- 1Laboratory of Dr. Joan W. Berman, Department of Pathology, Albert Einstein College of Medicine, Bronx, NY, United States
- 2Laboratory of Dr. Joan W. Berman, Department of Microbiology and Immunology, Albert Einstein College of Medicine, Bronx, NY, United States
HIV associated neurocognitive disorders (HAND) are a group of neurological deficits that affect approximately half of people living with HIV (PLWH) despite effective antiretroviral therapy (ART). There are currently no reliable molecular biomarkers or treatments for HAND. Given the national opioid epidemic, as well as illegal and prescription use of opioid drugs among PLWH, it is critical to characterize the molecular interactions between HIV and opioids in cells of the CNS. It is also important to study the role of opioid substitution therapies in the context of HIV and CNS damage in vitro and in vivo. A major mechanism contributing to HIV neuropathogenesis is chronic, low-level inflammation in the CNS. HIV enters the brain within 4–8 days after peripheral infection and establishes CNS reservoirs, even in the context of ART, that are difficult to identify and eliminate. Infected cells, including monocytes, macrophages, and microglia, produce chemokines, cytokines, neurotoxic mediators, and viral proteins that contribute to chronic inflammation and ongoing neuronal damage. Opioids have been shown to impact these immune cells through a variety of molecular mechanisms, including opioid receptor binding and cross desensitization with chemokine receptors. The effects of opioid use on cognitive outcomes in individuals with HAND in clinical studies is variable, and thus multiple biological mechanisms are likely to contribute to the complex relationship between opioids and HIV in the CNS. In this review, we will examine what is known about both HIV and opioid mediated neuropathogenesis, and discuss key molecular processes that may be impacted by HIV and opioids in the context of neuroinflammation and CNS damage. We will also assess what is known about the effects of ART on these processes, and highlight areas of study that should be addressed in the context of ART.
Introduction
People living with HIV (PLWH) now have much longer lifespans, and the incidence of AIDS related deaths has declined due to the advent of antiretroviral therapy (ART) and increased access to HIV care (1). Despite ART, HIV-1 (HIV) remains a major public health issue with 37 million people infected globally and over 38,000 new annual cases reported in the United States in 2017 (2). Currently, there is a major opioid abuse epidemic closely associated with HIV (3, 4). It is estimated that 2 million people in the United States have opioid use disorder (OUD), which is characterized by the abuse of prescription opioids and/or heroin that is commonly taken intravenously (4–6). Intravenous (IV) drug use is a major route for HIV infection. From the onset of the HIV epidemic, IV drug use accounted for approximately 36% of HIV cases in the United States (7). Thus, the risk of HIV infection and transmission is high among IV drug abusers. It has been shown that addiction to prescription opioids can lead to risky behaviors and injection drug use that facilitate HIV acquisition, indicating that the opioid epidemic may help to perpetuate the HIV epidemic (3, 8, 9). Not only does opioid abuse increase the risk for HIV infection, but also it is estimated that 20–50% of PLWH are prescribed opioids and are more likely to have OUD than their uninfected counterparts (10). Long term opioid use increases the risk of death in PLWH compared to such use in uninfected people (11). Thus, it is imperative to understand the unique relationships between opioids and HIV infection, and importantly, their roles in contributing to HIV-associated comorbidities.
One highly significant comorbidity is HIV associated neurocognitive disorders (HAND). It is estimated that 15–55% of HIV infected individuals will develop some form of HAND despite ART (12–15). HAND is a spectrum of neurocognitive deficits and impacts many cognitive domains, for which there is no treatment or prevention (15). It significantly impacts quality of life and other health outcomes in PLWH (15). HAND is diagnosed by a battery of neurocognitive testing. The results from this testing are classified into three categories, asymptomatic neurocognitive impairment (ANI), mild neurocognitive disorder (MND), and HIV associated dementia (16, 17). Pre-ART, HAD was a prevalent form of HAND. Due to increased access to ART and HIV care, HAD is seen infrequently in developed nations and most HAND diagnoses fall in the ANI and MND categories (14, 16). Yet, the prevalence of HAND has not changed significantly from the pre-ART era (15, 17). Although individuals with ANI do not manifest deficits in everyday cognitive function, evidence suggests that a diagnosis of ANI can transition to one of the more severe forms of HAND (14, 18, 19). It was shown that PLWH with ANI had an increased risk of cognitive decline compared to PLWH who were not cognitively impaired (18).
Currently, there are no therapies to eliminate HAND. Therefore, it is imperative to understand mechanisms that contribute to its development and severity. Evidence suggests that opioids impact cognitive function and outcomes in PLWH, indicating that they contribute to HAND pathogenesis (7, 20–25). PLWH who chronically use opioids have worse cognitive outcomes compared to PLWH who do not abuse these drugs (21). There is also an association between lifetime use of heroin with worse recall and working memory (21). These data highlight the consequences of opioid abuse in PLWH, and underscore that opioid abuse likely contributes to HAND.
Opioids may contribute to HAND by altering immune cell functions (26). The ability of opioids to affect the functions of cells critical to HIV neuropathogenesis, infection, and protection is being studied extensively. In this review, we will examine the mechanisms by which opioids impact HIV neuropathogenesis and the development of HAND.
Effects of Opioids on Molecular Mechanisms That Mediate HIV Neuropathogenesis
Mechanisms That Contribute to HIV Neuropathogenesis
HIV enters the CNS within 4–8 days after peripheral infection, establishing viral reservoirs in the brain most often before someone is aware of their HIV status (27, 28). These reservoirs are difficult to eliminate and persist despite effective ART, which is now prescribed at the time of detected seropositivity (27–29). The long-term consequences of taking ART daily on mechanisms that mediate HIV neuropathogenesis are largely unknown.
HIV enters the brain through the transmigration of a mature subset of monocytes that expresses CD14, the LPS co-receptor, and CD16, the FCγIII receptor, across the blood brain barrier (BBB) (30, 31). Monocyte transmigration is a multistep process in response to chemokines including CCL2 and CXCL12 (32–34). CCL2 and CXCL12 are potent monocyte chemoattractant proteins elevated in the brains of HIV infected individuals despite effective ART (33–35). They are produced in the CNS, translocated across the BBB, and presented on the surface of brain microvascular endothelial cells (BMVEC) (36, 37). The binding of CCL2 to CCR2, and CXCL12 to CXCR4 and/or CXCR7, facilitates the firm arrest of monocytes to the endothelium (38, 39). This is mediated, in part, by the binding of activated lymphocyte function-associated antigen 1 (LFA-1) and very late antigen 4 (VLA-4) to intercellular adhesion molecule 1 (ICAM-1) and vascular cell adhesion molecule 1 (VCAM-1), respectively (38–40). This process is followed by crawling and diapedesis of monocytes across the BBB into the CNS (41). Diapedesis occurs by homophilic interactions between junctional proteins of the Ig superfamily, including activated leukocyte cell adhesion molecule (ALCAM) and junctional adhesion molecule A (JAM-A), expressed on the surface of both monocytes and BMVEC (42–45). ALCAM and JAM-A are increased on the surface of HIV-infected mature monocytes, facilitating their transmigration across the BBB (46). The effects of ART and opioids on these steps have not been extensively examined.
In the brain, HIV-infected mature monocytes infect and activate other cell types such as macrophages, microglia, and astrocytes to a lesser extent, and can differentiate into long-lived perivascular macrophages (46–48). This differentiation replenishes HIV CNS reservoirs, contributing to the development of HAND. HIV-infected and activated cells also facilitate recruitment of additional monocytes into the CNS, establishing and propagating chronic neuroinflammation (34, 49–51). While neurons do not become infected with HIV, they are impacted by viral proteins, cytokines, and neurotoxins, all of which contribute to neuronal damage and loss and the development of neurocognitive impairment (48, 52–54). Table 1 summarizes the effects of opioids and ART on the various cell types that contribute to HIV neuropathogenesis.
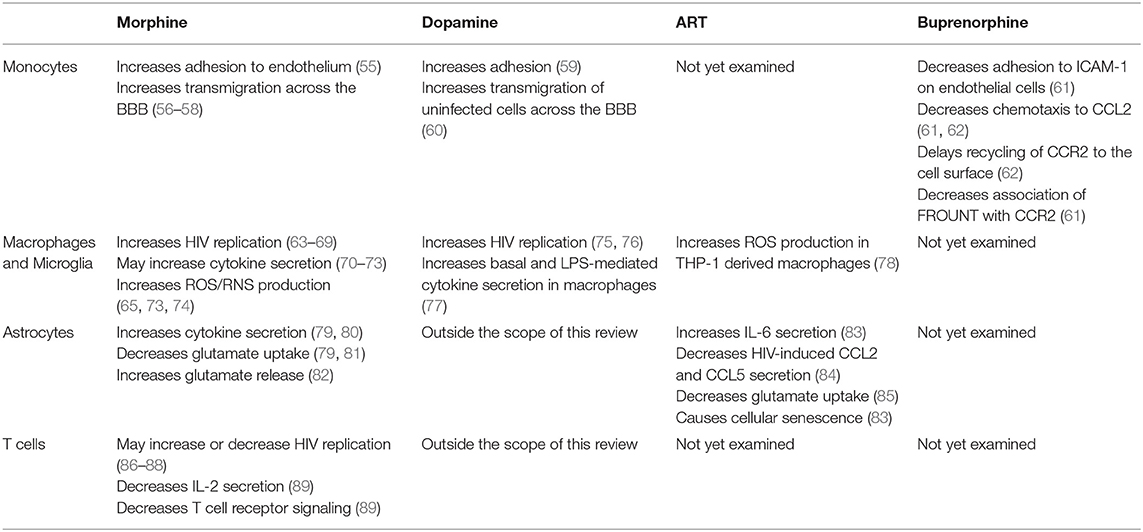
Table 1. Effects of opioids, dopamine, antiretroviral therapy (ART), and buprenorphine on cellular functions that contribute to HIV neuropathogenesis.
Another proposed mechanism that may contribute to HAND is immunosuppression due to HIV infection and chronic opioid usage. This can result in increased susceptibility to secondary infections and dissemination of bacteria into the CNS, increasing damage that causes neurocognitive dysfunction (26). These processes will not be discussed further as they are outside the scope of this review.
HIV, Opioids, and Monocyte Transmigration Across the Blood-Brain-Barrier
Data both in vitro and in animal models suggest that opioids increase the transmigration of monocytes across the BBB in the context of HIV (55–58, 90, 91). Mice exposed intravenously to morphine and HIV Tat have increased numbers of inflammatory monocytes in the CNS compared to placebo mice and mice treated with Tat alone (58). Research in macaques demonstrated that exposure to morphine and infection with SIV increases the number of monocytes and macrophages in the brain, potentially contributing to neuropathogenesis (57). Findings from in vitro studies substantiated these results by demonstrating that morphine increases PBMC and monocyte adhesion to the endothelium that is further enhanced with exposure to gp120 (55, 91). Together, these data suggest that morphine exacerbates HIV neuropathogenesis by increasing monocyte entry into the CNS. Additional studies are needed to determine how morphine specifically increases uninfected and HIV-infected human monocyte transmigration, especially in the context of ART.
Opioids may increase monocyte transmigration by impairing integrity of the BBB (90–93). In human BMVEC, morphine and Tat decreased tight junction proteins, ZO-1, JAM-2, and occludin, leading to decreased transendothelial electric resistance and increased PBMC transmigration (90). Another group demonstrated that morphine treatment of BMVEC for up to 72 h increased ICAM-1 and VCAM-1, facilitating adhesion of PBMC to BMVEC monolayers (91). Although this group also found that morphine did not impair endothelial permeability to FITC-labeled dextrans, other evidence suggests that more chronic morphine exposure may increase BBB permeability (92, 94). Experiments using fluorescently labeled dextrans showed that transgenic mice expressing Tat have increased BBB permeability compared to control mice, and that morphine treatment for 5–7 days similarly increased permeability (94). Interestingly, this increased permeability did not correlate with increased penetration of antiretrovirals into the brain. Morphine treatment was shown to decrease antiretroviral concentrations in the brain by increasing P-glycoprotein (94). This finding is underscored by studies in rats demonstrating that morphine treatment for 5 days increases P-glycoprotein in the hippocampus and cerebral cortex (93). Thus, decreased penetration of ART into the CNS may also exacerbate HIV neuropathogenesis. The impact of opioids and ART together on BBB permeability, junctional protein expression, and adhesion of monocytes to the endothelium remain important to characterize in future studies.
Opioids increase dopamine concentrations in the CNS, contributing to their euphoric effects (95–97). Although dopamine does not cross the BBB to the periphery, the effects of increased extracellular dopamine on HIV neuropathogenesis are important to characterize because monocytes express surface receptors that bind dopamine as they cross the BBB (59, 60, 98). In vitro studies showed that dopamine and D1 receptor agonists increase monocyte adhesion, as well as transmigration of uninfected human CD14+CD16+ monocytes across a human BBB model (59, 60). These results suggest that monocyte influx is increased in regions of the brain that have increased dopamine concentrations (59, 60). This may be facilitated by increased active ADAM17, a metalloproteinase that cleaves the complex of Mac-1 on monocytes bound to endothelial ICAM-1 as they begin to diapedese (60). Primary human monocyte derived macrophages (MDM) also express dopamine receptors. Dopamine increases viral entry into these cells, resulting in increased viral replication (75, 76). In response to dopamine, human MDM also increase basal and LPS-mediated cytokine secretion (77). These data indicate that dopamine released in response to opioids or other drugs of abuse may increase monocyte migration into the CNS, and increase HIV infection and cytokine secretion by macrophages, contributing to HIV neuropathogenesis.
HIV, Opioids, and the Roles of Microglia and Macrophages
Macrophages and microglia are phagocytic cells responsible for, among other functions, clearance of extracellular materials and production of cytokines and chemokines (99–102). In general, microglia are derived from the yolk sac and enter the CNS during embryonic development, while monocytes arise from hematopoietic progenitors, enter the brain throughout life, and can differentiate into long-lived macrophages (99, 100). There may be certain subsets of microglia that derive from the bone marrow as well (103, 104). Recent findings indicate that, within the CNS, macrophages and microglia have unique transcriptional profiles, suggesting that they may contribute to HIV neuropathogenesis by both similar and distinct mechanisms (105, 106). Some differentially expressed genes suggest that microglia modulate CNS neurotransmitter levels, while brain-resident macrophages promote toll-like receptor signaling and regulate growth factor concentrations and signaling (105).
Findings indicate that HIV reservoirs in microglia and macrophages still persist despite suppressive ART. Studies in animal models and PLWH taking ART have shown that HIV DNA and RNA colocalize with both macrophages and microglia in post-mortem brain tissue (107–109). Data from SIV-infected macaques on ART also demonstrated that brain macrophages in the CNS can still produce active virus, as shown using quantitative viral outgrowth assays (QVOA) (108). HIV-infected microglia and macrophages release neurotoxic viral and host factors, including HIV proteins, gp120, Tat, and Vpr, and reactive nitrogen/oxygen species (ROS/RNS), which damage neurons and activate other CNS cells, including astrocytes and endothelial cells (101, 110–113). Chronic disruption of CNS homeostasis leads to neurocognitive decline characteristic of HAND. Both macrophages and microglia are also activated by the microbial product, LPS, which is increased in the serum of PLWH even with ART (100, 110, 114). In response to LPS, microglia and macrophages increase production of TNFα, IL-1β, CCL2, and IL-8 that can damage neurons directly and increase transmigration of monocytes across the BBB (110, 112, 115). The mechanisms by which opioids contribute to these processes in microglia and macrophages in the context of ART are especially important to characterize such that they can be targeted therapeutically to reduce viral reservoirs and HAND.
Opioids may contribute to HIV-mediated CNS damage by regulating immune cell functions through opioid receptors. There are three classical opioid receptor subtypes, the μ (MOR), κ (KOR), and δ-opioid (DOR) receptors, all of which are expressed on the surfaces of microglia and macrophages (110, 116–118). There is a fourth, nociceptin receptor (NOP), that will not be discussed further in this review (119).
Results from many in vitro experiments demonstrated that morphine increases HIV replication in infected macrophages and microglia, although these results vary based on duration of opioid exposure (63–66). Increased viral replication may be due to a morphine mediated increase in galectin-1, as well as inhibition of anti-viral interferon signaling and microRNAs that target HIV genes for degradation (64–69, 110). Importantly, increased CCR5 expression in response to opioids also increases HIV replication in macrophages (63). This indicates that opioid mediated increased HIV replication is dependent on an HIV coreceptor that can be targeted therapeutically. Maraviroc is a known blocker of CCR5 that improves neurocognitive functioning in people with HAND (120, 121). It was also shown to decrease the number of CD16-expressing monocytes in PLWH, suggesting that maraviroc reduces the pool of infected monocytes in the periphery that can enter the CNS (121). Maraviroc may also reduce opioid mediated neuropathogenesis in PLWH by attenuating viral reservoirs in macrophages and microglia.
Although most studies found that morphine increases HIV replication, one study in MDM demonstrated that morphine may contribute to neuropathogenesis by increasing cytokines without affecting HIV replication (70). Other studies refuted this finding and showed no significant difference in cytokine production with opioids compared to HIV infection alone (71, 117). The mechanisms by which changes in viral replication may occur are important to characterize to reduce CNS viral load. Some studies suggest that this process is dependent on both MOR activation and on the subtype of MOR expressed by the cell (64, 110). While acute exposure to opioids has been shown to decrease intracellular cAMP through inhibitory G proteins, more chronic opioid exposure may increase cAMP (72, 102, 118). This increase leads to activation of proteins such as cAMP response element binding protein (CREB), which may bind to the 5′ LTR of the HIV genome to increase viral replication (66). Microglia and macrophages play critical roles as HIV harboring cells in the CNS. Thus, more studies are needed to address how opioid mediated changes in HIV replication occur in both cell types.
Chronic generation of reactive oxygen and nitrogen species has been implicated in the pathogenesis of HAND (110, 112). Productive HIV infection and/or exposure to HIV proteins, including Tat, increase ROS production in macrophages and microglia (69, 73, 110, 122). Additionally, multiple ART drugs, especially protease inhibitors (PI), including lopinavir and nelfinavir, and nucleoside reverse transcriptase inhibitors (NRTI), including zidovudine and stavudine, increase ROS in macrophages derived from THP-1 cells (78). The contribution of opioids to oxidative stress in the context of chronic HIV infection and/or exposure to neurotoxic HIV proteins, is complex (123). Studies in J774 murine macrophages showed that acute exposure to morphine decreases nitric oxide production, while morphine treatment of human MDM increases ROS through induction of certain miRNAs (65, 74). Findings in peritoneal macrophages demonstrated an increase in ROS when mice were given morphine twice daily for 5 days (117). Additional experiments showed that acute exposure to morphine and Tat synergistically increases ROS in murine microglia, an effect dependent on MOR (73). These results highlight that opioids may contribute to disease processes in the CNS by increasing oxidative stress in microglia and macrophages that harbor HIV or are exposed to HIV proteins. Given that PLWH and people taking pre-exposure prophylaxis (PrEP) are prescribed daily ART, it is important to examine how opioids affect oxidative stress in this context (124). This is especially relevant for macrophages, which differentiate from monocytes that have been exposed to higher levels of ART in the blood prior to transmigration into the CNS (100, 125).
Opioids may impact cytokine secretion in CNS macrophages and microglia, perpetuating neuroinflammation that contributes to neurocognitive decline. One study in murine microglia demonstrated that 24 h of morphine treatment decreases Tat mediated upregulation of IL-6, MCP-1, and TNF-α secretion, while another using primary human microglia showed that exposure of primary human microglia to morphine for 24 h increases IL-8 secretion but does not significantly change IL-6, MCP-1, or TNF-α secretion (69, 73). Few studies have addressed the effects of morphine on cytokine secretion in macrophages infected with HIV or exposed to HIV proteins (68, 70, 71). Future studies should determine the interactive effects of ART, opioids, and exposure to HIV on neuroinflammation propagated by macrophages and microglia. These may characterize molecular mechanisms by which microglia and macrophages contribute to neurodegeneration in both opioid abusers who are HIV-positive and those taking PrEP with the goal of developing therapeutics that reduce CNS inflammation and subsequent neuronal damage.
HIV, Opioids, and Astrocytes
Astrocytes are the most abundant cells in the brain, and astrocyte homeostasis is essential for maintaining CNS function (126–129). Various studies demonstrated that astrocytes differ in morphology, suggesting varied functional capability (128, 130). Astrocytes regulate synaptic transmission through a well-characterized glutamate uptake mechanism, provide essential roles in synaptogenesis, regulate CNS ion homeostasis, produce ROS, and modulate CNS cells by responding to and producing chemokines and cytokines (126, 129, 131–133). Astrocytes also contact the endothelium to form part of the blood-brain-barrier (BBB), highlighting their importance in regulating monocyte entry into the CNS (134).
Astrocytes are less susceptible to productive HIV infection and may not effectively produce mature, infectious virions in vivo (107, 110). Early studies in post-mortem brain tissue demonstrated that HIV DNA and RNA colocalize with astrocytes, although more recent studies using tissue from PLWH who took ART did not find such colocalization (107, 135–137). Regardless of their infection status and ability to produce active virus, astrocytes produce and respond to neurotoxic viral proteins, gp120, Tat, and Nef (135, 137, 138). Exposure to these proteins leads to increased glutamate, nitric oxide, and cytokines that contribute directly to neuronal damage (79, 135, 137, 139). HIV infection of astrocytes increases Cx43, a protein that forms part of gap junctions and hemichannels, increasing delivery of neurotoxic factors and chemokines both directly to other astrocytes and to the extracellular space (140, 141). Upregulation of Cx43 colocalized with apoptosis of BBB cells in tissue from PLWH and SIV-infected macaques, which could contribute to a leaky BBB and enhance monocyte entry into the CNS (142).
Microarray and gene ontology studies showed that treatment of astrocytes with Nef induces expression of specific long non-coding RNAs (lncRNAs) that increase secretion of CXCL9, CXCL10, and CXCL11 (143). CXCL10 had already been shown to cause neuronal damage in response to Nef. These microarray and gene ontology data suggest that CXCL9 and CXCL11 secreted by astrocytes may also be important in HIV neuropathogenesis (143). Astrocytes treated with Tat and co-cultured with microglia increased release of intact exosomes containing miR-9, a miRNA that was taken up by microglia and stimulated their migration in vitro (144). Recent findings also demonstrated that astrocytes exposed to HIV, but not productively infected, have increased mitochondrial damage and ROS, activating NLRP3 inflammasomes and increasing IL-1β secretion (145). Conversely, astrocytes harboring HIV cleared damaged mitochondria, leading to decreased ROS and IL-1β. These data emphasize that even uninfected astrocytes can contribute to HIV neuropathogenesis through increased ROS and cytokine expression.
Astrocytes may play a significant role in opioid mediated neuropathogenesis in the context of HIV given their abundance and expression all three opioid receptor subtypes (MOR, KOR, DOR) (146–148). Opioids can exacerbate CNS damage through disruption of normal astrocyte functions (80, 81, 147, 148). Astrocytes are critical to regulating local synaptic glutamate concentrations, and increased synaptic glutamate leads to neuronal excitotoxicity (126, 135, 137). Opioids decrease astrocytic expression of glutamate transporters, GLT-1 and GLAST, which are essential for glutamate uptake (81). Recent studies demonstrated that opioids also increase glutamate release from astrocytes through specialized potassium channels, a process dependent on Gαi-coupled GPCR activity (80). Morphine has also been shown to decrease glutamate uptake in astrocytes infected with HIV or exposed to HIV proteins (79). These findings suggest that opioids may increase CNS damage by furthering disruption of astrocytic functions already impaired in the context of HIV (148).
The interactive effects between HIV and opioids on cytokine production in astrocytes are complex (79, 80, 84). While morphine by itself has minimal effects on cytokine secretion, astrocytes treated with morphine and Tat secreted more IL-6, CCL2, and TNF-α compared to cells treated with Tat alone. HIV-infected astrocytes treated with morphine produced more CCL2, IL-8, and TNF-α compared to astrocytes infected with HIV but not treated with morphine (79, 80). This increase in cytokines may enhance local inflammation that damages neurons, recruits additional infected cells from other regions of the CNS, and promotes further monocyte transmigration across the BBB. Transcription of these cytokines is regulated by NFκB, which is activated downstream of MOR (139, 149, 150). Productively infected primary human astrocytes also express TLR4 and produce cytokines in response to pathogen associated molecular patterns (PAMPs) like LPS (138, 139, 151). Given that morphine itself may activate TLR4, agonism at this receptor could act synergistically with MOR to increase NFκB mediated inflammatory cytokines (152).
Crosstalk between chemokine and opioid receptors in astrocytes may also contribute to opioid mediated HIV neuropathogenesis (132, 133). In neuron-astrocyte co-cultures, expression of CCR5 by astrocytes was shown to mediate opioid driven exacerbation of Tat induced neuronal damage (153). When CCR5 was deleted from glial cells or blocked pharmacologically with maraviroc, morphine treatment resulted in a surprising neuroprotective effect in response to Tat (153). These results emphasize that the impact of opioids on neuronal damage during HIV infection may change based upon chemokine receptor signaling. These data also suggest that maraviroc may improve neurocognitive outcomes in PLWH with OUD through its effects on astrocytes in addition to monocytes and macrophages (120, 121).
Potential mechanisms that mediate astrocyte dysfunction in the context of ART remain important to characterize. Some studies examined the effects of ART on astrocyte function, although few have addressed effects on HIV neuropathogenesis during exposure to opioids and ART together (83–85). One study using a combination of ritonavir, abacavir, and lamivudine demonstrated that treatment of astrocytes for 7 days leads to a cellular senescent phenotype characterized by increased cell cycle inhibitor, p21, increased oxidative stress, and increased IL-6 (83). Another study demonstrated that the PIs, amprenavir and lopinavir, decrease glutamate transporter, EAAT2, exacerbating defects in glutamate uptake (85). Others examined the effects of opioids and ART together on viral replication and cytokine expression in astrocytes infected in vitro (84). While a combination of the NRTI, emtricitabine, and two PIs, ritonavir and atazanavir, effectively reduced p24 production over 7 days, this effect was attenuated when astrocytes were co-incubated with morphine (84). This ART combination also inhibited CCL5 and CCL2 production in HIV-infected cells. However, when HIV-infected cells were treated with ART and morphine, they produced more CCL5 and CCL2 than infected cells that did not receive ART or morphine (84). These findings indicate that ART drugs may disrupt astrocyte homeostasis, underscoring the need to study the effects of ART in the presence and absence of opioids on the contribution of astrocytes to neuropathogenesis in PLWH.
Opioids, HIV, and the Potential Role of T Cells
T cells play a significant role in many inflammatory processes in the CNS (48, 154). While in vitro evidence for a role of T cells in HIV neuropathogenesis is limited, some clinical studies suggest that T cells contribute to HIV neuropathology (111, 155–157). Two studies of brains from HIV-positive individuals demonstrated the presence of CD8+ T cells (155, 157). However, these studies were done in the context of HIV-encephalitis (HIVE) and HIV-associated dementia (HAD), which are not as prevalent as milder forms of HAND in the ART era (155, 157). There is also a significant correlation between nadir CD4+ T cell level and neurocognitive impairment in PLWH, but this association could indicate more generally severe systemic disease, and does not substantiate direct T cell contribution to neuropathogenesis (156). A population of CD8+ T cells is increased in the CSF of PLWH with cognitive impairment (158, 159). One study demonstrated that CD8+ T cells present in the CSF of PLWH have increased VLA-4 and CXCR3, while another found that levels of IFN-γ in these CSF CD8+ T cells correlated with severity of neurocognitive impairment (158, 159). These results suggest that the inflammatory signature of T cells in the context of HIV neuropathogenesis may be distinct and could perhaps be targeted therapeutically (158). Release of factors such as IFN-γ from T cells may be indicative of a compensatory anti-viral response that inadvertently promotes activation and polarization of M1 macrophages to further neuroinflammation. However, the contribution of T cell transmigration across the BBB to development of HAND is less clear (110).
In most cases, opioids have immunosuppressive effects in T cells (89, 160). In fact, heroin-addicted individuals have increased regulatory T cells (Tregs) in peripheral blood compared to healthy controls or to opioid abusers currently undergoing opioid substitution therapy (161). While little is known about the contribution of opioids to the potential roles of T cells in HIV neuropathogenesis, some studies have examined the effects of opioids on T cell functions (89, 160–162). Opioids can impact T cell functions by binding surface MOR, KOR, and DOR (86, 87, 160). Opioid receptor activity has been shown to inhibit T cell proliferation and activation through suppression of IL-2 secretion and T cell receptor signaling, and exacerbate cell death induced by gp120 (89, 163). All of these may contribute to systemic immunosuppression characteristic of chronic opioid abuse and increase susceptibility to infection (72). Both KOR and DOR activity have been shown to inhibit production of HIV in CD4+ T cells, and opioid receptor activity does not seem to play a role in reactivation of HIV in latently infected cells (86, 87, 163). However, naltrexone treatment of CD4+ T cells, which blocks opioid receptors, may potentiate the antiviral effects of ART drugs, such as AZT and indinavir. Thus, it is necessary to study the effects of opioids on T cell functions in the context of ART (88). For CD8+ T cells, which may be increased in the CNS, morphine has been shown to inhibit IFN-γ dependent anti-HIV activity (164). These results indicate that T cells in the HIV-infected CNS exposed to opioids may be limited in their capacity to control CNS viral replication, exacerbating CNS damage (88, 161, 162, 164, 165). Further studies are needed to determine how opioids, opioid substitution therapy, and ART regulate T cell activation and suppression to impact CNS damage in PLWH with OUD.
Opioid Substitution Therapy
One way to treat opioid addiction is through opioid substitution therapy (OST), including methadone and buprenorphine (166). Currently, little is known about the molecular impacts of these therapies and specifically their contribution to HIV neuropathogenesis in the ART era.
Methadone and buprenorphine are two of the most commonly used OSTs (167). Methadone is a synthetic opioid and full MOR agonist with long lasting effects (168). Its pharmacological actions are similar to morphine (168). Buprenorphine is a semi-synthetic opioid, long lasting partial agonist of MOR and a full antagonist of KOR (169). While both methadone and buprenorphine are effective at treating opioid dependence, some studies suggest these treatments may differentially impact cognitive outcomes in people with OUD. Buprenorphine treated drug abusers had improved cognitive outcomes compared to baseline or those treated with methadone (170–172). However, other studies found no difference in cognitive performance between methadone and buprenorphine treated opioid users (173). Overall, these studies were done with small sample sizes. Studies with larger numbers of subjects are needed to address definitively whether one therapy has improved outcomes compared to the other. A few studies also compared cognitive outcomes of drug users taking buprenorphine or methadone to healthy controls (171, 174, 175). In these studies, both buprenorphine treated and methadone treated individuals performed worse in multiple cognitive domains than their healthy counterparts (171, 174, 175). The healthy controls were individuals who did not have OUD or a history of chronic drug use. It is important note that individuals who are taking methadone or buprenorphine for OUD may already have cognitive deficits due to chronic drug use (12, 21, 25). A healthy individual who does not chronically abuse drugs may not manifest the same deficits as an individual who does. While comparing cognitive outcomes among these groups will address their effects on normal cognition, it may mask positive effects of buprenorphine and methadone on cognitive outcomes in people with OUD.
Some evidence suggests that PLWH are at higher risk for OUD than their uninfected counterparts (10). Thus, it is important to understand the effects of OST in the context of HIV neuropathogenesis and ART. Few studies have directly addressed the impact of methadone or buprenorphine on HIV-mediated CNS damage. Both methadone and buprenorphine reduce illicit opioid use and risky behaviors associated with HIV infection (176). In some cases, individuals taking methadone or buprenorphine were more likely to initiate or remain on ART and have improved CD4+ T cell counts (20, 22, 176). This demonstrates that OST can successfully treat opioid dependence and improves both the health and quality of life of PLWH who have OUD (22, 171, 177–181). However, in one study, HIV infected men taking methadone performed worse on cognitive tests than those who were not taking methadone (182). In contrast, some evidence suggests that PLWH taking buprenorphine have improved quality of life and neuropsychological functioning (12, 179, 183). Thus, buprenorphine appears to be a promising therapy in the context of HIV mediated CNS damage. More studies are needed to evaluate the effects of both methadone and buprenorphine on cognitive outcomes in PLWH, especially in the context of the current guidelines to initiate ART at the time of diagnosis.
Data from our laboratory propose that buprenorphine may decrease HIV neuropathogenesis through its effects on monocytes, and underscore the potential therapeutic effects of buprenorphine as a treatment for HAND (61, 62). Buprenorphine decreased important steps in the transmigration of mature monocytes across the BBB, including CCL2-mediated adhesion to ICAM-1 and chemotaxis (Table 1) (61, 62). These results indicate that buprenorphine may reduce HIV neuropathogenesis and cognitive impairment by decreasing monocyte entry into the brain (Figure 1A, Table 1).
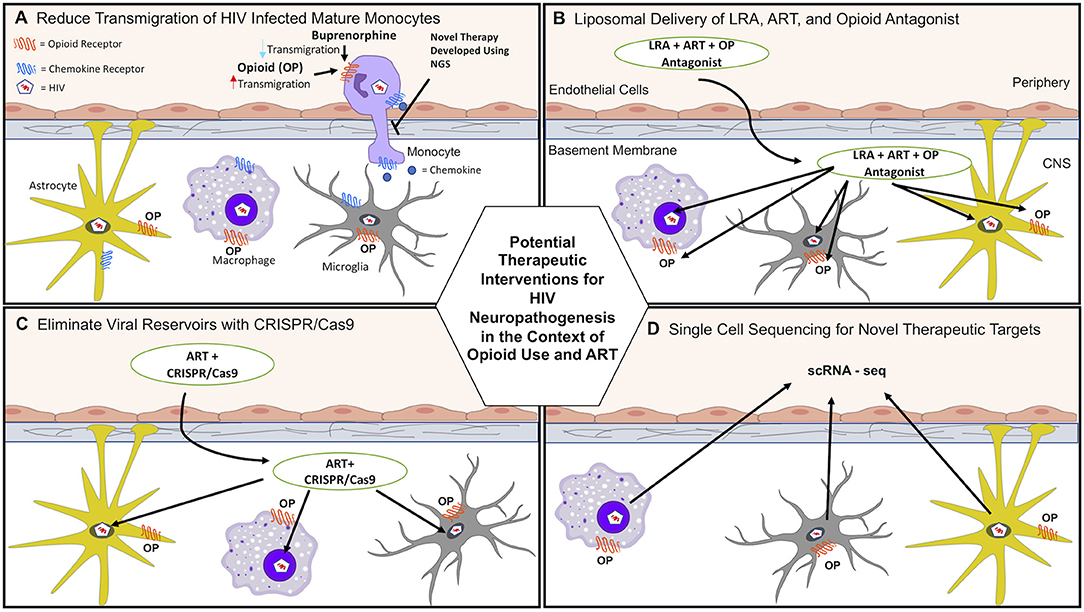
Figure 1. Potential therapeutic interventions for HAND in the context of opioid use and antiretroviral therapy (ART). (A) HIV induced neuroinflammation and viral seeding can be reduced by decreasing monocyte transmigration into the brain that is increased with opioid abuse. Buprenorphine and/or novel therapies developed using next generation sequencing (NGS) may reduce transmigration of HIV-infected and uninfected monocytes. (B) Current ART penetration into the brain does not eradicate viral reservoirs. Latency reactivating agents (LRA), ART, and opioid antagonists can be transported across the BBB and target infected cells in the CNS using liposomal nanoparticle delivery systems. (C) ART and CRISPR/Cas9 can be transported across the BBB to help eradicate HIV reservoirs using liposomal nanoparticle delivery systems. (D) scRNA-seq can be used to identify novel molecular mechanisms by which opioids and HIV infection increase CNS damage. These mechanisms can then be targeted to reduce HAND.
The mechanisms by which buprenorphine mediates these effects are unclear. One reason may be due to its unique pharmacological properties (169). Buprenorphine is a partial MOR agonist with high affinity for the receptor but low intrinsic activity (166). This is distinct from other opioids such as morphine, codeine, fentanyl, and methadone that are full MOR agonists (169, 184). Full agonists and partial agonists may have different binding properties that facilitate unique signaling responses. This process is called biased agonism, and occurs when different ligands bind the same receptor but activate different intracellular signaling pathways.
Both opioid receptors and chemokine receptors, including CCR2 and HIV co-receptors, belong to a family of G-protein coupled receptors (GPCRs) that signal through the inhibitory Gα protein, Gαi. GPCRs are downregulated by desensitization, one form of which is heterologous desensitization (185, 186). During heterologous desensitization, activation of one GPCR inactivates signaling from a different GPCR (185, 187, 188). This may be one mechanism by which buprenorphine impacts monocyte function. Buprenorphine may inhibit downstream inflammatory signaling of chemokine receptors like CCR2 through its actions at MOR and KOR (62). We showed that buprenorphine decreases the association of FROUNT with CCR2 (62). This association mediates CCR2 signaling and enhances CCR2 induced monocyte transmigration (189). Thus, buprenorphine may decrease monocyte transmigration into the brain by limiting the interaction of FROUNT with CCR2.
Buprenorphine may also regulate GPCR signaling through heterodimers consisting of one opioid receptor subunit and one chemokine receptor subunit, resulting in desensitization upon simultaneous binding of opioids and chemokines to their receptors (5). It has been shown that DOR can heterodimerize with CXCR4 (190). Concomitant treatment with buprenorphine and a chemokine may result in heterodimers of opioid and chemokine receptors, thus affecting the signaling of each receptor subunit (191, 192). This may also affect the transmigration of mature monocytes across the BBB to contribute to HAND. More studies are needed to characterize the effects of buprenorphine on transmigration of monocytes and other immune cells in vitro and in vivo, as well as to address the impacts of methadone and buprenorphine on cognitive functions in PLWH, especially in the context of OUD and ART.
New Technologies and Potential Therapies
New developments in molecular, genetic, computational, and next generation sequencing (NGS) techniques provide exceptional opportunities to characterize mechanisms by which opioids and HIV infection in the ART era impact neuroinflammation and neuronal damage, and may ultimately lead to development of therapies. Figure 1 illustrates novel approaches for identifying mechanisms as well as potential therapeutic interventions to reduce the burden of HIV neuropathogenesis in the context of OUD. Table 2 summarizes new technologies and therapeutic strategies to target HIV mediated CNS damage in the context of opioid use.
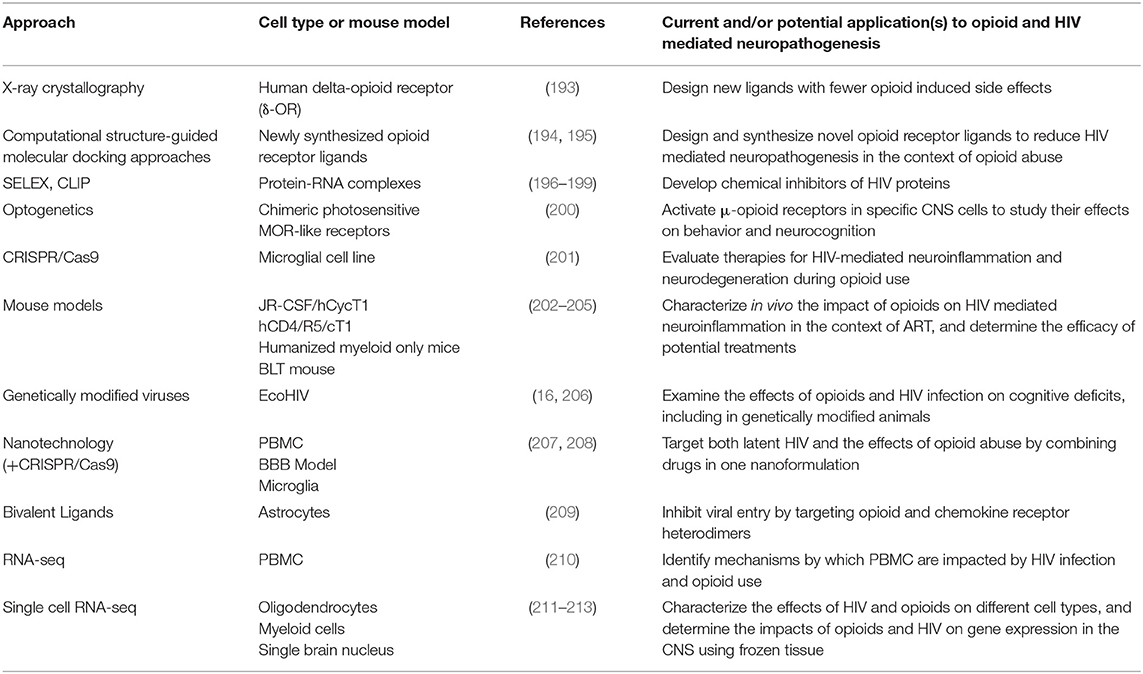
Table 2. Novel approaches for targeting processes that contribute to HIV neuropathogenesis in the context of opioid use.
Novel Technologies to Characterize Opioid Receptor Function
Structural and functional characterization of opioid receptors is especially important for understanding signal transduction after ligand binding. Advances in high resolution x-ray crystallographic techniques have led to crystal structures for all four known opioid receptors, including structures of receptors with associated peptides (193). These advancements are pivotal to develop new opioid receptor ligands with increased therapeutic actions and fewer side effects (Table 2). For example, structure-guided molecular docking approaches enable screening of millions of candidate compounds by computational studies, leading to the synthesis and optimization of selected molecules with novel biological effects on opioid receptors (194, 195). These studies could lead to development of compounds that reduce neuropathogenesis caused by morphine induced cytokine secretion, increased viral replication, and other important functions of microglia, macrophages, and astrocytes.
Multiple isoforms of opioid receptor subtypes can be generated by alternative splicing, and HIV infection has been suggested to play a role in promoting formation of these isoforms. One study found that an increase in the MOR-1K isoform correlates with severity of neurocognitive impairment in PLWH (214). The mechanisms by which HIV mediates these alterations in splicing remain uncharacterized, but modifications in several specific RNA-binding proteins may be involved (215). Selected evolution of ligands through exponential enrichment (SELEX) and cross-linking and immunoprecipitation (CLIP) have been used to characterize the role of these proteins (196, 197). Understanding these mechanisms led to screening of chemical inhibitors developed to inhibit key HIV regulatory proteins (198, 199) (Table 2). Therefore, a more extensive characterization of how HIV can impact the pharmacology of individual opioid isoforms is essential to understand how opioids contribute to HAND in the context of ART.
Another technology is optogenetics. Optogenetics is an experimental approach that modulates neural activity by activating and inhibiting light-sensitive proteins (216). Advances in optogenetics led to generation of chimeric photosensitive MOR-like receptors. This enables researchers to study the spatiotemporal control of opioid receptor signaling in vitro and in vivo. It was shown that in vitro photostimulation of cultured murine dorsal root ganglia neurons resulted in similar signaling and receptor internalization to that of the unmodified μ-opioid receptor. Using this approach in vivo, this group also found that MOR-like receptor signaling can be modified with photostimulation, resulting in behavioral responses similar to those observed when stimulating the unmodified receptor (200). This technology could be used in HIV-infected animal models to activate MOR in specific CNS cells types and study effects on behavior and cognitive status (Table 2).
Genome Editing Technologies
Novel genome editing technologies have led to new models to study opioid mediated HIV neuropathogenesis. One study used the CRISPR/Cas9 system to integrate a modified HIV-provirus expressing a nanoluciferase reporter into the genome of a microglial cell line. This enables rapid assaying of HIV proviral activity induced by pharmacological manipulation, and would be useful to evaluate therapies for HIV-associated neuroinflammation and neurodegeneration in the context of opioid use (Table 2) (201). Mouse models have also been developed to study HIV neuropathogenesis. The JR-CSF/hCycT1 is a transgenic mouse that produces HIV, leading to plasma viremia. This model is particularly useful to study the pathogenesis of HIV-mediated neuroinflammation because these mice express a full-length HIV provirus virus that replicates in CD4+ T cells and monocytes (202). Another transgenic mouse model, the hCD4/R5/cT1, has been modified to enable acute in vivo HIV infection after intravenous, intravaginal, and intrasplenic inoculation, resulting in local infection and plasma viremia (203). Thus, these models provide opportunities to study the impact of opioids on HIV replication and inflammation in the context of ART in vivo, and to determine the efficacy of potential therapies. Additional new approaches include genetically modified viruses, such as EcoHIV, which is used to infect mice as a model for studying chronic HIV CNS disease (16, 206). This model has a low level of infection and develops neurocognitive impairment. It can also be used to study the effects of opioids and HIV infection on cognitive deficits in genetically manipulated mice (206). Other model murine systems include humanized myeloid-only and BLT mice (204, 205) (Table 2).
Novel Therapeutic Approaches
Current ART does not eradicate HIV CNS reservoirs or improve the complications of HIV infection in the context of opioid abuse. One strategy involves using encapsulated liposomal magnetic nanoparticles containing a combination of ART drugs to prevent HIV replication, latency reactivating agents to activate CNS viral reservoirs, and drug abuse antagonists to ameliorate associated side effects. These novel nanoparticles have several advantages. Different drugs can be combined in a single nanoformulation to target simultaneously latent HIV and opioid induced CNS damage (Table 2). Additionally, they can be targeted to a specific tissue by non-invasive magnetic forces applied at adjustable intensities. They can also cross the BBB, avoiding a major restriction of the transport of many ART drugs (Figure 1B) (207).
Although nanotechnology is a promising approach to treat opioid associated neuropathogenesis in PLWH, there are few in vivo studies showing site specific targeting for antiretrovirals and drug abuse antagonists. Thus, BBB transmigration efficiency, appropriate concentrations of drug to be added, and magnetic treatment parameters have to be optimized for human application.
Another nanotechnology-based approach includes gene editing tools to eradicate HIV brain reservoirs in drug abusers. CRISPR/Cas9 technology is being used to eliminate latent HIV from the genome by specifically targeting the enhancer of the HIV LTR promoter (217). However, this technique still requires an effective method for delivery across the BBB. To overcome this obstacle, it has been combined with magnetic nanodelivery systems, showing a significant HIV-LTR reduction, indicating a potential therapy to eliminate latent HIV infection in the brains of opioid abusers (Figure 1C) (208).
Structural and functional characterization of MOR-CCR5 heterodimers may help to study synergistic effects of HIV infection and opioid abuse in the CNS, as discussed earlier in this review. One study used novel synthesized bivalent ligands, combining a MOR antagonist and a CCR5 antagonist into one molecule, to target these heterodimers in HIV-infected astrocytes, inhibiting HIV entry two-fold compared to a mixture of individual MOR and CCR5 antagonists (Table 2) (209).
Next Generation Sequencing Technologies
RNA sequencing (RNA-seq) studies have been used to analyze transcriptional profiles of different brain regions in the context of long-term opioid abuse leading to neurodegeneration. Chronic oxycodone self-administration in mice was shown to increase expression of genes related to inflammation and/or immune function, including CCR5, in the dorsal and ventral striatum (218). Increased CCR5 expression facilitates migration of cells to sites of inflammation in response to chemokines, increasing their susceptibility to infection (7, 63, 64, 219, 220). RNA-seq studies have also been performed with cells from human cohorts. One study in PBMC from long-term heroin users showed upregulation of genes related to neurological and psychiatric disorders, including spinocerebellar ataxia type 2 and posttraumatic stress disorder (210) (Table 2). These cells can enter the CNS, underscoring the importance of using new tools to understand mechanisms by which they contribute to neuropathogenesis related to opioid abuse.
The brain is a heterogeneous organ, and therefore RNA-seq analysis may not fully identify and assign unique gene expression profiles to specific cells in each brain region. To overcome this limitation, single cell transcriptome analytic approaches can be used. Single cell RNA sequencing (scRNA-seq) is a powerful tool that can be used to address many questions relevant to HIV infection and OUD (Figure 1D). It is of particular interest to determine how different cell types in brain regions are affected by chronic opioid exposure. A recent study demonstrated that acute morphine treatment of mice results in unique transcriptional responses by oligodendrocytes, suggesting a link between opioid abuse and myelin dysregulation (211).
ScRNA-seq analysis can characterize how HIV impacts individual CNS cell types by modifying their gene expression. One study performed this analysis on cells obtained from CSF and blood from adults with and without HIV infection, and identified a rare subset of myeloid cells present only in CSF (Table 2). The gene expression profile of these cells showed significant similarities to the profiles of neurodegenerative disease–associated microglia (212). scRNA-seq can also be used to identify the molecular characteristics of CNS and peripheral blood cell types that harbor HIV during opioid abuse. Our group found that CD14+CD16+ monocytes that specifically harbor HIV preferentially transmigrate across an in vitro human BBB model in response to chemokines compared to uninfected monocytes exposed but not harboring the virus (46, 221). Thus, the identification of gene expression profiles of individual HIV-infected cells may provide new strategies to eliminate HIV in the CNS of opioid abusers. However, although scRNA-seq is widely used to study individual cell transcriptomes, isolation of single cells from complex tissues such as the brain is difficult. Newly improved single cell sequencing techniques, such as single-nucleus RNA sequencing, enables analysis of gene expression within a single brain nucleus, reducing cell biases caused during cell isolation and facilitating the use of frozen samples (213).
Next generation sequencing technology, and especially single cell genomics, will be important tools for the molecular characterization of opioid abuse in the context of HIV infection and ART. Studies using these technologies may lead to identification of novel therapeutic targets and diagnostic biomarkers, enabling early intervention to improve neurocognitive outcomes in PLWH with OUD.
Conclusion
HIV remains a significant worldwide health issue as does the relationship between HIV infection and the opioid epidemic. HAND persists despite successful ART, for which there are currently no treatments. Ultimately, the combined effects of opioid use and HIV infection have negative consequences on neurocognitive outcomes. Thus, it is important to understand the mechanisms by which opioids contribute to HIV mediated CNS damage, especially in the context of suppressive ART. Opioids may contribute to HIV neuropathogenesis through regulation of immune cell functions. The specific mechanisms by which opioids and HIV infection interact remain unclear. Buprenorphine appears to have beneficial effects on cognitive outcomes in PLWH. More studies are needed to examine how opioids and OST contribute to or limit HIV neuropathogenesis. Now that PLWH are prescribed ART at the time of diagnosis. Current and new technologies will characterize how opioids impact HIV infection of the brain in the ART era, and will direct the development of novel therapies for HAND in PLWH with OUD.
Author Contributions
AM, JB, PM-A, VC, and MJ-B contributed to discussions about manuscript content, writing, and editing of the manuscript. JWB contributed to discussions of the manuscript and its editing.
Funding
This work was funded by R01DA048609 (JWB), R01DA04458 (VC, JWB), R01MH11239 (PM-A, JWB), R01DA041931 (MJ-B, JWB, AM, JB), T32GM00728846 (JB), 1TL1TR002557 (AM).
Conflict of Interest
The authors declare that the research was conducted in the absence of any commercial or financial relationships that could be construed as a potential conflict of interest.
Acknowledgments
We would like to thank Rosiris León Rivera for her help in editing the manuscript and Dr. Laura Cheney for her input on Figure 1. Image credited to BioRender.
Abbreviations
HAND, HIV associated neurocognitive disorder; PLWH, People living with HIV; OUD, Opioid use disorder; OST, Opioid substitution therapy; ICAM-1, Intracellular Adhesion Molecule 1; VCAM-1, Vascular Adhesion Molecule 1; LFA-1, Lymphocyte lymphocyte function-associated antigen 1 (LFA-1); VLA-4, Very late antigen 4 (VLA-4); JAM-A, Junctional adhesion molecule A; SIV, Simian immunodeficiency virus; CNS, Central nervous system; BMVEC, Brain microvascular endothelial cells; JAM-2, Junctional adhesion molecule 2; ALCAM, Activated Leukocyte Cell Adhesion molecule; ANI, Asymptomatic neurocognitive impairment; MND, Mild neurocognitive disorder; HAD, HIV associated dementia; ART, Antiretroviral Therapy; BBB, Blood brain barrier; ADAM17, A disintegrin and metalloproteinase 17; MDM, Monocyte derived macrophages; MOR, μ-opioid receptor; KOR, κ-opioid receptor; DOR, δ-opioid receptor; NOP, Nociceptin opioid receptor; ROS, Reactive oxygen species; RNS, Reactive nitrogen species; PI, Protease inhibitor; NRTI, Nucleoside reverse transcriptase inhibitor; lncRNAs, long non-coding RNA; GPCR, G protein coupled receptors; HIVE, HIV encephalitis; CSF, Cerebrospinal fluid; NGS, Next generation sequencing; SELEX, selected evolution of ligands through exponential enrichment; CLIP, cross-linking and immunoprecipitation; RNA-seq, RNA sequencing; PBMC, Peripheral blood mononuclear cells; scRNA-seq, single cell RNA sequencing; ZO-1, Zonula occludens 1; QVOA, Quantitative viral outgrowth assay; LPS, Lipopolysaccharide; CREB, Cyclic AMP response element binding protein; LTR, Long terminal chain repeat; NLRP3, NLR family pyrin domain containing 3; GLT-1, Glutamate transporter 1; GLAST, Glutamate aspartate transporter 1; TLR, Toll-like receptor; PAMP, Pathogen associated molecular patterns; AZT, zidovudine; LRA, Latency Reactivating Agents; OP, Opioid; PrEP, Pre-exposure prophylaxis.
References
1. Antiretroviral Therapy Cohort Collaboration. Survival of HIV-positive patients starting antiretroviral therapy between 1996 and 2013: a collaborative analysis of cohort studies. Lancet HIV. (2017) 4:e349–56. doi: 10.1016/S2352-3018(17)30066-8
3. Peters PJ, Pontones P, Hoover KW, Patel MR, Galang RR, Shields J, et al. HIV infection linked to injection use of oxymorphone in Indiana, 2014-2015. N Engl J Med. (2016) 375:229–39. doi: 10.1056/NEJMoa1515195
4. Williams AR, Bisaga A. From AIDS to opioids - how to combat an epidemic. N Engl J Med. (2016). 375:813–5. doi: 10.1056/NEJMp1604223
5. Allen SJ, Crown SE, Handel TM. Chemokine: receptor structure, interactions, and antagonism. Annu Rev Immunol. (2007) 25:787–820. doi: 10.1146/annurev.immunol.24.021605.090529
6. Bose J, Hedden SL, Lipari RN, Lee EP. Key Substance Use and Mental Health Indicators in the United States: Results from the 2017 National Survey on Drug Use and Health. (2017). Substance Abuse and Mental Health Services Administration 2018. Available online at: https://www.samhsa.gov/data/sites/default/files/cbhsq-reports/NSDUHFFR2017/NSDUHFFR2017.htm
7. Dutta R, Roy S. Mechanism(s) involved in opioid drug abuse modulation of HAND. Curr HIV Res. (2012) 10:469–77. doi: 10.2174/157016212802138805
8. Young AM, Havens JR. Transition from first illicit drug use to first injection drug use among rural Appalachian drug users: a cross-sectional comparison and retrospective survival analysis. Addiction. (2012) 107:587–96. doi: 10.1111/j.1360-0443.2011.03635.x
9. Guarino H, Jessell L, Teper A. Injection and sexual HIV/HCV risk behaviors associated with nonmedical use of prescription opioids among young adults in New York City. J Subst Abuse Treat. (2015) 48:13–20. doi: 10.1016/j.jsat.2014.07.002
10. Cunningham CO. Opioids and HIV infection: from pain management to addiction treatment. Top Antivir Med. (2018) 25:143–6. Available online at: https://www.iasusa.org/wp-content/uploads/2018/04/25-4-143.pdf
11. Weisberg DF, Gordon KS, Barry DT, Becker WC, Crystal S, Edelman EJ, et al. Long-term prescription of opioids and/or benzodiazepines and mortality among HIV-infected and uninfected patients. J Acquir Immune Defic Syndr. (2015) 69:223–33. doi: 10.1097/QAI.0000000000000591
12. Springer SA, Copenhaver MM, Altice FL. Neurocognitive impairment and HIV risk factors: a reciprocal relationship. AIDS Behav. (2010) 14:1213–26. doi: 10.1007/s10461-010-9684-1
13. Clifford DB, Ances BM. HIV-associated neurocognitive disorder. Lancet Infect Dis. (2013) 13:976–86. doi: 10.1016/S1473-3099(13)70269-X
14. Heaton RK, Clifford DB, Franklin DR, Woods SP, Ake C, Vaida F, et al. HIV-associated neurocognitive disorders persist in the era of potent antiretroviral therapy: CHARTER Study. Neurology. (2010) 75:2087–96. doi: 10.1212/WNL.0b013e318200d727
15. Saylor D, Dickens AM, Sacktor N, Haughey N, Slusher B, Pletnikov M, et al. HIV-associated neurocognitive disorder - pathogenesis and prospects for treatment. Nat Rev Neurol. (2016) 12:309. doi: 10.1038/nrneurol.2016.53
16. Gu CJ, Borjabad A, Hadas E, Kelschenbach J, Kim BH, Chao W, et al. EcoHIV infection of mice establishes latent viral reservoirs in T cells and active viral reservoirs in macrophages that are sufficient for induction of neurocognitive impairment. PLoS Pathog. (2018) 14:e1007061. doi: 10.1371/journal.ppat.1007061
17. Sanmarti M, Ibanez L, Huertas S, Badenes D, Dalmau D, et al. HIV-associated neurocognitive disorders. J Mol Psychiatry. (2014) 2:2. doi: 10.1186/2049-9256-2-2
18. Grant I, Franklin DR Jr, Deutsch R, Woods SP, Vaida F, Ellis RJ, et al. Asymptomatic HIV-associated neurocognitive impairment increases risk for symptomatic decline. Neurology. (2014) 82:2055–62. doi: 10.1212/WNL.0000000000000492
19. Sacktor N, Skolasky RL, Seaberg E, Munro C, Becker JT, Martin E, et al. Prevalence of HIV-associated neurocognitive disorders in the Multicenter AIDS Cohort Study. Neurology. (2016) 86:334–40. doi: 10.1212/WNL.0000000000002277
20. Altice FL, Bruce RD, Lucas GM, Lum PJ, Korthuis PT, Flanigan TP, et al. HIV treatment outcomes among HIV-infected, opioid-dependent patients receiving buprenorphine/naloxone treatment within HIV clinical care settings: results from a multisite study. J Acquir Immune Defic Syndr. (2011) 56(Suppl. 1):S22–32. doi: 10.1097/QAI.0b013e318209751e
21. Byrd DA, Fellows RP, Morgello S, Franklin D, Heato RK, Deutsch R, et al. Neurocognitive impact of substance use in HIV infection. J Acquir Immune Defic Syndr. (2011) 58:154–62. doi: 10.1097/QAI.0b013e318229ba41
22. Fiellin DA, Weiss L, Botsko M, Egan JE, Altice FL, Bazerman LB, et al. Drug treatment outcomes among HIV-infected opioid-dependent patients receiving buprenorphine/naloxone. J Acquir Immune Defic Syndr. (2011) 56(Suppl. 1):S33–8. doi: 10.1097/QAI.0b013e3182097537
23. Hauser KF, Fitting S, Dever SM, Podhaizer EM, Knapp PE, Opiate drug use and the pathophysiology of neuroAIDS. Curr HIV Res. (2012) 10:435–52. doi: 10.2174/157016212802138779
24. Klinkenberg WD. S. Sacks & for the HIV/aids treatment adherence, health outcomes and cost study group, mental disorders and drug abuse in persons living with HIV/AIDS. AIDS Care. (2004) 16(Suppl. 1):S22–42. doi: 10.1080/09540120412331315303
25. Pirastu R, Fais R, Messina M, Bini V, Spiga S, Falconieri D, et al. Impaired decision-making in opiate-dependent subjects: effect of pharmacological therapies. Drug Alcohol Depend. (2006) 83:163–8. doi: 10.1016/j.drugalcdep.2005.11.008
26. Roy S, Ninkovic J, Banerjee S, Charboneau RG, Das S, Dutta R, et al. Opioid drug abuse and modulation of immune function: consequences in the susceptibility to opportunistic infections. J Neuroimmune Pharmacol. (2011) 6:442–65. doi: 10.1007/s11481-011-9292-5
27. Valcour V, Chalermchai T, Sailasuta N, Marovich M, Lerdlum S, Suttichom D, et al. Central nervous system viral invasion and inflammation during acute HIV infection. J Infect Dis. (2012) 206:275–82. doi: 10.1093/infdis/jis326
28. Witwer KW, Gama L, Li M, Bartizal CM, Queen SE, Varrone JJ, et al. Coordinated regulation of SIV replication and immune responses in the CNS. PLoS ONE. (2009) 4:e8129. doi: 10.1371/journal.pone.0008129
29. Dahl V, Peterson J, Fuchs D, Gisslen M, Palmer S, Price RW. Low levels of HIV-1 RNA detected in the cerebrospinal fluid after up to 10 years of suppressive therapy are associated with local immune activation. AIDS. (2014) 28:2251–8. doi: 10.1097/QAD.0000000000000400
30. Ellery PJ, Tippett E, Chiu Y, Paukovics G, Cameron PU, Solomon A, et al. The CD16+ monocyte subset is more permissive to infection and preferentially harbors HIV-1 in vivo. J Immunol. (2007) 178:6581–9. doi: 10.4049/jimmunol.178.10.6581
31. Williams DW, Veenstra M, Gaskill PJ, Morgello S, Calderon TM, Berman JW. Monocytes mediate HIV neuropathogenesis: mechanisms that contribute to HIV associated neurocognitive disorders. Curr HIV Res. (2014) 12:85–96. doi: 10.2174/1570162X12666140526114526
32. Buckner CM, Luers AJ, Calderon TM, Eugenin EA, Berman JW. Neuroimmunity and the blood-brain barrier: molecular regulation of leukocyte transmigration and viral entry into the nervous system with a focus on neuroAIDS. J Neuroimmune Pharmacol. (2006) 1:160–81. doi: 10.1007/s11481-006-9017-3
33. Eugenin EA, Osiecki K, Lopez L, Goldstein H, Calderon TM, Berman JW. CCL2/monocyte chemoattractant protein-1 mediates enhanced transmigration of human immunodeficiency virus (HIV)-infected leukocytes across the blood-brain barrier: a potential mechanism of HIV-CNS invasion and NeuroAIDS. J Neurosci. (2006) 26:1098–106. doi: 10.1523/JNEUROSCI.3863-05.2006
34. Rostasy K, Egles C, Chauhan A, Kneissl M, Bahrani P, Yiannoutsos C, et al. SDF-1alpha is expressed in astrocytes and neurons in the AIDS dementia complex: an in vivo and in vitro study. J Neuropathol Exp Neurol. (2003) 62:617–26. doi: 10.1093/jnen/62.6.617
35. Maus U, Henning S, Wenschuh H, Mayer K, Seeger W, Lohmeyer J. Role of endothelial MCP-1 in monocyte adhesion to inflamed human endothelium under physiological flow. Am J Physiol Heart Circ Physiol. (2002) 283:H2584–91. doi: 10.1152/ajpheart.00349.2002
36. Ge S, Song L, Serwanski DR, Kuziel WA, Pachter JS. Transcellular transport of CCL2 across brain microvascular endothelial cells. J Neurochem. (2008) 104:1219–32. doi: 10.1111/j.1471-4159.2007.05056.x
37. Volpe S, Cameroni E, Moepps B, Thelen S, Apuzzo T, Thelen M. CCR2 acts as scavenger for CCL2 during monocyte chemotaxis. PLoS ONE. (2012) 7:e37208. doi: 10.1371/journal.pone.0037208
38. Mestas J, Ley K. Monocyte-endothelial cell interactions in the development of atherosclerosis. Trends Cardiovasc Med. (2008) 18:228–32. doi: 10.1016/j.tcm.2008.11.004
39. Meerschaert J, Furie MB. The adhesion molecules used by monocytes for migration across endothelium include CD11a/CD18, CD11b/CD18, and VLA-4 on monocytes and ICAM-1, VCAM-1, and other ligands on endothelium. J Immunol. (1995) 154:4099–112.
40. Gerszten RE, Garcia-Zepeda EA, Lim YC, Yoshida M, Ding HA, Gimbrone MA Jr, et al. MCP-1 and IL-8 trigger firm adhesion of monocytes to vascular endothelium under flow conditions. Nature. (1999) 398:718–23. doi: 10.1038/19546
41. Williams DW, Eugenin EA, Calderon TM, Berman JW. Monocyte maturation, HIV susceptibility, and transmigration across the blood brain barrier are critical in HIV neuropathogenesis. J Leukoc Biol. (2012). 91:401–15. doi: 10.1189/jlb.0811394
42. Cayrol R, Wosik K, Berard JL, Dodelet-Devillers A, Ifergan I, Kebir H, et al. Activated leukocyte cell adhesion molecule promotes leukocyte trafficking into the central nervous system. Nat Immunol. (2008) 9:137–45. doi: 10.1038/ni1551
43. Martìn-Padura I, Lostaglio S, Schneemann M, Williams L, Romano M, Fruscella P, et al. Junctional adhesion molecule, a novel member of the immunoglobulin superfamily that distributes at intercellular junctions and modulates monocyte transmigration. J Cell Biol. (1998) 142:117–27. doi: 10.1083/jcb.142.1.117
44. Sullivan DP, Muller WA. Neutrophil and monocyte recruitment by PECAM, CD99, and other molecules via the LBRC. Semin Immunopathol. (2014) 36:193–209. doi: 10.1007/s00281-013-0412-6
45. Weber C, Fraemohs L, Dejana E. The role of junctional adhesion molecules in vascular inflammation. Nat Rev Immunol. (2007) 7:467–77. doi: 10.1038/nri2096
46. Williams DW, Calderon TM, Lopez L, Carvallo-Torres L, Gaskill PJ, Eugenin EA, et al. Mechanisms of HIV entry into the CNS: increased sensitivity of HIV infected CD14+CD16+ monocytes to CCL2 and key roles of CCR2, JAM-A, and ALCAM in diapedesis. PLoS ONE. (2013) 8:e69270. doi: 10.1371/journal.pone.0069270
47. Churchill MJ, Gorry PR, Cowley D, Lal L, Sonza S, Purcell DF, et al. Use of laser capture microdissection to detect integrated HIV-1 DNA in macrophages and astrocytes from autopsy brain tissues. J Neurovirol. (2006) 12:146–52. doi: 10.1080/13550280600748946
48. Hong S, Banks WA. Role of the immune system in HIV-associated neuroinflammation and neurocognitive implications. Brain Behav Immun. (2015) 45:1–12. doi: 10.1016/j.bbi.2014.10.008
49. Thompson KA, Cherry CL, Bell JE, McLean CA. Brain cell reservoirs of latent virus in presymptomatic HIV-infected individuals. Am J Pathol. (2011) 179:1623–9. doi: 10.1016/j.ajpath.2011.06.039
50. Conant K, Garzino-Demo A, Nath A, McArthur JC, Halliday W, Power C, et al. Induction of monocyte chemoattractant protein-1 in HIV-1 Tat-stimulated astrocytes and elevation in AIDS dementia. Proc Natl Acad Sci USA. (1998) 95:3117–21. doi: 10.1073/pnas.95.6.3117
51. Dhillon NK, Williams R, Callen S, Zien C, Narayan O, Buch S. Roles of MCP-1 in development of HIV-dementia. Front Biosci. (2008) 13:3913–8. doi: 10.2741/2979
52. Kaul M, Garden GA, Lipton SA. Pathways to neuronal injury and apoptosis in HIV-associated dementia. Nature. (2001) 410:988–94. doi: 10.1038/35073667
53. Lindl KA, Marks DR, Kolson DL, Jordan-Sciutto KL. HIV-associated neurocognitive disorder: pathogenesis and therapeutic opportunities. J Neuroimmune Pharmacol. (2010) 5:294–309. doi: 10.1007/s11481-010-9205-z
54. Nash B, Festa L, Lin C, Meucci O. Opioid and chemokine regulation of cortical synaptodendritic damage in HIV-associated neurocognitive disorders. Brain Res. (2019) 1723:146409. doi: 10.1016/j.brainres.2019.146409
55. Stefano GB, Salzet M, Bilfinger TV. Long-term exposure of human blood vessels to HIV gp120, morphine, and anandamide increases endothelial adhesion of monocytes: uncoupling of nitric oxide release. J Cardiovasc Pharmacol. (1998) 31:862–8. doi: 10.1097/00005344-199806000-00009
56. Hatsukari I, Hitosugi N, Dinda A, Singhal PC. Morphine modulates migration of monocytes. Nephron. (1996) 73:526–31. doi: 10.1159/000189135
57. Bokhari SM, Hegde R, Callen S, Yao H, Adany I, Li Q, et al. Morphine potentiates neuropathogenesis of SIV infection in rhesus macaques. J Neuroimmune Pharmacol. (2011) 6:626–39. doi: 10.1007/s11481-011-9272-9
58. Dutta R, RoyS. Chronic morphine and HIV-1 Tat promote differential central nervous system trafficking of CD3+ and Ly6C+ immune cells in a murine Streptococcus pneumoniae infection model. J Neuroinflammation. (2015) 12:120. doi: 10.1186/s12974-015-0341-5
59. Coley JS, Calderon TM, Gaskill PJ, Eugenin EA, Berman JW. Dopamine increases CD14+CD16+ monocyte migration and adhesion in the context of substance abuse and HIV neuropathogenesis. PLoS ONE. (2015) 10:e0117450. doi: 10.1371/journal.pone.0117450
60. Calderon TM, Williams DW, Lopez L, Eugenin EA, Cheney L, Gaskill PJ, et al. Dopamine Increases CD14(+)CD16(+) Monocyte Transmigration across the Blood Brain Barrier: implications for substance abuse and HIV neuropathogenesis. J Neuroimmune Pharmacol. (2017) 12:353–70. doi: 10.1007/s11481-017-9726-9
61. Jaureguiberry-Bravo M, Lopez L, Berman JW. Frontline science: buprenorphine decreases CCL2-mediated migration of CD14(+) CD16(+) monocytes. J Leukoc Biol. (2018) 104:1049–59. doi: 10.1002/JLB.3HI0118-015R
62. Carvallo L, Lopez L, Che FY, Lim J, Eugenin EA, Williams DW, et al. Buprenorphine decreases the CCL2-mediated chemotactic response of monocytes. J Immunol. (2015) 194:3246–58. doi: 10.4049/jimmunol.1302647
63. Guo CJ, Li Y, Tian S, Wang X, Douglas SD, Ho WZ, et al. Morphine enhances HIV infection of human blood mononuclear phagocytes through modulation of beta-chemokines and CCR5 receptor. J Investig Med. (2002) 50:435–42. doi: 10.1136/jim-50-06-03
64. Li Y, Merrill JD, Mooney K, Song L, Wang X, Guo CJ, et al. Morphine enhances HIV infection of neonatal macrophages. Pediatr Res. (2003) 54:282–8. doi: 10.1203/01.PDR.0000074973.83826.4C
65. Dave RS, Khalili K. Morphine treatment of human monocyte-derived macrophages induces differential miRNA and protein expression: impact on inflammation and oxidative stress in the central nervous system. J Cell Biochem. (2010) 110:834–45. doi: 10.1002/jcb.22592
66. Reddy PV, Pilakka-Kanthikeel S, Saxena SK, Saiyed Z, Nair MP. Interactive effects of morphine on HIV infection: role in HIV-associated neurocognitive disorder. AIDS Res Treat. (2012) 2012:953678. doi: 10.1155/2012/953678
67. Reynolds JL, Law WC, Mahajan SD, Aalinkeel R, Nair B, Sykes DE, et al. Morphine and galectin-1 modulate HIV-1 infection of human monocyte-derived macrophages. J Immunol. (2012) 188:3757–65. doi: 10.4049/jimmunol.1102276
68. Wang Y, Wang X, Ye L, Li J, Song L, Fulambarkar N, et al. Morphine suppresses IFN signaling pathway and enhances AIDS virus infection. PLoS ONE. (2012) 7:e31167. doi: 10.1371/journal.pone.0031167
69. El-Hage N, Rodriguez M, Dever SM, Masvekar RR, Gewirtz DA, Shacka JJ. HIV-1 and morphine regulation of autophagy in microglia: limited interactions in the context of HIV-1 infection and opioid abuse. J Virol. (2015) 89:1024–35. doi: 10.1128/JVI.02022-14
70. Dave RS, Khalili K. Morphine affects HIV-induced inflammatory response without influencing viral replication in human monocyte-derived macrophages. FEMS Immunol Med Microbiol. (2012) 64:228–36. doi: 10.1111/j.1574-695X.2011.00894.x
71. Franchi S, Castelli M, Moretti S, Panerai A, Sacerdote P, et al. Evaluation of murine macrophage cytokine production after in vivo morphine treatment. Methods Mol Biol. (2015) 1230:253–61. doi: 10.1007/978-1-4939-1708-2_21
72. Plein LM, Rittner HL. Opioids and the immune system - friend or foe. Br J Pharmacol. (2018) 175:2717–25. doi: 10.1111/bph.13750
73. Turchan-Cholewo J, Dimayuga FO, Gupta S, Keller JN, Knapp PE, Hauser KF, et al. Morphine and HIV-Tat increase microglial-free radical production and oxidative stress: possible role in cytokine regulation. J Neurochem. (2009) 108:202–15. doi: 10.1111/j.1471-4159.2008.05756.x
74. Iuvone T, Capasso A, D'Acquisto F, Carnuccio R. Opioids inhibit the induction of nitric oxide synthase in J774 macrophages. Biochem Biophys Res Commun. (1995) 212:975–80. doi: 10.1006/bbrc.1995.2065
75. Gaskill PJ, Yano HH, Kalpana GV, Javitch JA, Berman JW. Dopamine receptor activation increases HIV entry into primary human macrophages. PLoS ONE. (2014) 9:e108232. doi: 10.1371/journal.pone.0108232
76. Gaskill PJ, Calderon TM, Luers AJ, Eugenin EA, Javitch JA, Berman JW. Human immunodeficiency virus (HIV) infection of human macrophages is increased by dopamine: a bridge between HIV-associated neurologic disorders and drug abuse. Am J Pathol. (2009) 175:1148–59. doi: 10.2353/ajpath.2009.081067
77. Gaskill PJ, Carvallo L, Eugenin EA, Berman JW. Characterization and function of the human macrophage dopaminergic system: implications for CNS disease and drug abuse. J Neuroinflammation. (2012) 9:203. doi: 10.1186/1742-2094-9-203
78. Lagathu C, Eustace B, Prot M, Frantz D, Gu Y, Bastard JP, et al. Some HIV antiretrovirals increase oxidative stress and alter chemokine, cytokine or adiponectin production in human adipocytes and macrophages. Antivir Ther. (2007) 12:489–500. Available online at: https://www.intmedpress.com/serveFile.cfm?sUID=a33f4ee5-ec5d-4ab8-b3ac-dd22af6a4270
79. Rodriguez M, Lapierre J, Ojha CR, Estrada-Bueno H, Dever SM, Gewirtz DA, et al. Importance of autophagy in mediating human immunodeficiency virus (HIV) and morphine-induced metabolic dysfunction and inflammation in human astrocytes. Viruses. (2017) 9:201. doi: 10.3390/v9080201
80. El-Hage N, Bruce-Keller AJ, Yakovleva T, Bazov I, Bakalkin G, Knapp PE, et al. Morphine exacerbates HIV-1 Tat-induced cytokine production in astrocytes through convergent effects on [Ca(2+)](i), NF-kappaB trafficking and transcription. PLoS ONE. (2008) 3:e4093. doi: 10.1371/journal.pone.0004093
81. Ozawa T, Nakagawa T, Shige K, Minami M, Satoh M. Changes in the expression of glial glutamate transporters in the rat brain accompanied with morphine dependence and naloxone-precipitated withdrawal. Brain Res. (2001) 905:254–8. doi: 10.1016/S0006-8993(01)02536-7
82. Woo DH, Bae JY, Nam MH, An H, Ju YH, Won J, et al. Activation of astrocytic mu-opioid receptor elicits fast glutamate release through TREK-1-containing K2P channel in hippocampal astrocytes. Front Cell Neurosci. (2018) 12:319. doi: 10.3389/fncel.2018.00319
83. Cohen J, D'Agostino L, Wilson J, Tuzer F, Torres C. Astrocyte senescence and metabolic changes in response to HIV antiretroviral therapy drugs. Front Aging Neurosci. (2017) 9:281. doi: 10.3389/fnagi.2017.00281
84. Rodriguez M, Lapierre J, Ojha CR, Estrada-Bueno H, Dever SM, Gewirtz DA, et al. Importance of autophagy in mediating human immunodeficiency virus (HIV) and morphine-induced metabolic dysfunction and inflammation in human astrocytes. Viruses. (2017) 9:201.
85. Vivithanaporn P, Asahchop EL, Acharjee S, Baker GB, Power C. HIV protease inhibitors disrupt astrocytic glutamate transporter function and neurobehavioral performance. AIDS. (2016) 30:543–52. doi: 10.1097/QAD.0000000000000955
86. Sharp BM, McAllen K, Gekker G, Shahabi NA, & Peterson PK Immunofluorescence detection of delta opioid receptors (DOR) on human peripheral blood CD4+ T cells and DOR-dependent suppression of HIV-1 expression. J Immunol. (2001) 167:1097–102. doi: 10.4049/jimmunol.167.2.1097
87. Peterson PK, Gekker G, Lokensgard JR, Bidlack JM, Chang AC, Fang X, et al. Kappa-opioid receptor agonist suppression of HIV-1 expression in CD4+ lymphocytes. Biochem Pharmacol. (2001) 61:1145–51. doi: 10.1016/S0006-2952(01)00574-3
88. Gekker G, Lokensgard JR, Peterson PK. Naltrexone potentiates anti-HIV-1 activity of antiretroviral drugs in CD4+ lymphocyte cultures. Drug Alcohol Depend. (2001) 64:257–63. doi: 10.1016/S0376-8716(01)00140-5
89. Borner C, Warnick B, Smida M, Hartig R, Lindquist JA, Schraven B, et al. Mechanisms of opioid-mediated inhibition of human T cell receptor signaling. J Immunol. (2009) 183:882–9. doi: 10.4049/jimmunol.0802763
90. Mahajan SD, Aalinkeel R, Sykes DE, Reynolds JL, Bindukumar B, Fernandez SF, et al. Tight junction regulation by morphine and HIV-1 tat modulates blood-brain barrier permeability. J Clin Immunol. (2008) 28:528–41. doi: 10.1007/s10875-008-9208-1
91. Strazza M, Pirrone V, Wigdahl B, Dampier W, Lin W, Feng R, et al. Prolonged morphine exposure induces increased firm adhesion in an in vitro model of the blood-brain barrier. Int J Mol Sci. (2016) 17:916.
92. Wen H, Lu Y, Yao H, Buch S. Morphine induces expression of platelet-derived growth factor in human brain microvascular endothelial cells: implication for vascular permeability. PLoS ONE. (2011) 6:e21707. doi: 10.1371/journal.pone.0021707
93. Yousif S, Saubaméa B, Cisternino S, Marie-Claire C, Dauchy S, Scherrmann JM, et al. Effect of chronic exposure to morphine on the rat blood-brain barrier: focus on the P-glycoprotein. J Neurochem. (2008) 107:647–57. doi: 10.1111/j.1471-4159.2008.05647.x
94. Leibrand CR, Paris JJ, Jones AM, Masuda QN, Halquist MS, Kim WK, et al. HIV-1 tat and opioids act independently to limit antiretroviral brain concentrations and reduce blood-brain barrier integrity. J Neurovirol. (2019) 25:560–77.
95. Volkow ND, Morales M. The brain on drugs: from reward to addiction. Cell. (2015) 162:712–25. doi: 10.1016/j.cell.2015.07.046
96. Volkow ND, Fowler JS, Wang GJ, Swanson JM, Telang F. Dopamine in drug abuse and addiction: results of imaging studies and treatment implications. Arch Neurol. (2007) 64:1575–9. doi: 10.1001/archneur.64.11.1575
97. Di Chiara G. The role of dopamine in drug abuse viewed from the perspective of its role in motivation. Drug Alcohol Depend. (1995) 38:95–137. doi: 10.1016/0376-8716(95)01118-I
98. McKenna F, McLaughlin PJ, Lewis BJ, Sibbring GC, Cummerson JA, Bowen-Jones D, et al. Dopamine receptor expression on human T- and B-lymphocytes, monocytes, neutrophils, eosinophils and NK cells: a flow cytometric study. J Neuroimmunol. (2002) 132:34–40. doi: 10.1016/S0165-5728(02)00280-1
99. Kofler J, Wiley CA. Microglia: key innate immune cells of the brain. Toxicol Pathol. (2011) 39:103–14. doi: 10.1177/0192623310387619
100. Faraco G, Park L, Anrather J, Iadecola C. Brain perivascular macrophages: characterization and functional roles in health and disease. J Mol Med (Berl). (2017) 95:1143–52. doi: 10.1007/s00109-017-1573-x
101. Block ML, Zecca L, Hong JS. Microglia-mediated neurotoxicity: uncovering the molecular mechanisms. Nat Rev Neurosci. (2007) 8:57–69. doi: 10.1038/nrn2038
102. Ninkovic J, Roy S. Morphine decreases bacterial phagocytosis by inhibiting actin polymerization through cAMP-, Rac-1-, and p38 MAPK-dependent mechanisms. Am J Pathol. (2012) 180:1068–79. doi: 10.1016/j.ajpath.2011.11.034
103. Ataka K, Asakawa A, Nagaishi K, Kaimoto K, Sawada A, Hayakawa Y, et al. Bone marrow-derived microglia infiltrate into the paraventricular nucleus of chronic psychological stress-loaded mice. PLoS ONE. (2013) 8:e81744. doi: 10.1371/journal.pone.0081744
104. Jin N, Gao L, Fan X, Xu H. Friend or foe? resident microglia vs bone marrow-derived microglia and their roles in the retinal degeneration. Mol Neurobiol. (2017) 54:4094–112. doi: 10.1007/s12035-016-9960-9
105. Cronk JC, Filiano AJ, Louveau A, Marin I, Marsh R, Ji E, et al. Peripherally derived macrophages can engraft the brain independent of irradiation and maintain an identity distinct from microglia. J Exp Med. (2018) 215:1627–47. doi: 10.1084/jem.20180247
106. Haage V, Semtner M, Vidal RO, Hernandez DP, Pong WW, Chen Z, et al. Comprehensive gene expression meta-analysis identifies signature genes that distinguish microglia from peripheral monocytes/macrophages in health and glioma. Acta Neuropathol Commun. (2019) 7:20. doi: 10.1186/s40478-019-0665-y
107. Ko A, Kang G, Hattler JB, Galadima HI, Zhang J, Li Q, et al. Macrophages but not astrocytes harbor HIV DNA in the brains of HIV-1-infected aviremic individuals on suppressive antiretroviral therapy. J Neuroimmune Pharmacol. (2018) 14:110–9. doi: 10.1007/s11481-018-9809-2
108. Avalos CR, Price SL, Forsyth ER, Pin JN, Shirk EN, Bullock BT, et al. Quantitation of productively infected monocytes and macrophages of simian immunodeficiency virus-infected macaques. J Virol. (2016) 90:5643–56. doi: 10.1128/JVI.00290-16
109. Clements JE, Babas T, Mankowski JL, Suryanarayana K, Piatak M Jr, Tarwater PM, et al. The central nervous system as a reservoir for simian immunodeficiency virus (SIV): steady-state levels of SIV DNA in brain from acute through asymptomatic infection. J Infect Dis. (2002) 186:905–13. doi: 10.1086/343768
110. Hauser KF, Knapp PE. Interactions of HIV and drugs of abuse: the importance of glia, neural progenitors, and host genetic factors. Int Rev Neurobiol. (2014) 118:231–313. doi: 10.1016/B978-0-12-801284-0.00009-9
111. Saylor D, Dickens AM, Sacktor N, Haughey N, Slusher B, Pletnikov M, et al. HIV-associated neurocognitive disorder–pathogenesis and prospects for treatment. Nat Rev Neurol. (2016) 12:234–48. doi: 10.1038/nrneurol.2016.27
112. Rappaport J, Volsky DJ. Role of the macrophage in HIV-associated neurocognitive disorders and other comorbidities in patients on effective antiretroviral treatment. J NeuroVirol. (2015) 21:235–41. doi: 10.1007/s13365-015-0346-y
113. Zhu DM, Shi J, Liu S, Liu Y, Zheng D. HIV infection enhances TRAIL-induced cell death in macrophage by down-regulating decoy receptor expression and generation of reactive oxygen species. PLoS ONE. (2011) 6:e18291. doi: 10.1371/journal.pone.0018291
114. Marchetti G, Cozzi-Lepri A, Merlini E, Bellistri GM, Castagna A, Galli M, et al. Microbial translocation predicts disease progression of HIV-infected antiretroviral-naive patients with high CD4+ cell count. AIDS. (2011) 25:1385–94. doi: 10.1097/QAD.0b013e3283471d10
115. Rothwell N. Interleukin-1 and neuronal injury: mechanisms, modification, and therapeutic potential. Brain BehavImmun. (2003) 17:152–7. doi: 10.1016/S0889-1591(02)00098-3
116. Pasternak GW, Pan YX. Mu opioids and their receptors: evolution of a concept. Pharmacol Rev. (2013) 65:1257–317. doi: 10.1124/pr.112.007138
117. Parker KE, Bruchas MR. NOP receptor signaling cascades. Handb Exp Pharmacol. (2019) 254:131–9. doi: 10.1007/164_2019_215
118. Filipczak-Bryniarska I, Nowak B, Sikora E, Nazimek K, Woron J, Wordliczek J, et al. The influence of opioids on the humoral and cell-mediated immune responses in mice. The role of macrophages. Pharmacol Rep. (2012) 64:1200–15. doi: 10.1016/S1734-1140(12)70916-7
119. Franchi S, Moretti S, Castelli M, Lattuada D, Scavullo C, Panerai AE, et al. Mu opioid receptor activation modulates Toll like receptor 4 in murine macrophages. Brain Behav Immun. (2012) 26:480–8. doi: 10.1016/j.bbi.2011.12.010
120. Ananworanich J, Chomont N, Fletcher JL, Pinyakorn S, Schuetz A, Sereti I, et al. Markers of HIV reservoir size and immune activation after treatment in acute HIV infection with and without raltegravir and maraviroc intensification. J Virus Erad. (2015) 1:116–22. Available online at: http://viruseradication.com/journal-details/Markers_of_HIV_reservoir_size_and_immune_activation_after_treatment_in_acute_HIV_infection_with_and_without_raltegravir_and_maraviroc_intensification/.
121. Ndhlovu LC, Umaki T, Chew GM, Chow DC, Agsalda M, Kallianpur KJ, et al. Treatment intensification with maraviroc (CCR5 antagonist) leads to declines in CD16-expressing monocytes in cART-suppressed chronic HIV-infected subjects and is associated with improvements in neurocognitive test performance: implications for HIV-associated neurocognitive disease (HAND). J Neurovirol. (2014) 20:571–82. doi: 10.1007/s13365-014-0279-x
122. Gill AJ, Kovacsics CE, Vance PJ, Collman RG, Kolson DL. Induction of heme oxygenase-1 deficiency and associated glutamate-mediated neurotoxicity is a highly conserved HIV phenotype of chronic macrophage infection that is resistant to antiretroviral therapy. J Virol. (2015) 89:10656–67. doi: 10.1128/JVI.01495-15
123. Akay C, Cooper M, Odeleye A, Jensen BK, White MG, Vassoler F, et al. Antiretroviral drugs induce oxidative stress and neuronal damage in the central nervous system. J Neurovirol. (2014) 20:39–53. doi: 10.1007/s13365-013-0227-1
124. Krakower DS, Jain S, Mayer KH. Antiretrovirals for primary HIV prevention: the current status of pre- and post-exposure prophylaxis. Curr HIV/AIDS Rep. (2015) 12:127–38. doi: 10.1007/s11904-014-0253-5
125. Nwogu JN, Ma Q, Babalola CP, Adedeji WA, Morse GD, Taiwo B, et al. Pharmacokinetic, pharmacogenetic, and other factors influencing CNS penetration of antiretrovirals. AIDS Res Treat. (2016) 2016:2587094. doi: 10.1155/2016/2587094
126. Vasile F, Dossi E, Rouach N. Human astrocytes: structure and functions in the healthy brain. Brain Struct Funct. (2017) 222:2017–29. doi: 10.1007/s00429-017-1383-5
127. Verkhratsky A, Nedergaard M. Physiology of astroglia. Physiol Rev. (2018) 98:239–389. doi: 10.1152/physrev.00042.2016
128. Oberheim NA, Takano T, Han X, He W, Lin JH, Wang F, et al. Uniquely hominid features of adult human astrocytes. J Neurosci. (2009) 29:3276–87. doi: 10.1523/JNEUROSCI.4707-08.2009
129. Kimelberg HK, Nedergaard M. Functions of astrocytes and their potential as therapeutic targets. Neurotherapeutics. (2010) 7:338–53. doi: 10.1016/j.nurt.2010.07.006
130. Zhang Y, Sloan SA, Clarke LE, Caneda C, Plaza CA, Blumenthal PD, et al. Purification and characterization of progenitor and mature human astrocytes reveals transcriptional and functional differences with mouse. Neuron. (2016) 89:37–53. doi: 10.1016/j.neuron.2015.11.013
131. Ru W, Tang SJ. HIV-associated synaptic degeneration. Mol Brain. (2017) 10:40. doi: 10.1186/s13041-017-0321-z
132. Croitoru-Lamoury J, Guillemin GJ, Boussin FD, Mognetti B, Gigout LI, Cheret A, et al. Expression of chemokines and their receptors in human and simian astrocytes: evidence for a central role of TNF alpha and IFN gamma in CXCR4 and CCR5 modulation. Glia. (2003) 41:354–70. doi: 10.1002/glia.10181
133. Dorf ME, Berman MA, Tanabe S, Heesen M, Luo Y. Astrocytes express functional chemokine receptors. J Neuroimmunol. (2000) 111:109–21. doi: 10.1016/S0165-5728(00)00371-4
134. Abbott NJ, Ronnback L, Hansson E. Astrocyte-endothelial interactions at the blood-brain barrier. Nat Rev Neurosci. (2006) 7:41–53. doi: 10.1038/nrn1824
135. Al-Harti L, Joseph J, Nath A. Astrocytes as an HIV CNS reservoir: highlights and reflections of an NIMH-sponsored symposium. J Neurovirol. (2018) 24:665–669. doi: 10.1007/s13365-018-0691-8
136. Desplats P, Dumaop W, Smith D, Adame A, Everall I, Letendre S, et al. Molecular and pathologic insights from latent HIV-1 infection in the human brain. Neurology. (2013) 80:1415–23. doi: 10.1212/WNL.0b013e31828c2e9e
137. Brack-Werner R. Astrocytes: HIV cellular reservoirs and important participants in neuropathogenesis. AIDS. (1999) 13:1–22. doi: 10.1097/00002030-199901140-00003
138. Serramia MJ, Munoz-Fernandez MA, Alvarez S. HIV-1 increases TLR responses in human primary astrocytes. Sci Rep. (2015) 5:17887. doi: 10.1038/srep17887
139. Krasovska V, Doering LC. Regulation of IL-6 secretion by astrocytes via TLR4 in the fragile X mouse model. Front Mol Neurosci. (2018) 11:272. doi: 10.3389/fnmol.2018.00272
140. Berman JW, Carvallo L, Buckner CM, Luers A, Prevedel L, Bennett MV, et al. HIV-tat alters Connexin43 expression and trafficking in human astrocytes: role in NeuroAIDJS Neuroinflammation. (2016) 13:54. doi: 10.1186/s12974-016-0510-1
141. Orellana JA, Saez JC, Bennett MV, Berman JW, Morgello S, Eugenin EA. HIV increases the release of dickkopf-1 protein from human astrocytes by a Cx43 hemichannel-dependent mechanism. J Neurochem. (2014) 128:752–63. doi: 10.1111/jnc.12492
142. Eugenin EA, Clements JE, Zink MC, Berman JW. Human immunodeficiency virus infection of human astrocytes disrupts blood-brain barrier integrity by a gap junction-dependent mechanism. J Neurosci. (2011) 31:9456–65. doi: 10.1523/JNEUROSCI.1460-11.2011
143. Zhou F, Liu X, Zuo D, Xue M, Gao L, Yang Y, et al. HIV-1 Nef-induced lncRNA AK006025 regulates CXCL9/10/11 cluster gene expression in astrocytes through interaction with CBP/P300. J Neuroinflammation. (2018) 15:303. doi: 10.1186/s12974-018-1343-x
144. Yang L, Niu F, Yao H, Liao K, Chen X, Kook Y, et al. Exosomal miR-9 released from HIV tat stimulated astrocytes mediates microglial migration. J Neuroimmune Pharmacol. (2018) 13:330–44. doi: 10.1007/s11481-018-9779-4
145. Ojeda DS, Grasso D, Urquiza J, Till A, Vaccaro MI, Quarleri J. Cell death is counteracted by mitophagy in HIV-productively infected astrocytes but is promoted by inflammasome activation among non-productively infected cells. Front Immunol. (2018) 9:2633. doi: 10.3389/fimmu.2018.02633
146. Nam MH, Han KS, Lee J, Bae JY, An H, Park S, et al. Expression of micro-opioid receptor in CA1 hippocampal astrocytes. Exp Neurobiol. (2018) 27:120–8. doi: 10.5607/en.2018.27.2.120
147. Liang J, Chao D, Sandhu HK, Yu Y, Zhang L, Balboni G, et al. delta-Opioid receptors up-regulate excitatory amino acid transporters in mouse astrocytes. Br J Pharmacol. (2014) 171:5417–30. doi: 10.1111/bph.12857
148. Zou S, Fitting S, Hahn YK, Welch SP, El-Hage N, Hauser KF, et al. Morphine potentiates neurodegenerative effects of HIV-1 Tat through actions at mu-opioid receptor-expressing glia. Brain. (2011) 134(Pt 12):3616–31. doi: 10.1093/brain/awr281
149. Shah A, Verma AS, Patel KH, Noel R, Rivera-Amill V, Silverstein PS, et al. HIV-1 gp120 induces expression of IL-6 through a nuclear factor-kappa B-dependent mechanism: suppression by gp120 specific small interfering RNA. PLoS ONE. (2011) 6:e21261. doi: 10.1371/journal.pone.0021261
150. Happel C, Kutzler M, Rogers TJ. Opioid-induced chemokine expression requires NF-kappaB activity: the role of PKCzeta. J Leukoc Biol. (2011) 89:301–9. doi: 10.1189/jlb.0710402
151. Du SH, Qiao DF, Chen CX, Chen S, Liu C, Lin Z, et al. Toll-like receptor 4 mediates methamphetamine-induced neuroinflammation through caspase-11 signaling pathway in astrocytes. Front Mol Neurosci. (2017) 10:409. doi: 10.3389/fnmol.2017.00409
152. Hutchinson MR, Northcutt AL, Hiranita T, Wang X, Lewis SS, Thomas J, et al. Opioid activation of toll-like receptor 4 contributes to drug reinforcement. J Neurosci. (2012) 32:11187–200. doi: 10.1523/JNEUROSCI.0684-12.2012
153. Kim S, Hahn YK, Podhaizer EM, McLane VD, Zou S, Hauser KF, et al. A central role for glial CCR5 in directing the neuropathological interactions of HIV-1 Tat and opiates. J Neuroinflammation. (2018) 15:285. doi: 10.1186/s12974-018-1320-4
154. Giunti D, Borsellino G, Benelli R, Marchese M, Capello E, Valle MT, et al. Phenotypic and functional analysis of T cells homing into the CSF of subjects with inflammatory diseases of the CNS. J Leukoc Biol. (2003) 73:584–90. doi: 10.1189/jlb.1202598
155. Nguyen TP, Soukup VM, Gelman BB. Persistent hijacking of brain proteasomes in HIV-associated dementia. Am J Pathol. (2010) 176:893–902. doi: 10.2353/ajpath.2010.090390
156. Ellis RJ, Badiee J, Vaida F, Letendre S, Heaton RK, Clifford D, et al. CD4 nadir is a predictor of HIV neurocognitive impairment in the era of combination antiretroviral therapy. AIDS. (2011) 25:1747–51. doi: 10.1097/QAD.0b013e32834a40cd
157. Petito CK, Adkins B, McCarthy M, Roberts B, Khamis I, et al. CD4+ and CD8+ cells accumulate in the brains of acquired immunodeficiency syndrome patients with human immunodeficiency virus encephalitis. J Neurovirol. (2003) 9:36–44. doi: 10.1080/13550280390173391
158. Schrier RD, Hong S, Crescini M, Ellis R, Perez-Santiago J, Spina C, et al. Cerebrospinal fluid (CSF) CD8+ T-cells that express interferon-gamma contribute to HIV associated neurocognitive disorders (HAND). PLoS ONE. (2015) 10:e0116526. doi: 10.1371/journal.pone.0116526
159. Shacklett BL, Cox CA, Wilkens DT, Karl Karlsson R, Nilsson A, Nixon DF, et al. Increased adhesion molecule and chemokine receptor expression on CD8+ T cells trafficking to cerebrospinal fluid in HIV-1 infection. J Infect Dis. (2004) 189:2202–12. doi: 10.1086/421244
160. Manfredi B, Sacerdote P, Bianchi M, Locatelli L, Veljic-Radulovic J, Panerai AE. Evidence for an opioid inhibitory effect on T cell proliferation. J Neuroimmunol. (1993) 44:43–8. doi: 10.1016/0165-5728(93)90266-2
161. Riss GL, Chang DI, Wevers C, Westendorf AM, Buer J, Scherbaum N, et al. Opioid maintenance therapy restores CD4+ T cell function by normalizing CD4+CD25(high) regulatory T cell frequencies in heroin user. Brain Behav Immun. (2012) 26:972–8. doi: 10.1016/j.bbi.2012.05.008
162. McCarthy L, Szabo I, Nitsche JF, Pintar JE, Rogers TJ. Expression of functional mu-opioid receptors during T cell development. J Neuroimmunol. (2001) 114:173–80. doi: 10.1016/S0165-5728(01)00248-X
163. Moorman J, Zhang Y, Liu B, LeSage G, Chen Y, Stuart C, et al. HIV-1 gp120 primes lymphocytes for opioid-induced, beta-arrestin 2-dependent apoptosis. Biochim Biophys Acta. (2009) 1793:1366–71. doi: 10.1016/j.bbamcr.2009.05.007
164. Prottengeier J, Koutsilieri E, Scheller C. The effects of opioids on HIV reactivation in latently-infected T-lymphoblasts. AIDS Res Ther. (2014) 11:17. doi: 10.1186/1742-6405-11-17
165. Wang X, Tan N, Douglas SD, Zhang T, Wang YJ, Ho WZ. Morphine inhibits CD8+ T cell-mediated, noncytolytic, anti-HIV activity in latently infected immune cells. J Leukoc Biol. (2005) 78:772–6. doi: 10.1189/jlb.0305167
166. Whelan PJ, Remski K. Buprenorphine vs methadone treatment: a review of evidence in both developed and developing worlds. J Neurosci Rural Pract. (2012) 3:45–50. doi: 10.4103/0976-3147.91934
167. Mattick RP, Breen C, Kimber J, Davoli M. Buprenorphine maintenance versus placebo or methadone maintenance for opioid dependence. Cochr Database Syst Rev. (2014) 2:CD002207. doi: 10.1002/14651858.CD002207.pub4
168. Anderson IB, Kearney TE. Use of methadone. West J Med. (2000) 172:43–6. doi: 10.1136/ewjm.172.1.43
169. Lutfy K, Cowan A. Buprenorphine: a unique drug with complex pharmacology. Curr Neuropharmacol. (2004) 2:395–402. doi: 10.2174/1570159043359477
170. Scott TM, Rivera Mindt M, Cunningham CO, Arias F, Coulehan K, Mangalonzo A, et al. Neuropsychological function is improved among opioid dependent adults who adhere to opiate agonist treatment with buprenorphine-naloxone: a preliminary study. Subst Abuse Treat Prev Policy. (2017) 12:48. doi: 10.1186/s13011-017-0133-2
171. Rapeli P, Fabritius C, Alho H, Salaspuro M, Wahlbeck K, Kalska H, Methadone vs. buprenorphine/naloxone during early opioid substitution treatment: a naturalistic comparison of cognitive performance relative to healthy controls. BMC Clin Pharmacol. (2007) 7:5. doi: 10.1186/1472-6904-7-5
172. Soyka M, Limmer C, Lehnert R, Koller G, Martin G, Küfner H, et al. A comparison of cognitive function in patients under maintenance treatment with heroin, methadone, or buprenorphine and healthy controls: an open pilot study. Am J Drug Alcohol Abuse. (2011) 37:497–508. doi: 10.3109/00952990.2011.600381
173. Loeber S, Kniest A, Diehl A, Mann K, Croissant B. Neuropsychological functioning of opiate-dependent patients: a nonrandomized comparison of patients preferring either buprenorphine or methadone maintenance treatment. Am J Drug Alcohol Abuse. (2008) 34:584–93. doi: 10.1080/00952990802308239
174. Darke S, Sims J, McDonald S, Wickes W. Cognitive impairment among methadone maintenance patients. Addiction. (2000) 95:687–95. doi: 10.1046/j.1360-0443.2000.9556874.x
175. Mazhari S, Keshvari Z, Sabahi A, Mottaghian S. Assessment of cognitive functions in methadone maintenance patients. Addict Health. (2015) 7:109–16.
176. Mukandavire C, Low A, Mburu G, Trickey A, May MT, Davies CF, et al. Impact of opioid substitution therapy on the HIV prevention benefit of antiretroviral therapy for people who inject drugs. AIDS. (2017) 31:1181–90. doi: 10.1097/QAD.0000000000001458
177. Ahamad K, Hayashi K, Nguyen P, Dobrer S, Kerr T, Schutz CG, et al. Effect of low-threshold methadone maintenance therapy for people who inject drugs on HIV incidence in Vancouver BC, Canada: an observational cohort study. Lancet HIV. (2015) 2:e445–50. doi: 10.1016/S2352-3018(15)00129-0
178. Altice FL, Sullivan LE, Smith-Rohrberg D, Basu S, Stancliff S, Eldred L. The potential role of buprenorphine in the treatment of opioid dependence in HIV-infected individuals and in HIV infection prevention. Clin Infect Dis. (2006) 43(Suppl. 4):S178–83. doi: 10.1086/508181
179. Korthuis PT, Tozzi MJ, Nandi V, Fiellin DA, Weiss L, Egan JE, et al. Improved quality of life for opioid-dependent patients receiving buprenorphine treatment in HIV clinics. J Acquir Immune Defic Syndr. (2011) 56(Suppl. 1):S39–45. doi: 10.1097/QAI.0b013e318209754c
180. Lawrinson P, Ali R, Buavirat A, Chiamwongpaet S, Dvoryak S, Habrat B. Key findings from the WHO collaborative study on substitution therapy for opioid dependence and HIV/AIDS. Addiction. (2008) 103:1484–92. doi: 10.1111/j.1360-0443.2008.02249.x
181. Marsch LA. The efficacy of methadone maintenance interventions in reducing illicit opiate use, HIV risk behavior and criminality: a meta-analysis. Addiction. (1998) 93:515–32. doi: 10.1046/j.1360-0443.1998.9345157.x
182. Vázquez-Justo E, Vergara-Moragues E, Blanco AP, Gestoso CG, Pérez-García M. Neuropsychological functioning in methadone maintenance patients with HIV. Revista Latinoamericana de Psicología. (2016) 48:147–8. doi: 10.1016/j.rlp.2015.06.008
183. Springer SA, Chen S, Altice FL. Improved HIV, and substance abuse treatment outcomes for released HIV-infected prisoners: the impact of buprenorphine treatment. J Urban Health. (2010) 87:592–602. doi: 10.1007/s11524-010-9438-4
184. Khanna IK, Pillarisetti S. Buprenorphine - an attractive opioid with underutilized potential in treatment of chronic pain. J Pain Res. (2015) 8:859–70. doi: 10.2147/JPR.S85951
185. Rankovic Z, Brust TF, Bohn LM. Biased agonism: an emerging paradigm in GPCR drug discovery. Bioorg Med Chem Lett. (2016) 26:241–50. doi: 10.1016/j.bmcl.2015.12.024
186. Szabo I, Chen XH, Xin L, Adler MW, Howard OM, Oppenheim JJ, et al. Heterologous desensitization of opioid receptors by chemokines inhibits chemotaxis and enhances the perception of pain. Proc Natl Acad Sci USA. (2002) 99:10276–81. doi: 10.1073/pnas.102327699
187. Zhang N, Rogers TJ, Caterina M, Oppenheim JJ. Proinflammatory chemokines, such as C-C chemokine ligand 3, desensitize mu-opioid receptors on dorsal root ganglia neurons. J Immunol. (2004) 173:594–9. doi: 10.4049/jimmunol.173.1.594
188. Chen C, Li J, Bot G, Szabo I, Rogers TJ, Liu-Chen LY. Heterodimerization and cross-desensitization between the mu-opioid receptor and the chemokine CCR5 receptor. Eur J Pharmacol. (2004) 483:175–86. doi: 10.1016/j.ejphar.2003.10.033
189. Steele AD, Szabo I, Bednar F, Rogers TJ. Interactions between opioid and chemokine receptors: heterologous desensitization. Cytokine Growth Factor Rev. (2002) 13:209–22. doi: 10.1016/S1359-6101(02)00007-2
190. Zhang J, Patel L, Pienta KJ. Targeting chemokine (C-C motif) ligand 2 (CCL2) as an example of translation of cancer molecular biology to the clinic. Prog Mol Biol Transl Sci. (2010) 95:31–53. doi: 10.1016/B978-0-12-385071-3.00003-4
191. Pello OM, Martinez-Munoz L, Parrillas V, Serrano A, Rodriguez-Frade JM, Toro MJ, et al. Ligand stabilization of CXCR4/delta-opioid receptor heterodimers reveals a mechanism for immune response regulation. Eur J Immunol. (2008) 38:537–49. doi: 10.1002/eji.200737630
192. Ugur M, Derouiche L, Massotte D. Heteromerization modulates mu opioid receptor functional properties in vivo. Front Pharmacol. (2018) 9:1240. doi: 10.3389/fphar.2018.01240
193. Fenalti G, Zatsepin NA, Betti C, Giguere P, Han GW, Ishchenko A, et al. Structural basis for bifunctional peptide recognition at human delta-opioid receptor. Nat Struct Mol Biol. (2015) 22:265–8. doi: 10.1038/nsmb.2965
194. Manglik A, Lin H, Aryal DK, McCorvy JD, Dengler D, Corder G, et al. Structure-based discovery of opioid analgesics with reduced side effects. Nature. (2016) 537:185–90. doi: 10.1038/nature19112
195. Zheng Z, Huang XP, Mangano TJ, Zou R, Chen X, Zaidi SA, et al. Structure-based discovery of new antagonist and biased agonist chemotypes for the kappa opioid receptor. J Med Chem. (2017) 60:3070–81. doi: 10.1021/acs.jmedchem.7b00109
196. Bieniasz PD, Kutluay SB. CLIP-related methodologies and their application to retrovirology. Retrovirology. (2018) 15:35. doi: 10.1186/s12977-018-0417-2
197. Jeong S. SR proteins: binders, regulators, and connectors of RNA. Mol Cells. (2017) 40:1–9. doi: 10.14348/molcells.2017.2319
198. Soret J, Bakkour N, Maire S, Durand S, Zekri L, Gabut M, et al. Selective modification of alternative splicing by indole derivatives that target serine-arginine-rich protein splicing factors. Proc Natl Acad Sci USA. (2005) 102:8764–9. doi: 10.1073/pnas.0409829102
199. Bakkour N, Lin YL, Maire S, Ayadi L, Mahuteau-Betzer F, Nguyen CH, et al. Small-molecule inhibition of HIV pre-mRNA splicing as a novel antiretroviral therapy to overcome drug resistance. PLoS Pathog. (2007) 3:1530–9. doi: 10.1371/journal.ppat.0030159
200. Siuda ER, Copits BA, Schmidt MJ, Baird MA, Al-Hasani R, Planer WJ, et al. Spatiotemporal control of opioid signaling and behavior. Neuron. (2015) 86:923–35. doi: 10.1016/j.neuron.2015.03.066
201. Campbell LA, Richie CT, Zhang Y, Heathward EJ, Coke LM, Park EY, et al. In vitro modeling of HIV proviral activity in microglia. FEBS J. (2017) 284:4096–114. doi: 10.1111/febs.14293
202. Sun J, Soos T, Kewalramani VN, Osiecki K, Zheng JH, Falkin L, et al. CD4-specific transgenic expression of human cyclin T1 markedly increases human immunodeficiency virus type 1 (HIV-1) production by CD4+ T lymphocytes and myeloid cells in mice transgenic for a provirus encoding a monocyte-tropic HIV-1 isolate. J Virol. (2006) 80:1850–62. doi: 10.1128/JVI.80.4.1850-1862.2006
203. Seay K, Qi X, Zheng JH, Zhang C, Chen K, Dutta M, et al. Mice transgenic for CD4-specific human CD4, CCR5 and cyclin T1 expression: a new model for investigating HIV-1 transmission and treatment efficacy. PLoS ONE. (2013) 8:e63537. doi: 10.1371/journal.pone.0063537
204. Honeycutt JB, Thayer WO, Baker CE, Ribeiro RM, Lada SM, Cao Y, et al. HIV persistence in tissue macrophages of humanized myeloid-only mice during antiretroviral therapy. Nat Med. (2017) 23:638–643. doi: 10.1038/nm.4319
205. Karpel ME, Boutwell CL, Allen TM. BLT humanized mice as a small animal model of HIV infection. Curr Opin Virol. (2015) 13:75–80. doi: 10.1016/j.coviro.2015.05.002
206. Sindberg GM, Sharma U, Banerjee S, Anand V, Dutta R, Gu CJ, et al. An infectious murine model for studying the systemic effects of opioids on early HIV pathogenesis in the gut. J Neuroimmune Pharmacol. (2015) 10:74–87. doi: 10.1007/s11481-014-9574-9
207. Sagar V, Pilakka-Kanthikeel S, Atluri VS, Ding H, Arias AY, Jayant RD, et al. Therapeutical neurotargeting via magnetic nanocarrier: implications to opiate-induced neuropathogenesis and NeuroAIDS. J Biomed Nanotechnol. (2015). 11:1722–33. doi: 10.1166/jbn.2015.2108
208. Kaushik A, Yndart A, Atluri V, Tiwari S, Tomitaka A, Gupta P, et al. Magnetically guided non-invasive CRISPR-Cas9/gRNA delivery across blood-brain barrier to eradicate latent HIV-1 infection. Sci Rep. (2019) 9:3928. doi: 10.1038/s41598-019-40222-4
209. Yuan Y, Arnatt CK, El-Hage N, Dever SM, Jacob JC, Selley DE. A bivalent ligand targeting the putative mu opioid receptor and chemokine receptor CCR5 heterodimers: binding affinity versus functional activities. Medchemcomm. (2013) 4:847–51. doi: 10.1039/c3md00080j
210. Zhu M, Xu Y, Wang H, Shen Z, Xie Z, Chen F, et al. Heroin abuse results in shifted RNA expression to neurodegenerative diseases and attenuation of TNFalpha signaling pathway. Sci Rep. (2018) 8:9231. doi: 10.1038/s41598-018-27419-9
211. Avey D, Sankararaman S, Yim AKY, Barve R, Milbrandt J, Mitra RD. Single-cell RNA-seq uncovers a robust transcriptional response to morphine by glia. Cell Rep. (2018) 24:3619–3629 e4. doi: 10.1016/j.celrep.2018.08.080
212. Farhadian SF, Mehta SS, Zografou C, Robertson K, Price RW, Pappalardo J, et al. Single-cell RNA sequencing reveals microglia-like cells in cerebrospinal fluid during virologically suppressed HIV. JCI Insight. (2018) 3:121718. doi: 10.1172/jci.insight.121718
213. Bakken TE, Hodge RD, Miller JA, Yao Z, Nguyen TN, Aevermann B, et al. Single-nucleus and single-cell transcriptomes compared in matched cortical cell types. PLoS ONE. (2018) 13:e0209648. doi: 10.1371/journal.pone.0209648
214. Dever SM, Costin BN, Xu R, El-Hage N, Balinang J, Samoshkin A, et al. Differential expression of the alternatively spliced OPRM1 isoform mu-opioid receptor-1K in HIV-infected individuals. AIDS. (2014) 28:19–30. doi: 10.1097/QAD.0000000000000113
215. Regan PM, Langford D, Khalili K. Regulation and functional implications of opioid receptor splicing in opioid pharmacology and HIV pathogenesis. J Cell Physiol. (2016) 231:976–85. doi: 10.1002/jcp.25237
216. Deisseroth K, Feng G, Majewska AK, Miesenbock G, Ting A, Schnitzer MJ. Next-generation optical technologies for illuminating genetically targeted brain circuits. J Neurosci. (2006) 26:10380–6. doi: 10.1523/JNEUROSCI.3863-06.2006
217. Zhang Y, Yin C, Zhang T, Li F, Yang W, Kaminski R, et al. CRISPR/gRNA-directed synergistic activation mediator (SAM) induces specific, persistent and robust reactivation of the HIV-1 latent reservoirs. Sci Rep. (2015) 5:16277. doi: 10.1038/srep16277
218. Zhang Y, Liang Y, Levran O, Randesi M, Yuferov V, Zhao C, et al. Alterations of expression of inflammation/immune-related genes in the dorsal and ventral striatum of adult C57BL/6J mice following chronic oxycodone self-administration: a RNA sequencing study. Psychopharmacology (Berl). (2017) 234:2259–75. doi: 10.1007/s00213-017-4657-y
219. Mahajan SD, Schwartz SA, Shanahan TC, Chawda RP, Nair MP. Morphine regulates gene expression of alpha- and beta-chemokines and their receptors on astroglial cells via the opioid mu receptor. J Immunol. (2002) 169:3589–99. doi: 10.4049/jimmunol.169.7.3589
220. Steele AD, Henderson EE, Rogers TJ. Mu-opioid modulation of HIV-1 coreceptor expression and HIV-1 replication. Virology. (2003) 309:99–107. doi: 10.1016/S0042-6822(03)00015-1
Keywords: HIV-associated neurocognitive disorders, substance abuse, buprenorphine, next generation sequencing, monocytes, macrophages, central nervous system
Citation: Murphy A, Barbaro J, Martínez-Aguado P, Chilunda V, Jaureguiberry-Bravo M and Berman JW (2019) The Effects of Opioids on HIV Neuropathogenesis. Front. Immunol. 10:2445. doi: 10.3389/fimmu.2019.02445
Received: 31 May 2019; Accepted: 01 October 2019;
Published: 18 October 2019.
Edited by:
Sabita Roy, University of Miami, United StatesReviewed by:
Yolande Richard, Institut National de la Santé et de la Recherche Médicale (INSERM), FranceKatherina Psarra, Evaggelismos General Hospital, Greece
Copyright © 2019 Murphy, Barbaro, Martínez-Aguado, Chilunda, Jaureguiberry-Bravo and Berman. This is an open-access article distributed under the terms of the Creative Commons Attribution License (CC BY). The use, distribution or reproduction in other forums is permitted, provided the original author(s) and the copyright owner(s) are credited and that the original publication in this journal is cited, in accordance with accepted academic practice. No use, distribution or reproduction is permitted which does not comply with these terms.
*Correspondence: Joan W. Berman, am9hbi5iZXJtYW4mI3gwMDA0MDtlaW5zdGVpbi55dS5lZHU=
†These authors have contributed equally to this work