- 1Department of Experimental Neuroimmunology, Klinikum rechts der Isar, Technical University of Munich, Munich, Germany
- 2Department of Neurology, Klinikum rechts der Isar, Technical University of Munich, Munich, Germany
- 3Munich Cluster for Systems Neurology (SyNergy), Munich, Germany
Foxp3+ Treg cells are indispensable for maintaining self-tolerance in secondary lymphoid organs (SLOs). However, Treg cells are also recruited to non-lymphoid tissues (NLTs) during inflammation. Recent advances in the understanding of Treg cell biology provided us with molecular mechanisms—both transcriptional and epigenetic—that enable Treg cells to retain their identity in an inflammatory milieu that is per se hostile to sustained expression of high levels of Foxp3. While Treg cells are recruited to sites of inflammation in order to resolve inflammation and re-establish appropriate organ function, it is increasingly recognized that a series of inflammatory (but also non-inflammatory) perturbations of organ function lead to the constitution of relatively long lived populations of Treg cells in NLTs. NLT Treg cells are heterogeneous according to their respective site of residence and it will be an important goal of future investigations to determine how these NLT Treg cells are maintained, e.g., what the role of antigen recognition by NLT Treg cells is and which growth factors are responsible for their self-renewal in the relative deficiency of IL-2. Finally, it is an open question what functions NLT Treg cells have besides their role in maintaining immunologic tolerance. In this review, we will highlight and summarize major ideas on the biology of NLT Treg cells (in the central nervous system but also at other peripheral sites) during inflammation and in steady state.
Introduction
Foxp3+ Treg cells have been intensely studied over the last three decades (1). Treg cells in secondary lymphoid tissues are indispensable for maintaining immune tolerance because elimination of Treg cells either in newborn individuals or in adults results in multiorgan autoimmunity within a couple of weeks (2, 3). It is an intriguing concept to exploit Treg cells for therapeutic interventions—either enhancing their function in autoimmunity or dampening their effect in cancer. Proof of concept trials in graft-vs.-host disease and even in type 1 diabetes have been undertaken in humans (4, 5). However, antigen specificity of Treg cells (most trials were performed with polyclonal Treg cell populations), their trafficking behavior, and their stability after adoptive transfer into human hosts remain challenges on the way to a broader application of adoptive Treg cell therapy (6). Perhaps it is now time to take a step back and consider novel and unconventional concepts about Foxp3+ Treg cells that might nevertheless be fundamental to the understanding of Treg cells in homeostasis and in disease conditions: First, Treg cells might have some plasticity and adapt to the functional context, in which they need to be operational (7). For instance, during inflammation, Foxp3+ Treg cells upregulate pathways that secure the preservation of their identity as Treg cells but might have additional (as yet unknown) functions for the establishment of tissue-resident Treg cell subsets. Second, Treg cells do not only reside in secondary lymphoid tissue but also in NLT. These “tissue-resident” Treg cells are distinct from circulating lymphoid tissue Treg cells and might in some cases populate distinct tissue niches (8). Limited information exists as to whether tissue-resident Treg cells are differentially recruited from the systemic repertoire or whether their functions are imprinted in situ in their particular niche. Also, their TCR repertoire and the role of antigen for their maintenance is not known. Finally, they might exert “non-canonical” functions in these tissues that do not have anything to do with the regulation of immune responses in the first place but with tissue development and organ homeostasis. In this review, we will discuss some of these aspects in the central nervous system (CNS) and in those peripheral organs where Treg cells have been investigated in non-lymphoid tissue niches.
Stability of Foxp3 Treg Cells in the CNS in the Context of Autoimmunity
Treg cells are crucial for the regulation of autoimmune inflammation in the CNS. Depletion of Treg cells lowers the threshold for autoimmune CNS inflammation in individuals whose T cell receptor repertoire contains large fractions of CNS reactive T cells (9). Moreover, depletion of Treg cells prior to or after onset of experimental autoimmune encephalomyelitis (EAE) worsens the disease and prevents recovery (10–12).
Since it is clear that Foxp3+ Treg cells are recruited to the target tissue of autoimmune reactions not only in the CNS (13, 14) but also in other organs including the joints (15), the pancreas (16), or the skin (17, 18), a major area of investigation in Treg cell biology in the recent years has been their stability in an inflammatory environment. Since it has been recognized that Foxp3+ Treg cells are recruited directly to the site of inflammation, Treg cells must dispose of active mechanisms of resilience to maintain their functional phenotype in spite of inflammatory cues in their environment. A variety of pathways have been described, which all ultimately result in keeping the expression of Foxp3 at high levels when factors of the inflammatory milieu activate pathways that otherwise would destabilize Foxp3 expression. The overarching concept is that Foxp3 interacts with (16–19) or is co-expressed with various combinations of transcription factors in Treg cells to induce an effector Treg (eTreg) program and to adapt to the quality of the inflammatory response that is supposed to be controlled by these Treg cells (19–21) while at the same time preserving their identity as Treg cells. Here, direct transactivators of Foxp3 as well as transcriptional inhibitors of effector T cell programs have been described (Table 1).
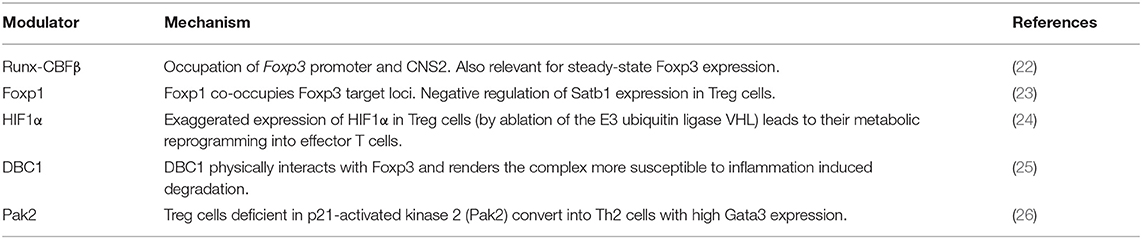
Table 1. Selection of molecules directly involved in the transcriptional regulation of Foxp3 in murine NLT Treg cells.
Moreover, the significance of epigenetic modifications both of the chromatin in the vicinity of the Foxp3 locus and of the Foxp3 locus itself in regulating the expression of Foxp3 in distinct milieus is increasingly appreciated (27, 28). In addition to the promoter of Foxp3, three conserved non-coding regions (CNS1-3) have been identified in the Foxp3 locus, whose methylation status determines the efficacy with which Foxp3 is transcribed since for instance, Ets-1 transcription factors only bind to CNS2 [i.e., the conserved non-coding sequence in the first intron of the Foxp3 locus that has also been termed Treg specific demethylated region (TSDR) (29)] in its demethylated state and thus increase the enhancer activity of CNS2 for Foxp3 (30).
During local inflammation, the central nervous system milieu represents a particular challenge to the identity and function of eTreg cells. The most relevant molecular mechanisms that preserve the “identity” of Treg cells (e.g., their sustained expression of Foxp3) have been a matter of debate. Recently, it has been shown that both TCR/Irf4 signaling and NFκB signaling are required independently of each other to establish the eTreg cell transcriptional program (31, 32); and the transcriptional modifier Blimp1 is a master controller of the eTreg program in Treg cells (33). Loss of Blimp1 in Treg cells in steady state does not produce an inflammatory phenotype, most likely due to the fact that steady state Treg cells in secondary lymphoid tissue only express low levels of Blimp1. In contrast, loss of Blimp1 in Treg cells in the inflamed CNS or in the colon has major consequences: First, these NLT Treg cells lose their effector Treg phenotype. For instance, since Blimp1 is a direct transactivator of Il10, Blimp1 deficient Treg cells are unable to produce IL-10 (34–36). Second, Blimp1 is also required to maintain the very identity of Treg cells in an inflammatory milieu and loss of Blimp1 eventually leads to downregulation of Foxp3 expression (35). Mechanistically, Foxp3 is not a transcriptional target of Blimp1. Rather Blimp1 appears to be controlling the expression of Foxp3 in an indirect manner by preventing the inflammatory environment (and particularly IL-6) from methylating CNS2. In fact, Blimp1 suppresses the expression of the methyl transferase Dnmt3a in Treg cells in an inflammatory environment (35).
In principle, genomic CpG islands are methylated and demethylated by DNA methyltransferases and Tet methylcytosine dioxygenases, respectively (37). The modulation of the demethylating enzymes Tet2 and Tet3 in Treg cells affects CNS2 methylation and Treg cell stability (38). Notably, the activity of the Tet enzymes might be controlled by intermediates of the mitochondrial tricarboxylic acid cycle. In fact, ablation of the mitochondrial transcription factor A (Tfam) leads to altered succinate/α-ketoglutarate and fumarate/α-ketoglutarate levels in Treg cells—and as a consequence—to reduced activity of the Tet enzymes and a failure to maintain the demethylated state of CNS2 (39). By this mechanism, intermediary metabolism in Treg cells is coupled to their stability. Conversely, DNA methyltransferases (and in particular Dnmt1 and Dnmt3a) are able to methylate CNS2 (and other CpG islands in the Foxp3 locus) and thus dampen Foxp3 expression. Here, an interesting model has recently been suggested (40): STAT5 (downstream of IL-2) binds to CNS2 irrespectively of the methylation status of CNS2 and increases its enhancer activity as to the expression of Foxp3 (41). Both STAT3 (downstream of IL-6) and STAT6 (downstream of IL-4) can compete with STAT5 for its binding sites in CNS2. In contrast to STAT5, both STAT3 and (more prominently) STAT6 appear to physically interact with Dnmt1 and Dnmt3a (40, 42), and thus can mediate their recruitment to CNS2. Methylation of CNS2 by these methyl transferases would then lead to the silencing of Foxp3. This model provides a rationale for inflammation (IL-4 and IL-6) induced downregulation of Foxp3 in Treg cells and IL-2 dependent counteraction of this process.
Apart from epigenetic modulation of the Foxp3 locus itself, histone modifications also control Foxp3 transcription. Interestingly, partial methylation of CNS2, which occurs in Treg cells exposed to an inflammatory environment, results in the recruitment of methyl CpG binding protein 2 (MeCP2) to the methylated CpG islands of CNS2. MeCP2 in turn leads to the acetylation of H3, which reinforces Foxp3 transcription (43). Also, Ezh2, a chromatin modifying enzyme that catalyzes the trimethylation of H3K27, is co-expressed in Treg cells in response to CD28 stimulation. Foxp3 cooperates with Ezh2 to repress its target genes, and in the absence of Ezh2, Foxp3 is still present in Treg cells but fails to reinforce its transcriptional program (44).
Taken together, the stability of Foxp3+ Tregs at sites of inflammation is instructed by an active process. Treg cell extrinsic cues (TCR stimulation, co-stimulation, cytokines) are required to secure the stability of Treg cells and both direct transcriptional as well as epigenetic mechanisms are involved in maintaining high levels of Foxp3 expression and thus the functional phenotype of Treg cells in inflamed tissues.
Maintenance of NLT Foxp3+ Treg Cells During and After Inflammation
Compelling evidence suggests that IL-2 is a non-redundant growth factor of Treg cells in secondary lymphoid tissues. Treg cells do not produce IL-2 themselves but rely on extrinsic sources of IL-2, which is mostly provided by conventional T cells. Lack of IL-2 leads to the attrition of Treg cells and to the development of multi-organ autoimmunity (45). Mechanistically, IL-2 counteracts the apoptosis prone transcriptional program of Treg cells (46). It is quite likely that during organ specific inflammation—due to massive infiltration of conventional T cells—the source of IL-2 is sufficient also in NLTs to fuel the maintenance and even expansion of Foxp3+ Treg cells (47). In fact, a decrease in the threshold of IL-2 responsiveness in Treg cells or more efficient STAT5 signaling, which can be modulated by a plethora of mechanisms (23, 40, 48), have been identified as a key “stability” mechanism of Treg cells in NLTs under conditions of limited availability of IL-2 (see above).
However, after the contraction of the effector T cell population in the target tissue of the inflammation, the source of IL-2 (and thus the relevant growth factor for Treg cells) becomes limiting. As a consequence, the Treg cell population in NLTs will also contract (47). However, we and others have observed that after a traumatic or inflammatory event in the central nervous system (but also in other tissues), Foxp3+ Treg cells remain in the central nervous system for extended periods of time and might even establish a population of resident Treg cells in the relative absence of conventional T cells (13). Little is known about this “meta-homeostatic” Treg cell population (Figure 1). Previous studies suggested that Treg cells which infiltrate the CNS during EAE were most likely exclusively thymus-derived and not peripherally induced from conventional T cells (13). In particular, as mice, which are unable to generate peripherally induced Treg cells due to a mutation of the Foxp3 locus lacking CNS1 but have regular numbers of thymus derived Treg cells, do not have an EAE phenotype, Treg cells in the inflamed central nervous system must be thymus-derived (49). However, it is an open question how this NLT Treg cell population is selected or whether its specific properties are locally imprinted and also how it is maintained.
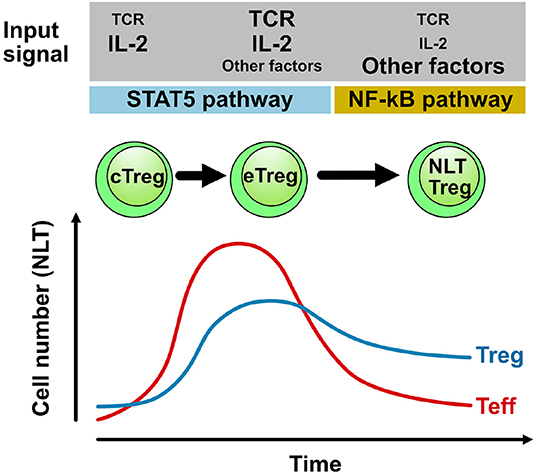
Figure 1. Population dynamics and maintenance of Treg cells in NLTs. During an adaptive immune response in NLT (e.g., in the central nervous system during EAE) conventional T cells (Teff) infiltrate the target tissue and expand providing sufficient IL-2 to also drive the differentiation and expansion of Treg cells. After contraction of the conventional effector T cell (Teff) population (due to active regulation by Treg cells), IL-2 becomes limiting and persisting Treg cells likely depend on alternative signals for self-renewal. Here, signals that activate the NF-κB pathway might be important cues not only for NLT function but also for their maintenance (31). TCR triggering of Treg cells and downstream modulation of the TCR signal by the transcriptional modulator IRF4 is a non-redundant event in the differentiation of central Treg cells (cTreg) in secondary lymphoid tissues into effector Treg cells (eTreg) in the inflamed tissue. The requirement of the TCR signal and other cues in NLT Treg cells that reside in specific niches over extended periods of time needs to be determined and likely depends on the anatomical niche that might then also drive a distinct functional specialization of NLT Treg cells.
In skin and colon NLT Treg cells have been characterized by scRNAseq as to a specific “barrier tissue” transcriptional program and as to their provenance and development (in pseudotime analyses) (50). By analyzing NLT Treg cells, it has been possible to define a transcriptional program reminiscent of the effector Treg (eTreg) phenotype that has been coined for Treg cells isolated from the visceral adipose tissue (33, 51). While definitive proof by provenance mapping systems is still lacking, based on these scRNAseq analysis, the instruction of a Treg cell trait consistent with tissue residency might be initiated in secondary lymphoid tissues. Further imprinting of functional states then occurs in the NLT (Figure 2). Best evidence for this model has been presented for VAT Treg cells: By using PPAR-γ reporter mice, a previous study has identified a small population of PPAR-γlow expressing Treg cells in secondary lymphoid organs (SLO), in which part of the VAT Treg transcriptional program, in particular those genes associated with Treg activation, was already active, and these cells were shown to be capable of adopting the full VAT Treg signature upon their migration into the tissue (52), most likely in response to local tissue-specific cues. Based on this finding, it was proposed that the bona-fida VAT Treg gene signature/phenotype might be adopted in a sequential, step-wise process, that is initiated in the SLO and then finalized within the non-lymphoid compartment. The first step appears to be a “priming” step in SLOs, where Treg cells receive an activating cue, e.g., in response to cognate self antigen recognition. Then they gain the capacity to infiltrate into NLTs. In a second step, Treg cells that re-encounter their specific antigen in the NLT, might be preferentially retained in situ and receive further tissue-specific cues that impart the full NLT phenotype and function (52).
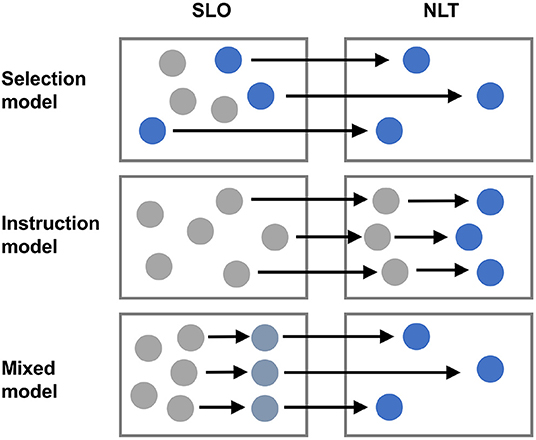
Figure 2. Heterogeneity of Treg cells in NLTs. Treg cells are heterogeneous and the NLT milieu is likely an important determinant of this heterogeneity. However, it is unclear whether specific hard-wired subsets of Treg cells are selectively recruited from SLOs to distinct NLTs (selection model) or whether Treg cells that enter a given NLT are locally instructed to adopt specific properties and specialize according to a niche specific program (instruction model). Alternatively, some degree of imprinting might occur in SLOs during priming and further commitment of Treg cells to certain tissue specific properties might then take place in NLTs (mixed model).
In particular, NFκB (RelA) signaling appears to be a hallmark of the eTreg cell [and NLT Treg cell) program. Whether TNF family members (including TNF-ligand related molecule 1 (31, 53)] or IL-1 family members [including IL-33 (51) and IL-18 (54)] are more crucial or differentially important to guide the NLT Treg program, remains to be determined. Also, it remains to be determined whether NFκB signaling into Treg cells secures their maintenance outside SLOs where IL-2 derived from conventional T cells, which is a major nutrient of Treg cells in lymphoid tissues, is scarce.
Very little is known about the “Treg cell growth and maintenance” factors in NLTs and whether they are universal for all peripheral tissues or private to distinct anatomical niches. The alarmin IL-33, a member of the IL-1 family of cytokines, plays a major role in driving the expansion/accumulation of VAT-resident Treg cells (51, 52, 55). IL-33 is constitutively expressed in the CNS even under physiological conditions (56). Given the high expression of its receptor ST2 (encoded by Il1rl1) on brain Treg cells and the observation that Treg cells fail to properly expand in the injured ischaemic brain in ST2- and IL-33-deficient mice (57), IL-33 might be a prominent candidate capable of substituting for the role IL-2 in mediating Treg cell survival in the post-inflammatory central nervous system. Another such candidate could be the neurotransmitter serotonin (5-HT). Its receptor 5-HT7 protein (encoded by Htr7) is specifically upregulated on Treg cells accumulating in the ischaemic brain (57), and it was demonstrated that both serotonin or the inhibition of its uptake could expand brain Treg cell numbers in vivo.
In summary, NLT Treg cells (in the central nervous system but also in other tissues) likely populate niches that provide a distinct environment for their survival. The molecular pathways that support their survival (and possible self renewal) are likely different from the IL-2 driven Treg cell maintenance system in secondary lymphoid organs.
Non-Canonical Functions of Treg Cells
While thymus-derived Treg cells that populate SLOs are non-redundant in maintaining self tolerance, eTreg cells in NLTs also clearly regulate effector responses of conventional T cells during adaptive immune responses in order to clear inflammation and reduce immune pathology. However, since Treg cells are found in NLTs in steady state and after re-establishment of homeostasis after any kind of injury (meta-homeostasis), it has been suggested that tissue intrinsic (non-immune) functions of these peripheral tissues might be controlled by local Treg cells (8).
Various NLTs, including barrier tissues like the skin, the colonic lamina propria and the lung, as well as non-barrier sites, like the visceral adipose tissue (VAT) of lean mice or the skeletal muscle are populated by a distinct population of regulatory T cells. As outlined above, NLT Treg cells show a high degree of phenotypic and functional adaptation to the NLT environment in which they reside and share a set of common NLT-specific transcripts that distinguish them from their counterparts in SLOs. The vast majority of NLT Treg cells display an elevated expression of genes typically associated with an eTreg phenotype, such as the effector molecules Ctla4, Icos, Klrg1, and GzmB. Despite a certain overlap in the transcriptomes between NLT Treg cells from different NLTs, there are a number of transcripts specifically and distinctively upregulated in Treg cells from particular non-lymphoid compartments, which might endow these NLT Treg cells with specialized functions in organ homeostasis.
For example, VAT Treg cells highly express the transcription factor peroxisome proliferator-activated receptor (PPAR)-γ, the master regulator of adipocyte differentiation. PPAR-γ governs much of the unique transcriptional signature of VAT Treg cells (58), including the expression of genes involved in lipid metabolism, such as Dgat1 (diacylglycerol O-acyltransferase 1), encoding an enzyme involved in triacylglycerol biosynthesis, Pcyt1a (choline-phosphate cytidylyl transferase A), which encodes an enzyme involved in phosphatidylcholine synthesis, as well as Cd36, encoding the lipid scavenger receptor CD36 (59). Specific deletion of PPAR-γ in Treg cells (using Foxp3-Cre x Ppargflox/flox mice) impairs their accumulation in VAT, but not in SLOs, and promotes an increased inflammatory state in the VAT associated with enhanced systemic insulin resistance (59).
In other NLTs, further functions of resident Treg cells have been described that are unrelated to the control of immune responses in the first place. For example, in the steady state skin, Foxp3+ Treg cells are localized in close proximity to hair follicles where they promote the proliferation of hair follicle stem cells and the telogen to anagen transition of the hair follicle by providing the Notch-ligand Jag1, which is sensed by hair follicle stem cells (60). In lung tissue and in muscle tissue, Treg cells contribute to tissue regeneration after injury by producing the epidermal growth factor receptor ligand amphiregulin, to which bronchial epithelial cells respond with proliferation and differentiation (54) and muscle satellite cells respond with myogenic differentiation (61), respectively.
In contrast to NLT such as the VAT, intestine, lung or skin, “immune-privileged” compartments like the central nervous system are largely devoid of Treg cells during steady state conditions. However, Treg cells readily accumulate in the CNS in response to acute local injury (e.g., hypoxia/stroke) (62) or autoimmune inflammation (13). Interestingly, Treg cell numbers remain elevated in the post-EAE central nervous system for a prolonged period of time, raising the question of whether these cells might also play a significant role in promoting tissue repair in the recovering central nervous system. In fact, Treg cells have already been shown to be capable of supporting myelin regeneration by promoting the differentiation of oligodendrocyte progenitor cells and their production of myelin in both the brain and the spinal cord in vivo in a model of toxic demyelination (lysolecithin or cuprizone injection) (63). Central nervous system Treg cells have a transcriptional profile that clearly differs from that of SLO Treg cells (35, 57), and in many ways resembles the profile of other NLT Treg populations. Microarray analysis of Treg cells that had infiltrated the brain after acute ischaemic injury revealed that these Treg cells appear to be transcriptionally related to VAT and skeletal muscle Treg cells, as demonstrated by the high level expression of genes that encode IL-10, amphiregulin (Areg); Klrg1, ST2, and PPAR-γ. In addition, brain Treg cells upregulate certain central nervous system-specific genes, such as neuropeptide Y (Npy), preproenkephalin (Penk), serotonin receptor type 7 (Htr7), and arginine vasopressin receptor (Avpr1a) (57). In particular the increased expression of the epidermal growth factor ligand amphiregulin by CNS recruited Treg cells seems important for neurological recovery as demonstrated by its capacity to suppress astrogliosis, neurological deficits and neurotoxic gene expression (57).
Therefore, NLT Treg cells might be private to their own niche of residence and intricately connected to tissue intrinsic non-immune processes in homeostasis and organ development.
Human NLT Treg Cells
NLT Treg cells have been extensively studied in mice, however it has become clear that essentially all human NLTs also harbor a distinct population of Treg cells (64, 65). The functional phenotype of human NLT Treg cells remains largely unexplored and it is possible that pathways identified as relevant for the maintenance and function of murine NLT Treg cells may not have the same importance for human NLT Treg cells. For example, omental fat tissue—the human correlate of murine VAT—has been reported to contain detectable levels of Foxp3 transcripts (58). Like their counterparts in mice, omental fat Treg cells have been shown to express ST2 (51) while other studies failed to detect ST2 expression on Foxp3+ Treg cells isolated from human omental adipose tissue and also other organs including colon and lung (66, 67), suggesting that the IL-33/ST2 axis may not be a universal pathway for the maintenance of human NLT Treg cells.
Treg cells are also abundant in healthy adult human skin, comprising about 20% of the total CD4+ T cell population. Human skin Treg cells exhibit an activated memory phenotype, as defined by their almost uniform expression of the memory markers CD45RO, CD27, and Bcl-2 and the increased expression of Foxp3 and Treg activation markers, such as CTLA-4, CD25, and ICOS relative to their counterparts in the peripheral blood. There is little overlap in the TCR repertoire between Treg cells and conventional T cells in healthy human skin, suggesting that these two NLT T cell populations recognize different antigens, similar to what has been observed for Treg cells and conventional T cells from murine VAT (68). Interestingly, similar to their murine equivalents (60), dermal Treg cells in normal human skin were found to preferentially reside in close proximity to hair follicles (68).
The currently available data suggest a considerable overlap in the expression of tissue-specific Treg cell markers between mice and humans, as demonstrated for skin- or gut-derived Treg cells (50). Also, the NLT core signature which is characterized by an enrichment of genes related to the TNFRSF-NF-κB pathway appears to be largely conserved between mouse and human Treg cells (50, 69), raising the possibility that human NLT Treg cells might undergo a similar process of local imprinting and tissue adaptation during their establishment in NLTs.
Perspective
Fundamental questions regarding NLT Treg cells are as yet unresolved: For example, during organ specific inflammatory responses, Treg cells that are recruited to the sites of inflammation are faced with issues of stability of their phenotype and deficiency in growth factors that they would otherwise be able to rely on in steady state in SLOs. Also, it is unclear whether subsets of Treg cells are pre-committed to certain phenotypes in the secondary lymphoid system and are then selectively recruited to certain anatomical niches where they expand or whether “naive” Treg cells are locally instructed to adopt a distinct phenotype only after the recruitment to specific organ systems. Answers to these questions will profoundly affect concepts of therapeutic interventions that intend to make use of tissue-resident Treg cells. It might in fact be oversimplified (and perhaps even dangerous) to aim for a “one-size-fits-all” Treg cell. Rather, more tailored strategies to either selectively expand subsets of Treg cells or to instruct them to adopt appropriate (organ-specific) features might have to be developed. Here, we face dramatic gaps in knowledge and a renewed interest in Treg cell centered therapeutic approaches that exploit the immune functions of Treg cells needs to consider the emerging profound organ specific heterogeneity of Foxp3+ Treg cells and their potential functions in tissue homeostasis and regeneration.
Author Contributions
TK conceptualized and wrote the review. AM wrote parts of the review and edited the text.
Funding
TK was funded by the Deutsche Forschungsgemeinschaft (SFB 1054, SFB TR 128, Synergy), by the German Ministry for Education and Research (KKNMS, T-B in NMO) and by the European Research Council (CoG 647215).
Conflict of Interest
The authors declare that the research was conducted in the absence of any commercial or financial relationships that could be construed as a potential conflict of interest.
References
1. Ohkura N, Kitagawa Y, Sakaguchi S. Development and maintenance of regulatory T cells. Immunity. (2013) 38:414–23. doi: 10.1016/j.immuni.2013.03.002
2. Sakaguchi S, Sakaguchi N, Asano M, Itoh M, Toda M. Immunologic self-tolerance maintained by activated T cells expressing IL-2 receptor alpha-chains (CD25). Breakdown of a single mechanism of self-tolerance causes various autoimmune diseases. J Immunol. (1995) 155:1151–64.
3. Kim JM, Rasmussen JP, Rudensky AY. Regulatory T cells prevent catastrophic autoimmunity throughout the lifespan of mice. Nat Immunol. (2007) 8:191–7. doi: 10.1038/ni1428
4. Brunstein CG, Miller JS, McKenna DH, Hippen KL, DeFor TE, Sumstad D, et al. Umbilical cord blood-derived T regulatory cells to prevent GVHD: kinetics, toxicity profile, and clinical effect. Blood. (2016) 127:1044–51. doi: 10.1182/blood-2015-06-653667
5. Bluestone JA, Buckner JH, Fitch M, Gitelman SE, Gupta S, Hellerstein MK, et al. Type 1 diabetes immunotherapy using polyclonal regulatory T cells. Sci Transl Med. (2015) 7:315ra189. doi: 10.1126/scitranslmed.aad4134
6. Bluestone JA, Tang Q. Treg cells-the next frontier of cell therapy. Science. (2018) 362:154–5. doi: 10.1126/science.aau2688
7. Littman DR, Rudensky AY. Th17 and regulatory T cells in mediating and restraining inflammation. Cell. (2010) 140:845–58. doi: 10.1016/j.cell.2010.02.021
8. Panduro M, Benoist C, Mathis D. Tissue Tregs. Annu Rev Immunol. (2016) 34:609–33. doi: 10.1146/annurev-immunol-032712-095948
9. Lowther DE, Chong DL, Ascough S, Ettorre A, Ingram RJ, Boyton RJ, et al. Th1 not Th17 cells drive spontaneous MS-like disease despite a functional regulatory T cell response. Acta Neuropathol. (2013) 126:501–15. doi: 10.1007/s00401-013-1159-9
10. Kohm AP, Carpentier PA, Anger HA, Miller SD. Cutting edge: CD4+CD25+ regulatory T cells suppress antigen-specific autoreactive immune responses and central nervous system inflammation during active experimental autoimmune encephalomyelitis. J Immunol. (2002) 169:4712–6. doi: 10.4049/jimmunol.169.9.4712
11. Mcgeachy MJ, Stephens LA, Anderton SM. Natural recovery and protection from autoimmune encephalomyelitis: contribution of CD4+CD25+ regulatory cells within the central nervous system. J Immunol. (2005) 175:3025–32. doi: 10.4049/jimmunol.175.5.3025
12. Koutrolos M, Berer K, Kawakami N, Wekerle H, Krishnamoorthy G. Treg cells mediate recovery from EAE by controlling effector T cell proliferation and motility in the CNS. Acta Neuropathol Commun. (2014) 2:163. doi: 10.1186/s40478-014-0163-1
13. Korn T, Reddy J, Gao W, Bettelli E, Awasthi A, Petersen TR, et al. Myelin-specific regulatory T cells accumulate in the CNS but fail to control autoimmune inflammation. Nat Med. (2007) 13:423–31. doi: 10.1038/nm1564
14. O'Connor RA, Malpass KH, Anderton SM. The inflamed central nervous system drives the activation and rapid proliferation of Foxp3+ regulatory T cells. J Immunol. (2007) 179:958–66. doi: 10.4049/jimmunol.179.2.958
15. Raghavan S, Cao D, Widhe M, Roth K, Herrath J, Engstrom M, et al. FOXP3 expression in blood, synovial fluid and synovial tissue during inflammatory arthritis and intra-articular corticosteroid treatment. Ann Rheum Dis. (2009) 68:1908–15. doi: 10.1136/ard.2008.100768
16. Tang Q, Adams JY, Penaranda C, Melli K, Piaggio E, Sgouroudis E, et al. Central role of defective interleukin-2 production in the triggering of islet autoimmune destruction. Immunity. (2008) 28:687–97. doi: 10.1016/j.immuni.2008.03.016
17. Sugiyama H, Gyulai R, Toichi E, Garaczi E, Shimada S, Stevens SR, et al. Dysfunctional blood and target tissue CD4+CD25high regulatory T cells in psoriasis: mechanism underlying unrestrained pathogenic effector T cell proliferation. J Immunol. (2005) 174:164–73. doi: 10.4049/jimmunol.174.1.164
18. Hirahara K, Liu L, Clark RA, Yamanaka K-I, Fuhlbrigge RC, Kupper TS. The majority of human peripheral blood CD4+CD25highFoxp3+ regulatory T cells bear functional skin-homing receptors. J Immunol. (2006) 177:4488–94. doi: 10.4049/jimmunol.177.7.4488
19. Koch MA, Tucker-Heard G, Perdue NR, Killebrew JR, Urdahl KB, Campbell DJ. The transcription factor T-bet controls regulatory T cell homeostasis and function during type 1 inflammation. Nat Immunol. (2009) 10:595–602. doi: 10.1038/ni.1731
20. Chaudhry A, Rudra D, Treuting P, Samstein RM, Liang Y, Kas A, et al. CD4+ regulatory T cells control TH17 responses in a Stat3-dependent manner. Science. (2009) 326:986–91. doi: 10.1126/science.1172702
21. Zheng Y, Chaudhry A, Kas A, Deroos P, Kim JM, Chu T-T, et al. Regulatory T-cell suppressor program co-opts transcription factor IRF4 to control T(H)2 responses. Nature. (2009) 458:351–6. doi: 10.1038/nature07674
22. Rudra D, Egawa T, Chong MMW, Treuting P, Littman DR, Rudensky AY. Runx-CBFbeta complexes control expression of the transcription factor Foxp3 in regulatory T cells. Nat Immunol. (2009) 10:1170–7. doi: 10.1038/ni.1795
23. Konopacki C, Pritykin Y, Rubtsov Y, Leslie CS, Rudensky AY. Transcription factor Foxp1 regulates Foxp3 chromatin binding and coordinates regulatory T cell function. Nat Immunol. (2019) 20:232–42. doi: 10.1038/s41590-018-0291-z
24. Lee JH, Elly C, Park Y, Liu Y-C. E3 ubiquitin ligase VHL regulates hypoxia-inducible factor-1α to maintain regulatory T cell stability and suppressive capacity. Immunity. (2015) 42:1062–74. doi: 10.1016/j.immuni.2015.05.016
25. Gao Y, Tang J, Chen W, Li Q, Nie J, Lin F, et al. Inflammation negatively regulates FOXP3 and regulatory T-cell function via DBC1. Proc Natl Acad Sci USA. (2015) 112:E3246–54. doi: 10.1073/pnas.1421463112
26. O'Hagan KL, Miller SD, Phee H. Pak2 is essential for the function of Foxp3+ regulatory T cells through maintaining a suppressive Treg phenotype. Sci Rep. (2017) 7:17097. doi: 10.1038/s41598-017-17078-7
27. Huehn J, Polansky JK, Hamann A. Epigenetic control of FOXP3 expression: the key to a stable regulatory T-cell lineage? Nat Rev Immunol. (2009) 9:83–9. doi: 10.1038/nri2474
28. Morikawa H, Sakaguchi S. Genetic and epigenetic basis of Treg cell development and function: from a FoxP3-centered view to an epigenome-defined view of natural Treg cells. Immunol Rev. (2014) 259:192–205. doi: 10.1111/imr.12174
29. Floess S, Freyer J, Siewert C, Baron U, Olek S, Polansky J, et al. Epigenetic control of the foxp3 locus in regulatory T cells. PLoS Biol. (2007) 5:e38. doi: 10.1371/journal.pbio.0050038
30. Polansky JK, Schreiber L, Thelemann C, Ludwig L, Krüger M, Baumgrass R, et al. Methylation matters: binding of Ets-1 to the demethylated Foxp3 gene contributes to the stabilization of Foxp3 expression in regulatory T cells. J Mol Med. (2010) 88:1029–40. doi: 10.1007/s00109-010-0642-1
31. Vasanthakumar A, Liao Y, Teh P, Pascutti MF, Oja AE, Garnham AL, et al. The TNF receptor superfamily-NF-κB axis is critical to maintain effector regulatory T cells in lymphoid and non-lymphoid tissues. Cell Rep. (2017) 20:2906–20. doi: 10.1016/j.celrep.2017.08.068
32. Rosenbaum M, Gewies A, Pechloff K, Heuser C, Engleitner T, Gehring T, et al. Bcl10-controlled Malt1 paracaspase activity is key for the immune suppressive function of regulatory T cells. Nat Commun. (2019) 10:2352. doi: 10.1038/s41467-019-10203-2
33. Cretney E, Xin A, Shi W, Minnich M, Masson F, Miasari M, et al. The transcription factors Blimp-1 and IRF4 jointly control the differentiation and function of effector regulatory T cells. Nat Immunol. (2011) 12:304–11. doi: 10.1038/ni.2006
34. Heinemann C, Heink S, Petermann F, Vasanthakumar A, Rothhammer V, Doorduijn E, et al. IL-27 and IL-12 oppose pro-inflammatory IL-23 in CD4(+) T cells by inducing Blimp1. Nat Commun. (2014) 5:3770. doi: 10.1038/ncomms4770
35. Garg G, Muschaweckh A, Moreno H, Vasanthakumar A, Floess S, Lepennetier G, et al. Blimp1 prevents methylation of Foxp3 and loss of regulatory T cell identity at sites of inflammation. Cell Rep. (2019) 26:1854–68 e5. doi: 10.1016/j.celrep.2019.01.070
36. Ogawa C, Bankoti R, Nguyen T, Hassanzadeh-Kiabi N, Nadeau S, Porritt RA, et al. Blimp-1 functions as a molecular switch to prevent inflammatory activity in Foxp3+RORγt+ regulatory T cells. Cell Rep. (2018) 25:19–28 e5. doi: 10.1016/j.celrep.2018.09.016
37. Lyko F. The DNA methyltransferase family: a versatile toolkit for epigenetic regulation. Nat Rev Genet. (2018) 19:81–92. doi: 10.1038/nrg.2017.80
38. Yue X, Lio C-WJ, Samaniego-Castruita D, Li X, Rao A. Loss of TET2 and TET3 in regulatory T cells unleashes effector function. Nat Commun. (2019) 10:2011. doi: 10.1038/s41467-019-09541-y
39. Weinberg SE, Singer BD, Steinert EM, Martinez CA, Mehta MM, Martínez-Reyes I, et al. Mitochondrial complex III is essential for suppressive function of regulatory T cells. Nature. (2019) 565:495–9. doi: 10.1038/s41586-018-0846-z
40. Feng Y, Arvey A, Chinen T, van der Veeken J, Gasteiger G, Rudensky AY. Control of the inheritance of regulatory T cell identity by a cis element in the Foxp3 locus. Cell. (2014) 158:749–63. doi: 10.1016/j.cell.2014.07.031
41. Ogawa C, Tone Y, Tsuda M, Peter C, Waldmann H, Tone M. TGF-β-mediated Foxp3 gene expression is cooperatively regulated by Stat5, Creb, and AP-1 through CNS2. J Immunol. (2014) 192:475–83. doi: 10.4049/jimmunol.1301892
42. Zhang Q, Wang HY, Marzec M, Raghunath PN, Nagasawa T, Wasik MA. STAT3- and DNA methyltransferase 1-mediated epigenetic silencing of SHP-1 tyrosine phosphatase tumor suppressor gene in malignant T lymphocytes. Proc Natl Acad Sci USA. (2005) 102:6948–53. doi: 10.1073/pnas.0501959102
43. Li C, Jiang S, Liu S-Q, Lykken E, Zhao L-T, Sevilla J, et al. MeCP2 enforces Foxp3 expression to promote regulatory T cells' resilience to inflammation. Proc Natl Acad Sci USA. (2014) 111:E2807–16. doi: 10.1073/pnas.1401505111
44. DuPage M, Chopra G, Quiros J, Rosenthal WL, Morar MM, Holohan D, et al. The chromatin-modifying enzyme Ezh2 is critical for the maintenance of regulatory T cell identity after activation. Immunity. (2015) 42:227–38. doi: 10.1016/j.immuni.2015.01.007
45. Malek TR, Bayer AL. Tolerance, not immunity, crucially depends on IL-2. Nat Rev Immunol. (2004) 4:665–74. doi: 10.1038/nri1435
46. Pierson W, Cauwe B, Policheni A, Schlenner SM, Franckaert D, Berges J, et al. Antiapoptotic Mcl-1 is critical for the survival and niche-filling capacity of Foxp3+ regulatory T cells. Nat Immunol. (2013) 14:959–65. doi: 10.1038/ni.2649
47. Liston A, Gray DHD. Homeostatic control of regulatory T cell diversity. Nat Rev Immunol. (2014) 14:154–65. doi: 10.1038/nri3605
48. Liu B, Salgado OC, Singh S, Hippen KL, Maynard JC, Burlingame AL, et al. The lineage stability and suppressive program of regulatory T cells require protein O-GlcNAcylation. Nat Commun. (2019) 10:354. doi: 10.1038/s41467-019-08300-3
49. Josefowicz SZ, Niec RE, Kim HY, Treuting P, Chinen T, Zheng Y, et al. Extrathymically generated regulatory T cells control mucosal TH2 inflammation. Nature. (2012) 482:395–9. doi: 10.1038/nature10772
50. Miragaia RJ, Gomes T, Chomka A, Jardine L, Riedel A, Hegazy AN, et al. Single-cell transcriptomics of regulatory T cells reveals trajectories of tissue adaptation. Immunity. (2019) 50:493–504 e7. doi: 10.1016/j.immuni.2019.01.001
51. Vasanthakumar A, Moro K, Xin A, Liao Y, Gloury R, Kawamoto S, et al. The transcriptional regulators IRF4, BATF and IL-33 orchestrate development and maintenance of adipose tissue-resident regulatory T cells. Nat Immunol. (2015) 16:276–85. doi: 10.1038/ni.3085
52. Li C, DiSpirito JR, Zemmour D, Spallanzani RG, Kuswanto W, Benoist C, et al. TCR transgenic mice reveal stepwise, multi-site acquisition of the distinctive fat-Treg phenotype. Cell. (2018) 174:285–99 e12. doi: 10.1016/j.cell.2018.05.004
53. Malhotra N, Leyva-Castillo JM, Jadhav U, Barreiro O, Kam C, O'Neill NK, et al. RORα-expressing T regulatory cells restrain allergic skin inflammation. Sci Immunol. (2018) 3:eaao6923. doi: 10.1126/sciimmunol.aao6923
54. Arpaia N, Green JA, Moltedo B, Arvey A, Hemmers S, Yuan S, et al. A distinct function of regulatory T cells in tissue protection. Cell. (2015) 162:1078–89. doi: 10.1016/j.cell.2015.08.021
55. Kolodin D, van Panhuys N, Li C, Magnuson AM, Cipolletta D, Miller CM, et al. Antigen- and cytokine-driven accumulation of regulatory T cells in visceral adipose tissue of lean mice. Cell Metab. (2015) 21:543–57. doi: 10.1016/j.cmet.2015.03.005
56. Gadani SP, Walsh JT, Smirnov I, Zheng J, Kipnis J. The glia-derived alarmin IL-33 orchestrates the immune response and promotes recovery following CNS injury. Neuron. (2015) 85:703–9. doi: 10.1016/j.neuron.2015.01.013
57. Ito M, Komai K, Mise-Omata S, Iizuka-Koga M, Noguchi Y, Kondo T, et al. Brain regulatory T cells suppress astrogliosis and potentiate neurological recovery. Nature. (2019) 565:246–50. doi: 10.1038/s41586-018-0824-5
58. Feuerer M, Herrero L, Cipolletta D, Naaz A, Wong J, Nayer A, et al. Lean, but not obese, fat is enriched for a unique population of regulatory T cells that affect metabolic parameters. Nat Med. (2009) 15:930–9. doi: 10.1038/nm.2002
59. Cipolletta D, Feuerer M, Li A, Kamei N, Lee J, Shoelson SE, et al. PPAR-γ is a major driver of the accumulation and phenotype of adipose tissue Treg cells. Nature. (2012) 486:549–53. doi: 10.1038/nature11132
60. Ali N, Zirak B, Rodriguez RS, Pauli ML, Truong H-A, Lai K, et al. Regulatory T cells in skin facilitate epithelial stem cell differentiation. Cell. (2017) 169:1119–29 e11. doi: 10.1016/j.cell.2017.05.002
61. Burzyn D, Kuswanto W, Kolodin D, Shadrach JL, Cerletti M, Jang Y, et al. A special population of regulatory T cells potentiates muscle repair. Cell. (2013) 155:1282–95. doi: 10.1016/j.cell.2013.10.054
62. Liesz A, Suri-Payer E, Veltkamp C, Doerr H, Sommer C, Rivest S, et al. Regulatory T cells are key cerebroprotective immunomodulators in acute experimental stroke. Nat Med. (2009) 15:192–9. doi: 10.1038/nm.1927
63. Dombrowski Y, O'Hagan T, Dittmer M, Penalva R, Mayoral SR, Bankhead P, et al. Regulatory T cells promote myelin regeneration in the central nervous system. Nat Neurosci. (2017) 20:674–80. doi: 10.1038/nn.4528
64. Pesenacker AM, Broady R, Levings MK. Control of tissue-localized immune responses by human regulatory T cells. Eur J Immunol. (2015) 45:333–43. doi: 10.1002/eji.201344205
65. Thome JJC, Bickham KL, Ohmura Y, Kubota M, Matsuoka N, Gordon C, et al. Early-life compartmentalization of human T cell differentiation and regulatory function in mucosal and lymphoid tissues. Nat Med. (2016) 22:72–7. doi: 10.1038/nm.4008
66. Wu D, Han JM, Yu X, Lam AJ, Hoeppli RE, Pesenacker AM, et al. Characterization of regulatory T cells in obese omental adipose tissue in humans. Eur J Immunol. (2019) 49:336–47. doi: 10.1002/eji.201847570
67. Lam AJ, MacDonald KN, Pesenacker AM, Juvet SC, Morishita KA, Bressler B, et al. Innate control of tissue-reparative human regulatory T cells. J Immunol. (2019) 202:2195–209. doi: 10.4049/jimmunol.1801330
68. Sanchez Rodriguez R, Pauli ML, Neuhaus IM, Yu SS, Arron ST, Harris HW, et al. Memory regulatory T cells reside in human skin. J Clin Invest. (2014) 124:1027–36. doi: 10.1172/JCI72932
Keywords: Treg—regulatory T cell, Foxp3, heterogeneity, central nervous system, stability, non-lymphoid tissues
Citation: Korn T and Muschaweckh A (2019) Stability and Maintenance of Foxp3+ Treg Cells in Non-lymphoid Microenvironments. Front. Immunol. 10:2634. doi: 10.3389/fimmu.2019.02634
Received: 05 August 2019; Accepted: 24 October 2019;
Published: 14 November 2019.
Edited by:
Lucy S. K. Walker, University College London, United KingdomReviewed by:
Adrian Liston, Flanders Institute for Biotechnology, BelgiumMegan K. Levings, University of British Columbia, Canada
Copyright © 2019 Korn and Muschaweckh. This is an open-access article distributed under the terms of the Creative Commons Attribution License (CC BY). The use, distribution or reproduction in other forums is permitted, provided the original author(s) and the copyright owner(s) are credited and that the original publication in this journal is cited, in accordance with accepted academic practice. No use, distribution or reproduction is permitted which does not comply with these terms.
*Correspondence: Thomas Korn, dGhvbWFzLmtvcm4mI3gwMDA0MDt0dW0uZGU=