- 1Istituto per la Ricerca e l'Innovazione Biomedica (IRIB), Consiglio Nazionale delle Ricerche, Palermo, Italy
- 2Institute of Microbiology of The Czech Academy of Sciences, Prague, Czechia
Extensive exploitation of titanium dioxide nanoparticles (TiO2NPs) augments rapid release into the marine environment. When in contact with the body fluids of marine invertebrates, TiO2NPs undergo a transformation and adhere various organic molecules that shape a complex protein corona prior to contacting cells and tissues. To elucidate the potential extracellular signals that may be involved in the particle recognition by immune cells of the sea urchin Paracentrotus lividus, we investigated the behavior of TiO2NPs in contact with extracellular proteins in vitro. Our findings indicate that TiO2NPs are able to interact with sea urchin proteins in both cell-free and cell-conditioned media. The two-dimensional proteome analysis of the protein corona bound to TiO2NP revealed that negatively charged proteins bound preferentially to the particles. The main constituents shaping the sea urchin cell-conditioned TiO2NP protein corona were proteins involved in cellular adhesion (Pl-toposome, Pl-galectin-8, Pl-nectin) and cytoskeletal organization (actin and tubulin). Immune cells (phagocytes) aggregated TiO2NPs on the outer cell surface and within well-organized vesicles without eliciting harmful effects on the biological activities of the cells. Cells showed an active metabolism, no oxidative stress or caspase activation. These results provide a new level of understanding of the extracellular proteins involved in the immune-TiO2NP recognition and interaction in vitro, confirming that primary immune cell cultures from P. lividus can be an optional model for swift and efficient immune-toxicological investigations.
Introduction
Titanium dioxide nanoparticles (TiO2NPs) are very appealing nanomaterials for a variety of emerging applications. Unique chemical and physical features combined with limited toxicity, inertness, and biocompatibility make these nanoparticles one of the most highly employed nanomaterials for water purification, soil remediation, medical products, skin-care products, sensors, electronics, and many others (1). The same nanoparticles may also have distinctive bioactive properties promoting or preventing human and environmental health. The growing use of TiO2NPs stimulated an outbreak of competing research (from molecules to ecosystems) aimed at delineating the safe limits of TiO2NPs use in occupational or environmental exposures. This emphasizes the great level of interest concerning the biosafety of TiO2NPs. However, a complete and comprehensive understanding of the biosafety of TiO2NPs has not yet been achieved, due to large gaps of information in risk management, disposal, knowledge on the biomedical potentiality, interaction with many different biological factors (molecules) in environments and microenvironments, and due to ethical issues. Nanomaterials interact with living organisms through biological fluids (including blood, lung fluids, and bile fluids depending on the route of exposure), and act as a scaffold for biomolecules (2). The 3R principles of reduce, refine, and replace (3) have evolved into essential aspects for planning scientific trials that use animal models. New and more feasible approaches have resulted in the development of the novel in vitro–ex vivo methods to limit the use of living organisms (4). Based on this aim, we use primary cell cultures that accurately represent the biological tissue microenvironment in which cells reside, because cell–cell signaling remains preserved (5). Thus, primary cultures have become a much more appropriate tool for biotechnological applications and nano-safety/nano-toxicity investigations.
The new frontier in immuno-nano research has highlighted the sea urchin in vitro-ex vivo model in the practical and vigilant application of the 3R principle. This model has been launched recently by the European Partnership for Alternative Approaches to Animal Testing and Registration, Evaluation, Authorization, and Restriction of Chemical Substances regulation to enhance safety through the proper recognition of emerging chemicals/materials. Immunity is a purveyor of the internal equilibrium of organisms, and a century of research has uncovered the conserved means and mechanisms by which the immune system recognizes danger and regulates its own activity (6). Sea urchin genome sequence highlighted strong similarities between sea urchin and mammalian immunity, and is an exceptional example of sensing capacity and immune system complexity in an invertebrate (7). In many cases, sea urchin immune cells are the sole source of functional biomolecules that are secreted into the coelomic fluid (CF) (the echinoid body fluid that shares some common functions with those of higher animal blood) to maintain the proper internal equilibrium and intercellular crosstalk (8), and act as highly robust in vitro (4). In vivo studies on sea urchin immune cells demonstrate that immune cells from Mediterranean Sea urchin, Paracentrotus lividus, show selective changes in specific pathways when exposed to a range of nanoparticles (9, 10), similar to what is known in humans. For example, authors support the evidence that P. lividus phagocytes interacting with TiO2NPs elicit a receptor-mediated phagocytic mechanism involving the TLR/p38 MAPK signaling pathway, but do not activate an inflammatory response or the Hsc70-dependent stress response (10).
Growing evidence suggests that the interaction between nanoparticles and native or non-native proteins (in vivo/in vitro) forms a dynamic biomolecule coating or “corona,” representing bio-nano interface that is perceived by cells (2). This bio-nano interaction can be affected by many factors, especially in the marine environment and the internal body fluids of marine invertebrates (11).
However, there is little current knowledge on the secreted functional biomolecules involved in the immune nanoscale interactions with TiO2NPs and subsequent intracellular signal transduction, cellular trafficking and fate, particularly with regards to marine invertebrates.
Here, we characterize the behavior of TiO2NPs in a complex physiological medium, with a focus on the identification of sea urchin extracellular proteins that form the protein corona on the particle surface. We demonstrate that TiO2NPs acquire a “biological identity” quickly, and that the main constituents shaping sea urchin cell-conditioned TiO2NP protein corona are adhesion proteins (Pl-toposome, Pl-galectin-8, Pl-nectin) and other ligands that mediate phagocytosis (actin, tubulin). These results provide new insights into the sea urchin extracellular signaling implicated in immune-TiO2NP recognition and interaction in vitro, suggesting intriguing ideas about the use of sea urchin immune cells as a powerful tool for fast nano-safety/nano-toxicity immune investigations in vitro.
Materials and Methods
TiO2NP Source
Aeroxide TiO2 P25 nanoparticles (primary particle size ranging from 10 to 65 nm, irregular and semi-spherical shape; mesoporous NPs, anatase, and rutile 4:1) were purchased from Evonik Degussa (Essen, Germany) and characterized previously in several aqueous solutions (from freshwater to seawater) by transmission electron microscopy (TEM), Brunauer, Emmett and Teller method, and dynamic light scattering (DLS), as described by Brunelli et al. (12) and Pinsino et al. (10). TiO2NP stock suspension (100 μg mL−1) was prepared in ultrapure water (18.2 MΩ cm−1) (Purelab Option-Q System, UK), vortexed for 5 min and sterilized under UV light prior to use.
Animals, Cell Cultures, and Sample Collection
Specimens of the sea urchin P. lividus were collected along the unpolluted coast of Sicily (Marine Protected Area, Italy) and were acclimatized and maintained under controlled conditions of temperature (16 ± 2°C), pH (8.1 ± 0.1), salinity (38–39%), and density (1.028–1.030 g/cm3). Animal handling, immune cell harvesting, and primary cell culture were performed as described by Pinsino and Alijagic (4). CF was collected diluted 1:1 in coelomocytes culture medium (CCM), and coelomocytes (immune cells) were counted and adjusted to 1.5 × 106 cells/mL and seeded into each well of a 25-well plate (Thermo Fisher Scientific, UK). A TiO2NP suspension was immediately added to the immune cell cultures (1 μg mL−1 final concentration) and incubated at 16 ± 2°C for 24 h. At 3 and 24-h, medium was partially aspirated and cells were pelleted by centrifugation (9,000 × g, 10 min, 4°C) to purify cell-free CF plus CCM containing TiO2NPs, which was centrifuged a second time as described below. The adherent cells over the bottom of the culture well were gently dislodged with a soft scraper, aspirated into the remaining CCM, centrifuged (9,000 × g, 10 min, 4°C), and pellets were stored in aliquots at −20 and −80°C for future use.
Quantitative in vitro-ex vivo Cell-Based Assays
All plate-based assays were performed in a 96-well white, opaque-walled plate (Thermo Fisher Scientific, UK) in a final volume of 100 μL. Immune cells were plated at a density of 1 × 105 cells/well. TiO2NP exposure started upon cell plating by the gentle drop-by-drop introduction of the particles into the medium (0.1, 1, 10, and 100 μg mL−1 final concentration), and incubated in the dark at 16 ± 2°C. Immune cell samples were obtained from at least five sea urchins. After incubation with the TiO2NPs, cell viability and cytotoxicity were evaluated by RealTime-Glo MT Cell Viability Assay (Promega, USA), and the non-lytic CellTox™ Green Cytotoxicity Assay (Promega, USA), respectively, according to the manufacturer's instructions, with minor changes (4). MT Cell Viability Substrate plus NanoLuc Enzyme, and/or CellTox Green Dye (equilibrated to 16 ± 2°C) were added to the wells 21 h after exposure, and 3 h before the first real-time cell viability and cytotoxicity measurements (from 24 to 72 h of exposure to TiO2NPs). Luminescence and fluorescence were measured using GloMax Discover high-performance Microplate Reader (Promega, USA).
After 24 h of cellular exposure to particles, caspase 3/7 activity was evaluated by Caspase-Glo 3/7 Assay (Promega, USA). Hydrogen Peroxide (H2O2) levels were evaluated by ROS-Glo H2O2 Assay, according to the manufacturer's instructions, with minor changes. Plates were gently shaken for 30 min at 16 ± 2°C prior to the measurement.
Nanoparticles Conditioning and Protein Corona Profiling
TiO2NPs were incubated in cell-free and/or cell-conditioned media in the dark at 16 ± 2°C in a plate shaker (bioSan, Latvia) for a maximum length of 24-h. TiO2NP incubation in cell-free medium (cell-free CF plus CCM) was prepared in TreffLab microcentrifuge tubes, whereas cell-conditioned medium was performed in a 25-well plate as described above (see Animals, cell cultures and sample collection section). After incubation, TiO2NPs with the associated proteins were recovered by centrifugation (21,130 × g, 20 min, 4°C) followed by three vigorous wash steps in ASW. The pellets of TiO2NPs with protein coronas were re-suspended with vigorous agitation in 10 μL of 2 × Laemmli sample buffer (Bio-Rad, USA) supplemented with β-mercaptoethanol, according to the manufacturer's instructions. Samples were boiled for 5 min to strip protein corona, and the TiO2NPs were removed by centrifugation (21,130 × g, 20 min, 4°C) following the method reported by Monopoli et al. (13) with slight modifications, as described. Eluted protein coronas were loaded into 4–20% Mini-PROTEAN TGX precast polyacrylamide gel (Bio-Rad, USA). Samples of media that were not incubated with TiO2NPs were included. Gels were run at a voltage of 150 V for 5 min and 100 V for 80 min. Gels were stained with Coomassie Brilliant Blue R250 to identify protein bands. All experiments were conducted at least in triplicate to ensure reproducibility.
To obtain the two-dimensional gel electrophoresis (2DE) map of the TiO2NP protein corona, eluted proteins recovered from 30 mL of cell-free CF plus CCM (1 μg mL−1 final concentration, 24-h of incubation with TiO2NPs) were incubated in a denaturing buffer [7 M urea, 2 M thiourea, 4% w/v CHAPS, 1% v/v Triton X-100, 40 mM Tris-HCl pH 8.8, 10 mM DTT (Sigma Aldrich), with 1.2% DeStreak (GE Healthcare), 0.2% v/v Bio-Lyte pH 3–10 (BioRad), 1% v/v Protease Inhibitor Mix (GE Healthcare), and 1 mM Na3VO4]. Samples were processed with ReadyPrep™ 2-D Cleanup Kit and ReadyPrep Reduction Alkylation Kit (BioRad), quantified by 2-D Quant kit (GE Healthcare), focused on 18 cm, pH 3–10 non-linear ReadyStrip™ IPG Strips (BioRad), and separated on 9–16% SDS-PAGE gel. Protein spots were detected by silver staining.
Extracellular Proteins, Protein Corona, and Immunoblotting Analyses
After incubation with the TiO2NPs, culture medium was analyzed for protein content as follows. Samples were concentrated by membrane ultrafiltration with 5 kDa cut-off Vivaspin-500 concentrators (Sartorius, UK). The protein content of each sample were quantified by the Bradford method with the BioRad assay kit (Hercules, CA, USA). Prior to SDS-PAGE, extracellular proteins (secretomes) were mixed with acetone (1:1) for overnight precipitation at −20°C. Acetone-precipitated extracellular proteins were suspended in SDS sample buffer supplemented by the β-mercaptoethanol, boiled for 5 min and separated on 4–20% Mini-PROTEAN TGX precast polyacrylamide gels (BioRad, USA), and transferred to nitrocellulose membrane (Amersham, UK) according to standard procedures. Non-specific binding sites were blocked with Odyssey blocking buffer (LI-COR Biosciences, USA) for 1 h at room temperature (RT). After blocking, membranes were incubated with either one of the following primary antibodies in Odyssey blocking buffer with 0.1% Tween20: (i) anti-Pl-toposome (BEVIB12b8) 1:200 dilution; (ii) anti-Pl-nectin 1:200 dilution; (iii) anti-Pl-galectin-8 1:800 dilution; (iv) anti-Hsp70 (SIGMA, Cat N. H-5147) 1:1,000 dilution; (v) anti-P-p38 MAP Kinase (Tr180/Tyr182) (Cell Signaling, 9211) 1:250 dilution; (vi) anti-β-actin (SIGMA, A5441) 1:500 dilution; anti-α-tubulin (SIGMA, T5168) 1:2,000 dilution. Following rinsing, membranes were incubated with a fluorescein-labeled secondary anti-mouse and/or anti-rabbit antibody (LI-COR Biosciences) and visualized with Odyssey Infrared Imager (LI-COR Biosciences). Immunoblotting for TiO2NPs protein coronas were performed following the procedures described in this section for secretomes.
Electron Microscopy Analysis
Scanning Electron Microscopy
Control and TiO2NPs-exposed immune cells (1 μg mL−1 final concentration, 24 h of exposure) that adhered to 12 mm circular glass coverslips (Menzel Gläser, Germany) were fixed with a 1:1 mixture of 6% glutaraldehyde in 100 mM cacodylate buffer and ASW (pH 8–8.5) for 1 h at RT. Coverslips were washed three times with cacodylate buffer, dehydrated through an ethanol series (25, 50, 75, 90, 96, and 100%), and dried in a K850 Critical Point Dryer (Quorum Technologies Ltd, Ringmer, UK). The dried coverslips were sputter coated with 3 nm platinum using high-resolution Turbo-Pumped Sputter Coater Q150T (Quorum Technologies Ltd, Ringmer, UK). The samples were examined in FEI Nova NanoSEM scanning electron microscope (FEI, Czech Republic) at 5 kV using CBS and TLD detectors.
Transmission Electron Microscopy
Immune cells were incubated in a 15 mL tube (Thermo Scientific, USA) (1 μg mL−1 final concentration for 24 h) with gentle agitation, followed by fixing as described above. After intensive washes, cells were post-fixed overnight at 4°C with 1% osmium tetroxide in cacodylate buffer. Samples were washed three times in cacodylate buffer at 4°C and double distilled H2O, warmed to the RT and embedded into 4% low-melt agarose. Solidified agarose was cut into 1 × 1 mm cubes, dehydrated in ethanol series (25, 50, 75, 90, 96, and 100%), and embedded into the epoxy resin (EMBed-812 Embedding kit; Electron Microscopy Sciences). Ultrathin sections were stained with uranyl acetate and lead citrate, and examined with a FEI Morgagni 268(D) electron microscope (FEI, Czech Republic).
Statistics
Statistical software GraphPad Prism Software 6.01 (USA) was used for data analysis. Statistical differences were estimated by one-way or two-way ANOVA (followed by the multiple comparison tests) and compared to the appropriate control. The level of significance was set at p ≤ 0.05. Data were presented as mean ± SE. Gel scans were processed by ImageJ software (NIH, USA).
Results and Discussion
Cell-Conditioned Protein Corona on TiO2NPs and Extracellular Signaling Include Sea Urchin Adhesion Proteins and Other Ligands That Mediate Phagocytosis
The extracellular environment is highly dynamic due to the process of “cell-conditioning,” in which cells deplete and secrete biomolecules (14). When engineered particles encounter an extracellular environment, they are coated by a complex biological fluid composed of proteins and other biomacromolecules, forming a protein corona, and providing a “biological identity” of nanosized materials (15). The biological identity of the NPs depends on the protein repertoire that they encounter plus their composition and structural properties, which are crucial for NP interactions with living cells. Because we found that cell-free medium contains an average protein concentration of about 7.093 ± 0.565 μg mL−1 (mean ± SE), we postulated that incubation of TiO2NPs within this medium would result in the adsorption of proteins onto the particle surface. To support this notion, we investigated if, when, and which of the secreted proteins may be involved in the immune nanoscale interaction with TiO2NPs.
Sea Urchin Protein Corona Profiling in a Cell-Free Biological Medium
To profile the sea urchin P. lividus corona proteins that have high affinity for the TiO2NPs, varying concentrations (1, 10, and 100 μg mL−1) of TiO2NPs were incubated with cell-free CF collected from donors diluted in the anticoagulant solution (CCM) containing EGTA (a chelating agent with a high affinity for Ca2+ that inhibits immune cell aggregation mediated by adhesion proteins) (4), followed by immediate centrifugation to pellet the particles. Proteins adsorbed onto the particle surface by 24 h were eluted, separated by reducing 1D SDS-PAGE, and labeled with Coomassie Brilliant Blue R250. Proteins adsorbed onto and extracted from the nanoparticle surface (first three lanes, namely 24 h corona) were compared to the total extracellular proteins (second three lanes, namely 24 h secretome) (Figure 1A). The process of corona formation is determined by the competition among proteins in the medium based on the affinity of each protein to the particle surface. A common feature in all protein corona samples was a selective enrichment of proteins of > 170 and about 100, 40, and 15 kDa (Figure 1A, black asterisks). There were other proteins adsorbed onto the particle surface, although to a much lesser extent, which was not easily visualized by 1D SDS-PAGE. We note that with increasing concentrations of particles, there were increased quantitative (but not qualitative) enrichments of the protein corona. Notably, a few proteins present in the corona showed a correlated reduction in TiO2NP-exposed secretomes compared to the control secretome in which the cell-free medium was not exposed to particles. Specifically, proteins ≥ 170, 55, 40 kDa showed strong bands in the control secretome but not in the TiO2NP-exposed secretomes (Figure 1A, red asterisks).
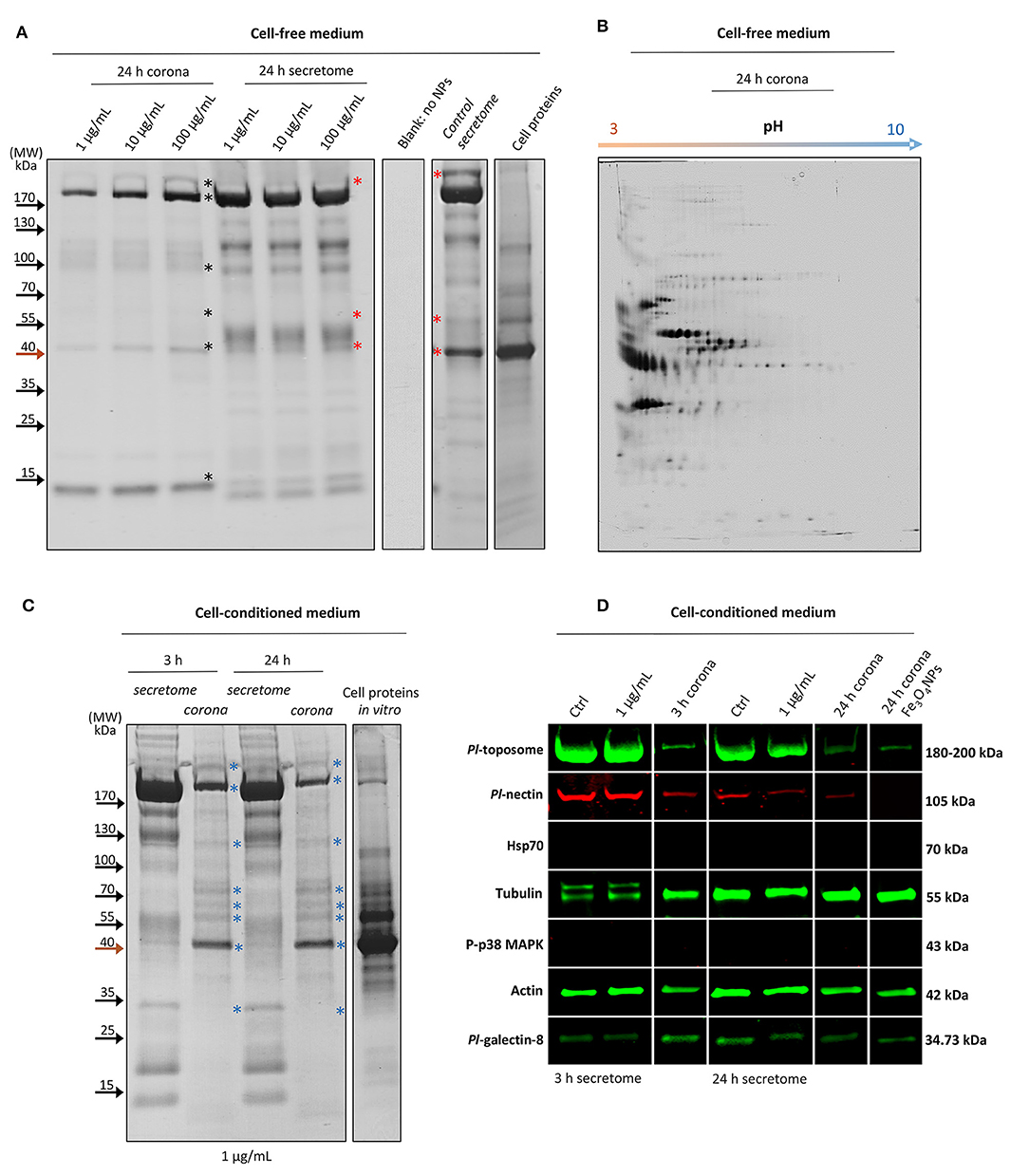
Figure 1. The interaction between Sea urchin secretome and TiO2NPs, extracellular signaling and protein corona identification. (A) One-dimensional profiling of the formed non-cell-conditioned protein corona at 1, 10, and 100 μg mL−1 TiO2NPs after 24 h of incubation. (B) Two-dimensional profiling of the TiO2NP protein corona after 24 h of incubation in the non-cell-conditioned medium (Cell free CF plus CCM). (C) One-dimensional profiling of the protein corona formed in situ (in vitro) at 3 and 24 h. (D) Representative immunoblotting results show limited influence of the TiO2NPs on sea urchin extracellular signaling and reveal the main constituents shaping the cell-conditioned protein corona (Pl-toposome, Pl-galectin-8, Pl-nectin, actin, tubulin). *, see text.
The analysis of interacting sea urchin extracellular proteins by 2DE permitted discrimination of the TiO2NP protein corona based on the pH of the proteins eluted from the particles. The 2DE map of the proteins from TiO2NPs (1 μg mL−1 final concentration) after incubation for 24 h in a cell-free medium showed that the majority of the proteins were negatively charged and distributed between the pH 3 and 6 (Figure 1B). Similarly, the TiO2NPs were also negatively charged between pH 7 and 8 (16) (this study: pH 7.4). The mechanism by which proteins are known to bind particle surfaces are principally hydrophobic/electrostatic interactions and hydrogen bonds, which are weak interactions. Here, both the TiO2NPs and the bound proteins were negatively charged, and therefore were not likely to form complexes based only on electrostatic interactions. Accordingly, the highly abundant proteins in the protein corona may be dependent on protein abundance, non-electrostatic affinity, solubility, and their degree of unfolding (17). For example, related studies on sea urchin extracellular protein/nanoparticle interaction that employed other engineered metal nanoparticles (e.g., iron oxide nanoparticles) resulted in a different protein corona profile compared to those of TiO2NPs (although there were some exceptions) (Supplementary Figure 1). This result supported the idea that TiO2NPs acquire a biological identity that may be based on selective affinity for a few proteins and a non-specific affinity for others. Notably, Fe3O4NPs are similar to TiO2NPs with regard to surface area, charge, and primary size, but have different crystalline structure and stability. Fe3O4NPs are well-known to have harmful effects on sea urchin immune cell biological functions in vivo (9).
It has been reported that proteins do not distribute randomly on particle surfaces, but form clusters in association with the first proteins that bind, which in turn attracts other proteins and continues until surface is filled (18). Therefore, mechanisms such as molecular relaxation and spreading depending on the time that proteins remain on the surface, can be a determining factor in making permanent attachment of the proteins.
Macromolecules of high molecular weight and/or negative charge favor steric or electrostatic colloidal TiO2NP stabilization (19). As is well-known, some ions of opposite charges adsorb onto NP surfaces, forming a Stern layer, while the high content in biomacromolecules—lipids, sugars, nucleic acids, and particularly proteins—alters the stability of NPs and changes surface chemical characteristics (20, 21). Moreover, divalent ions, such as Ca2+ and Mg2+, can act as an effective bridge to adsorb negatively charged proteins onto the negatively charged surface of TiO2NPs (22). It is therefore worth noting that CCM does not contain any organic molecules and is composed of divalent ions and a chelating agent with a high affinity for Ca2+ (4), whereas cell-free CF media contain functional biomacromolecules (8).
On the one hand, the high salt concentration of CCM tends to screen (reduce) the electrostatic repulsions between nearby nanoparticles, favoring homoaggregation (particle-particle complexes). On the other hand, the abundance of organic components suspended in cell-free CF media are likely to favor heteroaggregation (particle-protein complexes) (19).
Sea Urchin Protein Corona Profiling in a Cell-Conditioned Medium
To investigate the sea urchin cell-conditioned protein corona formed upon contact with the TiO2NPs in situ (in vitro), TiO2NPs (1 μg mL−1) were incubated for 3 and 24 h with both not-induced and induced cell secreted-proteins (proteins that were putatively secreted ex novo during cell incubation) (Figure 1C). At this concentration of particles, sea urchin immune cells did not show any harmful effects on biological functions (see the section on protein corona architecture intercedes TiO2NPs association and clearance by immune cells). After 3 and 24 h, cell-conditioned TiO2NP protein corona profiles showed the same band pattern without any apparent rearrangement of proteins on the surface, perhaps because the most abundant proteins that bound the particle surface also have had the highest binding affinity (the Vroman effect) (23). The coronas appeared much more enriched in proteins than those obtained from cell-free biological medium at the same particle concentration (1 μg mL−1) (cell conditioning) (Figure 1C, bands indicated with blue asterisks; compare to bands with black asterisks in Figure 1A).
These findings were consistent with the notion that immune cells secrete immunologically active proteins that interact with particles (24) that contribute to the generation of the protein corona. This result is in agreement with the activities of immune cells from the earthworm Eisenia fetida exposed to silver nanoparticles (25). Notably, by pre-incubating the TiO2NPs for 6 h with bovine serum albumin (BSA) (10 and 100 μg mL−1) prior to immune cell exposure in vitro or by adding BSA (10 or 100 μg mL−1) and TiO2NPs into the immune cell culture simultaneously, caused changes in the TiO2NP protein corona profile (Supplementary Figure 2). In agreement with recent reports, BSA has high affinity for TiO2NP surfaces that act to reduce the capability of other proteins to be adsorbed onto the particle surface thereby altering cell culture behavior (Supplementary Figure 3). The protein/nanoparticle interaction ranges from opsonisation by immune or non-immune proteins for uptake by phagocytic cells, and determines the subsequent immunological cascade that can either stimulate or mitigate the immune response (26).
Highlighting the Biological Identity of the TiO2NP-Protein Complexes in the Sea Urchin Immune Cells
The biological identity of a nanoparticle changes over time in the extracellular environment because of cell conditioning of the medium. To obtain a broad insight on the biological identity of the TiO2NPs in vitro, we identified a few key regulatory proteins that are putatively involved in the corona formation (Figure 1D).
Proteins for analysis were chosen by their approximate molecular weight that were comparable to bands identified by reducing SDS-PAGE, and based on proteins known to be involved in cellular adhesion (Pl-toposome, Pl-galectin-8, Pl-nectin), cytoskeleton organization (actin and tubulin), and immune/stress response (Hsp70, P-p38 MAPK). The toposome is essential for sea urchin cell adhesion and development, and is a modified iron-less calcium-binding transferrin of six identical polypeptides of 170 kDa (27). In the sea urchin P. lividus, both CF and immune cells contain the precursor of toposome (180–200 kDa) that can be secreted in large quantities into the CF upon injury (28–30). In agreement, the CF of immune cells maintained in culture for 3 and 24 h (both exposed to TiO2NPs and control) contained band of > 170 kDa consistent with the expected size of secreted toposome (Figure 1C). By immunoblotting, the toposome was detected as a large protein band with a molecular weight ranging from 180 and 200 kDa in both control and exposed samples (Figure 1D). The presence of a large amount of available toposome in the CF was not consistent with the minor band of ~200 kDa for extracted proteins in the corona (Figure 1D). The expectation was that the strong binding affinity with the particle surface would have resulted in a strong band in the eluted corona proteins. In contrast, two other well-known proteins involved in sea urchin cell adhesion (galectin-8 and nectin) appeared in much lower content, and their binding affinities to TiO2NPs were very similar (Figure 1D). Recently, studies have described the possibility that both galectin and nectin may take part in corona modelling (31, 32). It is known that galectin-8 functions in cell adhesion a substrate, which promotes their cytoskeletal organization, attachment, and spreading by triggering integrin-mediated signaling cascades (33). When galectin-8 is present in excess as a soluble ligand, it negatively regulates cell adhesion and inhibits cell-substrate adhesion (33). In contrast, nectin is involved in cell-cell adhesion and is essential for ontogenesis, regeneration, and tissue maintenance (34). Notably, the levels of these proteins in the sea urchin secretome exposed to TiO2NPs for 24 h was lower than that for the controls, perhaps because these proteins were sequestered on the particle surface. Both sea urchin toposome and nectin are glycoproteins (28, 35), while galectin-8 binds glycoproteins (36). Glycoproteins have good binding affinity to TiO2NP particle surface (37). Notably, glycoproteins may be very important in transport with good binding capacity for calcium (37), particularly given that calcium signaling has a key role in phagocytosis (38). The incorporation of cell-secreted proteins into the particle-protein complex may increase receptor affinity or may target additional receptors.
The main cytoskeleton proteins actin and tubulin were found to be strongly involved in the formation of the TiO2NP-corona complex both at 3 and 24 h (Figure 1D), suggesting a crucial role for these proteins in determining both TiO2NP stability and biological effect. As is well-known, actin and tubulin are present in the intracellular and extracellular space in a monomer/polymer dynamic exchange form (39, 40). Tubulin aids in maintaining cell shape and undergoes post-translational modifications (glutamylation and phosphorylation) to specify microtubule subpopulations for particular functions (41). In agreement with tubulin functions, after 3 hrs, anti-α-tubulin antibody recognized two bands rather than one, suggesting that the cultures contained unattached cells undergoing morphological rearrangement. Notably, only one of the bands was associated with the TiO2NP-corona complex. However, it could not be excluded that the two tubulin bands may be due to partial protein degradation resulting from the extraction procedure. Actin is a highly versatile and conserved protein that can be secreted upon immune challenge, and it is involved in mediating phagocytosis that lead to killing bacteria and inhibiting innate immune inflammatory cascades (42, 43). On the other hand, Hsp70 and phosphorylated p38 MAPK were not detectable in any extracellular environment. Hsp70 translocates to the plasma membrane after a stress challenge and is released into the extracellular environment in a membrane-associated form that activates immune cells (44). Our results indicated a general healthy state of sea urchin immune cells in culture with TiO2NP exposure, which was not perceived as a stress inducer that was confirmed because it did not stimulate the release of the Hsp70 into the extracellular environment. p38 MAPK is a member of intracellular kinases that serves as focal point for diverse extracellular stimuli (45), which was used here as negative control.
Sea urchin extracellular proteins of different function adhere with varying affinity to TiO2NPs, perhaps acting as the immunoregulatory biointerface between particles and immune cells. Notably, Pl-toposome, Pl-galectin-8, actin, and tubulin were also detected in the corona of Fe3O4NPs, whereas nectin was not detected (Figure 1D, last lane). The protein corona modifies the size and the surface composition of the NPs, giving them a new identity depending on a selective affinity for a few proteins and a non-specific affinity for others that determines the full physiological response.
Protein Corona Architecture Intercedes TiO2NPs Association and Clearance by Immune Cells
Paracentrotus lividus CF includes three different immune cell populations; phagocytes, amoebocytes, and vibratile cells, that show a phagocytic cell prevalence (>80%) (46). Because CF may contain host-associated microbiota and other non-self particles, phagocytic activity is essential in the particle detection and clearance that maintains intracellular homeostasis (8). To focus on understanding the interaction between TiO2NPs and immune cells, sea urchin immune cell topography and related TiO2NP surface distribution was evaluated by SEM for samples after 24 h of exposure in vitro (1 μg mL−1 TiO2NPs final concentration).
Phagocytic cells appeared strongly adherent and well spread to a greater extent than the control cells (compare Figures 2A,C to Figures 2B,D), revealing a tight adhesion that may have been mediated by the communication between cell surface receptors and protein ligands integrated into the biocorona or by any unbound surfaces of the NPs. No other cell types appeared to be involved in interactions with the TiO2NPs (not shown). A few small aggregates/agglomerates of the TiO2NPs protein complexes were adhered to the outer membrane surface of the sea urchin phagocytic cells (black arrowheads in Figures 2B,D). Notably, the TiO2NPs (1 μg mL−1) were internalized in well-organized cytoplasmic vesicles with ranging in size from 150 nm to 3 μm in diameter that were located toward the periphery of the cell near the plasma membrane (Figures 2E,F). This result was in agreement with our recent reports that TiO2NPs stimulate sea urchin immune cell phagocytic activity that involves the TLR/p38 MAPK signaling pathway in vivo (10). No disruption of the internal cellular membranes or cytoplasmic structures or organelles were noted. The TiO2NPs-protein complexes on the particle surface may function to enhance particle internalization by a broad spectrum of ligand bound receptors and cytoskeletal elements that are known to mediate internalization. These results suggest that cells take up these particles very promptly, safely and efficiently. We speculate that to maintain immune resilience toward the TiO2NPs, the TiO2NPs-protein complexes are recognized by the immune cells as “self,” thereby not eliciting any harmful immune effects of the particles.
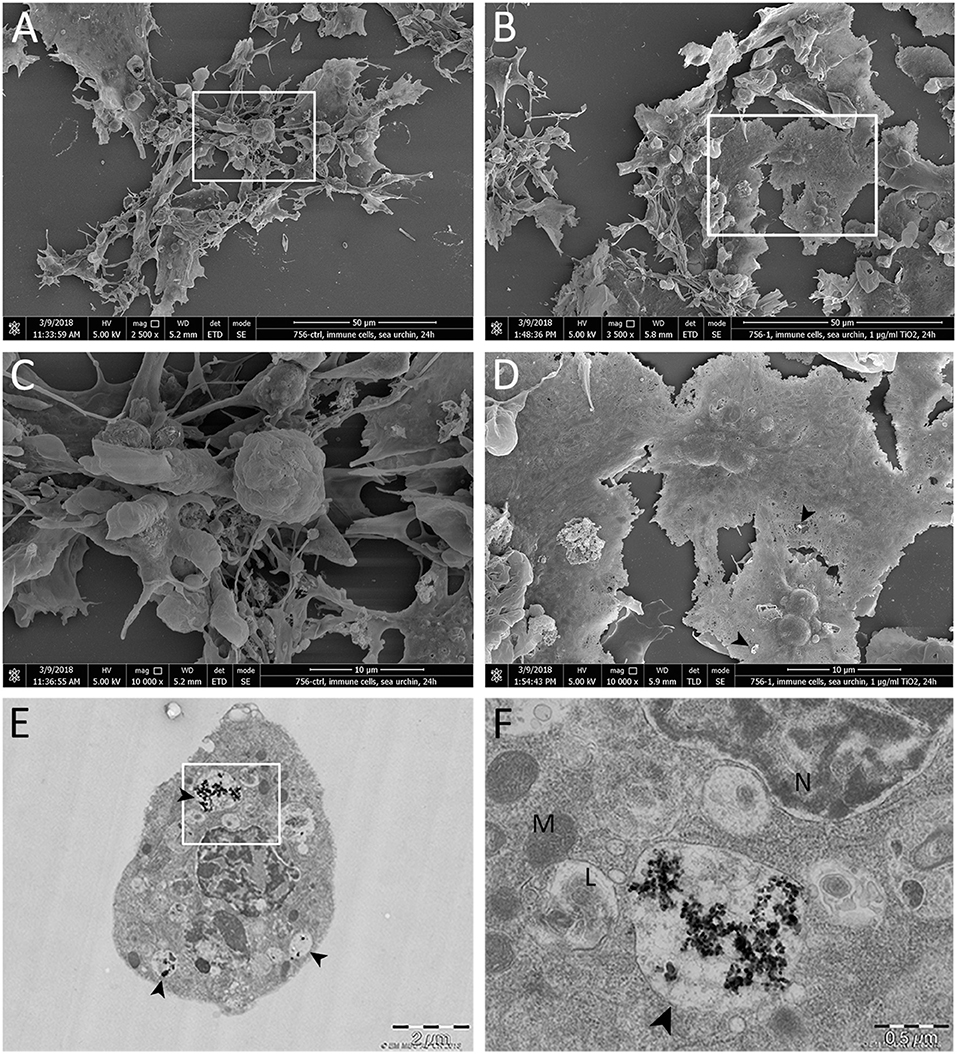
Figure 2. Scanning and transmission electron microscopy of the in situ (in vitro) interaction between TiO2NPs and sea urchin immune cells. (A,C) Control cells not exposed to TiO2NPs. (B,D) Immune cells exposed to 1 μg mL−1 TiO2NPs for 24 h. These figures illustrate interactions and selective binding of TiO2NPs on the immune cell (phagocyte) surface. Black arrowheads indicate small aggregate. (E,F) Sea urchin phagocyte after exposure to 1 μg mL−1 TiO2NPs for 24 h shows internalized TiO2NPs within a vesicular structure (black arrowhead) that is localized in the proximity with lysosomes (L). N, nucleus, M, mitochondria.
To support our speculation on the safety of the TiO2NPs, cell viability, cytotoxicity, caspase activity, and reactive oxygen species production were monitored in real-time measurement for cells exposed at increasing concentrations of the NPs (0.1, 1, 10, 100 μg mL−1) (Figure 3). The viability and cytotoxicity of exposed immune cells were similar to the controls during the 3 days of continuous monitoring, of which three measurement points (24, 48, 72 h) are shown. An exception was indicated for those cells exposed to the highest TiO2NP concentration (100 μg mL−1), which showed significantly decreased viability and significantly high toxicity (Figures 3A,B). For samples exposed to 0.1 to 10 μg mL−1 TiO2NPs, the RealTime-Glo MT cell viability assay indicated an increased trend (not statistically significant) in cell viability/metabolic activity compared to unexposed controls. Metabolites are largely controlled by extracellular signals, which direct the uptake, storage, and utilization of substrates (glucose, amino acids, nucleotide and fatty acids) (47). In turn, metabolites control the duration and intensity of innate or adaptive immune activation and memory cell formation. Studies elucidating metabolic profiles of immune cells exposed to TiO2NPs are necessary to clarify this fascinating result. We are continuing this analysis and our preliminary results suggest an increase of a few antioxidant metabolic pathways under exposure to TiO2NPs (1 μg mL−1 final concentration) (unpublished data).
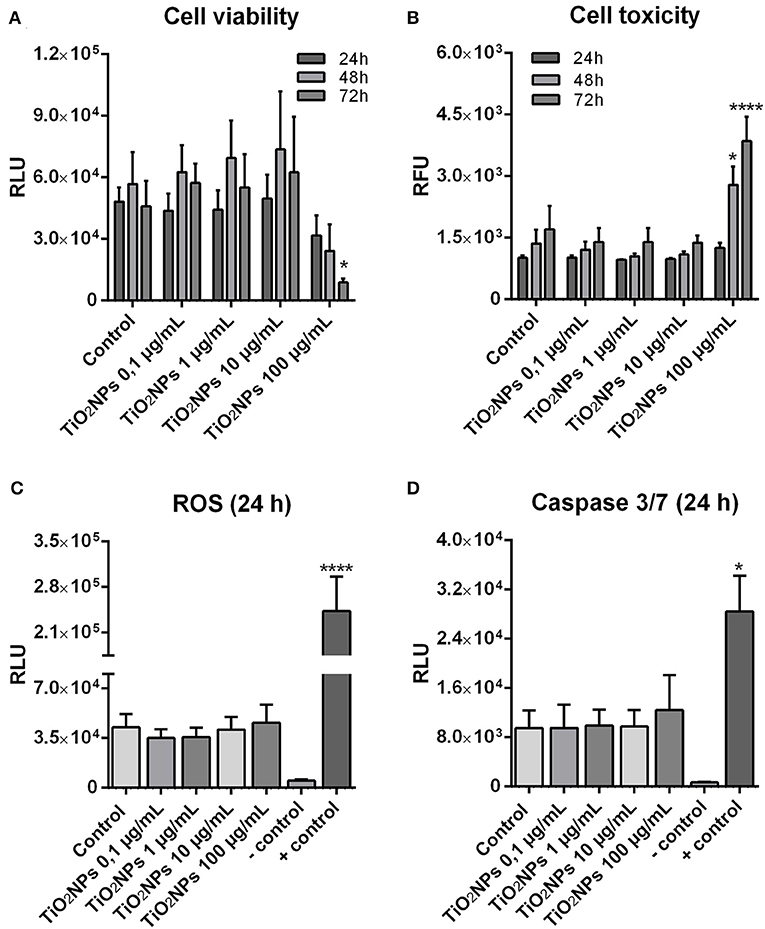
Figure 3. Impacts of TiO2NPs on the viability, toxicity, reactive oxygen species production and caspase activity on sea urchin immune cells. (A) Real-time viability assay over 72 h shows that only the highest dose of TiO2NPs (100 μg/mL) induces decrease in cell viability. (B) Cell toxicity shows an increase only in response to the highest TiO2NPs dose. (C) A Luciferase-based ROS assay does not indicate any significant increase in the ROS levels in response to TiO2NPs. Positive control (+ control): Hydrogen peroxide activates a strong increase in ROS production (24 h, 4 mM). (D) Caspase 3/7 activity shows that TiO2NPs (at all doses) does not induce an increase in the activity of these apoptotic enzymes. The positive controls (+ control) are cells exposed for 24 h to Iron oxide nanoparticles (100 μg/mL). Assays involved five biological replicates except for Caspase 3/7 assay, which had three replicates. Data are reported as the mean ± SE; stars (*) indicate significant differences among groups (*p < 0.05; ****p < 0.0001). RLU, Relative Luminescence Units; RFU, Relative Fluorescence Units. Negative control (- control) was medium plus particles without cells.
ROS production and caspase 3 and 7 levels did not show a significant dose-dependent increase in cells exposed to TiO2NPs (Figures 3C,D). In sea urchins, modest physiological levels of intracellular ROS are required throughout their entire lifespan (48). Powerful antioxidant machinery maintains sea urchin genomic stability through the activation of DNA repair actions, enabling long life and bypassing the outcomes of ageing (8). Critically, our findings support the notion that P. lividus immune cells act according to a simultaneously biphasic strategy: resistance and tolerance. Under homeostatic conditions, constitutive immunity maintains physiological order within the organism. Whereas, under perturbations such as TiO2NP exposure, the immune system induces a short-term and energetically expensive response perhaps explaining increase in metabolic activity that may result in the long-term activation of survival based on promoting tolerance (49, 50).
Conclusions
To the extent of our knowledge, this is the first study to describe an enriched cell conditioned TiO2NP protein corona profile from marine invertebrate immune cells in culture. The characterization of proteins bound to the NP surface is the first step toward understanding the true nature of NP-mediated biological effects. Living cells secrete extracellular molecules to retain cell cycle, nutrient uptake, cell signaling, and migration, and to cope with different foreign entities (51). These macromolecules are receiving much attention as they modify NPs, which affects their stability and fate that may stimulate or mitigate the immune response. Here, we demonstrated that several sea urchin extracellular proteins interact with TiO2NPs and bind swiftly to the particle surface. Recent studies describing NP-protein complexes formation on invertebrates highlighted the involvement of only single protein surrounding the NP surface (25, 52). In contrast, here we show that the main constituents shaping the TiO2NP protein corona from media conditioned by sea urchin immune cells are a subset of adhesion proteins and cytoskeletal proteins. This is in agreement with the most recent knowledge concerning a broad spectrum of proteins that form human NP-protein complexes (e.g., immunoglobulins, lipoproteins, complement components, acute-phase proteins, coagulation factors) (26). This finding is not surprising, given that the genome of Strongylocentrotus purpuratus sea urchin shows the close genetic relationship between sea urchins and humans (7), which further reinforces the relevance of this model organism as a proxy to humans. Protein corona formation on the surface of TiO2NPs modifies the physicochemical properties of the particles and changes their behavior in biological microenvironments, leading to aggregation and, in turn, the behavior of the interacting cells, leading to adherence, spreading, uptake capability, and increased metabolism. The TiO2NP-protein complex formation is mainly due to the intrinsic properties of extracellular proteins that form the corona (e.g., protein abundance, affinity, solubility). These proteins may have selective particle affinity (Pl-nectin) and/or non-selective affinity (e.g., Pl-toposome, Pl-galectin-8, actin and tubulin) for the TiO2NPs, as suggested by differences in protein complex formation on Fe3O4NPs and the BSA competition assay. Nectins are cell adhesion molecules that mediate the formation of cell adherence junctions. From an immunological point of view, nectins have become of particular interest due to their well-known involvement in mediating the immune responses of different immune cell types during interactions with several immune modulatory receptors expressed at the cell surface of immune cells (53). Our results provide evidence that the uptake mechanism for TiO2NP may be the outcome of receptor–ligand (e.g., nectin) interaction, even if a non-specific passive uptake mechanism cannot be excluded. Because nectin, toposome, galectin-8, actin, and tubulin first interact with the physical features of particles, these proteins are likely to have important roles in the recognition of the non-self material, both biological and non-biological by the sea urchin immune cells.
Data Availability Statement
The datasets generated for this study are available on request to the corresponding author.
Author Contributions
AP: conceptualization. AP and AA: methodology, data curation, and writing–original draft. AA: validation and formal analysis. AA and DC: cellular/biochemical investigation. AA, OB, and OK: SEM and TEM investigation. AP: writing-review, supervision, project administration, and funding acquisition.
Funding
This project has received funding from the European Union's Horizon 2020 research and innovation program under the Marie Skłodowska-Curie grant agreement [No. 671881].
Conflict of Interest
The authors declare that the research was conducted in the absence of any commercial or financial relationships that could be construed as a potential conflict of interest.
Acknowledgments
We would like to acknowledge Mr. Mauro Biondo for his technical support and sea urchin husbandry, Dr. Fanny Pojero for English editing and Matranga Lab for Pl antibodies (Matranga heritage). The authors gratefully acknowledge the access to the electron microscopy facility, supported by the project LO1509 of the Ministry of Education, Youth and Sports of the Czech Republic.
Supplementary Material
The Supplementary Material for this article can be found online at: https://www.frontiersin.org/articles/10.3389/fimmu.2019.02261/full#supplementary-material
Supplementary Figure 1. 2DE map of the proteins from Fe3O4NPs (1 μg mL−1 final concentration) after incubation for 24 h in a cell-free medium. The majority of the proteins are distributed between the pH 3 and 10 and are negatively charged, and show a different protein corona profile compared to TiO2NPs (although there were some exceptions). Results confirm that each particle is able to acquire a selective “biological identity” depending on the particle-protein affinity.
Supplementary Figure 2. TiO2NP protein corona competition assay. (A) One-dimensional profiling of the protein coronas formed on particles pre-incubated with BSA (10 and/or 100 μg mL−1) prior to immune cell exposure in vitro show the almost exclusive presence of the BSA (protein band of about 70 kDa). (B) One-dimensional profiling of the protein coronas formed on particle surface adding BSA (10 or 100 μg mL−1) and TiO2NPs (1 μg mL−1) into the immune cell culture simultaneously, show the presence of the BSA and the exclusion of the major sea urchin proteins. BSA has high affinity for TiO2NPs and reduce the capability of the sea urchin extracellular proteins to be adsorbed onto the particle surface (compare Figure 1C and Supplementary Figure 2). On the left: secretome samples; on the right: protein corona samples.
Supplementary Figure 3. Protein corona changes affect cellular behavior. (A) Sea urchin immune cells exposed to TiO2NPs for 24 h show well adherent phagocytes that spread their protrusions across the plate and maintain cell individuality. Amoebocytes morphology indicates the healthy state of these cells, as they do not show sign of evident degranulation and maintain an ovoid shape. (B) Immune cells exposed to BSA (10 μg mL−1) and TiO2NPs (1 μg mL−1) simultaneously, show a slightly reduced capability to maintain an uniform distribution, moderate clustering and ovoid amoebocytes. (C) Immune cells exposed to BSA (100 μg mL−1) and TiO2NPs (1 μg mL−1) simultaneously, show aggregation (cell-cell clustering) perhaps forming multinucleated syncytia, a reduced capability to form fine network fibers, and circular amoebocytes (>50%).
References
1. Cargnello M, Gordon TR, Murray CB. Solution-phase synthesis of titanium dioxide nanoparticles and nanocrystals. Chem Rev. (2014) 114:9319–45. doi: 10.1021/cr500170p
2. Mahon E, Salvati A, Baldelli Bombelli F, Lynch I, Dawson KA. Designing the nanoparticle–biomolecule interface for “targeting and therapeutic delivery”. J Control Release. (2012) 161:164–74. doi: 10.1016/j.jconrel.2012.04.009
4. Pinsino A, Alijagic A. Sea urchin Paracentrotus lividus immune cells in culture: formulation of the appropriate harvesting and culture media and maintenance conditions. Biol Open. (2019) 8:bio039289. doi: 10.1242/bio.039289
5. Bols NC, Pham PH, Dayeh VR, Lee LEJ. Invitromatics, invitrome, and invitroomics: introduction of three new terms for in vitro biology and illustration of their use with the cell lines from rainbow trout. In Vitro Cell Dev Biol Anim. (2017) 53:383–405. doi: 10.1007/s11626-017-0142-5
7. Sodergren E, Weinstock GM, Davidson EH, Cameron RA, Gibbs RA, Angerer RC, et al. The genome of the sea urchin Strongylocentrotus purpuratus. Science. (2006) 314:941–52. doi: 10.1126/science.1133609
8. Smith LC, Arizza V, Hudgell MAB, Barone G, Bodnar AG, Buckley KM, et al. Echinodermata: the complex immune system in echinoderms. In Cooper EL, editor. Advances in Comparative Immunology. Springer International Publishing AG (2018). p. 409–501. doi: 10.1007/978-3-319-76768-0_13
9. Falugi C, Aluigi MG, Chiantore MC, Privitera D, Ramoino P, Gatti MA, et al. Toxicity of metal oxide nanoparticles in immune cells of the sea urchin. Mar Environ Res. (2012) 76:114–21. doi: 10.1016/j.marenvres.2011.10.003
10. Pinsino A, Russo R, Bonaventura R, Brunelli A, Marcomini A, Matranga V. Titanium dioxide nanoparticles stimulate sea urchin immune cell phagocytic activity involving TLR/p38 MAPK-mediated signalling pathway. Sci Rep. (2015) 5:14492. doi: 10.1038/srep14492
11. Handy RD, Owen R, Valsami-Jones E. The ecotoxicology of nanoparticles and nanomaterials: current status, knowledge gaps, challenges, and future needs. Ecotoxicology. (2008) 17:315–25. doi: 10.1007/s10646-008-0206-0
12. Brunelli A, Pojana G, Callegaro S, Marcomini A. Agglomeration and sedimentation of titanium dioxide nanoparticles (n-TiO2) in synthetic and real waters. J Nanopart Res. (2013) 15:1684–93. doi: 10.1007/s11051-013-1684-4
13. Monopoli MP, Pitek AS, Lynch I, Dawson KA. Formation and characterization of the nanoparticle–protein corona. In: Bergese P, Hamad-Schifferli K, editors. Nanomaterial Interfaces in Biology. Totowa, NJ: Humana Press (2013). p. 137–55. doi: 10.1007/978-1-62703-462-3_11
14. Dai Q, Guo J, Yan Y, Ang CS, Bertleff-Zieschang N, Caruso F. Cell-conditioned protein coronas on engineered particles influence immune responses. Biomacromolecules. (2017) 18:431–9. doi: 10.1021/acs.biomac.6b01545
15. Monopoli MP, Åberg C, Salvati A, Dawson KA. Biomolecular coronas provide the biological identity of nanosized materials. Nat Nanotechnol. (2012) 7:779–86. doi: 10.1038/nnano.2012.207
16. Loosli F, Vitorazi L, Berret JF, Stoll S. Towards a better understanding on agglomeration mechanisms and thermodynamic properties of TiO2 nanoparticles interacting with natural organic matter. Water Res. (2015) 80:139–48. doi: 10.1016/j.watres.2015.05.009
17. Monopoli MP, Walczyk D, Campbell A, Elia G, Lynch I, Bombelli FB, et al. Physical-chemical aspects of protein corona: relevance to in vitro and in vivo biological impacts of nanoparticles. J Am Chem Soc. (2011) 133:2525–34. doi: 10.1021/ja107583h
18. Alaeddine S, Nygren H. Logarithmic Growth of Protein Films. In: Proteins at Interfaces II. Horbett AA, Brash JL, editors. Washington, DC: American Chemical Society (1995). p. 41–51. doi: 10.1021/bk-1995-0602.ch003
19. Slomberg DL, Ollivier P, Miche H, Angeletti B, Bruchet A, Philibert M, et al. Nanoparticle stability in lake water shaped by natural organic matter properties and presence of particulate matter. Sci Total Environ. (2019) 656:338–46. doi: 10.1016/j.scitotenv.2018.11.279
20. Noventa S, Rowea D, Gallowaya T. Mitigating effect of organic matter on the in vivo toxicity of metal oxide nanoparticles in the marine environment. Environ Sci Nano. (2018) 5:1764–77. doi: 10.1039/C8EN00175H
21. Guerrini L, Alvarez-Puebla R-A, Pazos-Perez N. Surface modifications of nanoparticles for stability in biological fluids. Materials. (2018) 11:E1154. doi: 10.3390/ma11071154
22. Klinger A, Steinberg D, Kohavi D, Sela MN. Mechanism of adsorption of human albumin to titanium in vitro. J Biomed Mater Res. (1997) 36:387–92.3. doi: 10.1002/(SICI)1097-4636(19970905)36:3<387::AID-JBM13>3.0.CO;2-B
23. Hirsh SL, McKenzie DR, Nosworthy NJ, Denman JA, Sezerman OU, Bilek MMM. The Vroman effect: competitive protein exchange with dynamic multilayer protein aggregates. Colloids Surf B Biointerfaces. (2013) 103:395–404. doi: 10.1016/j.colsurfb.2012.10.039
24. Szeto GL, Lavik EB. Materials design at the interface of nanoparticles and innate immunity, J Mater Chem B. (2016) 4:1610–8. doi: 10.1039/C5TB01825K
25. Hayashi Y, Miclaus T, Scavenius C, Kwiatkowska K, Sobota A, Engelmann P, et al. Species differences take shape at nanoparticles: protein corona made of the native repertoire assists cellular interaction. Environ Sci Technol. (2013) 47:14367–75. doi: 10.1021/es404132w
26. Lee YK, Choi EJ, Webster TJ, Kim SH, Khang D. Effect of the protein corona on nanoparticles for modulating cytotoxicity and immunotoxicity. Int J Nanomed. (2014) 10:97–113. doi: 10.2147/IJN.S72998
27. Noll H, Alcedo J, Daube M, Frei E, Schiltz E, Hunt J, et al. The toposome, essential for sea urchin cell adhesion and development, is a modified iron-less calcium-binding transferrin. Dev Biol. (2007) 310:54–70. doi: 10.1016/j.ydbio.2007.07.016
28. Cervello M, Matranga V. Evidence of a precursor-product relationship between vitellogenin and toposome, a glycoprotein complex mediating cell adhesion. Cell Differ Dev. (1989) 26:67–76. doi: 10.1016/0922-3371(89)90784-3
29. Cervello M, Arizza V, Lattuca G, Parrinello N, Matranga V. Detection of vitellogenin in a subpopulation of sea urchin coelomocytes, Eur J Cell Biol. (1994) 64:314–9.
30. Pinsino A, Thorndyke MC, Matranga V. Coelomocytes and post-traumatic response in the common sea star Asterias rubens. Cell Stress Chaperones. (2007) 12:331–41. doi: 10.1379/CSC-288.1
31. Ali N, Mattsson K, Rissler J, Marg Karlsson H, Svensson CR, Gudmundsson A, et al. Analysis of nanoparticle–protein coronas formed in vitro between nanosized welding particles and nasal lavage proteins. Nanotoxicology. (2016) 10:226–34. doi: 10.3109/17435390.2015.1048324
32. Giau VV, Park YH, Shim KH, Son SW, An SSA. Dynamic changes of protein corona compositions on the surface of zinc oxide nanoparticle in cell culture media. Front Chem Sci Eng. (2019) 13:90–7. doi: 10.1007/s11705-018-1766-z
33. Levy Y, Arbel-Goren R, Hadari YR, Eshhar S, Ronen D, Elhanany E, et al. Galectin-8 functions as a matricellular modulator of cell adhesion. J Biol Chem. (2001) 276:31285–95. doi: 10.1074/jbc.M100340200
34. Rikitake Y, Mandai K, Takai Y. The role of nectins in different types of cell–cell adhesion. J Cell Sci. (2012) 125:3713–22. doi: 10.1242/jcs.099572
35. Zito F, Nakano E, Sciarrino S, Matranga V. Regulative specification of ectoderm in skeleton disrupted sea urchin embryos treated with monoclonal antibody to Pl-nectin. Dev Growth Differ. (2000) 42:499–506. doi: 10.1046/j.1440-169x.2000.00531.x
36. Karakostis K, Costa C, Zito F, Matranga V. Heterologous expression of newly identified galectin-8 from sea urchin embryos produces recombinant protein with lactose binding specificity and antiadhesive activity. Sci Rep. (2015) 5:17665. doi: 10.1038/srep17665
37. Ribeiro AR, Gemini-Piperni S, Travassos R, Lemgruber L, Silva RC, Rossi AL, et al. Trojan-like internalization of anatase titanium dioxide nanoparticles by human osteoblast cells. Sci Rep. (2016) 6:23615. doi: 10.1038/srep23615
38. Nunes P, Demaurex N. The role of calcium signaling in phagocytosis. J Leukoc Biol. (2010) 88:57–68. doi: 10.1189/jlb.0110028
39. Walczak CE. Microtubule dynamics and tubulin interacting proteins. Curr Opin Cell Biol. (2000) 12:52–6. doi: 10.1016/S0955-0674(99)00056-3
40. Pollard TD, Blanchoin L, Mullins RD. Molecular mechanisms controlling actin filament dynamics in nonmuscle cells. Annu Rev Biophys Biomol Struct. (2000) 29:545–76. doi: 10.1146/annurev.biophys.29.1.545
41. Wloga D, Joachimiak E, Fabczak H. Tubulin post-translational modifications and microtubule dynamics. Int J Mol Sci. (2017) 18:E2207. doi: 10.3390/ijms18102207
42. Ahrens S. Extracellular Actin in Innate Immunity. Doctoral dissertation, UCL (University College London) (2014).
43. Sandiford SL, Dong Y, Pike A, Blumberg BJ, Bahia AC, Dimopoulos G. Cytoplasmic actin is an extracellular insect immune factor which is secreted upon immune challenge and mediates phagocytosis and direct killing of bacteria, and is a Plasmodium Antagonist. PLoS Pathog. (2015) 11:e1004631. doi: 10.1371/journal.ppat.1004631
44. Vega VL, Rodríguez-Silva M, Frey T, Gehrmann M, Diaz JC, Steinem C, et al. Hsp70 translocates into the plasma membrane after stress and is released into the extracellular environment in a membrane-associated form that activates macrophages. J Immunol. (2008) 180:4299–307. doi: 10.4049/jimmunol.180.6.4299
45. Ono K, Han J. The p38 signal transduction pathway activation and function. Cell Signal. (2000) 12:1–13. doi: 10.1016/S0898-6568(99)00071-6
46. Pinsino A, Matranga V. Sea urchin immune cells as sentinels of environmental stress. Dev Comp Immunol. (2015) 49:198–205. doi: 10.1016/j.dci.2014.11.013
47. Ganeshan K, Chawla A. Metabolic regulation of immune responses. Annu Rev Immunol. (2014) 32:609–34. doi: 10.1146/annurev-immunol-032713-120236
48. Du C, Anderson A, Lortie M, Parsons R, Bodnar A. Oxidative damage and cellular defense mechanisms in sea urchin models of aging. Free Radic Biol Med. (2013) 63:254–63. doi: 10.1016/j.freeradbiomed.2013.05.023
49. Schmid-Hempel P. Variation in immune defence as a question of evolutionary ecology. Proc Proc Biol Sci. (2003) 270:357–66. doi: 10.1098/rspb.2002.2265
50. Moreno-García M, Condé R, Bello-Bedoy R, Lanz-Mendoza H The damage threshold hypothesis and the immune strategies of insects. Infect Genet Evol. (2014) 24:25–33. doi: 10.1016/j.meegid.2014.02.010
51. Wolfaardt GM, Lawrence JR, Korber DR. Function of EPS. In: Microbial Extracellular Polymeric Substances. Berlin; Heidelberg: Springer (1999). p. 171–200. doi: 10.1007/978-3-642-60147-7_10
52. Canesi L, Ciacci C, Fabbri R, Balbi T, Salis A, Damonte G, et al. Interactions of cationic polystyrene nanoparticles with marine bivalve hemocytes in a physiological environment: role of soluble hemolymph proteins. Environ Res. (2016) 150:73–81. doi: 10.1016/j.envres.2016.05.045
Keywords: echinoderm, biocorona, immune-adhesome, extracellular signaling, in vitro-ex vivo model, proxy to human
Citation: Alijagic A, Benada O, Kofroňová O, Cigna D and Pinsino A (2019) Sea Urchin Extracellular Proteins Design a Complex Protein Corona on Titanium Dioxide Nanoparticle Surface Influencing Immune Cell Behavior. Front. Immunol. 10:2261. doi: 10.3389/fimmu.2019.02261
Received: 03 June 2019; Accepted: 06 September 2019;
Published: 20 September 2019.
Edited by:
L. Courtney Smith, George Washington University, United StatesReviewed by:
Vincenzo Arizza, University of Palermo, ItalySebastian Dudo Fugmann, Chang Gung University, Taiwan
Copyright © 2019 Alijagic, Benada, Kofroňová, Cigna and Pinsino. This is an open-access article distributed under the terms of the Creative Commons Attribution License (CC BY). The use, distribution or reproduction in other forums is permitted, provided the original author(s) and the copyright owner(s) are credited and that the original publication in this journal is cited, in accordance with accepted academic practice. No use, distribution or reproduction is permitted which does not comply with these terms.
*Correspondence: Annalisa Pinsino, YW5uYWxpc2EucGluc2lubyYjeDAwMDQwO2lyaWIuY25yLml0; YW5uYWxpc2EucGluc2lubyYjeDAwMDQwO2Nuci5pdA==