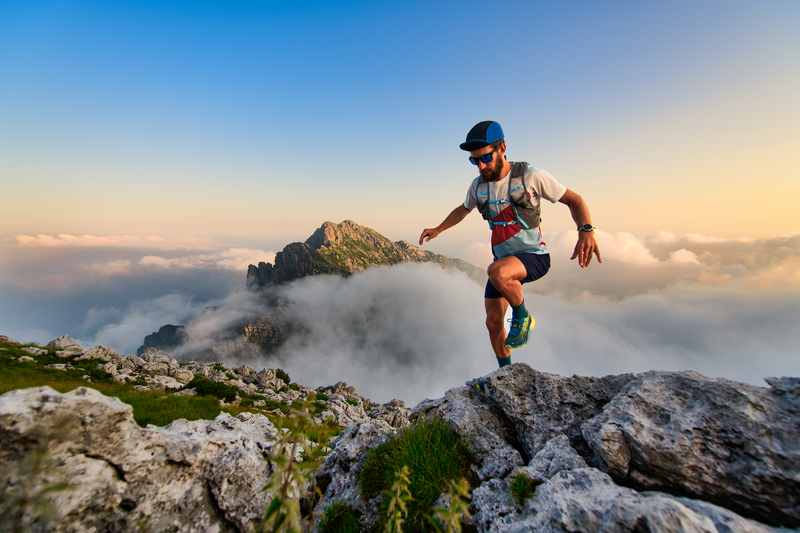
94% of researchers rate our articles as excellent or good
Learn more about the work of our research integrity team to safeguard the quality of each article we publish.
Find out more
ORIGINAL RESEARCH article
Front. Immunol. , 15 October 2019
Sec. Multiple Sclerosis and Neuroimmunology
Volume 10 - 2019 | https://doi.org/10.3389/fimmu.2019.02403
Human cytomegalovirus (HCMV) has been recently related with a lower susceptibility to multiple sclerosis (MS). HCMV promotes an adaptive development of NK cells bearing the CD94/NKG2C receptor with a characteristic phenotypic and functional profile. NK cells are proposed to play an immunoregulatory role in MS, and expansion of the NKG2C(+) subset was recently associated with reduced disability progression. To further explore this issue, additional adaptive NK cell markers, i.e., downregulation of FcεRIγ chain (FcRγ) and PLZF transcription factor, as well as antibody-dependent NK cell activation were assessed in controls and MS patients considering HCMV serology and clinical features. In line with previous reports, increased proportions of NKG2C(+), FcRγ(–), and PLZF(–) CD56dim NK cells were found in HCMV(+) cases. However, PLZF(–) NK cells were detected uncoupled from other adaptive markers within the CD56bright subset from HCMV(+) cases and among CD56dim NK cells from HCMV(–) MS patients, suggesting an additional effect of HCMV-independent factors in PLZF downregulation. Interferon-β therapy was associated with lower proportions of FcRγ(–) CD56dim NK cells in HCMV(+) and increased PLZF(–) CD56bright NK cells in HCMV(–) patients, pointing out to an influence of the cytokine on the expression of adaptive NK cell-associated markers. In addition, proportions of NKG2C(+) and FcRγ(–) NK cells differed in progressive MS patients as compared to controls and other clinical forms. Remarkably, an adaptive NK cell phenotype did not directly correlate with enhanced antibody-triggered degranulation and TNFα production in MS in contrast to controls. Altogether, our results provide novel insights into the putative influence of HCMV and adaptive NK cells in MS.
Multiple sclerosis (MS) is an immune-mediated disease of the central nervous system characterized by a highly variable clinical course. Both genetic and environmental factors are involved in MS, with an influence of herpesvirus infections supported by seroepidemiological studies. Whereas Epstein–Barr virus (EBV) is consistently associated with MS and might play a pathogenic role as an environmental trigger (1, 2), human cytomegalovirus (HCMV) was recently related to lower MS susceptibility (3–6); however, the basis for these observations remains uncertain. HCMV establishes a lifelong latent infection whose prevalence appears inversely related with socioeconomical development and, in line with the “hygiene hypothesis,” the reported associations with MS might be indirectly linked to the exposure to other environmental factors. On the other hand, a putative influence of HCMV in MS immunopathology is conceivable (7), as this herpesvirus may exert a profound impact on the immune system (8), and could potentially modulate the response to other pathogens (i.e., heterologous immunity) (9, 10). Nevertheless, whether HCMV may exert a beneficial or detrimental effect on MS is currently uncertain (11).
T lymphocytes and natural killer (NK) cells play complementary key roles in controlling HCMV, which, reciprocally, has developed a variety of immune evasion mechanisms (12). HCMV has been reported to induce a persistent reconfiguration of NK cell compartment, both in healthy individuals and under pathological conditions. The magnitude of this effect is variable and hallmarked by the differentiation and expansion of functionally mature NK cells displaying high levels of the CD94/NKG2C activating receptor encompassed by additional phenotypic and functional changes (13–16). Human adaptive NKG2C(+) NK cells are contained within the major circulating CD56dim subset, which mediates cytotoxicity and cytokine production upon interaction with target cells, either directly or through IgG engagement of FcγRIIIA (CD16A). As compared to other subsets, adaptive NKG2C(+) NK cells effectively mediate antibody-dependent effector functions, particularly cytokine production (17, 18). Additional associated-adaptive NK cell markers include epigenetic silencing of the promyelocytic leukemia zinc finger (PLZF) transcription factor, with unknown functional implications in NK cells (17, 19–21), and loss of the FcεRI gamma chain (FcRγ) adaptor coupled to CD16, whose replacement by the ζ chain may enhance antibody-dependent NK cell activation (17, 19, 22, 23). Some adaptive NK cell-associated features have also been detected in NKG2C(–) NK cell subsets, suggesting that NK cell differentiation is independent of NKG2C expression and may be induced by stimuli other than HCMV (17, 18, 24). Moreover, adaptive NK cell differentiation may not be a coordinated process, as supported by the delayed FcRγ loss observed in adaptive NK cells following HCMV infection in hematopoietic stem cell transplantation recipients (25). The cellular and molecular mechanisms underlying the development of adaptive NK cells and their implications in human health remain partially defined.
NK cells have been previously studied in the context of MS (26, 27), and changes associated with the response to some immunomodulatory therapies have been reported (28, 29), proposing an immunoregulatory role played by the minor circulating CD56bright NK cell subset (30). These cells display a CD16(–) NKG2A(+) phenotype and have a limited cytotoxic capacity but efficiently produce cytokines, constituting the predominant subset in peripheral lymphoid tissues. However, the role of NK cells in MS immunology is only partially understood. Recently, we described an association of HCMV-induced expansion of NKG2C(+) NK cells with a lower risk of disability progression in MS, suggesting an influence of these lymphocytes on the clinical course (31). In the present study, we aimed to further explore whether HCMV and adaptive NK cells play a protective role in MS. Adaptive NK cell immunophenotype and antibody-triggered NK cell activation against EBV-transformed B cell lines were evaluated in MS patients considering their HCMV serostatus and clinical profile, providing further insights into the involvement of NK cells in this disease.
A case–control study of MS patients was designed to analyze adaptive NK cell markers and antibody-dependent NK cell activation in accordance to HCMV serostatus and clinical characteristics. MS patients diagnosed based on McDonald criteria 2010 from Hospital del Mar, IMIM (Barcelona) and Hospital Ramón y Cajal (Madrid) (REEM, Spanish Network for MS research) were recruited from routine clinical visits. In addition, a subgroup of MS patients was prospectively evaluated after onset of interferon-beta (IFNβ) therapy to assess the influence of this treatment on adaptive NK cells. We excluded patients on disease-modifying therapies known to deplete peripheral lymphocytes or alter their trafficking (e.g., natalizumab, fingolimod, dimethyl fumarate), as well as those undergoing corticosteroid treatment in the last 30 days, pregnancy, or any severe concomitant disease. The following demographic and clinical characteristics were evaluated in every case: age, sex, disease duration, MS form (RRMS: relapsing–remitting MS, SPMS: secondary progressive MS, PPMS: primary progressive MS), disability scores (EDSS: Expanded Disability Status Scale, MSSS: multiple sclerosis severity score) and treatment. Healthy controls matched for age and sex were recruited from the same geographical area.
The study was approved by the local Ethics Committee, including participants after written informed consent. Blood samples obtained from venous puncture were obtained from controls and MS patients, performing standard clinical diagnostics test to evaluate EBV-(LIASON®) and HCMV-specific circulating antibodies (Roche®, Cobas602).
Peripheral blood mononuclear cells (PBMCs) from 47 healthy controls and 151 MS patients were isolated from blood samples collected in EDTA tubes using Ficoll–Hypaque density gradient centrifugation and cryopreserved in fetal calf serum with 10% dimethyl sulfoxide until analysis. Sample staining for flow cytometry analysis was performed using anti-NKG2A (clone Z199) indirectly labeled with a secondary goat anti-mouse PE-Cy7 (Biolegend) and the following fluorochrome-conjugated antibodies: anti-CD3-PerCP (BD Pharmigen), anti-CD56-APC (Biolegend), antiCD16-eFluor450 (eBioscience), and NKG2C-PE (R&D Systems). For the analysis of FcRγ (MS, n = 139; controls n = 47) and PLZF expression (MS, n = 86; controls n = 26), cells were treated with a fixation/permeabilization kit (BD Biosciences) followed by incubation with anti-FcRγ-FITC (Millipore) and anti-PLZF-PE CF594 (BD Biosciences). Samples were acquired in LSRFortessa (BD Biosciences) and data were analyzed using FlowJo software (Tree Star, Oregon, USA), using the gating strategy shown in Figure 1.
Figure 1. Gating strategy for adaptive NK cells. Lymphocytes were identified based on their forward scatter (FCS) and side scatter (SSC) characteristics, defining NK cells as CD3(–) CD56(+) lymphocytes. Representative examples were selected based on the expression of adaptive NK cell markers, showing a case with a low expression of the three adaptive markers (MS.01), a case with low NKG2C(+), FcRγ(–), and PLZF(–) expression in CD56dim NK cells but with a higher proportions of PLZF(–) CD56bright NK cells (HC.01), and a case with higher proportions of NKG2C(+), FcRγ(–), and PLZF(–) CD56dim NK cells.
PBMCs from 42 MS patients (22 RRMS, 8 SPMS and 12 PPMS) and 17 controls matched for HCMV serostatus were incubated overnight at 37°C with recombinant IL-2 (200 U/ml). The response of NK cells to the HLA class I-defective 721.221 B-lymphoblastoid cell line with or without rituximab (50 ng/ml) was assessed following a 4-h incubation (E/T = 1/1). A complementary approach was performed using EBV(+) AKBM cells as targets following induction of the lytic cycle in the presence of EBV(+) or EBV(–) sera, as previously described (32, 33). Surface expression of CD107 as a marker of degranulation and intracellular TNFα production was analyzed by flow cytometry as previously reported (34), using the anti-CD107-APC (BD Pharmigen) monoclonal antibody during incubation together with monensin (GolgiStop® BD) and brefeldin (GolgiPlug® BD). Cultures were then stained with anti-CD3-PerCP (BD Pharmigen), anti-CD56-APC-Cy7 (Biolegend), and anti-NKG2C-PE (R&D System), permeabilized, fixed and stained intracellularly with anti-TNFα-CFBlue (labeled by Immunostep), anti-FcRγ-FITC (Millipore), and anti-PLZF-PE CF594 (BD Biosciences). Data acquisition was performed with an LSRFortessa cytometer (BD Biosciences).
A multidimensional flow cytometry analysis was performed as previously described (35), compensating raw flow cytometry data using FlowJo software (Tree Star, Oregon, USA) and later imported into R using flowCore and openCyto packages. Lymphocytes were gated on forward scatter and side scatter characteristics and then on CD56dim NK cells. FITC channel was normalized using flowStats R package in order to reduce experimental variability on fluorescence intensity. Subsequently, randomly selected data from 500 CD56dim NK cells per sample was concatenated. The most positive and negative one per mille values for each parameter were reduced to their less extreme border. Next, Barnes-Hut t-SNE was conducted using the Rtsne package. Graphics were produced using ggplot2 and RcolorBrewer R packages.
Normal distribution was assessed using Kolmogorov–Smirnov test. Continuous variables were expressed as mean ± standard deviation (SD) or median (first–third quartile) for parametric and non-parametric variables, respectively. Relationship between continuous and dichotomous variables was assessed by Student's t-test or Mann–Whitney U-test, respectively. Pearson or Spearman correlation coefficients were calculated for pairwise comparison of continuous variables. Wilcoxon test for paired samples was used to compare matched prospectively followed-up samples before and after IFNβ therapy. Three-dimensional Venn analysis evaluated the co-expression of adaptive markers in NK cells. Multivariate lineal regression analysis determined predictors for adaptive NK cell markers adjusting the models for HCMV serostatus, clinical variables, and IFNβ treatment. Results were considered significant at the two-sided level of 0.05. Analyses were performed using SPSS v.23 software.
We comparatively studied in controls and MS patients the influence of HCMV on the expression of adaptive NK cell differentiation markers (NKG2C, FcRγ, and PLZF) based on the gating strategy for flow cytometry analysis illustrated in Figure 1. Demographic and clinical characteristics of MS patients and controls are shown in Table 1. In our study, HCMV seroprevalence was comparable in both groups (Table 1), and no clinical differences were observed in MS patients associated with HCMV serostatus (data not shown).
HCMV seropositivity was associated with greater proportions of NKG2C(+) NK cells, with no significant differences between MS patients and controls (Figure 2A). The expansion of NKG2C(+) cells was confined to the CD56dim NK cell subset, whereas detection of CD56bright NKG2C(+) NK cells appeared unrelated to HCMV serology (Figure 2A). Similarly, the frequency of CD56dim FcRγ(–) NK cells was also increased in HCMV(+) patients and controls as compared to HCMV(–) individuals (Figure 2B). By contrast, HCMV(+) controls and MS patients showed increased proportions of PLZF(–) cells not only in CD56dim NK cells but, remarkably, also among the CD56bright subset (Figure 2C); these PLZF(–) NK cells were confirmed to display the characteristic CD56bright NKG2A(+) CD16(–) phenotype (Supplementary Figure 1). Of note, an increased frequency of PLZF(–) cells was also detected among CD56dim NK cells from HCMV(–) MS patients as compared to seronegative controls (Figure 2C). Overall, these results support previous studies describing the HCMV-related expansion of adaptive NK cells displaying the NKG2C(+), FcRγ(–), and PLZF(–) markers in CD56dim NK cells; however, a lack of PLZF expression associated to HCMV seropositivity was also perceived in the CD56bright NK cell subset, in both controls and MS patients, in addition to an influence of HCMV-independent factors in PLZF downregulation in CD56dim NK cells found in MS cases.
Figure 2. Expression of adaptive markers in NK cells from controls and MS patients according to HCMV serostatus. (A) Proportions of NKG2C(+), (B) FcRγ(–), and (C) PLZF(–) in CD56dim and CD56bright NK cells from healthy controls (HC) and MS patients according to HCMV serostatus. Higher proportions of NKG2C(+), FcRγ(–), and PLZF(–) in CD56dim NK cells were found in HCMV(+) as compared to HCMV(–) cases (A–C), in addition to higher proportion of PLZF(–) NK cells perceived in the CD56bright subset (C). Increased proportions of PLZF(–) CD56dim NK cells were also found in HCMV(–) MS patients as compared to HCMV(–) controls (C). NKG2C, MS = 151, HC 139; FcRγ, MS = 139, HC = 47; PLZF, MS = 86, PLZF = 26. Individuals with an NKG2C del/del genotype (HC, n = 1; MS, n = 6) were excluded from the analysis of NKG2C expression. P values (Mann–Whitney U test): * <0.05, ** <0.01, *** <0.001, **** <0.0001.
We next evaluated the relation of adaptive NK cell markers in CD56dim NK cells from controls and MS patients. Three-dimensional Venn diagrams illustrated that NK cells co-expressing adaptive NK cell markers were increased in HCMV(+) compared to HCMV(–) MS patients (Figures 3A,B). The presence in MS patients of increased proportions of PLZF(–) CD56dim NK cells without other adaptive markers was also confirmed (Figure 3A).
Figure 3. Interrelationship of adaptive NK cell markers in MS. Venn diagrams depicting the proportions of NKG2C(+), FcRγ(–), and PLZF(–) CD56dim NK cells in healthy controls (HC) (n = 26) and MS patients (n = 86) (A), and stratifying results according to HCMV serostatus (B). Values expressed the mean proportion for each group. P-values (Mann–Whitney U-test): ** <0.01, comparing controls vs. MS patients; ## <0.01, comparing HCMV(–) vs. HCMV(+). (C) t-SNE plots of NKG2C, NKG2A, FcRγ, and PLZF expression in CD56dim NK cells evaluated in HC and MS patients according to HCMV serostatus.
t-SNE multidimensional analysis of adaptive markers in CD56dim NK cells (Figure 3C) revealed an HCMV-related expression of NKG2C, more pronounced in controls than in MS patients. By contrast, NKG2C(–) FcRγ(–) PLZF(–) NK cells were more evident in MS patients than in controls, with a less marked relation to HCMV serology (Figure 3C). These results further point out an uncoupled expression of adaptive markers in NK cells from MS patients as compared to controls, suggesting the influence of factors other than HCMV.
We next evaluated the impact of IFNβ therapy on adaptive NK cell markers in RRMS (mean time of treatment, 7.0 ± 4.2 years). As previously reported (36), IFNβ-treated patients displayed increased proportions of CD56bright NK cells as compared to untreated cases (14.8% ± 11.6 vs. 8.3% ± 7.1, p < 0.05), which appeared unrelated to any of the adaptive markers analyzed (data not shown). IFNβ therapy did not alter the effect of HCMV on the proportions of NKG2C(+) CD56dim NK cells (Figure 4A). However, lower proportions of FcRγ(–) CD56dim NK cells were found in IFNβ-treated patients as compared to untreated cases (9.6% ± 8.2 vs. 19.3% ± 19.7, p < 0.05), a finding only perceived in CD56dim NK cells from HCMV(+) but not in HCMV(–) MS cases, who displayed low proportions of FcRγ(–) NK cells independently of treatment (Figure 4B). Interestingly, PLZF(–) CD56bright NK cells tended to increase in IFNβ-treated HCMV(–) patients (Figure 4C), vanishing the differences related to HCMV serostatus noticed in both CD56dim and CD56bright NK cell subsets (Figure 2C). These results suggest that HCMV-driven adaptive NK cell development in RRMS might be modulated by IFNβ therapy. However, the above-mentioned IFNβ-related modifications in adaptive markers were not perceived in a subgroup of MS patients prospectively followed after 1 year of treatment (Supplementary Figure 2), indicating that the cytokine does not directly modify the adaptive NK cell immunophenotype.
Figure 4. Influence of IFNβ therapy in the expression of adaptive markers in NK cells from RRMS patients. Proportions of NKG2C(+) (A), FcRγ(–) (B), and PLZF(–) (C) in CD56dim and CD56bright NK cells according to the presence of IFNβ therapy and HCMV serostatus. The influence of IFNβ was mainly perceived in the expression of FcRγ in CD56dim NK cells in HCMV(+) cases, and in PLZF expression, which vanished differences related to HCMV serostatus in both PLZF(–) CD56dim and CD56bright NK cells. NKG2C(+): IFNβ-treated, n = 33, and untreated patients, n = 44; FcRγ: IFNβ-treated patients, n = 37, untreated patients, n = 33; PLZF, IFNβ-treated patients, n = 20, untreated patients, n = 24. Individuals with an NKG2C del/del genotype (RRMS, n = 5) were excluded from the analysis of NKG2C expression. P values (Mann–Whitney U-test): * <0.05, ** <0.01.
Considering MS form, NKG2C(+) CD56dim NK cell proportions were lower in PPMS patients as compared to SPMS patients (Supplementary Figure 3A). By contrast, proportions of FcRγ(–) NK cells appeared greater in SPMS patients as compared to RRMS, in both CD56dim and CD56bright NK cells (Supplementary Figure 3B). No differences were observed for PLZF expression according to MS form (Supplementary Figure 3C). After multivariate regression analysis, the association of MS form with adaptive markers appeared independent of HCMV and IFNβ-therapy only for the proportions of NKG2C(+) CD56dim and FcRγ(–) CD56bright NK cells (Supplementary Table 1). No additional correlations were observed between adaptive NK cell markers and age, disease duration, disability scores, or disease activity (data not shown). These results suggest that the expression of some adaptive NK cell markers may vary in progressive MS forms independently of the influence of HCMV and IFNβ therapy.
In order to evaluate antibody-dependent NK cell functions, degranulation and TNFα production were measured in NK cells from 42 MS patients and 17 controls following stimulation with the 721.221 cell line in the absence or presence of rituximab (Figure 5A). As expected, CD56dim NK cells in controls and MS patients showed a greater degranulation and TNFα production in the presence of rituximab (Figure 5B). In addition, an increased degranulation of CD56bright NK cells in the direct response to the 721.221 cell line without rituximab was perceived in MS patients (Figure 5B). Comparable results were observed in experiments using the EBV-infected AKBM cell line as target (Supplementary Figure 4A).
Figure 5. Antibody-dependent NK cell activation in MS patients and controls. (A) Experimental design evaluating NK cell degranulation (CD107) and intracellular TNFα production in functional experiments using 721.221 lymphoblastoid cell lines as NK cell targets. (B) CD107 expression and TNFα production by CD56dim and CD56bright NK cells in controls and MS patients. (C) CD107 expression and TNFα production by CD56dim NK cells in controls and MS patients according to clinical form. PBMC: peripheral blood mononuclear cells. Controls, n = 17; MS patients, n = 42 (RRMS, 22; SPMS, 8, PPMS 12). P-values (Mann–Whitney U-test for CD107 and Student's t-test for TNFα expression): * <0.05, ** <0.01, *** <0.001, **** <0.0001.
We subsequently evaluated antibody-dependent NK cell functions according to MS form (Figure 5C). RRMS patients displayed increased proportions of degranulating CD56dim NK cells as compared with controls and PPMS; moreover, the proportions of TNFα-secreting cells were lower in PPMS as compared to controls and other MS forms (Figure 5C). Similar results for TNFα production were obtained using the AKBM cell line (Supplementary Figure 4B). IFNβ therapy in MS patients did not influence the results obtained in these experiments (data not shown).
Next, we evaluated the relationship of adaptive markers in CD56dim NK cells and antibody-triggered responses. In controls, the proportions of NKG2C(+), FcRγ(–), and PLZF(–) CD56dim NK cells appeared unrelated to degranulation, whereas a direct correlation with TNFα production was observed (Figure 6). By contrast, the proportions of adaptive NK cells in MS patients did not correlate with TNFα secretion and, moreover, an inverse relation between degranulation and the proportions of FcRγ(–) and PLZF(–) CD56dim NK cells was detected (Figure 6); similar observations were found in CD56bright NK cells from MS patients (data not shown). An analysis considering the co-expression of adaptive markers in controls and MS patients showed that antibody-triggered responses were greater in NKG2C(+) CD56dim NK cells independently of FcRγ or PLZF expression (Supplementary Figure 5).
Figure 6. Correlations between adaptive NK markers and antibody-dependent CD56dim NK cell activation. Spearman rank correlations (R) between proportions of NKG2C(+), FcRγ(–), and PLZF(–) CD56dim NK cells and intracellular TNFα production (A), and CD107 expression (B) in functional experiments using rituximab and 721.221 lymphoblastoid cell lines as NK cell targets. HC, healthy controls; MS, multiple sclerosis. Controls, n = 17; MS patients, n = 42 (RRMS, 22; SPMS, 8, PPMS 12).
Overall, these results suggest that adaptive NK cell function may differ in MS according to clinical form, being greater in RRMS as compared to progressive MS forms; however, in contrast to healthy individuals, an adaptive NK cell profile in MS patients did not correlate with proficient antibody-triggered effector functions.
Considering the high prevalence of lifelong HCMV infection and its broad impact on the immune system (8), the possibility that this herpesvirus might somehow impact the development of autoimmune disorders is plausible (37). A lower HCMV seroprevalence has been reported in studies of pediatric and adult MS populations (3–7, 11), suggesting that this infection might have a protective effect. Among different hypotheses to explain how HCMV might influence MS, we considered the involvement of NK cells, which have been previously related with the disease (26–30). The virus promotes to a variable extent an adaptive differentiation of NK cells (13–15, 17, 19), which effectively mediate antibody-triggered cytotoxicity and cytokine production, thus potentially contributing to the immune response against HCMV and other microbial pathogens (10, 38–40). In line with reports relating HCMV with a reduced MS susceptibility (3–5), an association of HCMV-driven adaptive NKG2C(+) NK cell expansion with a lower risk of clinical progression was previously described (31). Yet, it remains uncertain whether and how adaptive NK cells might influence the development and clinical course of MS.
In agreement with extensive observations in healthy blood donors and different clinical settings, our results pointed out that HCMV infection is the leading factor that promotes the development of conventional adaptive NKG2C(+), FcRγ(–), and PLZF(–) CD56dim NK cells in MS patients (17–19, 22, 23). In previous reports, an uncoupled distribution of the expression of some adaptive NK cell-associated markers, with potential functional implications, was noticed (17, 18, 25). In this regard, we observed increased proportions of PLZF(–) NK cells, but not of NKG2C(+) or FcRγ(–) NK cells, among the CD56bright NK cell subset from HCMV(+) individuals. Such phenotype may result from an influence of HCMV at early stages of adaptive NK cell development, according to the linear model of differentiation from CD56bright NK cells (41), but also of HCMV-unrelated factors (20, 42), as suggested by the detection of increased PLZF(–) CD56dim NK cells in HCMV(–) MS patients.
No significant differences in NKG2C expression were perceived between controls and progressive forms; yet, lower proportions of NKG2C(+) NK cells in PPMS as compared to SPMS were detected, in line with the lower NKG2C pattern of expression in MS patients observed in the multidimensional analysis as well as in previous studies with a larger number of cases (31). An additional factor related with changes in the expression of adaptive NK cell markers in MS was IFNβ therapy, which was associated with reduced proportions of CD56dim FcRγ(–) NK cells in HCMV(+) RRMS patients. Moreover, HCMV-related differences in the proportions of PLZF(–) CD56dim and CD56bright NK cell subsets vanished in IFNβ-treated cases, an effect related with an increase of PLZF(–) cells in HCMV(–) patients. However, no phenotypic changes in adaptive NK cells were perceived in a subset of RRMS cases prospectively followed up for 1 year after initiating IFNβ therapy, pointing out that this effect likely results from complex mechanisms eventually requiring a longer exposure to be perceived. Further studies would be required to assess whether PLZF downregulation might be related to clinical response to IFNβ therapy.
Adaptive NK cells have been reported to effectively mediate antibody-triggered effector functions, particularly cytokine production (22, 23, 34). NK cell activation upon incubation with rituximab-coated 721.221 cells was mainly exerted by CD56dim NK cells, without significant differences between controls and MS patients, as previously reported (43). Other clinical variables (i.e., age and IFNβ-therapy) appeared as well unrelated with antibody-triggered responses mediated by CD56dim NK cells (data not shown). Notwithstanding, NK cell activation differed in MS according to the clinical form, being lower in PPMS as compared to RRMS patients. Similar results were obtained using as a target the AKBM cell line, suggesting a comparable pattern of NK cell response against EBV-infected targets. In accordance to previous studies addressing the relation of adaptive markers with NK cell antibody-triggered function (18, 25, 44), a proficient antibody-dependent activity was related to NKG2C expression in CD56dim NK cells rather than to other adaptive-associated markers. This finding, in conjunction with the low NKG2C expression found in progressive MS (31), deserves attention, suggesting that an adaptive NK cell dysfunction may be associated with a risk of disability progression.
Based on our observations, some considerations need to be made regarding the putative connection of HCMV infection and adaptive NK cells with MS immunopathology. First, the ability of NK cells to eliminate activated T lymphocytes suggested that they may play a relevant immunoregulatory role in the context of MS (27, 28, 30). Yet, adaptive NK cells do not effectively exert this function (17), possibly reflecting their expression of KIRs and ILT2 HLA class I-specific inhibitory receptors. Second, given their CD16-triggered antibody-dependent effector functions and proliferation, adaptive NK cells likely participate in the immune response to different microbial pathogens (17, 19, 22, 40). Thus, HCMV-induced adaptive NK cells might contribute to the control of other herpesviruses proposed to be involved in MS, as supported by their participation in the marked antibody-dependent response against EBV-infected cells (33). In this regard, the adaptive NK cell phenotype in MS patients was not associated with proficient antibody-dependent effector functions, in contrast to the pattern of response observed in healthy donors. Third, PLZF(–) CD56bright NK cells were detected in HCMV(+) controls and MS patients and, moreover, IFNβ therapy was associated with increased PLZF(–) CD56bright NK cells in HCMV(–) RRMS. This finding deserves further attention in the context of the regulatory role of CD56bright NK cells and their contribution to control EBV infection (45, 46).
In conclusion, our results provide novel insights into the putative influence of HCMV in MS involving the NK cell compartment. The study shows that the distribution of adaptive NK cell markers in MS may vary not only depending on HCMV seropositivity but also on IFNβ therapy and disease form, revealing differences in their antibody-dependent activation as compared to healthy individuals. Further studies are required to explore the involvement of HCMV in the immune response to EBV in MS patients, and the putative influence of HCMV-unrelated factors in PLZF downregulation.
The datasets generated for this study will not be made publicly available. The work is based on flow cytometry analysis of peripheral blood mononuclear cells. Data will be available under request.
The studies involving human participants were reviewed and approved by Comité Etic d'Investigacions Cliniques (CEIC), Hospital del Mar Medical Research Institute. The patients/participants provided their written informed consent to participate in this study.
AM, EA-P, AV, and JM-R designed the study, executed experiments, and performed the statistical analysis. AM, EA-P, ML-B, and JM-R contributed to interpretation of the results and wrote the final draft that was revised for all authors. AZ, EM, and ML contributed to data interpretation and critically reviewed the manuscript. MC-G performed and contributed to the interpretation of the t-SNE analysis. NV, LV, and RÁ-L provided samples, performed serological analysis, and critically reviewed the manuscript.
This work was supported by grants FIS/PI17/00254, SAF 2016-80363-C2-1-R (Spanish Ministry of Economy and Competitiveness and FEDER), the EU FP7-MINECO Infect-ERA Program (PCIN-2015-191-C02-01), and Red Española de Esclerosis Múltiple (REEM) from the Instituto de Salud Carlos III, the European Regional Development Fund (Grant RD16/0015/0011), and the Spanish Ministry of Economy and Competitiveness.
AM has received travel funding from Teva, Biogen Idec, Novartis, Almirall, Bayer, and Genzyme. EM has received personal fees for consulting services and lectures from Merck-Serono, Biogen Idec, Teva, Genzyme, Novartis, Bayer, and Almirall. AZ has received travel funding from Biogen Idec, Novartis, and Genzyme. RÁ-L has received honoraria for lectures from Merck-Serono, Novartis, and Biogen Idec, and grants from Merck-Serono, Teva, Sanofi-Avertis, Biogen Idec, and Bayer. LV reports personal fees for consulting services from Biogen Idec, and grants from Merck-Serono, Biogen Idec, Teva, and Genzyme. JM-R has participated as principal investigator in pharmaceutical company-sponsored clinical trials including Novartis, Roche, Merck-Serono, Actelion, and Celgene, and personal fees for consulting services and lectures from Novartis, Biogen Idec and Merck-Serono.
The remaining authors declare that the research was conducted in the absence of any commercial or financial relationships that could be construed as a potential conflict of interest.
The Supplementary Material for this article can be found online at: https://www.frontiersin.org/articles/10.3389/fimmu.2019.02403/full#supplementary-material
1. Ascherio A, Munger KL, Lennette ET, Spiegelman D, Hernán MA, Olek MJ, et al. Epstein-Barr virus antibodies and risk of multiple sclerosis: a prospective study. JAMA. (2001) 286:3083–8. doi: 10.1001/jama.286.24.3083
2. Jilek S, Schluep M, Meylan P, Vingerhoets F, Guignard L, Monney A, et al. Strong EBV-specific CD8+ T-cell response in patients with early multiple sclerosis. Brain. (2008) 131:1712–21. doi: 10.1093/brain/awn108
3. Waubant E, Mowry EM, Krupp L, Chitnis T, Yeh EA, Kuntz N, et al. Common viruses associated with lower pediatric multiple sclerosis risk. Neurology. (2011) 76:1989–95. doi: 10.1212/WNL.0b013e31821e552a
4. Sundqvist E, Bergström T, Daialhosein H, Nyström M, Sundström P, Hillert J, et al. Cytomegalovirus seropositivity is negatively associated with multiple sclerosis. Mult Scler J. (2014) 20:165–73. doi: 10.1177/1352458513494489
5. Makhani N, Banwell B, Tellier R, Yea C, McGovern S, O'Mahony J, et al. Viral exposures and MS outcome in a prospective cohort of children with acquired demyelination. Mult Scler J. (2016) 22:385–8. doi: 10.1177/1352458515595876
6. Langer-Gould A, Wu J, Lucas R, Smith J, Gonzales E, Amezcua L, et al. Epstein-Barr virus, cytomegalovirus, and multiple sclerosis susceptibility: a multiethnic study. Neurology. (2017) 89:1330–7. doi: 10.1212/WNL.0000000000004412
7. Alari-Pahissa E, Moreira A, Zabalza A, Alvarez-Lafuente R, Munteis E, Vera A, et al. Low cytomegalovirus seroprevalence in early multiple sclerosis: a case for the ‘hygiene hypothesis'? Eur J Neurol. (2018) 25:925–33. doi: 10.1111/ene.13622
8. Brodin P, Jojic V, Gao T, Bhattacharya S, Angel CJL, Furman D, et al. Variation in the human immune system is largely driven by non-heritable influences. Cell. (2015) 160:37–47. doi: 10.1016/j.cell.2014.12.020
9. White DW, Suzanne Beard R, Barton ES. Immune modulation during latent herpesvirus infection. Immunol Rev. (2012) 245:189–208. doi: 10.1111/j.1600-065X.2011.01074.x
10. Smithey MJ, Venturi V, Davenport MP, Buntzman AS, Vincent BG, Frelinger JA, et al. Lifelong CMV infection improves immune defense in old mice by broadening the mobilized TCR repertoire against third-party infection. Proc Natl Acad Sci USA. (2018) 115:E6817–25. doi: 10.1073/pnas.1719451115
11. Vanheusden M, Stinissen P, 't Hart BA, Hellings N. Cytomegalovirus: a culprit or protector in multiple sclerosis? Trends Mol Med. (2015) 21:16–23. doi: 10.1016/j.molmed.2014.11.002
12. Vieira Braga FA, Hertoghs KML, van Lier RAW, van Gisbergen KPJM. Molecular characterization of HCMV-specific immune responses: parallels between CD8+ T cells, CD4+ T cells, and NK cells. Eur J Immunol. (2015) 45:2433–45. doi: 10.1002/eji.201545495
13. Gumá M, Angulo A, Vilches C, Gómez-Lozano N, Malats N, López-Botet M. Imprint of human cytomegalovirus infection on the NK cell receptor repertoire. Blood. (2004) 104:3664–71. doi: 10.1182/blood-2004-05-2058
14. Lopez-Verges S, Milush JM, Schwartz BS, Pando MJ, Jarjoura J, York VA, et al. Expansion of a unique CD57+NKG2Chi natural killer cell subset during acute human cytomegalovirus infection. Proc Natl Acad Sci USA. (2011) 108:14725–32. doi: 10.1073/pnas.1110900108
15. Foley B, Cooley S, Verneris MR, Pitt M, Curtsinger J, Luo X, et al. Cytomegalovirus reactivation after allogeneic transplantation promotes a lasting increase in educated NKG2C+ natural killer cells with potent function. Blood. (2012) 119:2665–74. doi: 10.1182/blood-2011-10-386995
16. López-Botet M, Muntasell A, Vilches C. The CD94/NKG2C+ NK-cell subset on the edge of innate and adaptive immunity to human cytomegalovirus infection. Semin Immunol. (2014) 26:145–51. doi: 10.1016/j.smim.2014.03.002
17. Schlums H, Cichocki F, Tesi B, Theorell J, Beziat V, Holmes TD, et al. Cytomegalovirus infection drives adaptive epigenetic diversification of NK cells with altered signaling and effector function. Immunity. (2015) 42:443–56. doi: 10.1016/j.immuni.2015.02.008
18. Muntasell A, Pupuleku A, Cisneros E, Vera A, Moraru M, Vilches C, et al. Relationship of NKG2C copy number with the distribution of distinct cytomegalovirus-induced adaptive NK cell subsets. J Immunol. (2016) 196:3818–27. doi: 10.4049/jimmunol.1502438
19. Lee J, Zhang T, Hwang I, Kim A, Nitschke L, Kim M, et al. Epigenetic modification and antibody-dependent expansion of memory-like NK cells in human cytomegalovirus-infected individuals. Immunity. (2015) 42:431–42. doi: 10.1016/j.immuni.2015.02.013
20. Schlums H, Jung M, Han H, Theorell J, Bigley V, Chiang SCC, et al. Adaptive NK cells can persist in patients with GATA2 mutation depleted of stem and progenitor cells. Blood. (2017) 129:1927–39. doi: 10.1182/blood-2016-08-734236
21. Peppa D, Pedroza-Pacheco I, Pellegrino P, Williams I, Maini MK, Borrow P. Adaptive reconfiguration of natural killer cells in HIV-1 infection. Front Immunol. (2018) 9:474. doi: 10.3389/fimmu.2018.00474
22. Hwang I, Zhang T, Scott JM, Kim AR, Lee T, Kakarla T, et al. Identification of human NK cells that are deficient for signaling adaptor FcRγ and specialized for antibody-dependent immune functions. Int Immunol. (2012) 24:793–802. doi: 10.1093/intimm/dxs080
23. Zhang T, Scott JM, Hwang I, Kim S. Cutting edge: antibody-dependent memory-like NK cells distinguished by FcR deficiency. J Immunol. (2013) 190:1402–6. doi: 10.4049/jimmunol.1203034
24. Della Chiesa M, Falco M, Bertaina A, Muccio L, Alicata C, Frassoni F, et al. Human cytomegalovirus infection promotes rapid maturation of NK cells expressing activating killer Ig-like receptor in patients transplanted with NKG2C-/- umbilical cord blood. J Immunol. (2014) 192:1471–9. doi: 10.4049/jimmunol.1302053
25. Muccio L, Falco M, Bertaina A, Locatelli F, Frassoni F, Sivori S, et al. Late development of FcεRγneg adaptive natural killer cells upon human cytomegalovirus reactivation in umbilical cord blood transplantation recipients. Front Immunol. (2018) 9:1050. doi: 10.3389/fimmu.2018.01050
26. De Jager PL, Rossin E, Pyne S, Tamayo P, Ottoboni L, Viglietta V, et al. Cytometric profiling in multiple sclerosis uncovers patient population structure and a reduction of CD8low cells. Brain. (2008) 131:1701–11. doi: 10.1093/brain/awn118
27. Gross CC, Schulte-Mecklenbeck A, Wiendl H, Marcenaro E, Kerlero de Rosbo N, Uccelli A, et al. Regulatory functions of natural killer cells in multiple sclerosis. Front Immunol. (2016) 7:606. doi: 10.3389/fimmu.2016.00606
28. Bielekova B, Catalfamo M, Reichert-Scrivner S, Packer A, Cerna M, Waldmann TA, et al. Regulatory CD56bright natural killer cells mediate immunomodulatory effects of IL-2R -targeted therapy (daclizumab) in multiple sclerosis. Proc Natl Acad Sci USA.(2006) 103:5941–46. doi: 10.1073/pnas.0601335103
29. Martínez-Rodríguez JE, López-Botet M, Munteis E, Rio J, Roquer J, Montalban X, Comabella M. Natural killer cell phenotype and clinical response to interferon-beta therapy in multiple sclerosis. Clin Immunol. (2011) 141:348–356. doi: 10.1016/j.clim.2011.09.006
30. Laroni A, Armentani E, Kerlero de Rosbo N, Ivaldi F, Marcenaro E, Sivori S, Gandhi R, Weiner HL, Moretta A, Mancardi GL, et al. Dysregulation of regulatory CD56 bright NK cells/T cells interactions in multiple sclerosis. J Autoimmun. (2016) 72:8–18. doi: 10.1016/j.jaut.2016.04.003
31. Martínez-Rodríguez JE, Cobo-Calvo A, Villar LM, Munteis E, Blanco Y, Rasal R, et al. Adaptive natural killer cell response to cytomegalovirus and disability progression in multiple sclerosis. Mult Scler. (2016) 31:741–52. doi: 10.1177/1352458515601215
32. Ressing ME, Keating SE, van Leeuwen D, Koppers-Lalic D, Pappworth IY, Wiertz EJHJ, et al. Impaired transporter associated with antigen processing-dependent peptide transport during productive EBV infection. J Immunol. (2005) 174:6829–38. doi: 10.4049/jimmunol.174.11.6829
33. López-Montañés M, Alari-Pahissa E, Sintes J, Martínez-Rodríguez JE, Muntasell A, López-Botet M. Antibody-dependent NK cell activation differentially targets EBV-infected cells in lytic cycle and bystander B lymphocytes bound to viral antigen–containing particles. J Immunol. (2017) 199:656–65. doi: 10.4049/jimmunol.1601574
34. Costa-Garcia M, Vera A, Moraru M, Vilches C, López-Botet M, Muntasell A. Antibody-mediated response of NKG2C bright NK cells against human cytomegalovirus. J Immunol. (2015) 194:2715–24. doi: 10.4049/jimmunol.1402281
35. Amir ED, Davis KL, Tadmor MD, Simonds EF, Levine JH, Bendall SC, et al. viSNE enables visualization of high dimensional single-cell data and reveals phenotypic heterogeneity of leukemia. Nat Biotechnol. (2013) 31:545–52. doi: 10.1038/nbt.2594
36. Saraste M, Irjala H, Airas L. Expansion of CD56Bright natural killer cells in the peripheral blood of multiple sclerosis patients treated with interferon-beta. Neurol Sci. (2007) 28:121–6. doi: 10.1007/s10072-007-0803-3
37. Halenius A, Hengel H. Human cytomegalovirus and autoimmune disease. Biomed Res Int. (2014) 2014:1–15. doi: 10.1155/2014/472978
38. Kheav VD, Busson M, Scieux C, Peffault de Latour R, Maki G, Haas P, et al. Favorable impact of natural killer cell reconstitution on chronic graft-versus-host disease and cytomegalovirus reactivation after allogeneic hematopoietic stem cell transplantation. Haematologica. (2014) 99:1860–7. doi: 10.3324/haematol.2014.108407
39. Redondo-Pachón D, Crespo M, Yélamos J, Muntasell A, Pérez-Sáez MJ, Pérez-Fernández S, et al. Adaptive NKG2C + NK cell response and the risk of cytomegalovirus infection in kidney transplant recipients. J Immunol. (2017) 198:94–101. doi: 10.4049/jimmunol.1601236
40. Hart GT, Tran TM, Theorell J, Schlums H, Arora G, Rajagopalan S, et al. Adaptive NK cells in people exposed to Plasmodium falciparum correlate with protection from malaria. J Exp Med. (2019) 216:1280–90. doi: 10.1084/jem.20181681
41. Bjorkstrom NK, Riese P, Heuts F, Andersson S, Fauriat C, Ivarsson MA, et al. Expression patterns of NKG2A, KIR, and CD57 define a process of CD56dim NK-cell differentiation uncoupled from NK-cell education. Blood. (2010) 116:3853–64. doi: 10.1182/blood-2010-04-281675
42. Tesi B, Schlums H, Cichocki F, Bryceson YT. Epigenetic regulation of adaptive NK cell diversification. Trends Immunol. (2016) 37:451–61. doi: 10.1016/j.it.2016.04.006
43. Gross CC, Schulte-Mecklenbeck A, Rünzi A, Kuhlmann T, Posevitz-Fejfár A, Schwab N, et al. Impaired NK-mediated regulation of T-cell activity in multiple sclerosis is reconstituted by IL-2 receptor modulation. Proc Natl Acad Sci USA. (2016) 113:E2973–82. doi: 10.1073/pnas.1524924113
44. Holder KA, Lajoie J, Grant MD. Natural killer cells adapt to cytomegalovirus along a functionally static phenotypic spectrum in human immunodeficiency virus infection. Front Immunol. (2018) 9:2494. doi: 10.3389/fimmu.2018.02494
45. Strowig T, Brilot F, Arrey F, Bougras G, Thomas D, Muller WA, et al. Tonsilar NK cells restrict B cell transformation by the epstein-barr virus via IFN-γ. PLoS Pathog. (2008) 4:e27. doi: 10.1371/journal.ppat.0040027
Keywords: multiple sclerosis, natural killer cells, cytomegalovirus, NKG2C, FcεRIγ, PLZF
Citation: Moreira A, Alari-Pahissa E, Munteis E, Vera A, Zabalza A, Llop M, Villarrubia N, Costa-García M, Álvarez-Lafuente R, Villar LM, López-Botet M and Martínez-Rodríguez JE (2019) Adaptive Features of Natural Killer Cells in Multiple Sclerosis. Front. Immunol. 10:2403. doi: 10.3389/fimmu.2019.02403
Received: 11 June 2019; Accepted: 25 September 2019;
Published: 15 October 2019.
Edited by:
Zsolt Illes, University of Southern Denmark, DenmarkReviewed by:
Catharina C. Gross, University of Münster, GermanyCopyright © 2019 Moreira, Alari-Pahissa, Munteis, Vera, Zabalza, Llop, Villarrubia, Costa-García, Álvarez-Lafuente, Villar, López-Botet and Martínez-Rodríguez. This is an open-access article distributed under the terms of the Creative Commons Attribution License (CC BY). The use, distribution or reproduction in other forums is permitted, provided the original author(s) and the copyright owner(s) are credited and that the original publication in this journal is cited, in accordance with accepted academic practice. No use, distribution or reproduction is permitted which does not comply with these terms.
*Correspondence: Miguel López-Botet, bWlndWVsLmxvcGV6LWJvdGV0QHVwZi5lZHU=; Jose E. Martínez-Rodríguez, am1hcnRpbmV6ckBob3NwaXRhbGRlbG1hci5jYXQ=
Disclaimer: All claims expressed in this article are solely those of the authors and do not necessarily represent those of their affiliated organizations, or those of the publisher, the editors and the reviewers. Any product that may be evaluated in this article or claim that may be made by its manufacturer is not guaranteed or endorsed by the publisher.
Research integrity at Frontiers
Learn more about the work of our research integrity team to safeguard the quality of each article we publish.