- Fundacion Infant, Buenos Aires, Argentina
The landscape of infant bronchiolitis and viral pneumonia may be altered by preventive interventions against respiratory syncytial virus under evaluation today. Pediatric wards in 2018 in developing countries may differ from those attended by future generation pediatricians who may not witness the packed emergency rooms, lack of available beds, or emergency situations that all physicians caring for children with RSV experience every year. In this review, we describe and discuss different prevention strategies under evaluation to protect pediatric patients. Then, we outline a number of potential challenges, benefits, and concerns that may result from successful interventions after licensure.
In the next decade, we may witness a change in the burden of office and emergency room visits, and hospitalizations in infant wards during the winter. Swamped emergency rooms and pediatricians and nurses overwhelmed with work, caring for an endless number of young infants in respiratory distress may start to become a scene of the past. Vaccines, monoclonal antibodies of extended half-life and antivirals against respiratory syncytial virus (RSV) are being evaluated in clinical trials (1). It is reasonable to expect that several of these candidates will be partly or completely successful in the next 10 years and become part of the preventive and therapeutic tools for pediatric public health.
RSV is the main cause of hospitalization in infants and young children worldwide. Millions of children are hospitalized every year and the vast majority of them live in the developing world. While infants born prematurely, those with congenital heart disease and other specific subgroups are at increased risk for hospitalization, the majority of severe cases affect previously healthy infants and children. The only available intervention licensed to prevent severe disease today is the administration of a neutralizing anti-RSV humanized monoclonal antibody, palivizumab®. Palivizumab® is recommended for high risk populations in high and middle-income countries (2). But its cost is prohibitive for low income nations, where most of the fatal cases of RSV disease occur.
Evidently, a safe and effective, affordable preventive strategy against RSV is necessary. One of these strategies under evaluation is to immunize pregnant women against the virus to transfer high titers of protective antibody to infants before birth (1). Alternatively, long-lived monoclonal antibodies against neutralizing epitopes in the RSV F protein could be administered to newborns and young infants to prevent disease in the first months of life (1). Other attractive approaches under study include immunization of infants with recombinant live attenuated RSV vaccines or using a variety of live vectors carrying genes encoding RSV proteins (1). Even though natural infection with RSV does not induce lifelong protective immunity, antibodies against the RSV F glycoprotein can prevent severe disease in humans. RSV F is a target for neutralizing antibodies, as evidenced by the effectiveness of palivizumab for nearly two decades. Hence, F alone or in combination with other viral proteins is the preferred antigen in RSV candidate vaccines (3).
Eliciting Protective Immunity Against RSV LRTI
Humans are the only natural host for RSV. The virus is spread from person to person via respiratory droplets, and spreads into the respiratory tract, where it preferentially targets apical ciliated epithelial cells (4). The incubation period for RSV from time of infection to onset of illness is between 3 and 5 days (5). Natural immunity includes innate responses by polymorphonuclear (PMN) and mononuclear cells, activation of numerous pattern recognition receptors (e.g., TLR3, TLR2/6, TLR7/8, NOD-like, and RIG-I-like receptors), and type I and III interferon responses (6–9). These responses are important, as PMNs and macrophages have been postulated to enhance and prevent severe disease, and PRRs have been reported to modulate numerous responses during RSV infection (5, 10). Moreover, although often underappreciated because their levels peak before RSV symptoms become evident to pediatricians, IFNs are increasingly recognized as relevant actors in RSV immunity (9, 11). In phase I trials with intranasal live attenuated RSV vaccines in seronegative infants, a small but significant number of subjects is not infected despite direct inoculation, suggesting that innate immune responses may be an important barrier against infection.
Adaptive immunity is critical for protection against RSV disease. The humanized monoclonal antibody palivizumab® and polyclonal sera enriched for antibodies against the RSV fusion (F) protein, Respigam®, demonstrate that antibodies against RSV F can prevent severe RSV LRTI. RSV infection elicits polyclonal, high avidity, neutralizing antibody responses against RSV F (12), that cross-react between RSV subgroups A and B. The F protein is highly conserved between RSV subgroups, with amino acid sequence identities of >90% (5, 13). This protein mediates entry into host cells by converting from a metastable trimeric pre-fusion conformation (pre-F) to a highly stable post-fusion conformation (post-F) (14). There are two pre-F-specific antigenic sites Ø and V, target of the most potent neutralizing antibodies, and two sites that are present on both conformations II and IV (12, 14). Although present on both pre-F and post-F, antibodies against site III bind tighter to pre-F (14). Instead, site I antibodies bind tighter to post-F (14). A newly recognized antigenic site in F, designated antigenic site VIII, occupies an intermediate position between the previously defined sites II and Ø (15).
The RSV attachment (G) protein also elicits polyclonal neutralizing responses. RSV G is more variable than RSV F, with ~50% sequence homology between subgroups A and B (5). Both RSV subgroups co-circulate during yearly epidemics, although one typically predominates every season (16–18). While IgA responses are probably important in protecting the respiratory tract, their exact role in RSV immunity is only now becoming clearer and requires further study (11).
T cell responses are also important for protection against RSV. CD4+ T lymphocytes contribute to T-cell dependent antibody responses and indirectly to viral clearance (as evidenced by persistent infections in HIV-infected individuals) and CD8+ T lymphocytes clear the virus from infected cells (5, 19).
The aforementioned innate and adaptive immune responses are mimicked to different degrees by live attenuated vaccines against RSV, discussed below. But in specific situations, immune responses against RSV -particularly those elicited by non-replicating vaccines in naive individuals- can also potentiate disease severity.
The first time an enhanced form of RSV disease (ERD) was observed in children during a vaccine trial, its manifestations were not recognized by investigators. Between 1962 and 1963, a formalin-inactivated vaccine against RSV (FIRSV) was evaluated in 54 children in the United States. Unfortunately, none of them received placebo. Twenty-one of fifty-four (39%) vaccine recipients were infected with RSV, and 10 (18%) experienced severe disease. But only 5 years had passed since the isolation and initial characterization of the chimpanzee coryza agent (now RSV), and its burden of illness was still unclear (20). As a consequence, researchers attributed the observed severity to an unusually bad season (20).
In 1966, a similar formalin-inactivated vaccine against RSV was administered to infants and toddlers in four trials in the United States. Immunized subjects had measurable non-neutralizing, low avidity anti-F IgG responses, no RSV-specific cytotoxic T lymphocytes and a CD4+T lymphocyte response primed to respond to wild type infection with an exuberant production of Th2 cytokines (10, 21–23). Indeed, upon infection with wild type RSV during the winter of 1966-1967, non-protective, low avidity IgG coupled with the virus to activate the complement cascade and in synergy with a strong Th2 polarization of the immune response led to increased hospitalization rates and two deaths in toddlers due to this enhanced form of RSV disease presenting with wheezing and bronchopneumonia (20, 24–26). Consequently, generation of an IgG response dominated by low avidity, non-neutralizing antibodies against RSV F (Figure 1), and priming for a Th2 bias upon RSV infection are considered undesirable features for RSV vaccine candidates.
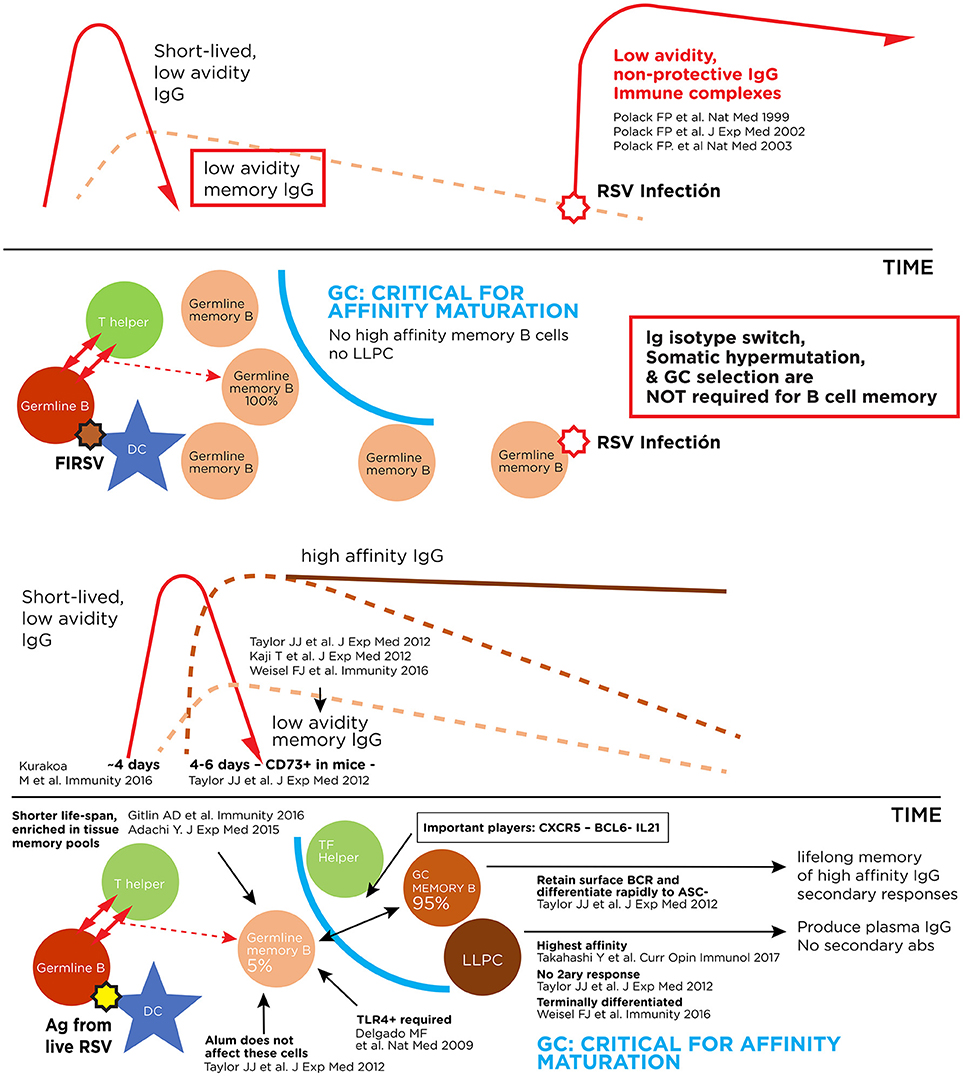
Figure 1. Pathogenic and protective antibody responses against RSV. Upper panel: Immune complex formation with antibodies of low affinity for the virus. Lower panel: Antibody response to live RSV infection with production of high affinity long-lived memory B cells and long-lived plasma cells (LLPC).
Immunizing Mothers to Protect Young Infants
Maternal immunization, a strategy well-accepted to prevent infant influenza and pertussis, aims to provide passive immunity to infants by transfer of maternal antibodies across the placenta. During the third trimester of pregnancy, IgG antibodies are actively transferred via the FcRn receptor in the placenta from the maternal to the fetal circulation. The first candidate in clinical trials to complete phase III evaluation in eleven countries enrolling approximately 4,600 women of childbearing age was the Novavax prefusogenic RSV F nanoparticle adjuvanted with alum (NCT02624947) (27). The Novavax vaccine elicited responses against epitopes displayed by both pre-fusion and post-fusion conformations of the F protein (28). The trial aimed to reduce the rate of medically significant RSV LRTI in infants through 90 days of life (27), but failed its primary endpoint despite an overall efficacy of 39.4% (95% CI, 5.3, 61.2) (29). Interestingly, the vaccine protected against RSV hospitalizations (40%) and severe disease (44%) worldwide (29), but its efficacy was radically different in the U.S. compared to developing countries: results in South Africa were considerably better than in the American population at 57% (95% CI, 32.7, 72.5) vs.−32.7% (95% CI,−238.9, 48.1). Unfortunately, the study lacked sufficient power to confidently ascertain whether these differences simply represented variations within the confidence interval.
Another vaccine designed for maternal immunization is a recombinant RSV protein F vaccine, engineered to preferentially maintain prefusion conformation by GSK. The vaccine progressed through early phase clinical trials in healthy adults and non-pregnant women (NCT02753413) (30–32). In addition, a subunit vaccine encoding a stabilized prefusion molecule developed by Pfizer also targets pregnant women (and the elderly) and is entering phase 2 trials (NCT03529773) (33–35). A fourth attractive investigational candidate developed by NIAID and entering clinical evaluation is RSV F DS-Cav1 (NCT03049488) (36), a formulation of engineered soluble site Ø–stabilized RSV F trimers (site Ø is only present on pre-fusion F) adjuvanted with alum (37, 38).
Long-Lived Monoclonal Antibodies
The concept of administering an optimized mAb with extended half-life and strong neutralizing activity to protect infants after birth is appealing. MEDI8897 from Medimmune is a highly potent, extended half-life antibody that completed phase 2 trials and is expected to enter phase 3 in 2019 (NCT02878330) (39). Recently, MEDI8897 was granted breakthrough therapy designation by the FDA. MEDI8897 is a recombinant human RSV monoclonal antibody with a modified Fc region. The antibody has been optimized from antibody D25, which targets site Ø in the pre-F conformation of the RSV F protein. MEDI8897, with a mean half-life 85 to 117 days, is intended to prevent RSV disease in all infants for the duration of the RSV season with a single dose (40). A triple-amino-acid (M252Y/S254T/T256E [YTE]) substitution within its Fc region extends its half-life by increasing binding to the major histocompatibility complex class I-related neonatal Fc receptor (FcRn) at acidic conditions and preventing degradation (40–42). A second long-lived monoclonal antibody candidate in clinical development is MK-1654 from Merck (NCT03524118) (43), undergoing early phase evaluations and targeting site IV in RSV F.
Despite the intuitive attraction of using a targeted mAb to neutralize RSV and prevent severe illness, this virus has proven once and again to be an annoying creature. A recent phase 3 study evaluating suptavumab (REGN2222), an antibody developed by Regeneron against site V in RSV F, did not meet its primary efficacy endpoint in healthy preterm infants (NCT02325791) (44). REGN2222 had a non-significant protective trend against medically attended infections in preterm infants up to day 150 of life caused by RSV subgroup A. But due to an unexpected mutation in site V of RSV F in circulating RSV B viruses, failed to prevent disease against this subgroup (44).
Live-Attenuated Vaccines Against RSV
Several live-attenuated RSV vaccines candidates to protect infants and young children are in clinical development (1). One of their strengths as infant vaccines is that decades of studies in infants have shown that these vaccines do not appear to prime for enhanced RSV disease in RSV-naïve infants (45). In addition, these vaccines are administrated intranasally, needle-free and, given that they replicate in the upper respiratory tract, can generate an immune response even in the presence of passively acquired maternal antibodies (46). Their greatest challenge is to attain the adequate balance between attenuation and immunogenicity. A clever deletion of the coding sequence for the RSV M2-2 protein in one of these candidates attenuates viral replication while upregulating gene transcription and antigen expression (NCT02237209, NCT02040831) (47, 48). The NS2 non-structural protein was deleted in a second candidate(NCT03422237, NCT03099291) (49, 50) reducing viral suppression of type interferon responses in the host (51). Both candidates were developed by the Laboratory of Infectious Diseases at NIAID and were safe in early studies in infants and young children and may soon progress to larger trials for evaluation. While no head-to-head comparison was described, the similarities in trial design suggest that the M2-2 vaccine has more restricted virus shedding but was more immunogenic than the live vaccine with the NS2 deletion (52).
Vectors Encoding RSV Genes
Vectored-based vaccines for children are now in clinical trials, using adenoviruses. Adenoviruses are highly immunogenic and induce both innate and adaptive immune responses (53). Moreover, adenovirus-based vaccines have been and are currently being investigated as vectors targeting viral, bacterial, and protozoan pathogens (53). The candidate Ad26.RSV.preF uses a human adenovirus 26 expressing pre-F RSV protein. Ad26.RSV.preF is now in phase 2 trials in adults and 12-24-month-old RSV seropositive toddlers (NCT03303625) (54). A second adenovirus-based candidate in phase 2 trials in seropositive children is ChAd155-RSV (NCT02927873) (55), using a replication-incompetent chimpanzee adenovirus 155. This vector encodes the F, N, and M2-1 RSV proteins (51, 55).
Finally, a chimeric candidate in clinical development is the rBCG-N-hRSV vaccine (NCT03213405) (56). A recombinant BCG expressing RSV N protein is targeted for use in newborns. BCG induces Th1 immunity, skewing responses away from undesirable Th2 priming (57). Vaccination with rBCG-N-hRSV is expected to elicit cellular immunity in addition to a humoral response against the virus (58).
The Future
Whether one or many of these vaccines and/or mAbs prove effective, several questions, concerns, and speculations remain to be answered through the trials and subsequent studies post-licensure. Some of these issues are discussed below.
Concerns of Enhanced RSV Disease
The mechanism of illness of ERD has not been completely elucidated, in part because every significant scientific advancement in structural virology and immunology continues to uncover new angles of a complex problem. For several years, two immune correlates described above have been accepted as indicators of candidate RSV vaccines that may prime for enhancement: the presence of low avidity, non-protective antibodies after immunization (Figure 1) (10, 21, 59), and a Th2 polarization of the immune response in the respiratory tract after RSV infection (23, 60). Now, novel observations in the field suggest a potential relationship between RSV F conformations in the vaccine and disease enhancement (14, 61); experience with other immunogens question whether route of vaccination may be an important determinant of RSV vaccine responses (62); improved understanding of B cell memory, class switching and affinity maturation may allow a clearer identification of primed B cell memory populations associated with undesirable outcomes (63–65); and detection of subpopulations with genetic mutations in molecules essential to B and T cell maturation interrogate whether enhancement could ever be possible in seropositive adults (66–68).
Importantly, given that memory B and T helper cells play a critical role in ERD pathogenesis, concerns for this problem are lower in several leading approaches to RSV prophylaxis that rely on passive acquisition of antibody: immunization of pregnant women to protect infants through transplacental transfer of antibody and administration of virus-specific monoclonal antibodies (mAb) of extended half-life (69, 70). Moreover, a series of maternal immunization studies and years of clinical experience with mAb in vulnerable infants never identified a case of ERD (2, 71, 72). Finally, infant intranasal immunization with live attenuated RSV vaccines (LAV) mimics natural infection, and after extensive testing in early phase trials were never found to associate with ERD in seronegative subjects (45).
Perhaps the greatest challenge will come from novel platforms that defy our traditional vaccine testing paradigms and may also alter our criteria for discriminating ERD in the future. For example, vaccine replication may not be necessary to prevent ERD priming in PAMP-adjuvanted vaccines (10, 73, 74), or a stabilized pre-fusion RSV F may elicit protective antibodies of high affinity.
Endotypes in RSV LRTI
The diversity in clinical presentations, combination of signs and symptoms, and variety in long-term consequences strongly suggest that RSV LRTI is not a single disease. In fact, it is probably a collective noun used to describe a set of clinical signs, which may obey different pathophysiological mechanisms. While subtypes of LRTI sharing similar observable characteristics are often designed as phenotypes, endotypes identify discrete subtypes based on specific mechanisms of illness. For example, middle-class urban and suburban infants with loss-of-function single nucleotide polymorphisms in Asp299Gly and/or Thr399Ile (TLR4+/−) are severely ill when infected with RSV due to an exaggerated Th2 responses in the respiratory tract. Moreover, these infants are not protected by the administration of RSV-specific mAb when premature (75). Children in Navajo and Apache reservations are also particularly susceptible to RSV (76). And a high-affinity mAb against RSV failed to prevent long-term recurrent wheezing in them, despite reducing the rate of severe acute RSV disease (77). Alaskan native children are equally susceptible to acute infection with the virus. Therefore, efficacy and subsequent effectiveness of preventive strategies against RSV may differ in certain RSV LRTI endotypes, and be affected by environmental exposures, population genotype, and/or circulating viruses.
Viral Replacement or Extended Protection
An interesting dilemma is whether a successful vaccine or mAb against RSV will lead to replacement of the virus by a different pathogen as a cause of pediatric illness or vaccination will impact other pulmonary ailments currently not known to be triggered by RSV.
In principle, vaccines and mAbs are not expected to prevent RSV infection in the upper respiratory tract, and therefore other agents would still compete for the niche with RSV in vaccinated subjects (78). However, recent data suggest that, upon prevention of RSV LRTI with palivizumab, other respiratory viruses may indeed replace a portion of the LRTI burden (78). A recent study in 429 premature infants, described a reduction in RSV LRTI, but reported no differences in the absolute number of respiratory episodes (78). These observations paralleled an increased rate of rhinovirus infections in recipients of palivizumab (79). A possible explanation may be that infants intermittently experience “windows of susceptibility” that allow pathogens to cause disease. RSV may outcompete other viruses for the nasopharyngeal niche in early life, but following immunization (or administration of mAb), infectivity of RSV may be “weakened”. Then, other viruses may outcompete this “weakened” version, becoming more frequent agents of LRTI. Therefore, it is conceivable that some of the burden caused today by RSV will be elicited in the future by other viral pathogens.
Interestingly, the maternal Novavax RSV prefusogenic vaccine evaluated worldwide conferred significant protection against all-cause LRTI (25.3%) and all-cause LRTI with significant hypoxemia (<92% O2 sat; 39.1%). Therefore, while it is conceivable that RSV may be replaced in certain situations, recent data suggest that significant, partly unexpected benefits may follow transplacental acquisition of maternal antibody in infants in developing nations.
Reducing Burden of Recurrent Wheezing and Asthma
RSV LRTI in infants and young children associates with 25–80% greater subsequent rates of recurrent wheezing and asthma when compared to children not experiencing severe RSV LRTI (80, 81). And while some of these RSV lung ailments affect infants genetically predisposed to develop asthma at an older age (82), severe RSV LRTI may also contribute to the inception of recurrent wheezing and asthma in children (80, 82). In fact, enough evidence exists today to prompt long term follow up of vaccinated subjects in clinical trials to ascertain a potential role for RSV vaccines in decreasing the burden of recurrent wheezing (77, 78, 83, 84).
A variety of studies in premature infants examined the efficacy or effectiveness of palivizumab® in preventing long term wheezing and asthma at ages 1 and 6 years (78, 83–86). Most of them described a protective role for RSV prevention against subsequent episodes of wheezing in infancy (78, 83, 84). The long-term effect elicited by RSV appears to be specific, and not triggered by infections with other viruses (78). However, a similar study with a virus-specific mAb in term, healthy Native Americans in Arizona prevented severe acute RSV LRTI but had no effect on rates of medically attended wheezing in children aged 1-3 years highlighting the potential importance of “endotypes” in future results (77).
At age 6 years in the randomized clinical trial in premature infants in The Netherlands, treatment significantly reduced parent-reported current asthma. The observation was based on different rates in infrequent wheezing (1-3 episodes/ year) (85), while physician diagnosed asthma and lung function were not different in drug and placebo recipients (85). In Japan, a second study revealed that palivizumab® prophylaxis administered to preterm infants did not suppress atopic asthma but lowered the incidence of recurrent wheezing (86).
Ongoing RCTs constitute a unique opportunity—perhaps the only ever- to settle these questions and determine the role of RSV in asthma inception. In recent years, a group of investigators reported guidelines for the evaluation of recurrent wheezing and asthma in upcoming studies (87). Yet, we must remain mindful of the fact that asthma is also a set of heterogeneous diseases sharing common symptoms. Therefore, it is highly likely that preventing severe RSV LRTI may affect one or few of these asthma endotypes but not others. And only a more sophisticated discrimination of the diseases under the “RSV LRTI and asthma umbrellas” will allow a definitive understanding of the mechanistic associations between both syndromes.
Decreasing Infant Mortality
In 2015, more than three million children were hospitalized with RSV LRTI and up to 118,000 died at hospitals and in the community. Ninety-nine percent of deaths occurred in developing countries (88). Deaths attributable to RSV in industrialized countries are not frequent, affecting children with congenital heart disease, chronic lung illness, neuromuscular disorders or genetic syndromes (89). In contrast, healthy term infants from socially vulnerable environments in the developing world die at the hospital with bacterial sepsis and/or affected by clinically significant pneumothoraxes (90). Importantly, approximately 50% of deaths due to RSV in the developing world are known to occur at home. These deaths affect infants and young children from families affected by serious socioeconomic challenges (91).
The overall impact of an effective RSV vaccine in the developing world remains to be determined. Shi et al. estimated that a vaccine against RSV with 80% efficacy would prevent 22,000 in-hospital deaths every year (88). But studies of RSV mortality show sequential seasonal peaks of RSV and pneumococcal disease suggesting a potential synergic association between both pathogens (92), and a third of community deaths during the winter associated with RSV in the only study with information about viral etiologies so far (91). In fact, the recently observed protection conferred by ResVax® against all-cause LRTI and against all-cause LRTI with significant hypoxemia suggest our estimations may require revision in the near future. In fact, detecting RSV using RT-PCR is challenging in underserved settings where deaths typically occur, and testing for it in critically ill patients is infrequent given the absence of specific therapies (93). Moreover, randomized clinical trials and formal studies enhance surveillance and standardize care, perhaps providing an overly optimistic perspective on this problem.
Breast Milk and RSV Vaccines
Breastfeeding is a critical asset to protect infants against respiratory viruses in developing countries (94). But the potential benefit conferred by antibodies in human milk elicited by maternal immunization is uncertain.
Numerous molecules in breast milk, passively transferred to the baby during lactation, have been postulated to confer protection. Among them, sIgA has been the most widely accepted example of this passive mechanism (95). However, this hypothesis has potential inconsistencies that require further consideration. First, protecting the lung with passively acquired defenses would require frequent “mini” gastric aspirations to coat the nasopharynx, as the predicted persistence of molecules in the upper respiratory tract is short lived (96). Such a mechanism would be highly inefficient, notwithstanding the repetitive risk for a more severe aspiration. Second, babies are often exposed to RSV simultaneously with their mothers (97). This timing is problematic, as Ig protection of the respiratory tract requires high levels of local antibody. Therefore, the situation would demand instantaneous boosting of maternal immunity to timely prevent infection in infants. Because it is not entirely logical to postulate that mothers carry protection in adequate concentrations against all pathogens at all times. Third, studies on RSV and nasal IgA neither exhibited a strong correlation between baseline IgA levels and ARI (98), nor were able to correlate neutralizing activity and anti-RSV IgA levels (99).
But there is another argument that supports the hypothesis that the main mechanism of protection against RSV in human milk is not through passive transfer of sIgA. At least five studies in different populations from different regions and risk condition, described sex differences in breast milk-mediated prevention of severe viral LRTI in children. In all these studies, milk protects female infants better than males (100–104). Therefore, human milk appears to activate a process in the baby. And this process, in line with evolution and preservation of the species, is better suited for females (100–104). It will be interesting to learn whether such benefits can be boosted through RSV immunization.
Benefits for Pregnant Women from Maternal Immunization
Influenza virus and B. pertussis maternal vaccines prevent severe disease in infants, but differ on their ability to affect maternal illness. Influenza virus is known to cause severe disease in pregnant women and its effects extend to influence both timing of delivery and fetal growth (105). Conversely, pertussis in adults is relatively mild (106). Post-vaccine licensure studies will define whether RSV vaccines belong to the first or the second group.
Defining RSV disease burden during pregnancy is challenging. Proper ascertainment of the problem would require very large prospective studies, intense outpatient follow-up, and consequently considerable funding. Therefore, most information today about RSV in pregnant women derives from post-hoc analyses of trials evaluating maternal immunogens against flu (107). This strategy is not perfect, particularly due to two important limitations: unlike in flu, fever is not a frequent clinical sign associated with RSV infection in pregnant women (108); and often RSV and influenza seasons do not overlap exactly (109). Then, studies using criteria tailored for influenza-like illness in flu studies to prompt sick visits or collect samples from mothers to study RSV probably underestimates the burden of illness (110). In absence of RSV-targeted studies, we cannot convincingly state today that RSV elicits milder disease than influenza during pregnancy. While RSV in pregnancy elicits mild illness, it can occasionally cause acute respiratory failure (111).
Strategy-Specific Challenges
Very specific logistical challenges may impact each immunization strategy. Maternal immunization may be most effective with different windows for immunization in pregnant women, should preparations differ. These decisions should be influenced by the levels of pre-existent antibodies in women and will also depend on the quality of prenatal care to maximize immunization rates. In the ResVax® RCT, immunization >30 days before delivery enhanced infant protection. In addition, acceptance of maternal immunization may differ in different populations due to cultural idiosyncrasies. Passive prophylaxis using mAb of extended half-life will require precise characterization of the local RSV season in different regions. This may prove challenging in tropical climates, where seasonality can oscillate by weeks in different years (112). And, importantly, cost of the drug in developing countries may become a critical factor for universal administration. These interventions are most needed in LMIC, where most morbidity and mortality occur. Finally, strategies like intranasal live attenuated RSV vaccine administration may demand a fine balance between optimal immunogenicity and no pathogenicity, require temperature stability for tropical climates, and inaugurate inoculation of RSV vaccines in older infants through a novel route of immunization, that for flu intranasal vaccines is not entirely comfortable.
In summary, the landscape of bronchiolitis and viral pneumonia may be altered directly and indirectly by the interventions under evaluation today. A long list of candidate vaccines (i.e., FIRSV, PFP-2) and mAb (i.e.: motavizumab®, suptavumab®) have failed over the years for different reason to control this feisty virus in young children. But both palivizumab® and the results from ResVax® in LMIC demonstrate that protection against RSV is possible. Just like many physicians in the industrialized world have rarely ever seen a case of measles, pediatricians in 2030 may be entirely unfamiliar with the packed emergency rooms, lack of available beds, or emergency situations that we experience every year due to RSV. Human metapneumovirus, rhinoviruses and human parainfluenza viruses may concentrate a lot more attention. A brighter future may be near.
Author Contributions
SSA and FPP reviewed the literature, conceived and wrote the manuscript.
Funding
This manuscript was funded under a grant from the Bill & Melinda Gates Foundation to FPP.
Conflict of Interest Statement
FPP received consulting funds from Novavax, GSK, Pfizer, Sanofi, MedImmune, Janssen, Merck, VirBio, Daiichi Sankyo, and Bavarian Nordic.
The remaining author declares that the research was conducted in the absence of any commercial or financial relationships that could be construed as a potential conflict of interest.
References
1. RSV Vaccine and mAb Snapshot-PATH Vaccine Resource Library. (2018). Available online at: http://vaccineresources.org/details.php?i=1562 (accessed December 12, 2018).
2. The IMpact-RSV Study Group. Palivizumab, a humanized respiratory syncytial virus monoclonal antibody, reduces hospitalization from respiratory syncytial virus infection in high-risk infants. The IMpact-RSV Study Group. Pediatrics. (1998) 102:531–7. doi: 10.1542/peds.102.3.531
3. Gilman MS, Castellanos CA, Chen M, Ngwuta JO, Goodwin E, Moin SM, et al. Rapid profiling of RSV antibody repertoires from the memory B cells of naturally infected adult donors. Sci Immunol. (2016) 1:eaaj1879. doi: 10.1126/sciimmunol.aaj1879
4. Schweitzer JW, Justice NA. Respiratory Syncytial Virus Infection (RSV). Treasure Island (FL): StatPearls Publishing StatPearls Publishing LLC (2019).
5. David M. Knipe PMH. Fields Virology, 6th ed. Respiratory Syncytial Virus. Philadelphia, PA: Lippincott Williams & Wilkins (2013). p.1086–123
6. Lambert L, Sagfors AM, Openshaw PJ, Culley FJ. Immunity to RSV in early-life. Front. Immunol. (2014) 5:466. doi: 10.3389/fimmu.2014.00466
7. Sun Y, Lopez CB. The innate immune response to RSV: Advances in our understanding of critical viral and host factors. Vaccine. (2017) 35:481–8. doi: 10.1016/j.vaccine.2016.09.030
8. Marr N, Turvey SE, Grandvaux N. Pathogen recognition receptor crosstalk in respiratory syncytial virus sensing: a host and cell type perspective. Trends Microbiol. (2013) 21:568–74. doi: 10.1016/j.tim.2013.08.006
9. Hillyer P, Shepard R, Uehling M, Krenz M, Sheikh F, Thayer KR, et al. Differential responses by human respiratory epithelial cell lines to respiratory syncytial virus reflect distinct patterns of infection control. J Virol. (2018) 92:e02202–17. doi: 10.1128/JVI.02202-17
10. Delgado MF, Coviello S, Monsalvo AC, Melendi GA, Hernandez JZ, Batalle JP, et al. Lack of antibody affinity maturation due to poor Toll-like receptor stimulation leads to enhanced respiratory syncytial virus disease. Nat Med. (2009) 15:34–41. doi: 10.1038/nm.1894
11. Hijano DR, Siefker DT, Shrestha B, Jaligama S, Vu LD, Tillman H, et al. Type I interferon potentiates IgA immunity to respiratory syncytial virus infection during infancy. Sci Rep. (2018) 8:11034. doi: 10.1038/s41598-018-29456-w
12. Graham BS. Vaccine development for respiratory syncytial virus. Curr Opin Virol. (2017) 23:107–12. doi: 10.1016/j.coviro.2017.03.012
13. McLellan JS, Ray WC, Peeples ME. Structure and function of respiratory syncytial virus surface glycoproteins. Curr Topics Microbiol Immunol. (2013) 372:83–104. doi: 10.1007/978-3-642-38919-1_4
14. Goodwin E, Gilman MSA, Wrapp D, Chen M, Ngwuta JO, Moin SM, et al. Infants infected with respiratory syncytial virus generate potent neutralizing antibodies that lack somatic hypermutation. Immunity. (2018) 48:339–49.e5. doi: 10.1016/j.immuni.2018.01.005
15. Mousa JJ, Kose N, Matta P, Gilchuk P, Crowe JE Jr. A novel pre-fusion conformation-specific neutralizing epitope on the respiratory syncytial virus fusion protein. Nat Microbiol. (2017) 2:16271. doi: 10.1038/nmicrobiol.2016.271
16. Liu W, Chen D, Tan W, Xu D, Qiu S, Zeng Z, et al. Epidemiology and clinical presentations of respiratory syncytial virus subgroups A and B detected with multiplex real-time PCR. PLoS ONE. (2016) 11:e0165108. doi: 10.1371/journal.pone.0165108
17. Hendry RM, Pierik LT, McIntosh K. Prevalence of respiratory syncytial virus subgroups over six consecutive outbreaks: 1981-1987. J Infect Dis. (1989) 160:185–90. doi: 10.1093/infdis/160.2.185
18. Dudas RA, Karron RA. Respiratory syncytial virus vaccines. Clin Microbiol Rev. (1998) 11:430–9. doi: 10.1128/CMR.11.3.430
19. Schmidt ME, Varga SM. Cytokines and CD8 T cell immunity during respiratory syncytial virus infection. Cytokine. (2018). doi: 10.1016/j.cyto.2018.07.012. [Epub ahead of print].
20. Kim HW, Canchola JG, Brandt CD, Pyles G, Chanock RM, Jensen K, et al. Respiratory syncytial virus disease in infants despite prior administration of antigenic inactivated vaccine. Am J Epidemiol. (1969) 89:422–34. doi: 10.1093/oxfordjournals.aje.a120955
21. Polack FP, Teng MN, Collins PL, Prince GA, Exner M, Regele H, et al. A role for immune complexes in enhanced respiratory syncytial virus disease. J Exp Med. (2002) 196:859–65. doi: 10.1084/jem.20020781
22. Acosta PL, Caballero MT, Polack FP. Brief history and characterization of enhanced respiratory syncytial virus disease. Clin Vac Immunol. (2015) 23:189–95. doi: 10.1128/CVI.00609-15
23. Knudson CJ, Hartwig SM, Meyerholz DK, Varga SM. RSV vaccine-enhanced disease is orchestrated by the combined actions of distinct CD4 T cell subsets. PLoS Pathog. (2015) 11:e1004757. doi: 10.1371/journal.ppat.1004757
24. Chin J, Magoffin RL, Shearer LA, Schieble JH, Lennette EH. Field evaluation of a respiratory syncytial virus vaccine and a trivalent parainfluenza virus vaccine in a pediatric population. Am J Epidemiol. (1969) 89:449–63. doi: 10.1093/oxfordjournals.aje.a120957
25. Kapikian AZ, Mitchell RH, Chanock RM, Shvedoff RA, Stewart CE. An epidemiologic study of altered clinical reactivity to respiratory syncytial (RS) virus infection in children previously vaccinated with an inactivated RS virus vaccine. Am J Epidemiol. (1969) 89:405–21. doi: 10.1093/oxfordjournals.aje.a120954
26. Fulginiti VA, Eller JJ, Sieber OF, Joyner JW, Minamitani M, Meiklejohn G. Respiratory virus immunization. I. A field trial of two inactivated respiratory virus vaccines; an aqueous trivalent parainfluenza virus vaccine and an alum-precipitated respiratory syncytial virus vaccine. Am J Epidemiol. (1969) 89:435–48. doi: 10.1093/oxfordjournals.aje.a120956
27. A Study to Determine the Safety and Efficacy of the RSV F Vaccine to Protect Infants via Maternal Immunization. (2018). Available online at https://clinicaltrials.gov/ct2/show/NCT02624947 (accessed December 08, 2018).
28. Positive Topline Data From Phase 2 Older Adult Trial and Path Forward for RSV F Vaccine Programs. (2018). Available online at: http://novavax.com/download/files/presentation/Novavax_RSV_Analyst_Day_7-24-17_PDF2.pdf (accessed December 08, 2018).
29. Novavax Announces Topline Results from Phase 3 PrepareTM Trial of ResVax™ for Prevention of RSV Disease in Infants via Maternal Immunization. (2019). Available online at: http://ir.novavax.com/news-releases/news-release-details/novavax-announces-topline-results-phase-3-preparetm-trial (accessed March 11, 2019).
30. Study Register. (2017). Avaialble online at: https://www.gsk-studyregister.com/study/5392 (accessed December 08, 2018).
31. Safety and Reactogenicity Study of GlaxoSmithKline (GSK) Biologicals' Investigational Respiratory Syncytial Virus (RSV) Vaccine (GSK3003891A) in Healthy Women. (2018). Available online at: https://clinicaltrials.gov/ct2/show/NCT02753413 (accessed Decemeber 08, 2018).
32. Beran J, Lickliter JD, Schwarz TF, Johnson C, Chu L, Domachowske JB, et al. Safety and immunogenicity of 3 formulations of an investigational respiratory syncytial virus vaccine in nonpregnant women: results from 2 phase 2 trials. J Infect Dis. (2018) 217:1616–25. doi: 10.1093/infdis/jiy065
33. Pfizer Begins a Phase 1/2 Study to Evaluate Respiratory Syncytial Virus (RSV) Vaccine. (2018). Available online at: https://www.pfizer.com/news/press-release/press-release-detail/pfizer__begins__a__phase__1__2__study__to__evaluate__respiratory__syncytial__virus__rsv__vaccine-0 (accessed December 10, 2018).
34. Describe the Safety and Immunogenicity of a RSV Vaccine in Healthy Adults. (2018). Available online at: https://clinicaltrials.gov/ct2/show/NCT03529773 (accessed December 10, 2018).
35. The Action-Packed Search for Stability: A Breakthrough in the Fight Against RSV. (2019). Avaialble online at: https://www.getscience.com/disruptive-science/action-packed-search-stability-breakthrough-fight-against-rsv (accessed March 10, 2019).
36. Respiratory Syncytial Virus Vaccine Enters Clinical Testing. (2018) at https://www.nih.gov/news-events/news-releases/respiratory-syncytial-virus-vaccine-enters-clinical-testing. (accessed December 11, 2018).
37. Sastry M, Zhang B, Chen M, Joyce MG, Kong WP, Chuang GY, et al. Adjuvants and the vaccine response to the DS-Cav1-stabilized fusion glycoprotein of respiratory syncytial virus. PLoS ONE. (2017) 12:e0186854. doi: 10.1371/journal.pone.0186854
38. McLellan JS, Chen M, Joyce MG, Sastry M, Stewart-Jones GB, Yang Y, et al. Structure-based design of a fusion glycoprotein vaccine for respiratory syncytial virus. Science (New York, NY). (2013) 342:592–8. doi: 10.1126/science.1243283
39. A Study to Evaluate the Safety and Efficacy of MEDI8897 for the Prevention of Medically Attended RSV LRTI in Healthy Preterm Infants. (2018). Available online at https://clinicaltrials.gov/ct2/show/NCT02878330 (accessed December 08, 2018).
40. Griffin MP, Khan AA, Esser MT, Jensen K, Takas T, Kankam MK, et al. Safety, tolerability, and pharmacokinetics of MEDI8897, the respiratory syncytial virus prefusion F-targeting monoclonal antibody with an extended half-life, in healthy adults. Antimicrob Agents Chemother. (2017) 61:e0171416. doi: 10.1128/AAC.01714-16
41. Robbie GJ, Criste R, Dall'acqua WF, Jensen K, Patel NK, Losonsky GA, et al. A novel investigational Fc-modified humanized monoclonal antibody, motavizumab-YTE, has an extended half-life in healthy adults. Antimicrob Agents Chemotherap. (2013) 57:6147–53. doi: 10.1128/AAC.01285-13
42. Dall'Acqua WF, Kiener PA, Wu H. Properties of human IgG1s engineered for enhanced binding to the neonatal Fc receptor (FcRn). J Biol Chem. (2006) 281:23514–24. doi: 10.1074/jbc.M604292200
43. Safety Tolerability and Pharmacokinetics of MK-1654 in Infants. (2018) Available online at: https://clinicaltrials.gov/ct2/show/NCT03524118 (accessed December 13, 2018).
44. Study to Evaluate the Efficacy and Safety of Suptavumab (REGN2222) for the Prevention of Medically Attended RSV (Respiratory Syncytial Virus) Infection in Preterm Infants. (2018). Available online at https://clinicaltrials.gov/ct2/show/NCT02325791 (accessed December 13, 2018).
45. Wright PF, Karron RA, Belshe RB, Shi JR, Randolph VB, Collins PL, et al. The absence of enhanced disease with wild type respiratory syncytial virus infection occurring after receipt of live, attenuated, respiratory syncytial virus vaccines. Vaccine. (2007) 25:7372–8. doi: 10.1016/j.vaccine.2007.08.014
46. Karron RA, Buchholz UJ, Collins PL. Live-attenuated respiratory syncytial virus vaccines. Curr Topics Microbiol Immunol. (2013) 372:259–84. doi: 10.1007/978-3-642-38919-1_13
47. Safety and Immune Response to a Live-Attenuated Respiratory Syncytial Virus (RSV) Vaccine in RSV-Seronegative Infants and Children. (2019). Available online at https://clinicaltrials.gov/ct2/show/NCT02040831 (accessed March 10, 2019).
48. Safety and Immune Response to a Live-Attenuated Respiratory Syncytial Virus (RSV) Vaccine in RSV-Seronegative Infants and Children. (2018). Available online at: https://clinicaltrials.gov/ct2/show/NCT02237209 (accessed December 14, 2018).
49. Evaluating the Infectivity Safety and Immunogenicity of the Recombinant Live-Attenuated Respiratory Syncytial Virus (RSV) Vaccines RSV ΔNS2/Δ1313/I1314L or RSV 276 in RSV-Seronegative Infants and Children 6 to 24 Months of Age. (2019). Available online at: https://clinicaltrials.gov/ct2/show/NCT03422237 (accessed March 11, 2019).
50. Infectivity Safety and Immunogenicity of a Recombinant Live-Attenuated Respiratory Syncytial Virus Vaccine (D46/NS2/N/ΔM2-2-HindIII) in RSV-Seronegative Infants and Children 6 to 24 Months of Age. (2019). Available online at: https://clinicaltrials.gov/ct2/show/NCT03099291 (accessed March 11, 2019).
51. Mazur NI, Higgins D, Nunes MC, Melero JA, Langedijk AC, Horsley N, et al. The respiratory syncytial virus vaccine landscape: lessons from the graveyard and promising candidates. Lancet Infect Dis. (2018) 18:e295–e311. doi: 10.1016/S1473-3099(18)30292-5
52. Karron RA, Luongo C, Thumar B, Loehr KM, Englund JA, Collins PL, et al. A gene deletion that up-regulates viral gene expression yields an attenuated RSV vaccine with improved antibody responses in children. Sci Transl Med. (2015) 7:312ra175. doi: 10.1126/scitranslmed.aac8463
53. Majhen D, Calderon H, Chandra N, Fajardo CA, Rajan A, Alemany R, et al. Adenovirus-based vaccines for fighting infectious diseases and cancer: progress in the field. Human Gene Therapy. (2014) 25:301–17. doi: 10.1089/hum.2013.235
54. A Study to Evaluate The Safety, Tolerability, Immunogenicity of an Investigational RSV Vaccine Candidate (Ad26,.RSV.preF) in Adults 18 to 50 Years of Age, RSV-Seropositive Toddlers 12 to 24 Months of Age. (2018). Avaialble online at: https://clinicaltrials.gov/ct2/show/NCT03303625 (accessed December 11, 2018).
55. RSV Investigational Vaccine in RSV-Seropositive Infants Aged 12 to 23 Months. (2018). Available Online at: https://clinicaltrials.gov/ct2/show/NCT02927873 (accessed December 12, 2018).
56. A Study to Assess Safety Tolerability and Immunogenicity of the Live Attenuated hRSV Vaccine rBCG-N-hRSV (EVA-VRS01). (2018). Available online at: https://clinicaltrials.gov/ct2/show/NCT03213405 (accessed December 08, 2018).
57. Rey-Jurado E, Soto J, Gálvez N, Kalergis AM. A safe and efficient BCG vectored vaccine to prevent the disease caused by the human respiratory syncytial virus. Hum Vaccines Immunotherapeut. (2017) 13:2092–7. doi: 10.1080/21645515.2017.1334026
58. Bueno SM, González PA, Cautivo KM, Mora JE, Leiva ED, Tobar HE, et al. Protective T cell immunity against respiratory syncytial virus is efficiently induced by recombinant BCG. Proc Nat Acad Sci. (2008) 105:20822. doi: 10.1073/pnas.0806244105
59. Connors M, Kulkarni AB, Firestone CY, Holmes KL, Morse HC, Sotnikov AV, et al. Pulmonary histopathology induced by respiratory syncytial virus (RSV) challenge of formalin-inactivated RSV-immunized BALB/c mice is abrogated by depletion of CD4+ T cells. J Virol. (1992) 66:7444–51.
60. Graham BS, Henderson GS, Tang YW, Lu X, Neuzil KM, Colley DG. Priming immunization determines T helper cytokine mRNA expression patterns in lungs of mice challenged with respiratory syncytial virus. J Immunol. (1993) 151:2032–40.
61. Killikelly AM, Kanekiyo M, Graham BS. Pre-fusion F is absent on the surface of formalin-inactivated respiratory syncytial virus. Sci Rep. (2016) 6:34108. doi: 10.1038/srep34108
62. Polack FP, Lee SH, Permar S, Manyara E, Nousari HG, Jeng Y, et al. Successful DNA immunization against measles: neutralizing antibody against either the hemagglutinin or fusion glycoprotein protects rhesus macaques without evidence of atypical measles. Nat Med. (2000) 6:776–81. doi: 10.1038/77506
63. De Silva NS, Klein U. Dynamics of B cells in germinal centres. Nat Rev Immunol. (2015) 15:137–48. doi: 10.1038/nri3804
64. Kim ST, Choi JY, Lainez B, Schulz VP, Karas DE, Baum ED, et al. Human Extrafollicular CD4(+) Th Cells Help Memory B Cells Produce Igs. J Immunol. (2018) 201:1359–72. doi: 10.4049/jimmunol.1701217
65. Weisel FJ, Zuccarino-Catania GV, Chikina M, Shlomchik MJ. A Temporal switch in the germinal center determines differential output of memory b and plasma cells. Immunity. (2016) 44:116–30. doi: 10.1016/j.immuni.2015.12.004
66. Duan Z, Chen X, Liang Z, Zeng Y, Zhu F, Long L, et al. Genetic polymorphisms of CXCR5 and CXCL13 are associated with non-responsiveness to the hepatitis B vaccine. Vaccine. (2014) 32:5316–22. doi: 10.1016/j.vaccine.2014.07.064
67. Choi YS, Yang JA, Crotty S. Dynamic regulation of Bcl6 in follicular helper CD4 T (Tfh) cells. Curr Opin Immunol. (2013) 25:366–72. doi: 10.1016/j.coi.2013.04.003
68. Morita R, Schmitt N, Bentebibel SE, Ranganathan R, Bourdery L, Zurawski G, et al. Human blood CXCR5(+)CD4(+) T cells are counterparts of T follicular cells and contain specific subsets that differentially support antibody secretion. Immunity. (2011) 34:108–21. doi: 10.1016/j.immuni.2010.12.012
69. American Academy of Pediatrics Committee on Infectious Diseases and Committee on Fetus and Newborn. Revised indications for the use of palivizumab and respiratory syncytial virus immune globulin intravenous for the prevention of respiratory syncytial virus infections. Pediatrics. (2003) 112:1442–6. doi: 10.1542/peds.112.6.1442
70. August A, Glenn GM, Kpamegan E, Hickman SP, Jani D, Lu H, et al. A Phase 2 randomized, observer-blind, placebo-controlled, dose-ranging trial of aluminum-adjuvanted respiratory syncytial virus F particle vaccine formulations in healthy women of childbearing age. Vaccine. (2017) 35:3749–59. doi: 10.1016/j.vaccine.2017.05.045
71. Groothuis JR, Simoes EA, Levin MJ, Hall CB, Long CE, Rodriguez WJ, et al. Prophylactic administration of respiratory syncytial virus immune globulin to high-risk infants and young children. The Respiratory Syncytial Virus Immune Globulin Study Group. N Engl J Med. (1993) 329:1524–30. doi: 10.1056/NEJM199311183292102
72. Malley R, DeVincenzo J, Ramilo O, Dennehy PH, Meissner HC, Gruber WC, et al. Reduction of respiratory syncytial virus (RSV) in tracheal aspirates in intubated infants by use of humanized monoclonal antibody to RSV F protein. J Infect Dis. (1998) 178:1555–61. doi: 10.1086/314523
73. Lambert SL, Aslam S, Stillman E, MacPhail M, Nelson C, Ro B, et al. A novel respiratory syncytial virus (RSV) F subunit vaccine adjuvanted with GLA-SE elicits robust protective TH1-type humoral and cellular immunity in rodent models. PLoS ONE. (2015) 10:e0119509. doi: 10.1371/journal.pone.0119509
74. Blanco JC, Boukhvalova MS, Pletneva LM, Shirey KA, Vogel SN. A recombinant anchorless respiratory syncytial virus (RSV) fusion (F) protein/monophosphoryl lipid A (MPL) vaccine protects against RSV-induced replication and lung pathology. Vaccine. (2014) 32:1495–500. doi: 10.1016/j.vaccine.2013.11.032
75. Caballero MT, Serra ME, Acosta PL, Marzec J, Gibbons L, Salim M, et al. TLR4 genotype and environmental LPS mediate RSV bronchiolitis through Th2 polarization. J Clin Invest. (2015) 125:571–82. doi: 10.1172/JCI75183
76. Bockova J, O'Brien KL, Oski J, Croll J, Reid R, Weatherholtz RC, et al. Respiratory syncytial virus infection in Navajo and White Mountain Apache children. Pediatrics. (2002) 110:e20. doi: 10.1542/peds.110.2.e20
77. O'Brien KL, Chandran A, Weatherholtz R, Jafri HS, Griffin MP, Bellamy T, et al. Efficacy of motavizumab for the prevention of respiratory syncytial virus disease in healthy Native American infants: a phase 3 randomised double-blind placebo-controlled trial. Lancet Infect Dis. (2015) 15:1398–408. doi: 10.1016/S1473-3099(15)00247-9
78. Blanken MO, Rovers MM, Molenaar JM, Winkler-Seinstra PL, Meijer A, Kimpen JL, et al. Respiratory syncytial virus and recurrent wheeze in healthy preterm infants. N Engl J Med. (2013) 368:1791–9. doi: 10.1056/NEJMoa1211917
79. Achten NB, Wu P, Bont L, Blanken MO, Gebretsadik T, Chappell JD, et al. Interference between respiratory syncytial virus and human rhinovirus infection in infancy. J Infect Dis. (2017) 215:1102–6. doi: 10.1093/infdis/jix031
80. Stein RT, Sherrill D, Morgan WJ, Holberg CJ, Halonen M, Taussig LM, et al. Respiratory syncytial virus in early life and risk of wheeze and allergy by age 13 years. Lancet. (1999) 354:541–5. doi: 10.1016/S0140-6736(98)10321-5
81. Wu P, Dupont WD, Griffin MR, Carroll KN, Mitchel EF, Gebretsadik T, et al. Evidence of a causal role of winter virus infection during infancy in early childhood asthma. Am J Respir Crit Care Med. (2008) 178:1123–9. doi: 10.1164/rccm.200804-579OC
82. Krishnamoorthy N, Khare A, Oriss TB, Raundhal M, Morse C, Yarlagadda M, et al. Early infection with respiratory syncytial virus impairs regulatory T cell function and increases susceptibility to allergic asthma. Nat Med. (2012) 18:1525–30. doi: 10.1038/nm.2896
83. Yoshihara S, Kusuda S, Mochizuki H, Okada K, Nishima S, Simões EA, et al. Effect of palivizumab prophylaxis on subsequent recurrent wheezing in preterm infants. Pediatrics. (2013) 132:811–8. doi: 10.1542/peds.2013-0982
84. Simões EA, Carbonell-Estrany X, Rieger CH, Mitchell I, Fredrick L, Groothuis JR, et al. The effect of respiratory syncytial virus on subsequent recurrent wheezing in atopic and nonatopic children. J Allergy Clin Immunol. (2010) 126:25662. doi: 10.1016/j.jaci.2010.05.026
85. Scheltema NM, Nibbelke EE, Pouw J, Blanken MO, Rovers MM, Naaktgeboren CA, et al. RSV prevention in infancy and asthma in later life - Authors' reply. Lancet Respir Med. (2018) 6:e33. doi: 10.1016/S2213-2600(18)30232-7
86. Mochizuki H, Kusuda S, Okada K, Yoshihara S, Furuya H, Simões EAF, et al. Palivizumab prophylaxis in preterm infants and subsequent recurrent wheezing. six-year follow-up study. Am J Respir Crit Care Med. (2017) 196:29–38. doi: 10.1164/rccm.201609-1812OC
87. Caballero MT, Jones MH, Karron RA, Hartert TV, Simões EA, Stein RT, et al. The impact of respiratory syncytial virus disease prevention on pediatric asthma. Pediatr Infect Dis J. (2016) 35:820–2. doi: 10.1097/INF.0000000000001167
88. Shi T, McAllister DA, O'Brien KL, Simoes EAF, Madhi SA, Gessner BD, et al. Global, regional, and national disease burden estimates of acute lower respiratory infections due to respiratory syncytial virus in young children in 2015: a systematic review and modelling study. Lancet. (2017) 390:946–58. doi: 10.1016/S0140-6736(17)30938-8
89. Byington CL, Wilkes J, Korgenski K, Sheng X. Respiratory syncytial virus-associated mortality in hospitalized infants and young children. Pediatrics. (2015) 135:e24–31. doi: 10.1542/peds.2014-2151
90. Geoghegan S, Erviti A, Caballero MT, Vallone F, Zanone SM, Losada JV, et al. Mortality due to respiratory syncytial virus. burden and risk factors. Am J Respir Crit Care Med. (2017) 195:96–103. doi: 10.1164/rccm.201603-0658OC
91. Caballero MT, Bianchi AM, Nuno A, Ferretti AJP, Polack LM, Remondino I, et al. Mortality in children in the community associated with acute respiratory infections. J Infect Dis. (2018) 219:358–364. doi: 10.1093/infdis/jiy517
92. Lozano R, Naghavi M, Foreman K, Lim S, Shibuya K, Aboyans V, et al. Global and regional mortality from 235 causes of death for 20 age groups in 1990 and 2010: a systematic analysis for the Global Burden of Disease Study 2010. Lancet. (2012) 380:2095–128. doi: 10.1016/S0140-6736(12)61728-0
93. Djelantik IG, Gessner BD, Sutanto A, Steinhoff M, Linehan M, Moulton LH, et al. Case fatality proportions and predictive factors for mortality among children hospitalized with severe pneumonia in a rural developing country setting. J Trop Pediatr. (2003) 49:327–32. doi: 10.1093/tropej/49.6.327
94. Effect of breastfeeding on infant and child mortality due to infectious diseases in less developed countries: a pooled analysis. WHO Collaborative Study team on the role of breastfeeding on the prevention of infant mortality. Lancet. (2000) 355:451–5. doi: 10.1016/S0140-6736(00)82011-5
95. Goldman AS, Smith CW. Host resistance factors in human milk. J Pediatr. (1973) 82:1082–90. doi: 10.1016/S0022-3476(73)80453-6
96. Schipper NG, Verhoef JC, Merkus FW. The nasal mucociliary clearance: relevance to nasal drug delivery. Pharm Res. (1991) 8:807–14.
97. Munywoki PK, Koech DC, Agoti CN, Lewa C, Cane PA, Medley GF, et al. The source of respiratory syncytial virus infection in infants: a household cohort study in rural Kenya. J Infect Dis. (2014) 209:1685–92. doi: 10.1093/infdis/jit828
98. Hall CB, Walsh EE, Long CE, Schnabel KC. Immunity to and frequency of reinfection with respiratory syncytial virus. J Infect Dis. (1991) 163:693–8.
99. McIntosh K, Masters HB, Orr I, Chao RK, Barkin RM. The immunologic response to infection with respiratory syncytial virus in infants. J Infect Dis. (1978) 138:24–32.
100. Klein MI, Bergel E, Gibbons L, Coviello S, Bauer G, Benitez A, et al. Differential gender response to respiratory infectionsand to the protective effect of breast milk in preterm infants. Pediatrics. (2008) 121:e1510–6. doi: 10.1542/peds.2007-1757
101. Libster R, Bugna Hortoneda J, Laham FR, Casellas JM, Israele V, Polack NR, et al. Breastfeeding prevents severe disease in full term female infants with acute respiratory infection. Pediatr Infect Dis J. (2009) 28:131–4. doi: 10.1097/INF.0b013e31818a8a82
102. Sinha A, Madden J, Ross-Degnan D, Soumerai S, Platt R. Reduced risk of neonatal respiratory infections among breastfed girls but not boys. Pediatrics. (2003) 112:e303. doi: 10.1542/peds.112.4.e303
103. Wright AL, Holberg CJ, Martinez FD, Morgan WJ, Taussig LM. Breast feeding and lower respiratory tract illness in the first year of life. Group Health Medical Associates. BMJ. (1989) 299:946–9.
104. Acuña-Cordero R, Sossa-Briceño MP, Rodríguez-Martínez CE. Predictors of hospitalization for acute lower respiratory infections during the first two years of life in a population of preterm infants with bronchopulmonary dysplasia. Early Hum Dev. (2018) 127:53–7. doi: 10.1016/j.earlhumdev.2018.10.003
105. Steinhoff MC, Omer SB, Roy E, El Arifeen S, Raqib R, Dodd C, et al. Neonatal outcomes after influenza immunization during pregnancy: a randomized controlled trial. CMAJ. (2012) 184:645–53. doi: 10.1503/cmaj.110754
106. Domínguez A, Soldevila N, Caylà JA, García-Cenoz M, Ferrús G, Sala-Farré MR, et al. Assessment of clinical symptoms in household contacts of confirmed pertussis cases. J Infect. (2017) 75:426–32. doi: 10.1016/j.jinf.2017.08.008
107. Nunes MC, Cutland CL, Jones S, Downs S, Weinberg A, Ortiz JR, et al. Efficacy of maternal influenza vaccination against all-cause lower respiratory tract infection hospitalizations in young infants: results from a randomized controlled trial. Clin Infect Dis. (2017) 65:1066–71. doi: 10.1093/cid/cix497
108. Chu HY, Katz J, Tielsch J, Khatry SK, Shrestha L, LeClerq SC, et al. Clinical presentation and birth outcomes associated with respiratory syncytial virus infection in pregnancy. PLoS ONE. (2016) 11:e0152015. doi: 10.1371/journal.pone.0152015
109. Chaw L, Kamigaki T, Burmaa A, Urtnasan C, Od I, Nyamaa G, et al. Burden of influenza and respiratory syncytial virus infection in pregnant women and infants under 6 months in mongolia: a prospective cohort study. PLoS ONE. (2016) 11:e0148421. doi: 10.1371/journal.pone.0148421
110. Polack FP. Respiratory syncytial virus during pregnancy. Clin Infect Dis. (2018) 66:1666–7. doi: 10.1093/cid/cix1091
111. Wheeler SM, Dotters-Katz S, Heine RP, Grotegut CA, Swamy GK. Maternal effects of respiratory syncytial virus infection during pregnancy. Emerg Infect Dis. (2015) 21:1951–5. doi: 10.3201/eid2111.150497
Keywords: RSV, RSV vaccines, monoclonal antibodies, maternal immunization, live attenuated vaccines
Citation: Aranda SS and Polack FP (2019) Prevention of Pediatric Respiratory Syncytial Virus Lower Respiratory Tract Illness: Perspectives for the Next Decade. Front. Immunol. 10:1006. doi: 10.3389/fimmu.2019.01006
Received: 17 December 2018; Accepted: 18 April 2019;
Published: 07 May 2019.
Edited by:
Alexis M. Kalergis, Pontificia Universidad Católica de Chile, ChileReviewed by:
Juan Pablo Jaworski, National Council for Scientific and Technical Research (CONICET), ArgentinaBernhard Resch, Medical University of Graz, Austria
Copyright © 2019 Aranda and Polack. This is an open-access article distributed under the terms of the Creative Commons Attribution License (CC BY). The use, distribution or reproduction in other forums is permitted, provided the original author(s) and the copyright owner(s) are credited and that the original publication in this journal is cited, in accordance with accepted academic practice. No use, distribution or reproduction is permitted which does not comply with these terms.
*Correspondence: Fernando P. Polack, ZnBvbGFja0BpbmZhbnQub3JnLmFy