of the memory/activated T cells that mediate the long- lived host response against tuberculosis after bacillus Calmette-Guerin or DNA vaccination. Immunology. (1999) 97:573–81. doi: 10.1046/j.1365-2567.1999.00840.x
67. Chen T, Blanc C, Eder AZ, Prados-Rosales R, Oliveira Souza AC, Kim RS, et al. Association of human antibodies to arabinomannan with enhanced mycobacterial opsonophagocytosis and intracellular growth reduction. J Infect Dis. (2016) 214:300–10. doi: 10.1093/infdis/jiw141
68. Sebina I, Cliff JM, Smith SG, Nogaro S, Webb EL, Riley EM, et al. Long-lived memory B-cell responses following BCG vaccination. PLoS ONE. (2012) 7:e51381. doi: 10.1371/journal.pone.0051381
69. Perdomo C, Zedler U, Kühl AA, Lozza L, Saikali P, Sander LE, et al. Mucosal BCG Vaccination induces protective lung-resident memory T cell populations against tuberculosis. MBio. (2016) 7:1–11. doi: 10.1128/mBio.01686-16
70. Uranga S, Marinova D, Martin C, Aguilo N. Protective efficacy and pulmonary immune response following subcutaneous and intranasal BCG administration in mice. J Vis Exp. (2016) 115:e54440 doi: 10.3791/54440
71. Hansen IS, Baeten DLP, den Dunnen J. The inflammatory function of human IgA. Cell Mol Life Sci. (2019) 76:1041–55. doi: 10.1007/s00018-018-2976-8
72. Martinov T, Fife BT. Type 1 diabetes pathogenesis and the role of inhibitory receptors in islet tolerance. Ann New York Acad Sci. (2019). doi: 10.1111/nyas.14106. [Epub ahead of print].
73. Kowalewicz-Kulbat M, Locht C. BCG and protection against inflammatory and auto-immune diseases. Expert Rev Vaccines. (2017) 16:699–708. doi: 10.1080/14760584.2017.1333906
74. Shehadeh N, Bruchlim I, Vardi P, Calcinaro F, Lafferty K. Effect of adjuvant therapy on development of diabetes in mouse and man. Lancet. (1994) 343:706–7. doi: 10.1016/S0140-6736(94)91583-0
75. Allen HF, Simoes EAF, Klingensmith GJ, Hayward A, Jensen P, Chase HP. Effect of Bacillus Calmette-Guerin Vaccination on New-Onset Type 1 Diabetes. Diabetes Care. (1999) 22:1703–7. doi: 10.2337/diacare.22.10.1703
76. Faustman DL, Wang L, Okubo Y, Burger D, Ban L, Man G, et al. Proof-of-concept, randomized, controlled clinical trial of bacillus-calmette-guerin for treatment of long-term type 1 diabetes. PLoS ONE. (2012) 7:e41756. doi: 10.1371/journal.pone.0041756
77. Kuhtreiber W, Tran L, Nguyen B, Janes Se, Defusco AA, Faustman DL. Long-term reduction in hyperglycemia in advanced type 1 diabetes—the value of induced aerobic glycolysis by BCG vaccinations. Diabetes. (2018) 67:2339. doi: 10.2337/db18-2339-PUB
78. Sadelain MWJ, Qin HY, Lauzon J, Singh B. Prevention of type I diabetes in NOD mice by adjuvant immunotherapy. Diabetes. (1990) 39:583–9. doi: 10.2337/diabetes.39.5.583
79. Kodama S, Kühtreiber W, Fujimura S, Dale EA, Faustman DL. Islet regeneration during the reversal of autoimmune diabetes in NOD Mice. Science. (2003) 302:1223–7. doi: 10.1126/science.1088949
80. Vaughn CB, Benedict RHB, Zivadinov R, Weinstock- B. Epidemiology and treatment of multiple sclerosis in elderly populations. Nat Rev Neurol. (2004) 15:329–42. doi: 10.1038/s41582-019-0183-3
81. Ristori G, Buzzi MG, Sabatini U, Giugni E, Bastianello S, Viselli F, et al. Use of Bacille Calmette-Guérin (BCG) in multiple sclerosis. Neurology. (1999) 53:1588–1589. doi: 10.1212/WNL.53.7.1588
82. Ristori G, Romano S, Cannoni S, Visconti A, Tinelli E, Mendozzi L, et al. Effects of Bacille Calmette-Guérin after the first demyelinating event in the CNS. Neurology. (2014) 82:41–8. doi: 10.1212/01.wnl.0000438216.93319.ab
83. Lippens C, Garnier L, Guyonvarc'h PM, Santiago-Raber ML, Hugues S. Extended freeze-dried BCG instructed pDCs induce suppressive tregs and dampen EAE. Front Immunol. (2018) 9:2777. doi: 10.3389/fimmu.2018.02777
84. Kleinnijenhuis J, Quintin J, Preijers F, Benn CS, Joosten LAB, Jacobs C, et al. Long-lasting effects of BCG vaccination on both heterologous Th1/Th17 responses and innate trained immunity. J Innate Immun. (2015) 6:152–8. doi: 10.1159/000355628
85. Kagina BMN, Abel B, Scriba TJ, Hughes EJ, Keyser A, Soares A, et al. Specific T cell frequency and cytokine expression profile do not correlate with protection against tuberculosis after Bacillus Calmette-Guérin vaccination of newborns. Am J Respir Crit Care Med. (2010) 182:1073–9. doi: 10.1164/rccm.201003-0334OC
86. Arts RJW, Carvalho A, La Rocca C, Palma C, Rodrigues F, Silvestre R, et al. Immunometabolic pathways in BCG-induced trained immunity. Cell Rep. (2016) 17:2562–71. doi: 10.1016/j.celrep.2016.11.011
87. Procaccini C, Carbone F, Di Silvestre D, Brambilla F, De Rosa V, Galgani M, et al. The proteomic landscape of human ex vivo regulatory and conventional T cells reveals specific metabolic requirements. Immunity. (2016) 44:406–21. doi: 10.1016/j.immuni.2016.01.028
88. Ristori G, Faustman D, Matarese G, Romano S, Salvetti M. Bridging the gap between vaccination with Bacille Calmette-Guérin (BCG) and immunological tolerance: the cases of type 1 diabetes and multiple sclerosis. Curr Opin Immunol. (2018) 55:89–96. doi: 10.1016/j.coi.2018.09.016
89. Dalton DK, Haynes L, Chu CQ, Swain SL, Wittmer S. Interferon γ eliminates responding CD4 T cells during mycobacterial infection by inducing apoptosis of activated CD4 T cells. J Exp Med. (2000) 192:117–22. doi: 10.1084/jem.192.1.117
90. Lampropoulou V, Hoehlig K, Roch T, Neves P, Gómez EC, Sweenie CH, et al. TLR-activated B cells suppress T cell-mediated autoimmunity. J Immunol. (2008) 180:4763–73. doi: 10.4049/jimmunol.180.7.4763
91. Lardone RD, Chan AA, Lee AF, Foshag LJ, Faries MB, Sieling PA, et al. Mycobacterium bovis bacillus calmette–guérin alters melanoma microenvironment favoring antitumor T cell responses and improving M2 macrophage function. Front Immunol. (2017) 8:965. doi: 10.3389/fimmu.2017.00965
92. Herr HW, Schwalb DM, Zhang ZF, Sogani PC, Whitmore WF, Oettgen HF. Intravesical BCG therapy delays tumor progression and death from superficial bladder cancer: ten-year followup of a prospective randomized trial: author update. Class Pap Curr Comments Highlights Genitourin Cancer Res. (1998) 13:1404–8. doi: 10.1200/JCO.1995.13.6.1404
93. Bohle A, Gerdes J, Ulmer AJ, Hofstetter AG, Flad HD. Effects of local bacillus Calmette-Guerin therapy in patients with bladder carcinoma on immunocompetent cells of the bladder wall. J Urol. (1990) 144:53–8. doi: 10.1016/S0022-5347(17)39365-5
94. Netea MG, Quintin J, Van Der Meer JWM. Trained immunity: a memory for innate host defense. Cell Host Microbe. (2011) 9:355–61. doi: 10.1016/j.chom.2011.04.006
95. Kleinnijenhuis J, Van Crevel R, Netea MG. Trained immunity: consequences for the heterologous effects of BCG vaccination. Trans R Soc Trop Med Hyg. (2014) 109:29–35. doi: 10.1093/trstmh/tru168
96. Kleinnijenhuis J, Quintin J, Preijers F, Joosten LAB, Ifrim DC, Saeed S, et al. Bacille Calmette-Guerin induces NOD2-dependent nonspecific protection from reinfection via epigenetic reprogramming of monocytes. Proc Natl Acad Sci USA. (2012) 109:17537–42. doi: 10.1073/pnas.1202870109
97. Wout JW, Poell R, Furth R. The Role of BCG/PPD-activated macrophages in resistance against systemic candidiasis in mice. Scand J Immunol. (1992) 36:713–20. doi: 10.1111/j.1365-3083.1992.tb03132.x
98. Kleinnijenhuis J, Quintin J, Preijers F, Joosten LAB, Jacobs C, Xavier RJ, et al. BCG-induced trained immunity in NK cells: role for non-specific protection to infection. Clin Immunol. (2014) 155:213–9. doi: 10.1016/j.clim.2014.10.005
99. Guerra-Maupome M, Vang DX, McGill JL. Aerosol vaccination with Bacille Calmette-Guerin induces a trained innate immune phenotype in calves. PLoS ONE. (2019) 14:1–16. doi: 10.1371/journal.pone.0212751
100. Zhang Q, Cao X. Epigenetic regulation of the innate immune response to infection. Nat Rev Immunol. (2019) 19:417–32. doi: 10.1038/s41577-019-0151-6
101. Wen H, Dou Y, Hogaboam CM, Kunkel SL, DC W. Epigenetic regulation of dendritic cell – derived interleukin-12 facilitates immunosuppression after a severe innate immune response. Public Health. (2008) 111:1797–804. doi: 10.1182/blood-2007-08-106443
102. Foster SL, Medzhitov R. Gene-specific control of the TLR-induced inflammatory response. Clin Immunol. (2008) 130:7–15. doi: 10.1016/j.clim.2008.08.015
103. Doñas C, Fritz M, Manríquez V, Tejón G, Bono MR, Loyola A, et al. Trichostatin A promotes the generation and suppressive functions of regulatory T cells. Clin Dev Immunol. (2013) 2013:1–8. doi: 10.1155/2013/679804
104. Kouzarides T. Chromatin modifications and their function. Cell. (2007) 128:693–705. doi: 10.1016/j.cell.2007.02.005
105. Liu H, Li P, Wei Z, Zhang C, Xia M, Du Q, et al. Regulation of T cell differentiation and function by epigenetic modification enzymes. Semin Immunopathol. (2019) 41:315–26. doi: 10.1007/s00281-019-00731-w
106. Arts RJW, Blok BA, Aaby P, Joosten LAB, de Jong D, van der Meer JWM, et al. Long-term in vitro and in vivo effects of γ-irradiated BCG on innate and adaptive immunity. J Leukoc Biol. (2015) 98:995–1001. doi: 10.1189/jlb.4MA0215-059R
107. Arts RJW, Novakovic B, ter Horst R, Carvalho A, Bekkering S, Lachmandas E, et al. Glutaminolysis and fumarate accumulation integrate immunometabolic and epigenetic programs in trained immunity. Cell Metab. (2016) 24:807–19. doi: 10.1016/j.cmet.2016.10.008
108. Cheng S-C, Quintin J, Cramer RA, Shepardson KM, Saeed S, Kumar V, et al. mTOR/HIF1α-mediated aerobic glycolysis as metabolic basis for trained immunity. Science. (2014) 345:1–18. doi: 10.1126/science.1250684
109. Bekkering S, Arts RJW, Novakovic B, Kourtzelis I, van der Heijden CDCC, Li Y, et al. Metabolic induction of trained immunity through the mevalonate pathway. Cell. (2018) 172:135–46.e9. doi: 10.1016/j.cell.2017.11.025
110. Czarnewski P, Das S, Parigi SM, Villablanca EJ. Retinoic acid and its role in modulating intestinal innate immunity. Nutrients. (2017) 9:68. doi: 10.3390/nu9010068
111. Arts RJW, Blok BA, van Crevel R, Joosten LAB, Aaby P, Benn CS, et al. Vitamin A induces inhibitory histone methylation modifications and down-regulates trained immunity in human monocytes. J Leukoc Biol. (2015) 98:129–36. doi: 10.1189/jlb.6AB0914-416R
112. Arts RJW, Moorlag SJCFM, Novakovic B, Li Y, Wang SY, Oosting M, et al. BCG vaccination protects against experimental viral infection in humans through the induction of cytokines associated with trained immunity. Cell Host Microbe. (2018) 23:89–100.e5. doi: 10.1016/j.chom.2017.12.010
113. Mitroulis I, Ruppova K, Wang B, Chen LS, Grzybek M, Grinenko T, et al. Modulation of myelopoiesis progenitors is an integral component of trained immunity. Cell. (2018) 172:147–61.e12. doi: 10.1016/j.cell.2017.11.034
114. Kaufmann E, Sanz J, Dunn JL, Khan N, Mendonça LE, Pacis A, et al. BCG educates hematopoietic stem cells to generate protective innate immunity against tuberculosis. Cell. (2018) 172:176–90.e19. doi: 10.1016/j.cell.2017.12.031
115. Khader SA, Divangahi M, Hanekom W, Hill PC, Maeurer M, Makar KW, Mayer-Barber KD, et al. Targeting innate immunity for tuberculosis vaccination. J Clin Invest. (2019) 129:3482–91. doi: 10.1172/JCI128877
116. Zhu Y, Fennelly G, Miller C, Tarara R, Saxe I, Bloom B, et al. Recombinant Bacille Calmette-Guérin expressing the measles virus nucleoprotein protects infant rhesus macaques from measles virus pneumonia. Oxford Univ Press. (1997) 176:1445–53. doi: 10.1086/514140
117. Saadatnia G, Golkar M. A review on human toxoplasmosis. Scand J Infect Dis. (2012) 44:805–14. doi: 10.3109/00365548.2012.693197
118. Daniels HL, Sabella C. Bordetella pertussis (Pertussis). Pediatr Rev. (2018) 39:247–57. doi: 10.1542/pir.2017-0229
119. Nascimento IP, Dias WO, Mazzantini RP, Miyaji EN, Gamberini M, Quintilio W, et al. Recombinant Mycobacterium bovis BCG expressing pertussis toxin subunit S1 induces protection against an intracerebral challenge with live Bordetella pertussis in mice. Infect Immun. (2000) 68:4877–83. doi: 10.1128/IAI.68.9.4877-4883.2000
120. Medeiros MA, Armôa GR, Dellagostin OA, McIntosh D. Induction of humoral immunity in response to immunization with recombinant Mycobacterium bovis BCG expressing the S1 subunit of Bordetella pertussis toxin. Can J Microbiol. (2006) 51:1015–20. doi: 10.1139/w05-095
121. Nascimento IP, Dias WO, Quintilio W, Christ AP, Moraes JF, Vancetto MDC, et al. Neonatal immunization with a single dose of recombinant BCG expressing subunit S1 from pertussis toxin induces complete protection against Bordetella pertussis intracerebral challenge. Microbes Infect. (2008) 10:198–202. doi: 10.1016/j.micinf.2007.10.010
123. Loxton AG, Knaul JK, Grode L, Gutschmidt A, Meller C, Eisele B, et al. Safety and immunogenicity of the vaccine VPM1002 in HIV-unexposed newborn infants in South Africa. Clin Vaccine Immunol. (2017) 24: e00439–16. doi: 10.1128/CVI.00439-16
124. Kim BJ, Kim BR, Kook YH, Kim BJ. Development of a live recombinant BCG expressing human immunodeficiency virus type 1 (HIV-1) gag using a pMyong2 vector system: Potential use as a novel HIV-1 vaccine. Front Immunol. (2018) 9:643. doi: 10.3389/fimmu.2018.00643
125. Mahant A, Saubi N, Eto Y, Guitart N, Gatell JM, Hanke T, et al. Preclinical development of BCG.HIVA2auxo.int, harboring an integrative expression vector, for a HIV-TB pediatric vaccine. Enhancement of stability and specific HIV-1 T-cell immunity. Hum Vaccines Immunother. (2017) 13:1798–810. doi: 10.1080/21645515.2017.1316911
126. Hoft DF, Blazevic A, Selimovic A, Turan A, Tennant J, Abate G, et al. Safety and Immunogenicity of the recombinant BCG vaccine AERAS-422 in healthy BCG-Naïve adults: a randomized, active-controlled, first-in-human phase 1 trial. EBioMedicine. (2016) 7:278–86. doi: 10.1016/j.ebiom.2016.04.010
127. Nieuwenhuizen NE, Kulkarni PS, Shaligram U, Cotton MF, Rentsch CA, Eisele B, et al. The recombinant Bacille Calmette-Guérin vaccine VPM1002: Ready for clinical efficacy testing. Front Immunol. (2017) 8:1147. doi: 10.3389/fimmu.2017.01147
128. Lay MK, Céspedes PF, Palavecino CE, León MA, Díaz RA, Salazar FJ, et al. Human metapneumovirus infection activates the TSLP pathway that drives excessive pulmonary inflammation and viral replication in mice. Eur J Immunol. (2015) 45:1680–95. doi: 10.1002/eji.201445021
129. Céspedes PF, Rey-Jurado E, Espinoza JA, Rivera CA, Canedo-Marroquín G, Bueno SM, et al. A single, low dose of a cGMP recombinant BCG vaccine elicits protective T cell immunity against the human respiratory syncytial virus infection and prevents lung pathology in mice. Vaccine. (2017) 35:757–66. doi: 10.1016/j.vaccine.2016.12.048
130. Shi T, McAllister DA, O'Brien KL, Simoes EAF, Madhi SA, Gessner BD, et al. Global, regional, and national disease burden estimates of acute lower respiratory infections due to respiratory syncytial virus in young children in 2015: a systematic review and modelling study. Lancet. (2017) 390:946–58. doi: 10.1016/S0140-6736(17)30938-8
131. Mazur NI, Higgins D, Nunes MC, Melero JA, Langedijk AC, Horsley N, et al. The respiratory syncytial virus vaccine landscape: lessons from the graveyard and promising candidates. Lancet Infect Dis. (2018) 18:e295–311. doi: 10.1016/S1473-3099(18)30292-5
132. Saeed S, Quintin J, Kerstens HHD, Rao NA, Aghajanirefah A, Matarese F, et al. Epigenetic programming of monocyte-to-macrophage differentiation and trained innate immunity. Science. (2014) 345:1251086. doi: 10.1126/science.1251086
133. Xue KS, Moncla LH, Bedford T, Bloom JD. Within-host evolution of human influenza virus. Trends Microbiol. (2018) 26:781–93. doi: 10.1016/j.tim.2018.02.007
134. Harding AT, Heaton NS. Efforts to improve the seasonal influenza vaccine. Vaccines. (2018) 6:E19. doi: 10.3390/vaccines6020019
135. Nam HH, Ison MG. Respiratory syncytial virus infection in adults. BMJ. (2019) 366:l5021. doi: 10.1136/bmj.l5021
136. Witt DJ, Craven DE, McCabe WR. Bacterial infections in adult patients with the acquired immune deficiency syndrome (AIDS) and AIDS-related complex. Am J Med. (1987) 82:900–6. doi: 10.1016/0002-9343(87)90150-1
137. Shannon KM, Ammann AJ. Acquired immune deficiency syndrome in childhood. J Pediatr. (1985) 106:332–42. doi: 10.1016/S0022-3476(85)80320-6
138. Abzug MJ. Acute sinusitis in children: Do antibiotics have any role? J Infect. (2014) 68:S33–7. doi: 10.1016/j.jinf.2013.09.012
139. Wilson R, Sethi S, Anzueto A, Miravitlles M. Antibiotics for treatment and prevention of exacerbations of chronic obstructive pulmonary disease. J Infect. (2013) 67:497–515. doi: 10.1016/j.jinf.2013.08.010
140. Esposito S, Soto-Martinez ME, Feleszko W, Jones MH, Shen KL, Schaad UB. Nonspecific immunomodulators for recurrent respiratory tract infections, wheezing and asthma in children: a systematic review of mechanistic and clinical evidence. Curr Opin Allergy Clin Immunol. (2018) 18:198–209. doi: 10.1097/ACI.0000000000000433
141. Kline KA, Bowdish DME. Infection in an aging population. Curr Opin Microbiol. (2016) 29:63–7. doi: 10.1016/j.mib.2015.11.003
142. Honda M, Matsuo K, Nakasone T, Okamoto Y, Yoshizaki H, Kitamura K, et al. Protective immune responses induced by secretion of a chimeric soluble protein from a recombinant Mycobacterium bovis Bacillus Calmette-Guérin vector candidate vaccine for human immunodeficiency virus type 1 in small animals. Proc Natl Acad Sci USA. (1995) 92:10693–7. doi: 10.1073/pnas.92.23.10693
143. Me I, Bourguin I, Ensergueix D, Badell E, Gicquel B, Winter N. Plasmidic versus insertional cloning of heterologous genes in Mycobacterium bovis BCG: impact on in vivo antigen persistence and immune responses. Infect Immun. (2002) 70:303–14. doi: 10.1128/IAI.70.1.303-314.2002
144. Oliveira TL, Rizzi C, Dellagostin OA. Recombinant BCG vaccines: molecular features and their influence in the expression of foreign genes. Appl Microbiol Biotechnol. (2017) 101:6865–77. doi: 10.1007/s00253-017-8439-6
Keywords: BCG, innate immunity, trained immunity, heterologous protection, vaccine
Citation: Covián C, Fernández-Fierro A, Retamal-Díaz A, Díaz FE, Vasquez AE, Lay MK, Riedel CA, González PA, Bueno SM and Kalergis AM (2019) BCG-Induced Cross-Protection and Development of Trained Immunity: Implication for Vaccine Design. Front. Immunol. 10:2806. doi: 10.3389/fimmu.2019.02806
Received: 10 October 2019; Accepted: 15 November 2019;
Published: 29 November 2019.
Edited by:
Babita Agrawal, University of Alberta, CanadaReviewed by:
Mihai Netea, Radboud University Nijmegen, NetherlandsEstrella Mariel Levy, National Council for Scientific and Technical Research (CONICET), Argentina
Copyright © 2019 Covián, Fernández-Fierro, Retamal-Díaz, Díaz, Vasquez, Lay, Riedel, González, Bueno and Kalergis. This is an open-access article distributed under the terms of the Creative Commons Attribution License (CC BY). The use, distribution or reproduction in other forums is permitted, provided the original author(s) and the copyright owner(s) are credited and that the original publication in this journal is cited, in accordance with accepted academic practice. No use, distribution or reproduction is permitted which does not comply with these terms.
*Correspondence: Alexis M. Kalergis, YWthbGVyZ2lzJiN4MDAwNDA7YmlvLnB1Yy5jbA==