- 1CNRS, Laboratoire de PhysioMédecine Moléculaire, Faculté de Médecine, UMR7370, Nice, France
- 2Faculé de Médecine, Université Côte d'Azur, Nice, France
- 3Department of Pharmacy, Cardenal Herrera-CEU University, València, Spain
- 4Department of Periodontology, Academic Centre of Dentistry Amsterdam, University of Amsterdam and Vrije Univeristeit, Amsterdam, Netherlands
- 5Department of Biotechnological and Applied Clinical Sciences, University of L'Aquila, L'Aquila, Italy
- 6IRMB, INSERM, CHU Montpellier, Université Montpellier, Montpellier, France
Osteoclasts (OCLs) are key players in controlling bone remodeling. Modifications in their differentiation or bone resorbing activity are associated with a number of pathologies ranging from osteopetrosis to osteoporosis, chronic inflammation and cancer, that are all characterized by immunological alterations. Therefore, the 2000s were marked by the emergence of osteoimmunology and by a growing number of studies focused on the control of OCL differentiation and function by the immune system. At the same time, it was discovered that OCLs are much more than bone resorbing cells. As monocytic lineage-derived cells, they belong to a family of cells that displays a wide heterogeneity and plasticity and that is involved in phagocytosis and innate immune responses. However, while OCLs have been extensively studied for their bone resorption capacity, their implication as immune cells was neglected for a long time. In recent years, new evidence pointed out that OCLs play important roles in the modulation of immune responses toward immune suppression or inflammation. They unlocked their capacity to modulate T cell activation, to efficiently process and present antigens as well as their ability to activate T cell responses in an antigen-dependent manner. Moreover, similar to other monocytic lineage cells such as macrophages, monocytes and dendritic cells, OCLs display a phenotypic and functional plasticity participating to their anti-inflammatory or pro-inflammatory effect depending on their cell origin and environment. This review will address this novel vision of the OCL, not only as a phagocyte specialized in bone resorption, but also as innate immune cell participating in the control of immune responses.
Introduction
Bone-resorbing osteoclasts (OCLs) were first described 150 years ago (1). Their origin remained unclear for nearly 100 years before they were formally identified as cells of hematopoietic origin. This was evidenced thanks to the analysis of osteopetrotic mice defective in osteoclast function. Hematopoietic cell transfer from normal littermates restored OCL function in mi/mi mice and reciprocal transfer of hematopoietic cells from mi/mi mice induced osteopetrosis in normal recipient mice (2). The monocytic origin of OCLs was first demonstrated in colony assays of bone marrow cell fractions (3). From this moment, OCLs have been extensively studied to decipher the mechanisms of bone resorption leading to the identification of key factors required for OCL differentiation, fusion, bone adhesion and bone degradation activity. These studies defined a set of specific properties that cells must fulfill to be defined as bona fide OCLs, the most important being multinucleation, the expression of markers such as the tartrate-resistant acid phosphatase (TRAcP) and the capacity to degrade bone and mineralized matrix (4).
Among hematopoietic cells, OCLs belong to the monocytic family. This family of innate immune cells is characterized by its capacity to sense and respond to infections and tissue damage, its phagocytic properties and its high plasticity controlled by the tissue micro-environmental heterogeneity (5–7). Abundant literature addressed the origins and roles of monocytes (MNs), macrophages (Mϕs), and dendritic cells (DCs). Nowadays, it is clearly established that each of these populations includes distinct sub-groups that have specific origin and functional properties ranging from inflammatory to immune suppressive effects (8, 9). However, despite their common origin, the potential implication of OCLs as innate immune cells has been neglected for a long time. The immune face of OCLs emerged only 10 years ago when costimulatory signals mediated by ITAM motifs involved in immune cell activation were shown to be essential for OCL differentiation (10–12). This was further emphasized by the identification of the important link between DCs and OCLs through the ability of DCs to differentiate into bone-resorbing OCLs under pathological conditions (13, 14) (Table 1).
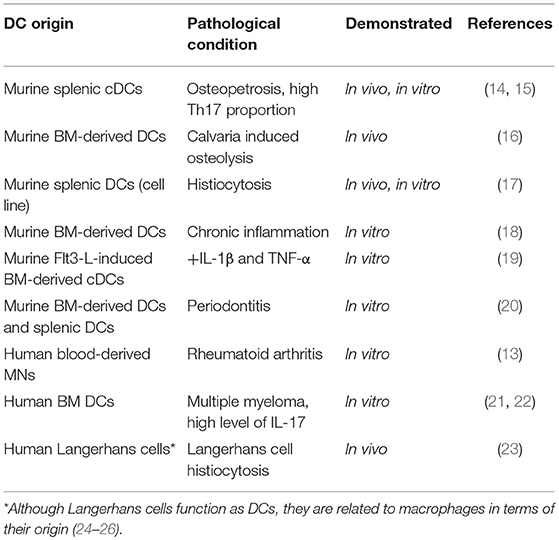
Table 1. Pathological conditions associated with inflammatory osteoclasts differentiated from dendritic cells.
In fact, OCLs share many similarities with Mϕs and DCs in their origin and function (Figure 1). Like Mϕs in tissues, OCLs are essential to maintain bone homeostasis and remodeling in steady state and to support bone healing after bone damage. Beside bone matrix resorption, they are able to take up apoptotic cells, calcium-phosphate particles or latex beads (27–31). More recently, OCLs have been shown to process, present and cross-present antigens resulting in T cell activation (18, 32, 33). They also produce cytokines and immunomodulatory factors that affect immune responses (34–36), as described below. Moreover, like their monocytic counterparts, OCLs display phenotypic heterogeneity and may arise from different progenitors depending on their environment, the stimuli they receive and their developmental stage (18, 37, 38). In particular, pathological conditions associated to inflammation or cancer provide molecular and cellular signals that stimulate specific monocytic subsets to differentiate into OCLs. Despite this heterogeneity in their origin and environment, OCLs are largely considered as a single population of cells. Consequently, thus far, under pathological conditions OCLs have been investigated for their increased or decreased differentiation and resorptive function but almost never with regard to the implication of different OCL subsets. Moreover, the immunological function of OCLs remains poorly explored. This review addresses the state-of-the-art of this novel vision of the OCL not just as a bone-resorbing cell but also as a cell having immune capacities.
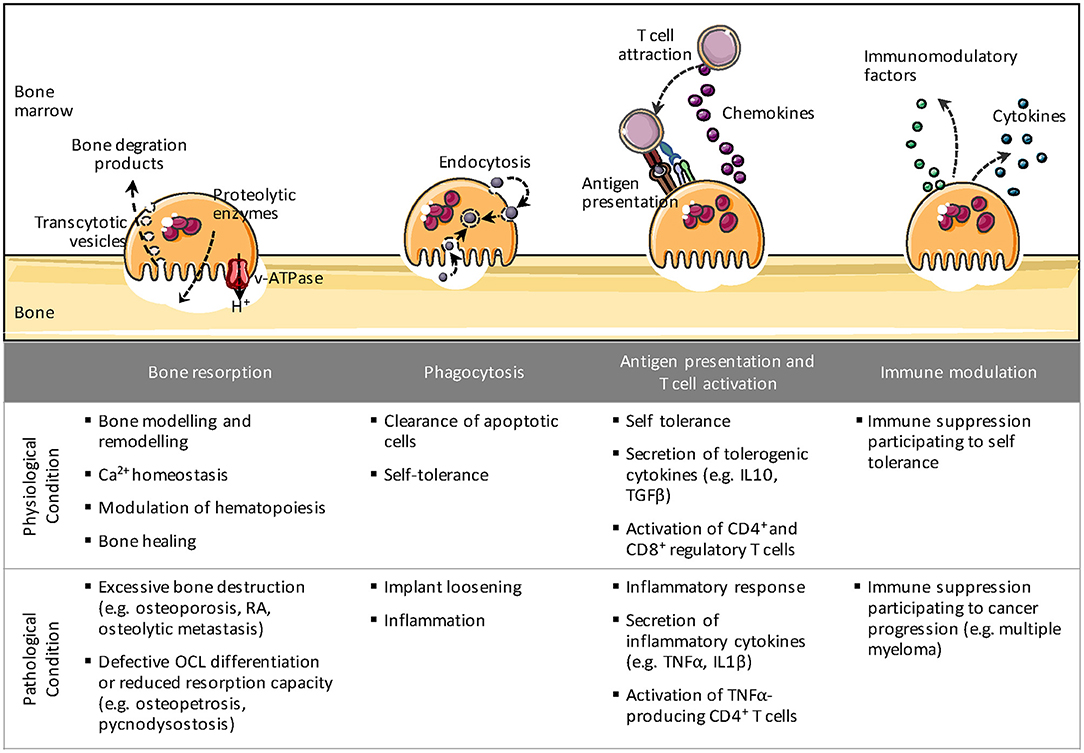
Figure 1. The different immune-related roles of osteoclasts. Besides bone resorption, osteoclasts share many properties with their precursor cells such as phagocytosis, antigen presentation, and immune modulation. These immune-related functions may possess divergent functionalities under physiological and pathological conditions.
Diversity in Osteoclast Origin
Bone Marrow Osteoclast Progenitors
Whereas, MN, Mϕ, and DC origin has been widely explored, the origin of OCLs remained more elusive and OCLs are usually forgotten in hematopoietic lineage trees. However, in adults a common early bone marrow (BM) progenitor for OCLs and other monocytic cells (Mϕ/OCL/DC progenitor, MODP) has been identified downstream of the granulocyte/Mϕ progenitor (GMP) (Figure 2) (39, 40). In human, CD11b−CD34+c-KIT+FLT3+IL-3Rαlow BM cells are common progenitors for Mϕs, DCs, OCLs and granulocytes. They give rise to CD11b−CD34+c-KIT+FLT3+IL-3Rαhigh cells that are restricted to Mϕ, DC, and OCL differentiation and represent <0.5% of BM cells (39). In mouse, the BM CD11b−/lowc-kit+CD115+ fraction contains a common precursor for Mϕs, OCLs, and DCs also representing <0.5% of BM cells (41–44). Within this population, the use of CD27 and Flt3 markers further discriminates subsets of oligopotent progenitors for Mϕ/OCL/DC development (CD27+Flt3+) vs. bipotent progenitors for Mϕ/OCL development (CD27low/−Flt3−) (40, 45). Moreover, analysis of mouse BM myeloid fractions revealed that early blasts (CD31hiLy6C−), myeloid blasts (CD31+Ly6C+) and MNs (CD31−Ly6Chi) have different capacity to differentiate into OCLs (46). BM myeloid blasts that express higher CD115 levels differentiate more efficiently and faster than early blats and MNs (46). Indeed, while c-kit is down regulated during murine OCL differentiation, CD115 expression is maintained (44), which is essential for osteoclastogenesis since binding of CD115 to its ligand M-CSF increases the expression of RANK, allowing further differentiation into OCLs under RANKL stimulation (41). Moreover, analysis using BrDU incorporation in mice revealed that quiescent CD115+ RANK+ progenitors represent committed OCL progenitors able to circulate and settle down in the bone (47, 48).
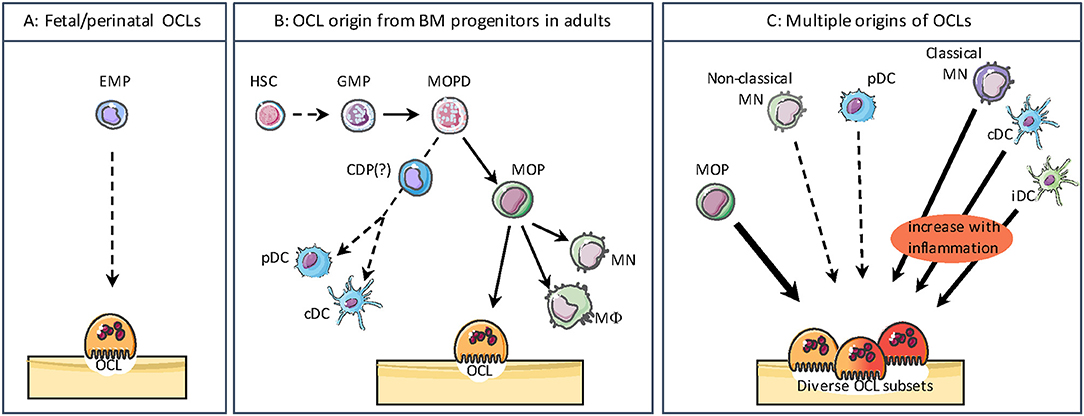
Figure 2. The different origins of osteoclasts. (A) During the embryonic and postnatal period, OCLs differentiate from the embryonic erythro-myeloid progenitor (EMP) lineage. (B) In adults, bone marrow progenitors for osteoclasts (OCLs) and other monocytic cells such as monocytes (MN), macrophages (MΦ), as well as conventional/classical dendritic cells (cDCs) and plasmacytoid DCs (pDCs) share common progenitors downstream of the granulocyte/MΦ progenitor (GMP). HSC, Hematopoietic Stem Cell; MOPD, MΦ/OCL/DC progenitor; CDP, common DC progenitor; MOP, MΦ/OC progenitor. The divergence of CDP from MODP is not clearly evidenced. (C) OCLs have multiple origins depending on their environment. Beside their origin from MOP, in pathological conditions OCLs can also arise from classical MNs, cDCs and inflammatory DCs (iDC). OCLs differentiation from non-classical and pDCs is much less efficient. These different sources of OCLs result in a heterogeneity as observed for other monocytic cells.
Interestingly, the origin of OCLs from BM HSCs and downstream progenitors appears to be restricted to adults. This was very recently demonstrated by Jacome-Galarza et al. who depleted RANK or CD115 expression in BM-HSC progenitors (using Csf1rcre mice) or in BM-HSC and erythro-myeloid progenitors (EMP) (using Flt3cre mice). Depletion of these genes in embryonic EMP resulted in osteopetrosis in newborns, whereas only adults were affected by specific depletion in HSC progenitors (38). This study elegantly demonstrated for the first time that OCLs involved in fetal bone formation and tooth eruption are originating from the same progenitors as tissue Mϕs and differ thereby from OCLs arising in adults (Figures 2A,B) (38, 49).
Therefore, the interplay between OCLs and other monocytic cells appears much more puzzling than the existence of a common BM progenitor. In vitro, OCLs can be generated in the presence of RANKL and M-CSF from hematopoietic cells originating from many tissues. BM in mouse and blood in human are major sources of OCL progenitors, but OCLs can also be obtained from hematopoietic cells from the liver, spleen, thymus and lymph nodes, suggesting a high heterogeneity of potential OCL precursors (3, 50–54). Indeed, in addition to their differentiation from BM progenitors, OCLs can also arise from cells already engaged in the MN or DC pathways (3, 13, 14, 55, 56). As described below, this process is associated with pathological conditions related to bone destruction making OCL differentiation more complex and dynamic than expected. Thus, there is a large variability in OCL precursor cells depending on the signals they receive from their normal or pathological environment. These observations strongly support that OCLs do not represent a single and homogeneous population but that they display the same heterogeneity in their origin and phenotype as other monocytic cells (Figure 2C).
Osteoclasts and Monocytes
Monocytes are innate immune cells characterized by a great level of plasticity and found in all tissues. Depending on the environmental cues, they can differentiate into DCs, Mϕs or OCLs. Being involved in both inflammation and bone resorption, MNs represent key regulators of bone tissue homeostasis. Moreover, they are heterogeneous and comprise several subtypes already committed to different functions. Knowledge on MNs has evolved a lot over the past decade thanks to new technologies such as fate mapping and single-cell RNA sequencing (scRNAseq). Historically, they were considered as an intermediate status in the peripheral circulation, between myeloid precursors from the BM and Mϕs in tissues. Now, we know that certain tissue-resident Mϕs are generated independently of MNs during early phases of embryogenesis and that fetal MNs derive from multipotent erythro-myeloid progenitors. Later and throughout life, MNs are generated from the HSC-derived hematopoiesis (49, 57), as described for OCLs (38). In healthy conditions, MNs are present in central (BM) and peripheral (spleen) reservoirs and engrafted into certain resident macrophage pools. They are not only involved in the repopulation of tissue Mϕ and DC compartments but also contribute to the establishment and resolution of local inflammatory reactions and participate in the innate immune surveillance of the organism (58). Under pathological conditions, they are rapidly mobilized in large numbers and recruited to the inflamed tissue where they display both inflammatory and pro-resolving properties to allow tissue repair. This last step must be transient. If monocyte numbers in tissues are not properly regulated and persist overtime, their actions become pathogenic for targeted tissues (59). In particular, massive recruitment of MNs in the BM is associated with increased OCL formation and bone destruction (56, 60).
Two main subsets of MNs exist both in mouse and human (61, 62). In mice, the “classical” monocytes are characterized by the combination of specific surface markers Ly6ChighCCR2+CX3CR1lowCD62L+Gr1+, and were previously named inflammatory monocytes because they can differentiate into inflammatory Mϕs and inflammatory DCs (63, 64). The “non-classical” MNs are characterized by the Ly6ClowCCR2lowCX3CR1+CD62L−Gr1− surface markers and are also named patrolling MNs because they survey endothelial cells and surrounding tissues for damage or viral infection. Their human counterparts are CD14+CD16− and CD14lowCD16+, respectively (61, 65). A recent scRNAseq analysis of human blood confirmed that classical and non-classical MN subsets represent two distinct clusters (66). Several genetic mouse models of deletion of transcription factors, cytokines and chemokines, together or not with acute or chronic inflammatory challenges, have been used to delineate functional heterogeneity of the Ly6Chigh and Ly6Clow monocyte subsets in vivo. Only few studies however addressed their respective capacity to differentiate into OCLs.
It is now well established that mature mouse Ly6Chigh MNs differentiate into the BM from unipotent common monocyte progenitors, with an intermediate Ly6ChighCXCR4+ pre-monocytic step (67). Under steady state conditions, mature Ly6Chigh MNs constantly egress from the BM in a CCR2-dependent manner (68) following circadian oscillations (69) and circulate for 1 day in the blood. Then, a minority (~1%) of these MNs convert into non classical Ly6Clow MNs that have longer circulating lifespans (~7 days), while the vast majority (~99%) of Ly6Chigh MNs leave the circulation and replenish specific pools of tissue resident Mϕs (70–72). For specific peripheral inflammatory responses to infections or tissue damages, the fate of mouse Ly6Chigh MNs is identical. Differences rely on the speed and amplitude of the mobilization from BM and spleen to target sites, the kinetic being faster and larger numbers being produced, leading to blood monocytosis as early as 4 h after endotoxin challenge (73). Additionally, differences also rely on the fraction of blood monocyte-derived Mϕs that replenishes tissue-resident Mϕs after recruitment (71). Mouse Ly6Chigh MNs are precursors of longer-lived Ly6Clow MNs that express higher levels of CX3CR1 (74) and continuously patrol the luminal side of the vasculature in a CX3CR1 and LFA-1/ICAM1-dependent crawling manner (58). Under physiological conditions, Ly6Clow MNs are the endothelium housekeepers, playing a key role to control endothelium integrity by scavenging luminal microparticles, recruiting neutrophils for focal necrosis of endothelial cells, phagocytizing cellular debris (75). Upon bacterial infection, Ly6Clow MNs secrete IL-10 and are recruited to tissue where they more likely differentiate into alternatively activated Mϕs, contributing to tissue repair. Ly6Clow monocytes can also promote tolerance to self-antigens contained in apoptotic cells through a PD-L1-dependent mechanism and thanks to their high capacity to phagocyte apoptotic cells in vivo (76). Overall, the multiple capacities of both MN subsets to differentiate into either regulatory or inflammatory mature Mϕs or DCs depend on the inflammatory signal and tissue microenvironment. Interestingly, both mouse MN subsets can go back to the BM thanks to a CXCR4-dependent signal (67). The respective role of Ly6Chigh and Ly6Clow MNs on bone turnover remain yet to be established.
Since MNs constitute a source of OCLs, it is expected that both MN subsets display OCL differentiation potential. Although the culture conditions used in vitro to monitor OCL differentiation diverge between studies, it appears that mouse OCLs develop from BM CD11b−/lowLy6Chigh monocytic progenitors (as described above) and from blood CD11bhighLy6Chigh MNs. In the BM, CD11b−/lowLy6Chigh monocytic progenitors are more prone than CD11b+ MNs to differentiate into OCLs (43) because of the negative role of CD11b and β2-integrin signaling on OCL differentiation (77). In vitro comparative studies based on BM treatment with various cytokines demonstrated that Ly6Chigh MNs were far more efficient than Ly6Clow monocytes to differentiate into mature OCLs (78). Importantly, the BM CD11b−/lowLy6Chigh population also displays an OCL differentiation capacity in vivo and is expanded in inflammatory arthritis models (79). In particular, the CX3CR1+ fraction of these cells is highly enriched in OCL precursors (79). In-depth phenotypic characterization allowed to further dissect CD11b−/lowLy6Chigh cells into three different populations with high osteoclastogenic potential based on the expression of the phenotypic marker CD117 (c-Kit) (43).
In the blood, the mouse Ly6Chigh MN subset also represents the major precursor cell population of OCLs (Figure 2C). Indeed, in vitro Ly6Chigh MNs are more efficient than the Ly6Clow subset to differentiate into TRAcP positive cells (55). In the context of inflammatory arthritis, disease severity is associated with Ly6Chigh blood monocytosis, and Ly6Chigh MNs more specifically migrate to the inflamed joints and contribute to bone erosion due to their excessive differentiation into OCLs (56). Importantly, in vivo delivery of therapeutic molecules to Ly6Chigh MNs, but not to Ly6Clow MNs, markedly interferes with pathogenic bone erosion in experimental arthritis, suggesting that the classical subset represents a candidate cell target for anti-osteoclastogenic strategy design (56). In human, OCLs generated from peripheral blood MNs originate from the classical CD14+CD16− subset, and not from the CD16+ subset, in an integrin β3-dependent manner (80, 81). Later studies refined this view showing that while the different human MN subsets can differentiate into OCLs when cultured on plastic, OCLs are predominantly formed from classical MNs when cultured on bone slices (82). In the context of inflammatory bone diseases, the distribution of MN subsets is skewed toward a higher proportion of CD14+CD16+ MNs (83). Interestingly, it seems that in these pathological conditions, CD14+CD16+ MNs are more prone to differentiate into OCLs than in healthy conditions, as demonstrated in psoriatic arthritis patients (84).
The origin of OCLs from MN progenitors or MNs is likely to be dictated by the BM cell environment. Indeed, monocytic OCL precursors show different expression level of cytokines/growth factors leading to different effect of inflammatory cytokines. In human, among the three MN subsets, only the CD14high CD16+ intermediate subset responds to IL-17 by forming larger OCLs having higher resorption capacity than in absence of this cytokine (82). In mouse, BM CD31highLy6C− early blasts, CD31+Ly6C+ myeloid blasts and CD31−Ly6Chigh MNs are differently affected by their environment. When assessing life span, IL-1β enhance OCL formation especially of myeloid blasts, which have rapidly formed and have a short life span, while OCLs derived from CD31−Ly6Chigh MNs are formed a later stage and have a longer life span (85). Remarkably, M-CSF pretreatment of myeloid blasts or TNFα pretreatment of MNs before addition of RANKL inhibit osteoclast formation from CD31−Ly6Chigh MNs but not from early blast or myeloid blast despite the expression of RANK (86, 87). These cells were able to regain their osteoclastogenesis capacity when cultured on bone slices, revealing the importance of the bone attachment and signaling in OCL differentiation. This is further supported by the finding that collagen specific motifs are ligands for OSCAR, a costimulatory receptor induced by RANKL and essential for OCL differentiation (88).
Osteoclasts and Dendritic Cells
Dendritic cells have been identified by Steinman and Cohn 45 years ago (89). More than through their phenotype and surface marker expression, DCs are also defined by their functional specificity. Being located in most tissues where they represent 1–5% of the hematopoietic cells, they act as sentinels of the immune system capturing and processing antigens and instructing adaptive immune cells (84). Contrasting with MNs and Mϕs, they have the unique capacity to migrate to the T cell zones of lymphoid organs where they present or cross-present antigens thanks to their expression of major histocompatibility complexes (MHC)-I and -II and activate naive T cells.
As MNs, DCs represent a heterogeneous population of cells. In mouse, DCs that reside in lymphoid organs are composed of CD8+ and CD8− conventional/classical DCs (cDC) and IFNα-producing plasmacytoid DCs (pDCs). Equivalent subsets are also present in human, namely CD1c+ (BDCA1) and CD141+ (BDCA3) cDCs and CD303+ (BDCA2) pDCs (90, 91). Plasmacytoid DCs have the capacity to produce high amounts of IFNα in response to viral and foreign nucleic acids stimulation and to prime naive T cells against viral antigens (92).
In murine non-lymphoid tissues, the two main cDC subsets are CD11b+ and CD103+CD11b− (90). These cDCs have a tremendous capacity to permanently sense their environment and uptake antigens in tissues and blood. They express high levels of MHC complexes and the machinery to process and present antigens, they have very high migratory capacity to the lymph nodes mainly governed by the chemokine receptor CCR7 (93) and they are highly efficient in naive T cell activation and polarization. By driving T cell differentiation toward different T helper (Th) subsets or regulatory T (Treg) cells depending on their activation and the cytokine they produce, DCs have the capacity to induce immune responses against foreign antigens or to stimulate self-antigen tolerance (94, 95).
The majority of splenic and lymphoid organ DCs are renewed from BM progenitors (96, 97) and Flt3L play a major role in their homeostasis (98, 99). In human and mouse, cDCs and pDCs arise from BM Flt3+CD115+c-kitlow/int common dendritic progenitors (CDP), downstream from progenitors common to MNs, DCs, Mϕs and OCLs (39, 97, 100, 101). DCs precursors egress from the BM and migrate to lymphoid organs and tissue to differentiate into immature cDCs (102). In contrast, pDCs are generated in the BM and then disseminate to lymphoid tissues (102). Upon inflammation or infection, inflammatory DCs are transiently generated from classical Ly6Chigh MNs that are recruited into inflamed tissues, and drive T cell activation in the draining lymph nodes (63, 103–105). Contrasting with cDCs, inflammatory DCs are not depend on Flt3L since they are generated in Flt3L−/− mice (106). In vitro, inflammatory DCs can be generated from MNs or BM cells in the presence of GM-CSF and IL-4 (105, 107). However, in vivo studies in knockout mice identified M-CSF receptor (CD115) as a major factor controlling their development (108). In addition to naive T cells in lymphoid organs, inflammatory DCs can also activate T cells in the inflamed tissues, in particular memory T cells (109). This function appears to be dependent on the antigen dose and the severity of inflammation (105, 106).
Over the past decade, different groups have reported on the capacity of DCs to give rise to OCLs, as described below. This was surprising as DCs are considered as fully differentiated cells and have been described to have a short life (~1.5 to 3 days) (110, 111). However, more recent data revealed that about 5% of DCs still maintain a proliferation capacity (96, 112–114). DC survival is increased in the presence of lymphotoxin LTα1β2 (112) and RANKL (115, 116). Moreover, mature DCs can undergo further proliferation and differentiation into regulatory DCs when they are in contact with splenic stromal cells (117). These observations support a high plasticity in the fate of DCs depending on their environment.
In regard of the huge number of publications dealing with DCs in secondary organs or even in tissues, very few are focused on mature BM DCs. As in other tissues, DCs represent a small population of BM cells (1–2%) expressing high levels of CD103 (118, 119). They are mainly located in the perivascular region where they form clusters, interact with T cells and provide survival signals to B cells (119). As BM is a major reservoir of memory T cells, the presence of DCs in this organ is thought to contribute to reactivation of these memory T cells (120, 121). In contrast, naive T cell activation is supposed to be restricted to secondary lymphoid organs. However, and surprisingly, BM DCs also have the capacity to present and cross-present antigens to naive CD4+ and CD8+ T cells and to activate them as efficiently as DCs from lymphoid organs (122, 123). This T cell-priming activity of BM DCs is independent of spleen and lymph nodes as it was also observed in mice lacking these organs (122). These observations revealed that the BM is not just a primary lymphoid organ but that it shares some features with secondary lymphoid organs.
The first demonstration of the differentiation of OCLs from DCs was evidenced using human immature DCs generated in vitro from blood CD14+ CD16low/− MNs. In response to M-CSF and RANKL, these cells differentiate as efficiently as MNs into bone-resorbing OCLs (13). Human MN-derived DCs differentiate faster than MNs and form OCLs having more nuclei than from MNs (13). This was later confirmed with murine DCs (Figure 2C). Splenic cDCs were shown to efficiently differentiate into OCLs in the presence of RANKL and M-CSF and among them the CD8− subpopulation is the more efficient OCL precursor, whereas OCL differentiation from pDCs is less efficient and takes longer than from cDCs (14). More importantly, this differentiation pathway was also demonstrated in vivo using osteopetrotic oc/oc mice (14, 15) in which OCLs are abundant but inactive (50). Transfer of cDCs from normal mice into oc/oc mice restored bone resorption and improved mice survival demonstrating that OCLs can differentiate from DCs in vivo and that osteopetrosis can be treated not only by hematopoietic stem cell transplantation but also by DCs infusion (14, 15). Interestingly, DC-derived OCLs and MN-derived OCLs show an equivalent expression of the main OCL markers (124) and both have the same capacity to resorb bone (13, 14) demonstrating that both populations correspond to bona fide OCLs.
As described above for MNs, the differentiation of OCLs from DCs is modulated by their environment. First, this differentiation has been confirmed in vitro and in vivo in a number of different pathologies related to inflammation or cancer, but never in a healthy context (125) (Table 1). Indeed, in vitro, TNFα, IL-1α, IL-17 or synovial fluid from arthritic patients enhance the differentiation of human MN-derived DCs into OCLs (13, 19). In vivo, the presence of Th17 cells or high levels of RANKL are required to induce the DC-to-OCL differentiation as demonstrated using osteopetrotic oc/oc mice or in the context of multiple myeloma (14, 21).
From all these observations, it appears that while adult OCLs differentiate from BM progenitors in steady state, they can also arise from differentiated MNs and DCs in pathological conditions. The origin of OCLs is therefore depending on the developmental stage, the BM environment and the BM recruitment of MNs or DCs. Moreover, OCLs are able to constantly incorporate new nuclei and split off other groups of nuclei both in vitro (126) and in vivo1 (38). Parabiosis experiments between Csf1rcre;Rosa26LSL−YFP and Csf1rcre;Rosa26LSL−tdTomato mice revealed that 0.5–2% of OCLs acquire new nuclei per day and can persist several weeks in vivo (38). Thus, depending on the cells that are present at their vicinity, it is very likely that OCLs are formed by a mix of different OCL progenitors instead of originating from a pure progenitor population, reflecting a high flexibility and the capacity to rapidly adapt to different pathological circumstances.
The Immune Function of Osteoclasts
A number of studies have been conducted on the regulation of OCLs by T cells under inflammatory conditions. Inflammation and bone destruction were observed to occur side by side, as shown for example for rheumatoid arthritis (RA) (127–129), periodontitis (130–133) or inflammatory bowel disease (IBD) (134, 135). During inflammatory states, it has been described that OCL progenitors respond to several interleukins produced by activated CD4+ T cells such as RANKL, TNFα, and IL-17, including in human (52, 116, 136–139). Among CD4+ T cells, only Th17 cells have been shown to induce or enhance OCL differentiation (56, 129, 131, 134–136). During inflammatory conditions, IL-17-expressing Th17 cells were also associated with increased bone destruction and osteoclastogenesis by up regulating RANK in OCL progenitors and by inducing RANKL expression in osteoblasts (60, 137, 140–142). Moreover, they increase the expression of monocyte-attractant chemokines such as MCP1 and MIP1α in osteoblasts and induce in vivo the recruitment of OCL monocytic progenitors in the BM (60). In contrast, other T cell-derived cytokines such as IFNγ, IL-4, IL-10, IL-12, and IL-18 as well as regulatory T cells were reported to have a negative effect on osteoclastogenesis (137, 143–146).
Besides numerous studies investigating the potential effect of T cells on OCLs, the reciprocal contribution of OCLs to immune modulation and the understanding of immune responses caused directly by OCLs, as for instance as antigen-presenting cells, remained undiscovered for a long time. Genome-wide expression analysis strengthened the idea that OCLs have an immune function. Indeed, comparative micro-array transcriptomic analysis revealed that in vitro differentiated human OCLs are transcriptionally closer to DCs than to MNs (124). Furthermore, during their in vitro differentiation process, murine OCLs arising from CD11b+ BM cells up regulate sets of genes involved phagocytosis and immune responses (147). More recently, studies emphasized OCLs as true antigen-presenting cells to regulate and control T cell activation as well as their ability to initiate T cell responses in an antigen-dependent manner (18, 32, 33). Considering OCLs as cells having an immune function besides the classical bone resorption activity is a very new concept that is elaborated in more detail in the following sections.
Bone Resorption and Phagocytosis
The best-known OCL function is bone resorption and OCLs are regarded as professional phagocytes specially adapted to resorb bone. Contrasting with classical phagocytosis in which internalized material is degraded in intracellular endo-lysosomal vesicles, this unique property of OCLs is accomplished through an extracellular mechanism that makes the OCL a peculiar “giant macrophage” with molecular machinery distinct from any other cell type. After mature OCLs have polarized onto the bone surface, a tight and dynamic seal segregates the underneath extracellular space (later becoming the resorption lacuna) from the rest of the extracellular bone marrow space (148). A massive extracellular proton and enzyme secretion occurs through exocytosis of lysosomes at the most peripheral area of the ruffled border (Figure 3) (4, 149). Lysosomal membranes are inserted into this plasma membrane domain, introducing here the vacuolar H+-ATPase (proton pump) and the ClC7 2Cl−/1H+ antiporter (chloride channel type 7), while the acidic hydrolases are released into the resorption lacuna microenvironment. Thus, the resorption lacuna has a composition very similar to the one of intracellular endosomes, with low pH, high calcium concentration and abundance of acidic hydrolyses (150, 151). The ruffled border shares membrane characteristics of late endosomes (152). When bone resorption is completed, the resorption lacuna is filled mainly with calcium and phosphate ions as well as small collagen type I fragments. Dynamic studies showed that the inner uptake domain of the ruffled border is specialized in the clathrin-mediated endocytosis of the degraded bone matrix (149, 153, 154) that allows internalization of nanosize particles (155). Then, by a small GTPase-dependent transcytosis trafficking, vacuoles cross the osteoclast cytoplasm to reach the functional secretory zone opposite to the ruffled border (153). Through this domain, the degraded bone products are released in the extracellular microenvironment and taken up by the vascular stream. Finally, although the OCL transcytosis function has been elegantly demonstrated by various groups, this does not exclude that degraded matrix components could leak out from the resorption lacuna when the tight seal of this environment is loosened and the OCL moves away from the previous resorption site to reach a new site where it re-polarizes and starts a new resorption cycle (156).
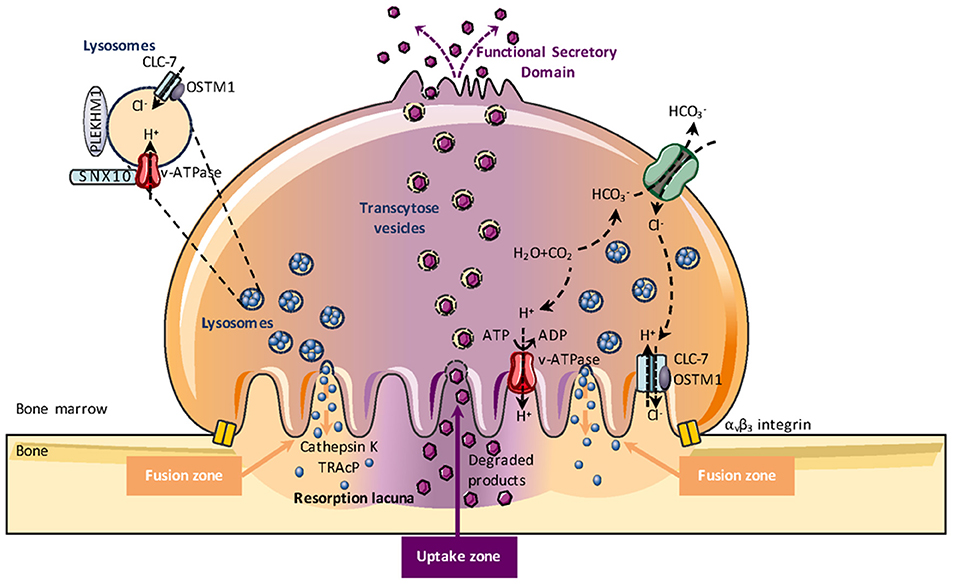
Figure 3. Osteoclast-mediated bone resorption. Bone degradation requires the extracellular secretion of lysosomal enzymes, such as TRAcP (Tartrate-resistant acid phosphatase) and cathepsin K into the resorption lacuna. This exocytosis of lysosomes occurs in the peripheral region of the ruffled border (fusion zone) and is guided by PLEKHM1, OSTM1, and SNX10. Proton pumps (v-ATPase) and the CLC7-antiporter are relocated at the ruffled border plasma membrane of bone-resorbing OCLs and mediate extracellular acidification for bone demineralization during bone resorption. The v-ATPase transports protons obtained by carbohydrase-dependent hydration from CO2 to H2CO3 into the resorption lacuna while HC is excreted via the Cl−/HCO3 exchanger. Molecular degradation products generated during bone resorption are released through the central region of the ruffled border (uptake zone). Through transcytosis trading, vacuoles are able to cross the OCL cytoplasm and exit the OCL from the so-called “functional secretory zone” at the basolateral plasma membrane into the vascular space.
Beside their specialization in bone resorption, OCLs display the classical phagocytic properties shared by monocytic cells. Phagocytosis is a key form of endocytosis for the clearance of large particles (with few μm range) such as pathogens, microorganisms and abnormal or dead cells and it is essential for tissue repair and modeling, and for fighting against infection (31, 155). Phagocytes express a set phagocytic receptors belonging to non-opsonic receptors (such as scavenger receptors or c-lectin and lectin-like receptors) (157, 158) and to opsonic receptors (such as complement receptors and Fc receptors) (159, 160) able to recognize different microbial components and altered cells. This binding creates a phagocytic synapse and invagination of the cell membrane, activates signaling pathways, and leads to the engulfment of the particles in phagosomes, a process associated with a high dynamic cytoskeleton remodeling (161). The phagosome undergoes maturation and fuses with lysosomes to form a phagolysosome having the capacity to degrade the ingested particle (31, 161). As the resorption lacuna, the phagolysosome is characterized by a low pH (4.5 to 5) maintained by V-ATPases and ClC family antiporters, and contains hydrolytic enzymes, bactericidal proteins, cationic peptides, and oxidants (162). Depending on the nature of the phagocytic cells, degradation of the ingested particles is different: while proteolysis is extensive in macrophages, it is more partial in DCs to allow the degraded peptides to associate with the MHC complexes for antigen presentation (31).
As other monocytic cells, OCL express a number of factors that play crucial roles in phagocytosis (30, 147). In OCLs, phagocytosis may provide an additional mechanism participating in degradation of calcium-phosphate (CaP) materials together with resorption (29, 163). Indeed, ultrastructural microscopy analysis revealed that OCLs are able to engulf in endophagosomes CaP crystals that undergo intracellular degradation instead of degradation in the resorption lacuna (29). They also internalize other particles such as latex beads or polymethylmethacrylate particles while they maintain their bone resorbing activity (164). This mechanism probably further increases the capacity of OCLs to degrade large particles that are difficult to resorb through classical bone resorption associated with clathrin-dependent endocytosis (29, 165). It can also be involved in implant loosening where phagocytosis of wear particles derived from implants is associated with bone destruction (29, 164).
Interestingly, phagocytosis can occur in glass-seeded OCLs that are not polarized, showing that this process is not necessarily associated with bone resorption (166). This has been demonstrated for phagocytosis of damaged cells. In vitro, OCLs have the capacity to recognize and engulf apoptotic bone cells, such as chondrocytes and osteocytes (30, 167, 168) but also other cell types as shown for instance for glutaraldehyde-fixed red blood cells (169) and apoptotic thymocytes, as efficiently as macrophages (30). In vivo, this mechanism may participate in regulating inflammation and autoimmunity by clearing apoptotic bone cells that are embedded in a matrix that only OCLs have the unique capacity to resorb (30). These data strongly support that bone resorption is not the sole function of OCLs and that these cells are participating more largely to the immune regulation in the BM.
Osteoclasts and Antigen Presentation
Beside this unique resorption function in bone homeostasis and repair, the close relationship of OCLs with MNs, Mϕs, and DCs raises the question of the potential immune function of OCLs. The fact that OCLs respond to immune signals and that the bone and the immune system are in close proximity to each other and interact through cellular and molecular exchanges led to the conclusion that OCLs are more than just simple bone resorbing cells (36, 170–172). Indeed, it would not be surprising that OCLs maintain and share similar functions to monocytic cells, in particular DCs that are known to be professional antigen presenting cells (APCs).
Professional APCs are defined as immune cells able to process and present antigens via major histocompatibility complex (MHC), and activate naive T cells through interaction between MHCs and T cell receptors (TCRs), costimulatory signals and cytokine production. Antigens are recognized by T cells in the form of short peptides that have been processed and presented on the APC surface bound to MHC class I or MHC class II molecules (173–175). While MHC-I are ubiquitously expressed on all nucleated cells to present intracellular antigens, MHC-II molecules are constitutively expressed on professional APCs to present exogenous antigens (176, 177). However, only cDCs have the feature to cross-present antigens through MHC-I complexes to prime CD8+ T cells. TCR on CD8+ and CD4+ T cells binds to the MHC-I and MHC-II complexes, respectively, in order to initiate naive T cell activation and to trigger TCR signaling (178, 179). A crucial step in T cell activation is mediated by co-stimulatory molecules such as CD80 (B7.1) and CD86 (B7.2) that determine the functional outcome of the TCR signaling (180, 181). Subsequently, APCs start to secrete cytokines that control the differentiation of activated T cells into effector T cell subsets such as Th1, Th2, or Th17 subsets to promote inflammatory responses or regulatory T cells that regulate immunosuppressive responses (181–184).
In addition to DCs, professional APCs also comprise B cells and Mϕs that have all constitutive expression of MHC-II (173, 185, 186). Other immune cells (including basophils, innate lymphoid cells, neutrophils, mast cells) and non-hematopoietic cells (including endothelial cells, mesenchymal stromal, epithelial cells) have been reported to upregulate MHC-II upon stimulation (187). But the absence of constitutive MHC-II expression, the lack of phagocytic function and a less evident capacity to prime naive T cells in an antigen-dependent manner make these cells atypical APCs (187). Rivollier et al. first showed that human OCLs in vitro differentiated from blood MN-derived DCs constitutively express HLA-DR and CD86 (13). This was further confirmed in murine OCLs differentiated in vitro from MNs or DCs (18), but also in vivo in bone marrow OCLs (18). The assumption that mature OCLs could act as APCs was equally reinforced by others (Figure 4). Li et al. observed that similar to DCs, human OCLs express MHC-I and II as well as the co-stimulatory molecules CD80, CD86, and CD40 (33). They also showed that OCLs are able to engulf soluble antigens and to present them on their cell surface demonstrating that OCLs can function as APCs. Additional cytokine assays confirmed that OCLs, similar to DCs, express IL-1β, IL-6, IL-10, transforming growth factor-beta (TGF-β), and TNFα further indicating that they meet the demands as APCs (18, 33). Grassi et al. strengthened this hypothesis by showing that OCLs derived from human monocytic precursors expressed MHC-II and upregulated the expression of CD80 and CD40 during their differentiation (34). Ibáñez et al. have explored this function in murine OCLs using DQ-OVA, a BODIPY-conjugated form of ovalbumin that becomes fluorescent after endolysosomal degradation. They showed that OCLs differentiated in vitro from MNs and from DCs both express MHC-II and costimulatory molecules and are able to process and present ovalbumin at least as efficiently as DCs (18). Furthermore, using I-Abβ-GFP knock-in mice that express a fusion protein between GFP and the I-Abβ subunit of the MHC-II complex, they demonstrated that OCLs constitutively express MHC-II in vivo (18). Lastly, OCLs were also shown to cross-present antigens via MHC-I, a feature considered to be specific for cDCs (32). Interestingly, OCLs process and present antigens even when they are cultured on plastic or when they are not able to resorb bone as shown for OCs from oc/oc mice (18). In addition, OCLs that process antigens still retain their bone-resorbing capacity, revealing that antigen presentation and bone resorption are independent functions in OCLs (18). These findings expanded the field of osteoimmunology by directly connecting OCLs to the immune system.
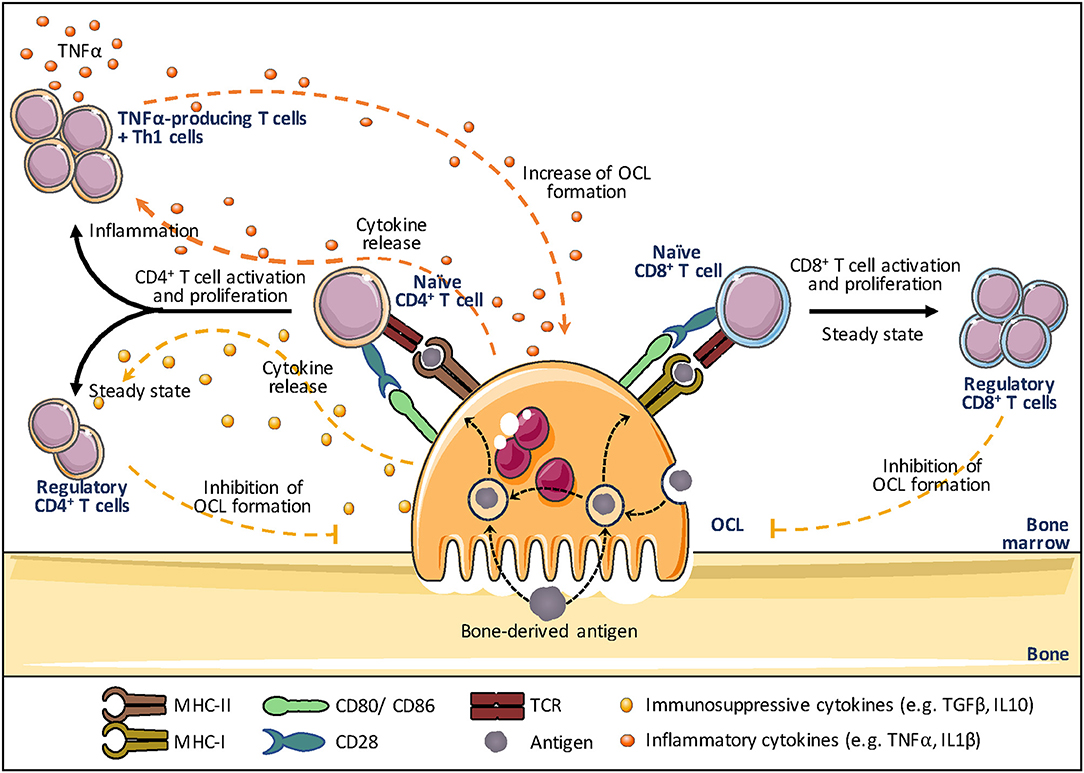
Figure 4. Osteoclasts and antigen presentation. Osteoclasts (OCLs) function as antigen presenting cells (APCs). OCLs process and present antigens (coming from the bone through bone resorption or the bone marrow) in the form of short peptides bound to major histocompatibility complex (MHC)-I or MHC-II molecules. The T cell receptor (TCR) on CD8+ and CD4+ T cells binds to the MHC-I and MHC-II complex, respectively, to initiate T cell activation and to trigger TCR signaling. A key step in T cell activation represents the T cell binding to co-stimulatory molecules (e.g. CD80 and CD86 on OCLs and CD28 on T cells), as they determine the functional outcome of the T cell receptor signaling. Subsequently, OCLs start secreting cytokines that control the differentiation of activated T cells. In steady state, OCLs produce mainly IL-10 and TGFβ and induce regulatory T cells (Treg) that are responsible for immunosuppressive responses and inhibit OCL differentiation, creating a negative feedback down regulating both inflammation and bone resorption. In contrast, in inflammation, OCLs induce effector TNFα-producing T cells that promote inflammation and may stimulate osteoclastogenesis thanks to their TNFα production. The effect of OCLs on CD8+ T cells in inflammatory conditions has not been explored yet.
Osteoclasts and T Cell Activation
While T cell activation is usually assumed to take place in secondary lymphoid organs such as the spleen and lymph nodes, naive T cell priming, activation and polarization into effector T cells as well as memory T cell reactivation can also occur in the BM, as described above (120–123). BM T cells represent ~3–8% of total BM cells and, compared with blood, the BM CD4/CD8 ratio is characteristically decreased (188, 189). Furthermore, in comparison to other lymphoid organs, memory CD4+ T cells specific for previously encountered antigens represent the vast majority of T cells observed in the BM, which includes central memory as well as effector memory T cells (190–192). Therefore, the BM microenvironment is often described as a major reservoir for memory lymphocytes (121, 193–195). Interestingly, T cells are often observed in the close vicinity of OCLs (34, 196–198) or adherent to OCLs (198). Furthermore, OCLs were shown to express chemokines involved in T cell chemotaxis and are able to attract CD4+ and CD8+ T cells in vitro as efficiently as DCs (18, 32, 34). Together with their APC function, this supports a contribution of OCLs to the activation of T cells in the bone marrow (Figure 4).
Based on transcriptomic analysis showing a high expression of genes related to antigen presentation in mature OCLs (147), Seifert et al. addressed the capacity of OCLs generated from murine BM cells to activate CD8+ T cells (32). Using an antigen-specific system, they reported that OCLs induce CD8+ T cell proliferation and activation by antigen cross-presentation (32), a function unique to DCs that participate in anti-infectious responses and self-tolerance (199). Interestingly, these OCL-primed CD8+ T cells expressed FoxP3, the master gene of regulatory T cells, and have a suppressive effect attested by their capacity to reduce the proliferation of other naive responder CD8+ T cells induced by DCs (32). This study clearly revealed for the first time that OCLs are not only regulated by T cells but can initiate T cell responses themselves, creating a feedback control loop. Indeed, Buchwald et al. (200) showed that the CD8+ Treg cells induced by OCLs are in turn able to suppress the resorptive function of OCLs as well as their differentiation.
Besides these findings, OCLs are also able to interact with naive CD4+ T cells. Using a reliable procedure to sort pure OCLs (18, 51), Ibanez et al. (18) demonstrated that OCLs from the BM of healthy mice induced FoxP3+ CD4+ T cells in an antigen-specific manner. These cells were shown to inhibit the activation of CD4+ T cells, confirming that they are immunosuppressive CD4+ Treg cells (18). This capacity was confirmed in human OCLs using T cells from tetanus toxoid (TT)-immunized healthy donors (33). OCLs pulsed with TT activate CD4+ T cells that produce high levels of the immunosuppressive cytokines IL-10 and TGF-β, evoking suppressive T cells (33). OCL-induced immunosuppression has also recently been reported in association with hematologic malignancies (35, 36, 201). In the context of multiple myeloma (MM), OCLs were shown to play a crucial role in the induction of an immunosuppressive microenvironment by upregulating inhibitory checkpoint molecules such as programmed death ligand 1 (PD-L1), CD200 and Galectin-9 as well as immunosuppressive cytokines (Figure 5). In addition, OCLs protect MM cells against T cell-mediated cytotoxicity through inhibition of CD4+ and CD8+ T cells and thereby support the development of the malignancy (35).
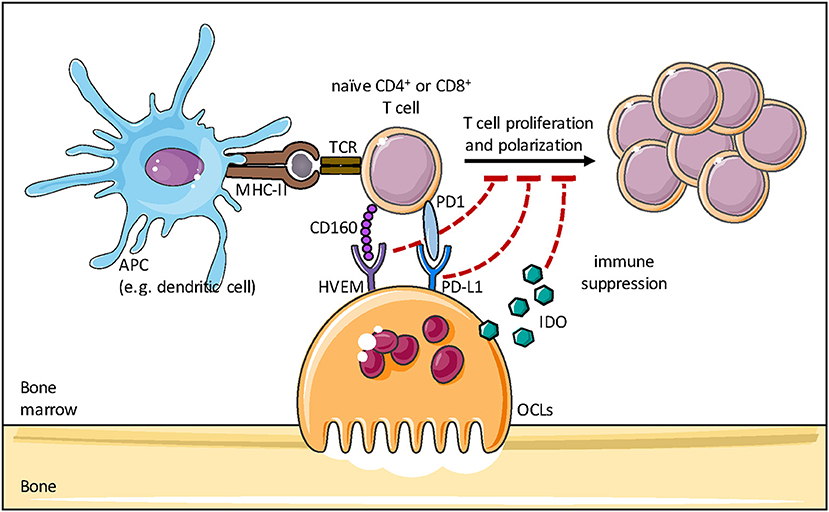
Figure 5. Osteoclasts and immune modulation. Osteoclasts are able to induce an immunosuppressive microenvironment by upregulating inhibitory checkpoint molecules such as programmed death ligand 1 (PD-L1), herpes virus entry mediator (HVEM) and Indoleamine 2,3-dioxygenase (IDO), thereby inhibiting the proliferation and polarization of naive T cells induced by antigen presenting cells (APCs).
However, OCLs are not restricted to immunosuppressive function. Indeed, while OCLs derived from healthy mice induce immunosuppressive CD4+ Treg cells, those derived from mice under inflammatory conditions induced TNFα-producing CD4+ T cells, as shown in the context of inflammatory bowel disease (18), a chronic inflammatory disease associated with increased OCL differentiation and severe bone destruction (60) (Figure 4). As OCLs from healthy mice, MN-derived OCLs induce CD4+ Treg cells and express higher levels of the immunosuppressive cytokine IL-10 than DC-derived OCLs (18). In contrast, as OCLs derived from mice affected by chronic inflammation, DC-derived OCLs very efficiently induce differentiation of TNFα+ CD4+ T cells and express higher levels of inflammatory cytokines (TNFα, IL-1β, IL-6) than MN-derived OCLs (18). These data unveiled for the first time the existence of different OCL subsets having opposite effects on the immune system depending on their context and cell origin. Searching for markers specific for murine OCL subsets, Ibanez et al. identified by flow cytometry the fractalkine receptor CX3CR1 as the first marker specifically expressed in inflammatory OCLs. Admittedly, only about 20% of inflammatory OCLs are positive for CX3CR1 (18) and the role of CX3CR1 in these OCLs as well as the function of CX3CR1+ and CX3CR1− inflammatory OCLs have not yet been addressed.
Physiological and Clinical Relevance of the Immune Function of Osteoclasts
In the end, the question remains what the biological relevance of antigen presentation by OCLs is. Comparable to APCs, OCLs mainly use clathrin-mediated endocytosis and micropinocytosis to internalize bone degradation products (179, 202). Thus, the physiological significance of the immune function of OCLs may be associated with the sustained release of self-peptides from bone resorption. These self-peptides can be presented by OCLs to inhibit self-responses by producing immunosuppressive cytokines and inducing suppressive Treg cells (18, 32, 200). In addition, OCLs have the ability to engulf and present antigens that are not coming from bone resorption (18, 34). This may also include antigens originating from the periphery such as blood-borne antigens (122, 123) or antigens carried by circulating DCs, neutrophils and B cells (120, 203) that can be presented directly on MHC-II molecules. Interestingly, the proportion of Treg cells among T cells in the BM is higher than in other tissues (204, 205) and they provide the BM with an immune privilege that is required for the maintenance of hematopoietic stem/progenitor cells (205). They also down regulate OCL differentiation participating thereby to the control of bone homeostasis (206–208). Thus, OCLs could therefore be regarded as BM resident APCs participating to maintain the BM immune tolerance under physiological conditions.
Contrary to this, OCL subsets generated under inflammatory conditions are devoid of the capacity to induce Treg cells but instead they induce effector CD4+ T cells that produce TNFα, an inflammatory cytokine that stimulates osteoclastogenesis and promotes inflammation (18). These T cells may also participate in auto-immune responses against bone antigens or in the activation of hematopoietic stem cells. Moreover, the cytokine production profile of OCLs is not only dependent on their physiological or inflammatory environment and cell origin (18) but also on their capacity to respond to bone and bone matrix proteins by secreting high levels of the pro-inflammatory cytokine IL-1β (83, 85, 86, 209). Overall, these data point inflammatory OCLs as major actors in a vicious circle linking bone destruction and inflammation.
This contribution of OCLs not only to bone resorption and homeostasis, but also to immune responses, encompasses the function of classical OCLs and sheds new light on the field of osteoimmunology. These insights make OCL an important target for anti-inflammatory therapies of chronic inflammatory diseases as well as for influencing the bone environment.
Of note, pathologies related to abnormal OCL activity/differentiation are frequently associated with immune dysfunctions not only in mouse but also in human. In osteopetrotic patients with defective OCL activity or differentiation, bone marrow failure is responsible for extramedullary hematopoiesis and contributes to immune deficiency and increases the risk of infections (210, 211). Besides, genes affected by osteopetrotic defects are not only essential for bone resorption but are also involved in immune responses. Among other examples, deficiency in Acp5 (encoding TRAcP) induces bone dysplasia but also autoimmunity (212, 213) and deletion or inhibition of Ctsk blocks both bone degradation and inflammation (214, 215). In osteoporosis, a number of immune-system related genes are differentially expressed in the BM of post-menopausal osteoporotic patients compared to non-osteoporotic individuals, including cytokines and factors involved in innate immunity (216–218). While such differences in gene expression and immune cell activation are clearly promoting bone destruction, the participation on OCLs in these immune modifications is still not known but cannot be excluded.
The immune function of OCLs emerged only recently, explaining why its contribution to OCL-associated diseases or its modulation by anti-resorption therapies has not yet been explored. A major issue for such investigation is the absence of markers specific for human OCL subsets that are required to identify inflammatory and anti-inflammatory OCLs. Thus, a deeper molecular profiling of human OCL sub-populations and characterization of their immune function remains essential to enable further studies in affected patients.
Conclusion
In the last decade, remarkable advances have been made in understanding the interactions between the skeletal and the immune system under both physiological and pathological conditions. In particular, the influence of T cells on OCL formation and activation through complex cytokine interactions including TNFα and RANKL were thoroughly investigated and immune cells were shown to regulate bone cell differentiation and activity. Today however, these interactions are known to be reciprocal, increasing further, the interest for OCLs as immune cells.
Depending on the context, different OCLs are described to derive from distinct progenitor cells. Based on the numerous OCL precursors described, and on the recent identification of an iterative fusion of mature OCLs with circulating monocytic cells2 (38), the possibility of OCL heterogeneity is huge. Additionally, some precursor cells seem to differentiate much more easily than others depending on their context. The existence of heterogeneous OCL populations appears unsurprising when considering that OCL precursors, including MNs and DCs, have been described as phenotypically and functionally heterogeneous for many years. Thus, bone destruction does not rely only on an increase in OCL differentiation and function, but also on the recruitment of OCL subsets that differ from steady state OCLs.
These novel insights in the field of osteoimmunology open new exciting perspectives and emphasize that OCL function is not restricted to bone resorption but expanded to immune cell differentiation and immunomodulation. Based on these observations and according to their immune function, OCLs could act as key players and regulators of the bone immune status in steady state as well as during inflammatory processes and they should not anymore be regarded only as bone-resorbing cells. Therefore, relying only on bone resorption may not be sufficient to block inflammatory bone destruction. New specific anti-resorptive agents targeting inflammatory OCLs and the associated T cell interaction could provide a very novel effective strategy to control inflammatory bone loss and the bone environment without compromising physiological bone remodeling by steady state OCLs.
Author Contributions
CB-W conceived the original idea and designed the project. M-BM, LI, TdV, AW, FA, and AT participated in writing the manuscript. M-BM and LI designed the figures. M-BM edited the figures. CB-W edited the manuscript.
Funding
This work was supported by the Fondation pour la Recherche Médicale (FRM, ECO20160736019), the Agence Nationale de la Recherche (ANR-16-CE14-0030), the University Côte d'Azur (ANR-15-IDEX-01), the Fondation Arthritis and the Société Française de Biologie des Tissus Minéralisés (SFBTM). Figures have been drawn from pictures from the “Servier Medical Art” gallery, http://smart.servier.com/.
Conflict of Interest Statement
The authors declare that the research was conducted in the absence of any commercial or financial relationships that could be construed as a potential conflict of interest.
Footnotes
1. ^https://www.armchairmedical.tv/anz-bone-mineral-society/videos/intravital-imaging-of-osteoclasts-in-vivo-reveals-a-novel-cell-fate-mechanism-dr-michelle-mcdonald
2. ^https://www.armchairmedical.tv/anz-bone-mineral-society/videos/intravital-imaging-of-osteoclasts-in-vivo-reveals-a-novel-cell-fate-mechanism-dr-michelle-mcdonald
References
1. Kölliker A. Die normale Resorption des Knochengewebes und Ihre Bedeutung für die Entstehung der Typischen Knochenformen. (1873). Available online at: https://archive.org/details/b22392610/page/n107
2. Walker DG. Control of bone resorption by hematopoietic tissue. The induction and reversal of congenital osteopetrosis in mice through use of bone marrow and splenic transplants. J Exp Med. (1975) 142:651–63. doi: 10.1084/jem.142.3.651
3. Udagawa N, Takahashi N, Akatsu T, Tanaka H, Sasaki T, Nishihara T, et al. Origin of osteoclasts: mature monocytes and macrophages are capable of differentiating into osteoclasts under a suitable microenvironment prepared by bone marrow-derived stromal cells. Proc Natl Acad Sci USA. (1990) 87:7260–4. doi: 10.1073/pnas.87.18.7260
4. Cappariello A, Maurizi A, Veeriah V, Teti A. The Great Beauty of the osteoclast. Arch Biochem Biophys. (2014) 558:70–8. doi: 10.1016/j.abb.2014.06.017
5. Gordon S, Taylor PR. Monocyte and macrophage heterogeneity. Nat Rev Immunol. (2005) 5:953. doi: 10.1038/nri1733
6. Lavin Y, Mortha A, Rahman A, Merad M. Regulation of macrophage development and function in peripheral tissues. Nat Rev Immunol. (2015) 15:731–44. doi: 10.1038/nri3920
7. Sprangers S, de Vries TJ, Everts V. Monocyte heterogeneity: consequences for monocyte-derived immune cells. J Immunol Res. (2016) 2016:1–10. doi: 10.1155/2016/1475435
8. Guilliams M, Mildner A, Yona S. Developmental and functional heterogeneity of monocytes. Immunity. (2018) 49:595–613. doi: 10.1016/j.immuni.2018.10.005
9. Mantovani A, Sica A, Locati M. New vistas on macrophage differentiation and activation. Eur J Immunol. (2007) 37:14–6. doi: 10.1002/eji.200636910
10. Mócsai A, Humphrey MB, Van Ziffle JAG, Hu Y, Burghardt A, Spusta SC, et al. The immunomodulatory adapter proteins DAP12 and Fc receptor gamma-chain (FcRgamma) regulate development of functional osteoclasts through the Syk tyrosine kinase. Proc Natl Acad Sci USA. (2004) 101:6158–63. doi: 10.1073/pnas.0401602101
11. Koga T, Inui M, Inoue K, Kim S, Suematsu A, Kobayashi E, et al. Costimulatory signals mediated by the ITAM motif cooperate with RANKL for bone homeostasis. Nature. (2004) 428:758–63. doi: 10.1038/nature02444
13. Rivollier A, Mazzorana M, Tebib J, Piperno M, Aitsiselmi T, Rabourdin-Combe C, et al. Immature dendritic cell transdifferentiation into osteoclasts: a novel pathway sustained by the rheumatoid arthritis microenvironment. Blood. (2004) 104:4029–37. doi: 10.1182/blood-2004-01-0041
14. Wakkach A, Mansour A, Dacquin R, Coste E, Jurdic P, Carle GF, et al. Bone marrow microenvironment controls the in vivo differentiation of murine dendritic cells into osteoclasts. Blood. (2008) 112:5074–83. doi: 10.1182/blood-2008-01-132787
15. Mansour A, Abou-Ezzi G, Sitnicka E, Jacobsen SEW, Wakkach A, Blin-Wakkach C. Osteoclasts promote the formation of hematopoietic stem cell niches in the bone marrow. J Exp Med. (2012) 209:537–49. doi: 10.1084/jem.20110994
16. Maitra R, Follenzi A, Yaghoobian A, Montagna C, Merlin S, Cannizzo ES, et al. Dendritic cell-mediated in vivo bone resorption. J Immunol. (2010) 185:1485–91. doi: 10.4049/jimmunol.0903560
17. Grosjean F, Nasi S, Schneider P, Chobaz V, Liu A, Mordasini V, et al. Dendritic cells cause bone lesions in a new mouse model of histiocytosis. PLoS ONE. (2015) 10:e0133917. doi: 10.1371/journal.pone.0133917
18. Ibáñez L, Abou-Ezzi G, Ciucci T, Amiot V, Belaïd N, Obino D, et al. Inflammatory osteoclasts prime TNFα-producing CD4(+) T cells and express CX3 CR1. J Bone Miner Res. (2016) 31:1899–908. doi: 10.1002/jbmr.2868
19. Speziani C, Rivollier A, Gallois A, Coury F, Mazzorana M, Azocar O, et al. Murine dendritic cell transdifferentiation into osteoclasts is differentially regulated by innate and adaptive cytokines. Eur J Immunol. (2007) 37:747–57. doi: 10.1002/eji.200636534
20. Alnaeeli M, Penninger JM, Teng Y-TA. Immune interactions with CD4+ T cells promote the development of functional osteoclasts from murine CD11c+ dendritic cells. J Immunol. (2006) 177:3314–26. doi: 10.4049/jimmunol.177.5.3314
21. Tucci M, Stucci S, Savonarola A, Ciavarella S, Cafforio P, Dammacco F, et al. Immature dendritic cells in multiple myeloma are prone to osteoclast-like differentiation through interleukin-17A stimulation. Br J Haematol. (2013) 161:821–31. doi: 10.1111/bjh.12333
22. Tucci M, Ciavarella S, Strippoli S, Brunetti O, Dammacco F, Silvestris F. Immature dendritic cells from patients with multiple myeloma are prone to osteoclast differentiation in vitro. Exp Hematol. (2011) 39:773–83.e1. doi: 10.1016/j.exphem.2011.04.006
23. da Costa CET, Annels NE, Faaij CMJM, Forsyth RG, Hogendoorn PCW, Egeler RM. Presence of osteoclast-like multinucleated giant cells in the bone and nonostotic lesions of Langerhans cell histiocytosis. J Exp Med. (2005) 201:687–93. doi: 10.1084/jem.20041785
24. Ginhoux F, Tacke F, Angeli V, Bogunovic M, Loubeau M, Dai X-M, et al. Langerhans cells arise from monocytes in vivo. Nat Immunol. (2006) 7:265–73. doi: 10.1038/ni1307
25. Hoeffel G, Wang Y, Greter M, See P, Teo P, Malleret B, et al. Adult Langerhans cells derive predominantly from embryonic fetal liver monocytes with a minor contribution of yolk sac-derived macrophages. J Exp Med. (2012) 209:1167–81. doi: 10.1084/jem.20120340
26. Kaplan DH. Ontogeny and function of murine epidermal Langerhans cells. Nat Immunol. (2017) 18:1068–75. doi: 10.1038/ni.3815
27. Wang W, Ferguson DJ, Quinn JM, Simpson AH, Athanasou NA. Biomaterial particle phagocytosis by bone-resorbing osteoclasts. J Bone Joint Surg Br. (1997) 79:849–56. doi: 10.1302/0301-620X.79B5.7780
28. Sakai E, Miyamoto H, Okamoto K, Kato Y, Yamamoto K, Sakai H. Characterization of phagosomal subpopulations along endocytic routes in osteoclasts and macrophages. J Biochem. (2001) 130:823–31. doi: 10.1093/oxfordjournals.jbchem.a003054
29. Heymann D, Guicheux J, Rousselle V. Ultrastructural evidence in vitro of osteoclast-induced degradation of calcium phosphate ceramic by simultaneous resorption and phagocytosis mechanisms. Histol Histopathol. (2001) 16:37–44. doi: 10.14670/HH-16.37
30. Harre U, Keppeler H, Ipseiz N, Derer A, Poller K, Aigner M, et al. Moonlighting osteoclasts as undertakers of apoptotic cells. Autoimmunity. (2012) 45:612–9. doi: 10.3109/08916934.2012.719950
31. Gordon S. Phagocytosis: an immunobiologic process. Immunity. (2016) 44:463–75. doi: 10.1016/j.immuni.2016.02.026
32. Kiesel JR, Buchwald ZS, Aurora R. Cross-presentation by osteoclasts induces FoxP3 in CD8+ T cells. J Immunol. (2009) 182:5477–87. doi: 10.4049/jimmunol.0803897
33. Li H, Hong S, Qian J, Zheng Y, Yang J, Yi Q. Cross talk between the bone and immune systems: osteoclasts function as antigen-presenting cells and activate CD4+ and CD8+ T cells. Blood. (2010) 116:210–7. doi: 10.1182/blood-2009-11-255026
34. Grassi F, Manferdini C, Cattini L, Piacentini A, Gabusi E, Facchini A, et al. T cell suppression by osteoclasts in vitro. J Cell Physiol. (2011) 226:982–90. doi: 10.1002/jcp.22411
35. An G, Acharya C, Feng X, Wen K, Zhong M, Zhang L, et al. Osteoclasts promote immune suppressive microenvironment in multiple myeloma: therapeutic implication. Blood. (2016) 128:1590–603. doi: 10.1182/blood-2016-03-707547
36. Mansour A, Wakkach A, Blin-Wakkach C. Emerging roles of osteoclasts in the modulation of bone microenvironment and immune suppression in multiple myeloma. Front Immunol. (2017) 8:954. doi: 10.3389/fimmu.2017.00954
37. Everts V, Korper W, Jansen DC, Steinfort J, Lammerse I, Heera S, et al. Functional heterogeneity of osteoclasts: matrix metalloproteinases participate in osteoclastic resorption of calvarial bone but not in resorption of long bone. FASEB J. (1999) 13:1219–30. doi: 10.1096/fasebj.13.10.1219
38. Jacome-Galarza CE, Percin GI, Muller JT, Mass E, Lazarov T, Eitler J, et al. Developmental origin, functional maintenance and genetic rescue of osteoclasts. Nature. (2019) 568:541–5. doi: 10.1038/s41586-019-1105-7
39. Xiao Y, Zijl S, Wang L, de Groot DC, van Tol MJ, Lankester AC, et al. Identification of the common origins of osteoclasts, macrophages, and dendritic cells in human hematopoiesis. Stem Cell Rep. (2015) 4:984–94. doi: 10.1016/j.stemcr.2015.04.012
40. Xiao Y, Palomero J, Grabowska J, Wang L, de Rink I, van Helvert L, et al. Macrophages and osteoclasts stem from a bipotent progenitor downstream of a macrophage/osteoclast/dendritic cell progenitor. Blood Adv. (2017) 1:1993–2006. doi: 10.1182/bloodadvances.2017008540
41. Arai F, Miyamoto T, Ohneda O, Inada T, Sudo T, Brasel K, et al. Commitment and differentiation of osteoclast precursor cells by the sequential expression of C-Fms and receptor activator of nuclear factor κb (Rank) receptors. J Exp Med. (1999) 190:1741–54. doi: 10.1084/jem.190.12.1741
42. Miyamoto T. Bifurcation of osteoclasts and dendritic cells from common progenitors. Blood. (2001) 98:2544–54. doi: 10.1182/blood.V98.8.2544
43. Jacome-Galarza CE, Lee S-K, Lorenzo JA, Aguila HL. Identification, characterization, and isolation of a common progenitor for osteoclasts, macrophages, and dendritic cells from murine bone marrow and periphery. J Bone Miner Res. (2013) 28:1203–13. doi: 10.1002/jbmr.1822
44. Jacquin C, Gran DE, Lee SK, Lorenzo JA, Aguila HL. Identification of multiple osteoclast precursor populations in murine bone marrow. J Bone Miner Res. (2005) 21:67–77. doi: 10.1359/JBMR.051007
45. Xiao Y, Song J-Y, de Vries TJ, Fatmawati C, Parreira DB, Langenbach GEJ, et al. Osteoclast precursors in murine bone marrow express CD27 and are impeded in osteoclast development by CD70 on activated immune cells. Proc Natl Acad Sci USA. (2013) 110:12385–90. doi: 10.1073/pnas.1216082110
46. de Vries TJ, Schoenmaker T, Hooibrink B, Leenen PJM, Everts V. Myeloid blasts are the mouse bone marrow cells prone to differentiate into osteoclasts. J Leukoc Biol. (2009) 85:919–27. doi: 10.1189/jlb.0708402
47. Mizoguchi T, Muto A, Udagawa N, Arai A, Yamashita T, Hosoya A, et al. Identification of cell cycle-arrested quiescent osteoclast precursors in vivo. J Cell Biol. (2009) 184:541–54. doi: 10.1083/jcb.200806139
48. Muto A, Mizoguchi T, Udagawa N, Ito S, Kawahara I, Abiko Y, et al. Lineage-committed osteoclast precursors circulate in blood and settle down into bone. J Bone Miner Res. (2011) 26:2978–90. doi: 10.1002/jbmr.490
49. Gomez Perdiguero E, Klapproth K, Schulz C, Busch K, Azzoni E, Crozet L, et al. Tissue-resident macrophages originate from yolk-sac-derived erythro-myeloid progenitors. Nature. (2015) 518:547–51. doi: 10.1038/nature13989
50. Blin-Wakkach C, Wakkach A, Sexton PM, Rochet N, Carle GF. Hematological defects in the oc/oc mouse, a model of infantile malignant osteopetrosis. Leukemia. (2004) 18:1505–11. doi: 10.1038/sj.leu.2403449
51. Madel M-B, Ibáñez L, Rouleau M, Wakkach A, Blin-Wakkach C. A novel reliable and efficient procedure for purification of mature osteoclasts allowing functional assays in mouse cells. Front Immunol. (2018) 9:2567. doi: 10.3389/fimmu.2018.02567
52. Boyle WJ, Simonet WS, Lacey DL. Osteoclast differentiation and activation. Nature. (2003) 423:337–42. doi: 10.1038/nature01658
53. Xing L. Osteoclast fusion and regulation by RANKL-dependent and independent factors. World J Orthop. (2012) 3:212. doi: 10.5312/wjo.v3.i12.212
54. Marino S, Logan JG, Mellis D, Capulli M. Generation and culture of osteoclasts. BoneKEy Rep. (2014) 3:570. doi: 10.1038/bonekey.2014.65
55. Seeling M, Hillenhoff U, David JP, Schett G, Tuckermann J, Lux A, et al. Inflammatory monocytes and Fcγ receptor IV on osteoclasts are critical for bone destruction during inflammatory arthritis in mice. Proc Natl Acad Sci USA. (2013) 110:10729–34. doi: 10.1073/pnas.1301001110
56. Ammari M, Presumey J, Ponsolles C, Roussignol G, Roubert C, Escriou V, et al. Delivery of miR-146a to Ly6C high monocytes inhibits pathogenic bone erosion in inflammatory arthritis. Theranostics. (2018) 8:5972–85. doi: 10.7150/thno.29313
57. Hoeffel G, Ginhoux F. Fetal monocytes and the origins of tissue-resident macrophages. Cell Immunol. (2018) 330:5–15. doi: 10.1016/j.cellimm.2018.01.001
58. Auffray C, Fogg D, Garfa M, Elain G, Join-Lambert O, Kayal S, et al. Monitoring of blood vessels and tissues by a population of monocytes with patrolling behavior. Science. (2007) 317:666–70. doi: 10.1126/science.1142883
59. Shi C, Pamer EG. Monocyte recruitment during infection and inflammation. Nat Rev Immunol. (2011) 11:762–74. doi: 10.1038/nri3070
60. Ciucci T, Ibáñez L, Boucoiran A, Birgy-Barelli E, Pène J, Abou-Ezzi G, et al. Bone marrow Th17 TNFα cells induce osteoclast differentiation, and link bone destruction to IBD. Gut. (2015) 64:1072–81. doi: 10.1136/gutjnl-2014-306947
61. Auffray C, Sieweke MH, Geissmann F. Blood monocytes: development, heterogeneity, and relationship with dendritic cells. Annu Rev Immunol. (2009) 27:669–92. doi: 10.1146/annurev.immunol.021908.132557
62. Geissmann F, Gordon S, Hume DA, Mowat AM, Randolph GJ. Unravelling mononuclear phagocyte heterogeneity. Nat Rev Immunol. (2010) 10:453–60. doi: 10.1038/nri2784
63. Varol C, Landsman L, Fogg DK, Greenshtein L, Gildor B, Margalit R, et al. Monocytes give rise to mucosal, but not splenic, conventional dendritic cells. J Exp Med. (2007) 204:171–80. doi: 10.1084/jem.20061011
64. Fogg DK, Sibon C, Miled C, Jung S, Aucouturier P, Littman DR, et al. A clonogenic bone marrow progenitor specific for macrophages and dendritic cells. Science. (2006) 311:83–7. doi: 10.1126/science.1117729
65. Cros J, Cagnard N, Woollard K, Patey N, Zhang S-Y, Senechal B, et al. Human CD14dim monocytes patrol and sense nucleic acids and viruses via TLR7 and TLR8 receptors. Immunity. (2010) 33:375–86. doi: 10.1016/j.immuni.2010.08.012
66. Villani A-C, Satija R, Reynolds G, Sarkizova S, Shekhar K, Fletcher J, et al. Single-cell RNA-seq reveals new types of human blood dendritic cells, monocytes, and progenitors. Science. (2017) 356. doi: 10.1126/science.aah4573
67. Chong SZ, Evrard M, Devi S, Chen J, Lim JY, See P, et al. CXCR4 identifies transitional bone marrow premonocytes that replenish the mature monocyte pool for peripheral responses. J Exp Med. (2016) 213:2293–314. doi: 10.1084/jem.20160800
68. Serbina NV, Pamer EG. Monocyte emigration from bone marrow during bacterial infection requires signals mediated by chemokine receptor CCR2. Nat Immunol. (2006) 7:311–7. doi: 10.1038/ni1309
69. Nguyen KD, Fentress SJ, Qiu Y, Yun K, Cox JS, Chawla A. Circadian gene Bmal1 regulates diurnal oscillations of Ly6C(hi) inflammatory monocytes. Science. (2013) 341:1483–8. doi: 10.1126/science.1240636
70. Patel AA, Zhang Y, Fullerton JN, Boelen L, Rongvaux A, Maini AA, et al. The fate and lifespan of human monocyte subsets in steady state and systemic inflammation. J Exp Med. (2017) 214:1913–23. doi: 10.1084/jem.20170355
71. Guilliams M, Scott CL. Does niche competition determine the origin of tissue-resident macrophages? Nat Rev Immunol. (2017) 17:451–60. doi: 10.1038/nri.2017.42
72. Yona S, Kim K-W, Wolf Y, Mildner A, Varol D, Breker M, et al. Fate mapping reveals origins and dynamics of monocytes and tissue macrophages under homeostasis. Immunity. (2013) 38:79–91. doi: 10.1016/j.immuni.2012.12.001
73. Swirski FK, Nahrendorf M, Etzrodt M, Wildgruber M, Cortez-Retamozo V, Panizzi P, et al. Identification of splenic reservoir monocytes and their deployment to inflammatory sites. Science. (2009) 325:612–6. doi: 10.1126/science.1175202
74. Sunderkötter C, Nikolic T, Dillon MJ, Van Rooijen N, Stehling M, Drevets DA, et al. Subpopulations of mouse blood monocytes differ in maturation stage and inflammatory response. J Immunol. (2004) 172:4410–7. doi: 10.4049/jimmunol.172.7.4410
75. Carlin LM, Stamatiades EG, Auffray C, Hanna RN, Glover L, Vizcay-Barrena G, et al. Nr4a1-dependent Ly6C(low) monocytes monitor endothelial cells and orchestrate their disposal. Cell. (2013) 153:362–75. doi: 10.1016/j.cell.2013.03.010
76. Peng Y, Latchman Y, Elkon KB. Ly6C(low) monocytes differentiate into dendritic cells and cross-tolerize T cells through PDL-1. J Immunol. (2009) 182:2777–85. doi: 10.4049/jimmunol.0803172
77. Park-Min K-H, Lee EY, Moskowitz NK, Lim E, Lee S-K, Lorenzo JA, et al. Negative regulation of osteoclast precursor differentiation by CD11b and β 2 integrin-B-cell lymphoma 6 signaling. J Bone Miner Res. (2013) 28:135–49. doi: 10.1002/jbmr.1739
78. Zhao Z, Hou X, Yin X, Li Y, Duan R, Boyce BF, et al. TNF induction of NF-κB RelB enhances RANKL-induced osteoclastogenesis by promoting inflammatory macrophage differentiation but also limits it through suppression of NFATc1 expression. PLoS ONE. (2015) 10:e0135728. doi: 10.1371/journal.pone.0135728
79. Charles JF, Hsu L-Y, Niemi EC, Weiss A, Aliprantis AO, Nakamura MC. Inflammatory arthritis increases mouse osteoclast precursors with myeloid suppressor function. J Clin Invest. (2012) 122:4592–605. doi: 10.1172/JCI60920
80. Komano Y, Nanki T, Hayashida K, Taniguchi K, Miyasaka N. Identification of a human peripheral blood monocyte subset that differentiates into osteoclasts. Arthritis Res Ther. (2006) 8:R152. doi: 10.1186/ar2046
81. Geissmann F, Jung S, Littman DR. Blood monocytes consist of two principal subsets with distinct migratory properties. Immunity. (2003) 19:71–82. doi: 10.1016/S1074-7613(03)00174-2
82. Sprangers S, Schoenmaker T, Cao Y, Everts V, de Vries TJ. Different blood-borne human osteoclast precursors respond in distinct ways to IL-17A. J Cell Physiol. (2016) 231:1249–60. doi: 10.1002/jcp.25220
83. De Vries TJ, el Bakkali I, Schett GA, Kamradt T, Jansen ID, D'Amelio P. What are the peripheral blood determinants for increased osteoclast formation in the various inflammatory diseases associated with bone loss? Front Immunol. (2019) 10:505. doi: 10.3389/fimmu.2019.00505
84. Chiu YG, Shao T, Feng C, Mensah KA, Thullen M, Schwarz EM, et al. CD16 (FcRγIII) as a potential marker of osteoclast precursors in psoriatic arthritis. Arthritis Res Ther. (2010) 12:R14. doi: 10.1186/ar2915
85. Cao Y, Jansen IDC, Sprangers S, Stap J, Leenen PJM, Everts V, et al. IL-1β differently stimulates proliferation and multinucleation of distinct mouse bone marrow osteoclast precursor subsets. J Leukoc Biol. (2016) 100:513–23. doi: 10.1189/jlb.1A1215-543R
86. de Vries TJ, Schoenmaker T, Aerts D, Grevers LC, Souza PPC, Nazmi K, et al. M-CSF priming of osteoclast precursors can cause osteoclastogenesis-insensitivity, which can be prevented and overcome on bone: bone activates osteoclast precursors. J Cell Physiol. (2015) 230:210–25. doi: 10.1002/jcp.24702
87. Cao Y, Jansen IDC, Sprangers S, de Vries TJ, Everts V. TNF-α has both stimulatory and inhibitory effects on mouse monocyte-derived osteoclastogenesis. J Cell Physiol. (2017) 232:3273–85. doi: 10.1002/jcp.26024
88. Barrow AD, Raynal N, Andersen TL, Slatter DA, Bihan D, Pugh N, et al. OSCAR is a collagen receptor that costimulates osteoclastogenesis in DAP12-deficient humans and mice. J Clin Invest. (2011) 121:3505–16. doi: 10.1172/JCI45913
89. Steinman RM, Cohn ZA. Identification of a novel cell type in peripheral lymphoid organs of mice. I. Morphology, quantitation, tissue distribution. J Exp Med. (1973) 137:1142–62. doi: 10.1084/jem.137.5.1142
90. Merad M, Sathe P, Helft J, Miller J, Mortha A. The dendritic cell lineage: ontogeny and function of dendritic cells and their subsets in the steady state and the inflamed setting. Annu Rev Immunol. (2013) 31:563–604. doi: 10.1146/annurev-immunol-020711-074950
91. Schlitzer A, McGovern N, Ginhoux F. Dendritic cells and monocyte-derived cells: two complementary and integrated functional systems. Semin Cell Dev Biol. (2015) 41:9–22. doi: 10.1016/j.semcdb.2015.03.011
92. Reizis B, Bunin A, Ghosh HS, Lewis KL, Sisirak V. Plasmacytoid dendritic cells: recent progress and open questions. Annu Rev Immunol. (2011) 29:163–83. doi: 10.1146/annurev-immunol-031210-101345
93. Ohl L, Mohaupt M, Czeloth N, Hintzen G, Kiafard Z, Zwirner J, et al. CCR7 governs skin dendritic cell migration under inflammatory and steady-state conditions. Immunity. (2004) 21:279–88. doi: 10.1016/j.immuni.2004.06.014
94. Walsh KP, Mills KHG. Dendritic cells and other innate determinants of T helper cell polarisation. Trends Immunol. (2013) 34:521–30. doi: 10.1016/j.it.2013.07.006
95. Wakkach A, Fournier N, Brun V, Breittmayer J-P, Cottrez F, Groux H. Characterization of dendritic cells that induce tolerance and T regulatory 1 cell differentiation in vivo. Immunity. (2003) 18:605–17. doi: 10.1016/S1074-7613(03)00113-4
96. Liu K, Waskow C, Liu X, Yao K, Hoh J, Nussenzweig M. Origin of dendritic cells in peripheral lymphoid organs of mice. Nat Immunol. (2007) 8:578–83. doi: 10.1038/ni1462
97. Liu K, Nussenzweig MC. Origin and development of dendritic cells. Immunol Rev. (2010) 234:45–54. doi: 10.1111/j.0105-2896.2009.00879.x
98. McKenna HJ, Stocking KL, Miller RE, Brasel K, De Smedt T, Maraskovsky E, et al. Mice lacking flt3 ligand have deficient hematopoiesis affecting hematopoietic progenitor cells, dendritic cells, and natural killer cells. Blood. (2000) 95:3489–97.
99. Shurin MR, Pandharipande PP, Zorina TD, Haluszczak C, Subbotin VM, Hunter O, et al. FLT3 ligand induces the generation of functionally active dendritic cells in mice. Cell Immunol. (1997) 179:174–84. doi: 10.1006/cimm.1997.1152
100. Lee J, Breton G, Oliveira TYK, Zhou YJ, Aljoufi A, Puhr S, et al. Restricted dendritic cell and monocyte progenitors in human cord blood and bone marrow. J Exp Med. (2015) 212:385–99. doi: 10.1084/jem.20141442
101. Hettinger J, Richards DM, Hansson J, Barra MM, Joschko A-C, Krijgsveld J, et al. Origin of monocytes and macrophages in a committed progenitor. Nat Immunol. (2013) 14:821–30. doi: 10.1038/ni.2638
102. Shortman K, Naik SH. Steady-state and inflammatory dendritic-cell development. Nat Rev Immunol. (2007) 7:19–30. doi: 10.1038/nri1996
103. Randolph GJ, Inaba K, Robbiani DF, Steinman RM, Muller WA. Differentiation of phagocytic monocytes into lymph node dendritic cells in vivo. Immunity. (1999) 11:753–61. doi: 10.1016/S1074-7613(00)80149-1
104. Domínguez PM, Ardavín C. Differentiation and function of mouse monocyte-derived dendritic cells in steady state and inflammation. Immunol Rev. (2010) 234:90–104. doi: 10.1111/j.0105-2896.2009.00876.x
105. Segura E, Amigorena S. Inflammatory dendritic cells in mice and humans. Trends Immunol. (2013) 34:440–5. doi: 10.1016/j.it.2013.06.001
106. Plantinga M, Guilliams M, Vanheerswynghels M, Deswarte K, Branco-Madeira F, Toussaint W, et al. Conventional and monocyte-derived CD11b+ dendritic cells initiate and maintain T helper 2 cell-mediated immunity to house dust mite allergen. Immunity. (2013) 38:322–35. doi: 10.1016/j.immuni.2012.10.016
107. Xu Y, Zhan Y, Lew AM, Naik SH, Kershaw MH. Differential development of murine dendritic cells by GM-CSF versus Flt3 ligand has implications for inflammation and trafficking. J Immunol. (2007) 179:7577–84. doi: 10.4049/jimmunol.179.11.7577
108. Greter M, Helft J, Chow A, Hashimoto D, Mortha A, Agudo-Cantero J, et al. GM-CSF controls nonlymphoid tissue dendritic cell homeostasis but is dispensable for the differentiation of inflammatory dendritic cells. Immunity. (2012) 36:1031–46. doi: 10.1016/j.immuni.2012.03.027
109. Wakim LM, Waithman J, van Rooijen N, Heath WR, Carbone FR. Dendritic cell-induced memory T cell activation in nonlymphoid tissues. Science. (2008) 319:198–202. doi: 10.1126/science.1151869
110. Kamath AT, Pooley J, O'Keeffe MA, Vremec D, Zhan Y, Lew AM, et al. The development, maturation, and turnover rate of mouse spleen dendritic cell populations. J Immunol. (2000) 165:6762–70. doi: 10.4049/jimmunol.165.12.6762
111. Kamath AT, Henri S, Battye F, Tough DF, Shortman K. Developmental kinetics and lifespan of dendritic cells in mouse lymphoid organs. Blood. (2002) 100:1734–41.
112. Kabashima K, Banks TA, Ansel KM, Lu TT, Ware CF, Cyster JG. Intrinsic lymphotoxin-beta receptor requirement for homeostasis of lymphoid tissue dendritic cells. Immunity. (2005) 22:439–50. doi: 10.1016/j.immuni.2005.02.007
113. Bogunovic M, Ginhoux F, Wagers A, Loubeau M, Isola LM, Lubrano L, et al. Identification of a radio-resistant and cycling dermal dendritic cell population in mice and men. J Exp Med. (2006) 203:2627–38. doi: 10.1084/jem.20060667
114. Merad M, Manz MG, Karsunky H, Wagers A, Peters W, Charo I, et al. Langerhans cells renew in the skin throughout life under steady-state conditions. Nat Immunol. (2002) 3:1135–41. doi: 10.1038/ni852
115. Josien R, Li HL, Ingulli E, Sarma S, Wong BR, Vologodskaia M, et al. TRANCE, a tumor necrosis factor family member, enhances the longevity and adjuvant properties of dendritic cells in vivo. J Exp Med. (2000) 191:495–502. doi: 10.1084/jem.191.3.495
116. Wong BR, Josien R, Lee SY, Sauter B, Li HL, Steinman RM, et al. TRANCE (tumor necrosis factor [TNF]-related activation-induced cytokine), a new TNF family member predominantly expressed in T cells, is a dendritic cell-specific survival factor. J Exp Med. (1997) 186:2075–80. doi: 10.1084/jem.186.12.2075
117. Zhang M, Tang H, Guo Z, An H, Zhu X, Song W, et al. Splenic stroma drives mature dendritic cells to differentiate into regulatory dendritic cells. Nat Immunol. (2004) 5:1124–33. doi: 10.1038/ni1130
118. Schirrmacher V. Cancer-reactive memory T cells from bone marrow: spontaneous induction and therapeutic potential (Review). Int J Oncol. (2015) 47:2005–16. doi: 10.3892/ijo.2015.3197
119. Sapoznikov A, Pewzner-Jung Y, Kalchenko V, Krauthgamer R, Shachar I, Jung S. Perivascular clusters of dendritic cells provide critical survival signals to B cells in bone marrow niches. Nat Immunol. (2008) 9:388–95. doi: 10.1038/ni1571
120. Cavanagh LL, Bonasio R, Mazo IB, Halin C, Cheng G, van der Velden AWM, et al. Activation of bone marrow-resident memory T cells by circulating, antigen-bearing dendritic cells. Nat Immunol. (2005) 6:1029–37. doi: 10.1038/ni1249
121. Mazo IB, Honczarenko M, Leung H, Cavanagh LL, Bonasio R, Weninger W, et al. Bone marrow is a major reservoir and site of recruitment for central memory CD8+ T cells. Immunity. (2005) 22:259–70. doi: 10.1016/j.immuni.2005.01.008
122. Feuerer M, Beckhove P, Garbi N, Mahnke Y, Limmer A, Hommel M, et al. Bone marrow as a priming site for T-cell responses to blood-borne antigen. Nat Med. (2003) 9:1151–7. doi: 10.1038/nm914
123. Milo I, Sapoznikov A, Kalchenko V, Tal O, Krauthgamer R, van Rooijen N, et al. Dynamic imaging reveals promiscuous crosspresentation of blood-borne antigens to naive CD8+ T cells in the bone marrow. Blood. (2013) 122:193–208. doi: 10.1182/blood-2012-01-401265
124. Gallois A, Lachuer J, Yvert G, Wierinckx A, Brunet F, Rabourdin-Combe C, et al. Genome-wide expression analyses establish dendritic cells as a new osteoclast precursor able to generate bone-resorbing cells more efficiently than monocytes. J Bone Miner Res. (2010) 25:661–72. doi: 10.1359/jbmr.090829
125. Laperine O, Blin-Wakkach C, Guicheux J, Beck-Cormier S, Lesclous P. Dendritic-cell-derived osteoclasts: a new game changer in bone-resorption-associated diseases. Drug Discov Today. (2016) 21:1345–54. doi: 10.1016/j.drudis.2016.04.022
126. Jansen IDC, Vermeer JAF, Bloemen V, Stap J, Everts V. Osteoclast fusion and fission. Calcif Tissue Int. (2012) 90:515–22. doi: 10.1007/s00223-012-9600-y
127. Gravallese EM, Manning C, Tsay A, Naito A, Pan C, Amento E, et al. Synovial tissue in rheumatoid arthritis is a source of osteoclast differentiation factor. Arthritis Rheum. (2000) 43:250. doi: 10.1002/1529-0131(200002)43:2<250::AID-ANR3>3.0.CO;2-P
128. Gravallese EM. Bone destruction in arthritis. Ann Rheum Dis. (2002) 61(Suppl 2):ii84–6. doi: 10.1136/ard.61.suppl_2.ii84
129. Jung SM, Kim KW, Yang C-W, Park S-H, Ju JH. Cytokine-mediated bone destruction in rheumatoid arthritis. J Immunol Res. (2014) 2014:1–15. doi: 10.1155/2014/263625
130. Baker PJ, Dixon M, Evans RT, Dufour L, Johnson E, Roopenian DC. CD4(+) T cells and the proinflammatory cytokines gamma interferon and interleukin-6 contribute to alveolar bone loss in mice. Infect Immun. (1999) 67:2804–9.
131. Taubman MA, Kawai T. Involvement of T-lymphocytes in periodontal disease and in direct and indirect induction of bone resorption. Crit Rev Oral Biol Med. (2001) 12:125–35. doi: 10.1177/10454411010120020301
132. Lerner UH. Inflammation-induced bone remodeling in periodontal disease and the influence of post-menopausal osteoporosis. J Dent Res. (2006) 85:596–607. doi: 10.1177/154405910608500704
133. de Vries TJ, Andreotta S, Loos BG, Nicu EA. Genes critical for developing periodontitis: lessons from mouse models. Front Immunol. (2017) 8:1395. doi: 10.3389/fimmu.2017.01395
134. Ali T, Lam D, Bronze MS, Humphrey MB. Osteoporosis in inflammatory bowel disease. Am J Med. (2009) 122:599–604. doi: 10.1016/j.amjmed.2009.01.022
135. Wakkach A, Rouleau M, Blin-Wakkach C. Osteoimmune interactions in inflammatory bowel disease: central role of bone marrow Th17 TNFα cells in osteoclastogenesis. Front Immunol. (2015) 6:640. doi: 10.3389/fimmu.2015.00640
136. Kong Y-Y, Feige U, Sarosi I, Bolon B, Tafuri A, Morony S, et al. Activated T cells regulate bone loss and joint destruction in adjuvant arthritis through osteoprotegerin ligand. Nature. (1999) 402:304–9. doi: 10.1038/46303
137. Sato K, Suematsu A, Okamoto K, Yamaguchi A, Morishita Y, Kadono Y, et al. Th17 functions as an osteoclastogenic helper T cell subset that links T cell activation and bone destruction. J Exp Med. (2006) 203:2673–82. doi: 10.1084/jem.20061775
138. Oostlander AE, Everts V, Schoenmaker T, Bravenboer N, van Vliet SJ, van Bodegraven AA, et al. T cell-mediated increased osteoclast formation from peripheral blood as a mechanism for Crohn's disease-associated bone loss. J Cell Biochem. (2012) 113:260–8. doi: 10.1002/jcb.23352
139. D'Amelio P, Grimaldi A, Di Bella S, Brianza SZM, Cristofaro MA, Tamone C, et al. Estrogen deficiency increases osteoclastogenesis up-regulating T cells activity: a key mechanism in osteoporosis. Bone. (2008) 43:92–100. doi: 10.1016/j.bone.2008.02.017
140. Adamopoulos IE, Chao C, Geissler R, Laface D, Blumenschein W, Iwakura Y, et al. Interleukin-17A upregulates receptor activator of NF-κB on osteoclast precursors. Arthritis Res Ther. (2010) 12:R29. doi: 10.1186/ar2936
141. Kotake S, Udagawa N, Takahashi N, Matsuzaki K, Itoh K, Ishiyama S, et al. IL-17 in synovial fluids from patients with rheumatoid arthritis is a potent stimulator of osteoclastogenesis. J Clin Invest. (1999) 103:1345–52. doi: 10.1172/JCI5703
142. Lubberts E, van den Bersselaar L, Oppers-Walgreen B, Schwarzenberger P, Coenen-de Roo CJJ, Kolls JK, et al. IL-17 promotes bone erosion in murine collagen-induced arthritis through loss of the receptor activator of NF-kappa B ligand/osteoprotegerin balance. J Immunol. (2003) 170:2655–62. doi: 10.4049/jimmunol.170.5.2655
143. Takahashi N, Mundy GR, Roodman GD. Recombinant human interferon-gamma inhibits formation of human osteoclast-like cells. J Immunol. (1986) 137:3544–49.
144. Moreno JL. IL-4 suppresses osteoclast development and mature osteoclast function by a STAT6-dependent mechanism: irreversible inhibition of the differentiation program activated by RANKL. Blood. (2003) 102:1078–86. doi: 10.1182/blood-2002-11-3437
145. Hong MH, Williams H, Jin CH, Pike JW. The inhibitory effect of interleukin-10 on mouse osteoclast formation involves novel tyrosine-phosphorylated proteins. J Bone Miner Res. (2000) 15:911–8. doi: 10.1359/jbmr.2000.15.5.911
146. Udagawa N, Horwood NJ, Elliott J, Mackay A, Owens J, Okamura H, et al. Interleukin-18 (interferon-gamma-inducing factor) is produced by osteoblasts and acts via granulocyte/macrophage colony-stimulating factor and not via interferon-gamma to inhibit osteoclast formation. J Exp Med. (1997) 185:1005–12. doi: 10.1084/jem.185.6.1005
147. Kiesel J, Miller C, Abu-Amer Y, Aurora R. Systems level analysis of osteoclastogenesis reveals intrinsic and extrinsic regulatory interactions. Dev Dyn. (2007) 236:2181–97. doi: 10.1002/dvdy.21206
148. Saltel F, Chabadel A, Bonnelye E, Jurdic P. Actin cytoskeletal organisation in osteoclasts: a model to decipher transmigration and matrix degradation. Eur J Cell Biol. (2008) 87:459–68. doi: 10.1016/j.ejcb.2008.01.001
149. Mulari MTK, Zhao H, Lakkakorpi PT, Väänänen HK. Osteoclast ruffled border has distinct subdomains for secretion and degraded matrix uptake: membrane turnover at osteoclast ruffled border. Traffic. (2003) 4:113–25. doi: 10.1034/j.1600-0854.2003.40206.x
150. Scott CC, Gruenberg J. Ion flux and the function of endosomes and lysosomes: pH is just the start: The flux of ions across endosomal membranes influences endosome function not only through regulation of the luminal pH. BioEssays. (2011) 33:103–10. doi: 10.1002/bies.201000108
151. Palokangas H, Mulari M, Väänänen HK. Endocytic pathway from the basal plasma membrane to the ruffled border membrane in bone-resorbing osteoclasts. J Cell Sci. (1997) 110:1767–80.
152. Baron R. Polarity and membrane transport in osteoclasts. Connect Tissue Res. (1989) 20:109–20. doi: 10.3109/03008208909023879
153. Mulari M, Vääräniemi J, Väänänen HK. Intracellular membrane trafficking in bone resorbing osteoclasts: membrane trafficking in osteoclasts. Microsc Res Tech. (2003) 61:496–503. doi: 10.1002/jemt.10371
154. Akisaka T, Yoshida H, Suzuki R. The ruffled border and attachment regions of the apposing membrane of resorbing osteoclasts as visualized from the cytoplasmic face of the membrane. J Electron Microsc. (2006) 55:53–61. doi: 10.1093/jmicro/dfl012
155. El-Sayed A, Harashima H. Endocytosis of gene delivery vectors: from clathrin-dependent to lipid raft-mediated endocytosis. Mol Ther. (2013) 21:1118–30. doi: 10.1038/mt.2013.54
156. Teti A, Marchisio PC, Zallone AZ. Clear zone in osteoclast function: role of podosomes in regulation of bone-resorbing activity. Am J Physiol-Cell Physiol. (1991) 261:C1–7. doi: 10.1152/ajpcell.1991.261.1.C1
157. Canton J, Neculai D, Grinstein S. Scavenger receptors in homeostasis and immunity. Nat Rev Immunol. (2013) 13:621–34. doi: 10.1038/nri3515
158. Dambuza IM, Brown GD. C-type lectins in immunity: recent developments. Curr Opin Immunol. (2015) 32:21–7. doi: 10.1016/j.coi.2014.12.002
159. Bakema JE, van Egmond M. The human immunoglobulin A Fc receptor FcαRI: a multifaceted regulator of mucosal immunity. Mucosal Immunol. (2011) 4:612–24. doi: 10.1038/mi.2011.36
160. van Lookeren Campagne M, Wiesmann C, Brown EJ. Macrophage complement receptors and pathogen clearance. Cell Microbiol. (2007) 9:2095–102. doi: 10.1111/j.1462-5822.2007.00981.x
161. Rosales C, Uribe-Querol E. Phagocytosis: a fundamental process in immunity. BioMed Res Int. (2017) 2017:1–18. doi: 10.1155/2017/9042851
162. Flannagan RS, Jaumouillé V, Grinstein S. The cell biology of phagocytosis. Annu Rev Pathol. (2012) 7:61–98. doi: 10.1146/annurev-pathol-011811-132445
163. Pierce AM. Attachment to and phagocytosis of mineral by alveolar bone osteoclasts. J Submicrosc Cytol Pathol. (1989) 21:63–71.
164. Wang W, Ferguson DJ, Quinn JM, Simpson AH, Athanasou NA. Osteoclasts are capable of particle phagocytosis and bone resorption. J Pathol. (1997) 182:92–8. doi: 10.1002/(SICI)1096-9896(199705)182:1<92::AID-PATH813>3.0.CO;2-E
165. Stenbeck G, Horton MA. Endocytic trafficking in actively resorbing osteoclasts. J Cell Sci. (2004) 117:827–36. doi: 10.1242/jcs.00935
166. Stenbeck G, Horton MA. Cell-matrix interaction in resorbing osteoclasts. J Cell Sci. (2000) 113:1577–87.
167. Boabaid F, Cerri PS, Katchburian E. Apoptotic bone cells may be engulfed by osteoclasts during alveolar bone resorption in young rats. Tissue Cell. (2001) 33:318–25. doi: 10.1054/tice.2001.0179
168. Soskolne WA. Phagocytosis of osteocytes by osteoclasts in femora of two week-old rabbits. Cell Tissue Res. (1978) 195:557–64. doi: 10.1007/BF00233897
169. Chambers TJ. Phagocytosis and trypsin-resistant glass adhesion by osteoclasts in culture. J Pathol. (1979) 127:55–60. doi: 10.1002/path.1711270202
170. Boyce BF, Yao Z, Xing L. Osteoclasts have multiple roles in bone in addition to bone resorption. Crit Rev Eukaryot Gene Expr. (2009) 19:171–80. doi: 10.1615/CritRevEukarGeneExpr.v19.i3.10
171. Lee S-H, Kim T-S, Choi Y, Lorenzo J. Osteoimmunology: cytokines and the skeletal system. BMB Rep. (2008) 41:495–510. doi: 10.5483/BMBRep.2008.41.7.495
172. Mansour A, Wakkach A, Blin-Wakkach C. Role of osteoclasts in the hematopoietic stem cell niche formation. Cell Cycle Georget Tex. (2012) 11:2045–6. doi: 10.4161/cc.20534
173. Steinman RM, Inaba K. The binding of antigen presenting cells to T lymphocytes. Adv Exp Med Biol. (1988) 237:31–41. doi: 10.1007/978-1-4684-5535-9_4
174. Jensen PE. Recent advances in antigen processing and presentation. Nat Immunol. (2007) 8:1041–8. doi: 10.1038/ni1516
175. Trombetta ES, Mellman I. Cell biology of antigen processing in vitro and in vivo. Annu Rev Immunol. (2005) 23:975–1028. doi: 10.1146/annurev.immunol.22.012703.104538
176. ten Broeke T, Wubbolts R, Stoorvogel W. MHC class II antigen presentation by dendritic cells regulated through endosomal sorting. Cold Spring Harb Perspect Biol. (2013) 5:a016873. doi: 10.1101/cshperspect.a016873
177. van den Elsen PJ. Expression regulation of major histocompatibility complex class I and class II encoding genes. Front Immunol. (2011) 2:48. doi: 10.3389/fimmu.2011.00048
178. Neefjes J, Jongsma MLM, Paul P, Bakke O. Towards a systems understanding of MHC class I and MHC class II antigen presentation. Nat Rev Immunol. (2011) 11:823–36. doi: 10.1038/nri3084
179. Blum JS, Wearsch PA, Cresswell P. Pathways of antigen processing. Annu Rev Immunol. (2013) 31:443–73. doi: 10.1146/annurev-immunol-032712-095910
180. Harding FA, McArthur JG, Gross JA, Raulet DH, Allison JP. CD28-mediated signalling co-stimulates murine T cells and prevents induction of anergy in T-cell clones. Nature. (1992) 356:607–9. doi: 10.1038/356607a0
181. Gutcher I, Becher B. APC-derived cytokines and T cell polarization in autoimmune inflammation. J Clin Invest. (2007) 117:1119–27. doi: 10.1172/JCI31720
182. Abbas AK, Murphy KM, Sher A. Functional diversity of helper T lymphocytes. Nature. (1996) 383:787–93. doi: 10.1038/383787a0
183. Sakaguchi S, Yamaguchi T, Nomura T, Ono M. Regulatory T cells and immune tolerance. Cell. (2008) 133:775–87. doi: 10.1016/j.cell.2008.05.009
184. Neurath MF, Finotto S, Glimcher LH. The role of Th1/Th2 polarization in mucosal immunity. Nat Med. (2002) 8:567–73. doi: 10.1038/nm0602-567
185. Banchereau J, Steinman RM. Dendritic cells and the control of immunity. Nature. (1998) 392:245–52. doi: 10.1038/32588
186. Unanue E, Allen P. The basis for the immunoregulatory role of macrophages and other accessory cells. Science. (1987) 236:551–7. doi: 10.1126/science.2437650
187. Kambayashi T, Laufer TM. Atypical MHC class II-expressing antigen-presenting cells: can anything replace a dendritic cell? Nat Rev Immunol. (2014) 14:719–30. doi: 10.1038/nri3754
188. Di Rosa F, Pabst R. The bone marrow: a nest for migratory memory T cells. Trends Immunol. (2005) 26:360–6. doi: 10.1016/j.it.2005.04.011
189. Westermann J, Pabst R. Distribution of lymphocyte subsets and natural killer cells in the human body. Clin Investig. (1992) 70:539–44. doi: 10.1007/BF00184787
190. Di Rosa F, Santoni A. Memory T-cell competition for bone marrow seeding. Immunology. (2003) 108:296–304. doi: 10.1046/j.1365-2567.2003.01593.x
191. Herndler-Brandstetter D, Landgraf K, Tzankov A, Jenewein B, Brunauer R, Laschober GT, et al. The impact of aging on memory T cell phenotype and function in the human bone marrow. J Leukoc Biol. (2012) 91:197–205. doi: 10.1189/jlb.0611299
192. Price PW, Cerny J. Characterization of CD4+ T cells in mouse bone marrow. I. Increased activated/memory phenotype and altered TCR Vbeta repertoire. Eur J Immunol. (1999) 29:1051–6. doi: 10.1002/(SICI)1521-4141(199903)29:03<1051::AID-IMMU1051>3.3.CO;2-P
193. Akatsuka Y, Torikai H, Inamoto Y, Tsujimura K, Morishima Y, Kodera Y, et al. Bone marrow may be a reservoir of long-lived memory T cells specific for minor histocompatibility antigen. Br J Haematol. (2006) 135:413–4. doi: 10.1111/j.1365-2141.2006.06313.x
194. Sabbagh L, Snell LM, Watts TH. TNF family ligands define niches for T cell memory. Trends Immunol. (2007) 28:333–9. doi: 10.1016/j.it.2007.06.001
195. Tokoyoda K, Zehentmeier S, Hegazy AN, Albrecht I, Grün JR, Löhning M, et al. Professional memory CD4+ T lymphocytes preferentially reside and rest in the bone marrow. Immunity. (2009) 30:721–30. doi: 10.1016/j.immuni.2009.03.015
196. Kikuta J, Wada Y, Kowada T, Wang Z, Sun-Wada G-H, Nishiyama I, et al. Dynamic visualization of RANKL and Th17-mediated osteoclast function. J Clin Invest. (2013) 123:866–73. doi: 10.1172/JCI65054
197. Pöllinger B, Junt T, Metzler B, Walker UA, Tyndall A, Allard C, et al. Th17 cells, not IL-17+ γδ T cells, drive arthritic bone destruction in mice and humans. J Immunol. (2011) 186:2602–12. doi: 10.4049/jimmunol.1003370
198. Moonen CGJ, Alders ST, Bontkes HJ, Schoenmaker T, Nicu EA, Loos BG, et al. Survival, retention, and selective proliferation of lymphocytes is mediated by gingival fibroblasts. Front Immunol. (2018) 9:1725. doi: 10.3389/fimmu.2018.01725
199. Heath WR, Carbone FR. Cross-presentation in viral immunity and self-tolerance. Nat Rev Immunol. (2001) 1:126–34. doi: 10.1038/35100512
200. Buchwald ZS, Kiesel JR, DiPaolo R, Pagadala MS, Aurora R. Osteoclast activated FoxP3+ CD8+ T-cells suppress bone resorption in vitro. PLoS ONE. (2012) 7:e38199. doi: 10.1371/journal.pone.0038199
201. Tai Y-T, Cho S-F, Anderson KC. Osteoclast immunosuppressive effects in multiple myeloma: role of programmed cell death ligand 1. Front Immunol. (2018) 9:1822. doi: 10.3389/fimmu.2018.01822
202. Zhao H. Membrane trafficking in osteoblasts and osteoclasts: new avenues for understanding and treating skeletal diseases. Traffic Cph Den. (2012) 13:1307–14. doi: 10.1111/j.1600-0854.2012.01395.x
203. Tacke F, Ginhoux F, Jakubzick C, van Rooijen N, Merad M, Randolph GJ. Immature monocytes acquire antigens from other cells in the bone marrow and present them to T cells after maturing in the periphery. J Exp Med. (2006) 203:583–97. doi: 10.1084/jem.20052119
204. Zou L, Barnett B, Safah H, Larussa VF, Evdemon-Hogan M, Mottram P, et al. Bone marrow is a reservoir for CD4+CD25+ regulatory T cells that traffic through CXCL12/CXCR4 signals. Cancer Res. (2004) 64:8451–5. doi: 10.1158/0008-5472.CAN-04-1987
205. Fujisaki J, Wu J, Carlson AL, Silberstein L, Putheti P, Larocca R, et al. in vivo imaging of Treg cells providing immune privilege to the haematopoietic stem-cell niche. Nature. (2011) 474:216–9. doi: 10.1038/nature10160
206. Buchwald ZS, Kiesel JR, Yang C, DiPaolo R, Novack DV, Aurora R. Osteoclast-induced Foxp3+ CD8 T-cells limit bone loss in mice. Bone. (2013) 56:163–73. doi: 10.1016/j.bone.2013.05.024
207. Zaiss MM, Frey B, Hess A, Zwerina J, Luther J, Nimmerjahn F, et al. Regulatory T cells protect from local and systemic bone destruction in arthritis. J Immunol. (2010) 184:7238–46. doi: 10.4049/jimmunol.0903841
208. Kelchtermans H, Geboes L, Mitera T, Huskens D, Leclercq G, Matthys P. Activated CD4+CD25+ regulatory T cells inhibit osteoclastogenesis and collagen-induced arthritis. Ann Rheum Dis. (2009) 68:744–50. doi: 10.1136/ard.2007.086066
209. Yao Z, Xing L, Qin C, Schwarz EM, Boyce BF. Osteoclast precursor interaction with bone matrix induces osteoclast formation directly by an interleukin-1-mediated autocrine mechanism. J Biol Chem. (2008) 283:9917–24. doi: 10.1074/jbc.M706415200
210. Villa A, Vezzoni P, Frattini A. Osteopetroses and immunodeficiencies in humans. Curr Opin Allergy Clin Immunol. (2006) 6:421–7. doi: 10.1097/01.all.0000246620.26623.5b
211. Stark Z, Savarirayan R. Osteopetrosis. Orphanet J Rare Dis. (2009) 4:5. doi: 10.1186/1750-1172-4-5
212. Briggs TA, Rice GI, Daly S, Urquhart J, Gornall H, Bader-Meunier B, et al. Tartrate-resistant acid phosphatase deficiency causes a bone dysplasia with autoimmunity and a type I interferon expression signature. Nat Genet. (2011) 43:127–31. doi: 10.1038/ng.748
213. Lausch E, Janecke A, Bros M, Trojandt S, Alanay Y, De Laet C, et al. Genetic deficiency of tartrate-resistant acid phosphatase associated with skeletal dysplasia, cerebral calcifications and autoimmunity. Nat Genet. (2011) 43:132–7. doi: 10.1038/ng.749
214. Hao L, Zhu G, Lu Y, Wang M, Jules J, Zhou X, et al. Deficiency of cathepsin K prevents inflammation and bone erosion in rheumatoid arthritis and periodontitis and reveals its shared osteoimmune role. FEBS Lett. (2015) 589:1331–9. doi: 10.1016/j.febslet.2015.04.008
215. Hao L, Chen J, Zhu Z, Reddy MS, Mountz JD, Chen W, et al. Odanacatib, a cathepsin K-specific inhibitor, inhibits inflammation and bone loss caused by periodontal diseases. J Periodontol. (2015) 86:972–83. doi: 10.1902/jop.2015.140643
216. Balla B, Kósa JP, Kiss J, Podani J, Takács I, Lazáry Á, et al. Transcriptional profiling of immune system-related genes in postmenopausal osteoporotic versus non-osteoporotic human bone tissue. Clin Immunol. (2009) 131:354–9. doi: 10.1016/j.clim.2009.01.004
217. Pineda B, Serna E, Laguna-Fernández A, Noguera I, Panach L, Hermenegildo C, et al. Gene expression profile induced by ovariectomy in bone marrow of mice: a functional approach to identify new candidate genes associated to osteoporosis risk in women. Bone. (2014) 65:33–41. doi: 10.1016/j.bone.2014.05.001
Keywords: osteoclast, osteoimmunology, monocyte heterogeneity, inflammation, immune modulation, dendritic cell
Citation: Madel M-B, Ibáñez L, Wakkach A, de Vries TJ, Teti A, Apparailly F and Blin-Wakkach C (2019) Immune Function and Diversity of Osteoclasts in Normal and Pathological Conditions. Front. Immunol. 10:1408. doi: 10.3389/fimmu.2019.01408
Received: 26 February 2019; Accepted: 04 June 2019;
Published: 19 June 2019.
Edited by:
Abbe N. de Vallejo, University of Pittsburgh, United StatesReviewed by:
Julia Charles, Brigham and Women's Hospital and Harvard Medical School, United StatesAlejandra Pera, Universidad de Córdoba, Spain
Copyright © 2019 Madel, Ibáñez, Wakkach, de Vries, Teti, Apparailly and Blin-Wakkach. This is an open-access article distributed under the terms of the Creative Commons Attribution License (CC BY). The use, distribution or reproduction in other forums is permitted, provided the original author(s) and the copyright owner(s) are credited and that the original publication in this journal is cited, in accordance with accepted academic practice. No use, distribution or reproduction is permitted which does not comply with these terms.
*Correspondence: Claudine Blin-Wakkach, YmxpbiYjeDAwMDQwO3VuaWNlLmZy