- 1Department of Biochemistry, Western University, London, ON, Canada
- 2London Regional Cancer Program, Lawson Health Research Institute, London, ON, Canada
- 3Department of Oncology, Biochemistry and Surgery, Schulich School of Medicine and Dentistry, Western University, London, ON, Canada
Hyaluronan (HA) is a glycosaminoglycan with a simple structure but diverse and often opposing functions. The biological activities of this polysaccharide depend on its molecular weight and the identity of interacting receptors. HA is initially synthesized as high molecular-weight (HMW) polymers, which maintain homeostasis and restrain cell proliferation and migration in normal tissues. These HMW-HA functions are mediated by constitutively expressed receptors including CD44, LYVE-1, and STABILIN2. During normal processes such as tissue remodeling and wound healing, HMW-HA is fragmented into low molecular weight polymers (LMW-HA) by hyaluronidases and free radicals, which promote inflammation, immune cell recruitment and the epithelial cell migration. These functions are mediated by RHAMM and TLR2,4, which coordinate signaling with CD44 and other HA receptors. Tumor cells hijack the normally tightly regulated HA production/fragmentation associated with wound repair/remodeling, and these HA functions participate in driving and maintaining malignant progression. However, elevated HMW-HA production in the absence of fragmentation is linked to cancer resistance. The controlled production of HA polymer sizes and their functions are predicted to be key to dissecting the role of microenvironment in permitting or restraining the oncogenic potential of tissues. This review focuses on the dual nature of HA in cancer initiation vs. resistance, and the therapeutic potential of HA for chemo-prevention and as a target for cancer management.
Introduction
Cancer is the second leading cause of mortality worldwide according to GLOBOCAN estimates, accounting for ~9.6 million death in 2018 (1). Two decades ago, Hanahan et al. proposed the six classic hallmarks during the multi-step development of cancer (sustained proliferative signaling, evasion of growth suppressors, limitless replicative potential, resistance of apoptosis, sustained angiogenesis, and invasion/metastasis) (2). Conceptual advancements in the past two decades have added evasion of immuno-surveillance, elimination of cell energy limitation, genome instability, and the participation of host cells in facilitating tumor initiation and progression (3, 4). Emphasis has historically been placed on the key role of mutations and genomic instability as drivers of tumor initiation and progression. However, the somatic mutation burden in physiologically normal tissues can approach the level of many cancers (5). These somatic mutations increase with age, and include cancer driver genes, which appear to be under strong positive selective pressure. They occur in the absence of evidence for tumorigenic conversion (5), suggesting that driver gene mutations by themselves are insufficient for cancer initiation. Experimental data also predict that the microenvironment can block or permit the oncogenic potential of mutations (6–9). For example, teratocarcinomas transplanted into early embryos do not form tumors but participate in the development of normal tissues (10). Moreover, the tumorigenic potential of genomically unstable breast cancer cell lines can be curtailed by blocking specific signaling pathways from the extracellular matrix (11–13) suggesting the paracrine interactions between the host cells, tumor cells, and the extracellular matrix can disable or enable the oncogenic potential of mutations. Therefore, dissecting the nature of such interactions is critical to identify therapeutic targets that manage tumor initiation, tumor progression, and post-treatment tumor recurrence.
The tissue polysaccharide, hyaluronan (HA), is one example of an extracellular matrix component that participates in cancer initiation and progression (5, 14–16). HA, like many factors, is multifunctional and has both tumor-promoting and -suppressing properties. HA is an anionic, linear, non-sulfated glycosaminoglycan (GAGs) composed of repeating disaccharide units of glucuronic acid, and N-acetylglucosamine that are synthesized by hyaluronan synthases (HAS1-3) and that can achieve molecular weights in excess of 1,000 kDa. Fragmentation of these large polymers by free radicals and hyaluronidases (HYAL1-3) generate low molecular-weight HA varying from 1 to 500 kDa. Despite its simple primary chemical structure, the large range of polymer sizes generates diverse physiochemical functions and signaling properties (14, 15). High molecular weight HA (HMW-HA, defined here as >500 kDa and in text) predominates in normal tissues, where it provides a scaffold for protein interactions and is essential in maintaining tissue homeostasis. The viscoelastic properties of HMW-HA contribute to porosity and malleability of extracellular matrices (e.g., stem cell niches) (17–20), which are important for resistance to somatic mutation, protection against mechanical damage, and regulating cell trafficking. HMW-HA is also anti-inflammatory and anti-proliferative, which may contribute to tumor resistance in normal tissues (21–24). Fragmentation of HMW-HA into low molecular-weight polymers (LMW-HA, defined here as 7–200 kDa, Figures 1A–D and in text) is minimal or absent in homeostatic tissues, but is increased during response-to-injury and remodeling events in the embryo and adult (25, 26) due to expression of HYAL1-3 and generation of ROS/NOS. LMW-HA activates signaling cascades that promote cell migration, proliferation, immune cell influx, and mesenchymal cell trafficking (27, 28) (Figures 1A–C). This review highlights the consequence of these opposing functions of HA polymer sizes to tumor resistance, initiation and progression. The potential of HA polymers and the processing/signaling machinery for these polymers as therapeutic targets in cancer prevention and management is emphasized.
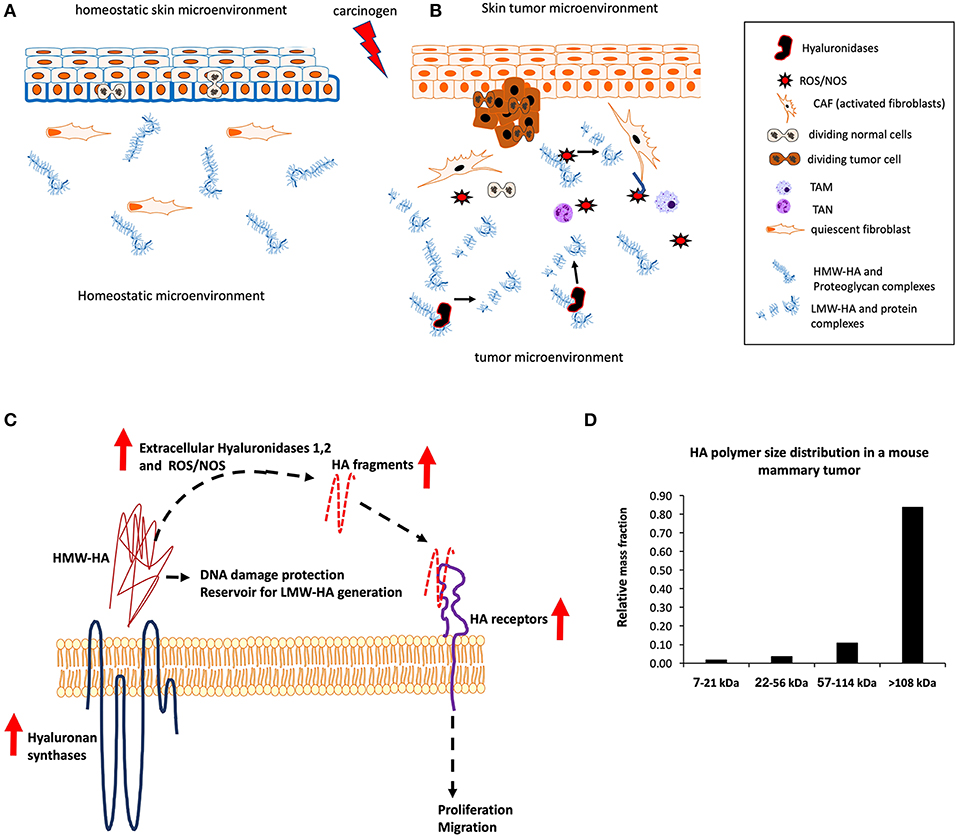
Figure 1. Hyaluronan synthesis and fragmentation in normal and tumor microenvironments. (A) Homeostatic skin is characterized by an organized epidermis with the regulated symmetric and asymmetric division of basal keratinocytes. These are covered by layers of HMW-HA coats (blue outline). Dermal fibroblasts are quiescent and HMW-HA is organized into complexes with proteins and proteoglycans. Extracellular LMW-HA accumulation is restricted. (B) Tumor-initiating events result in disorganized growth of epidermal cells and changes to HA organization and processing. HA synthesis increases, hyaluronidases are expressed/released and reactive oxygen/nitrogen species (ROS/NOS) production is high, resulting in HA fragmentation and reduced organization of macromolecular complexes. HMW-HA coats around epidermal cells are reduced. LMW-HA activates fibroblasts (CAFs, cancer-associated or activated fibroblasts) and attracts immune cells [tumor-associated neutrophils (TANs) and tumor-associated macrophages (TAMs)] that produce ROS/NOS. The fragmented and disorganized tumor microenvironment supports tumor proliferation and evasion of immune surveillance. (C) In disease states such as tumors or chronically inflamed tissues, native or HMW-HA synthesis is increased by constitutively elevated HAS expression. LMW-HA accumulates due to increased expression and activity of extracellular hyaluronidase activity and reactive oxygen/nitrogen species (ROS/NOS) produced by stressed tissues. LMW-HA activates pro-migratory and proliferation pathways through CD44, RHAMM and TLR2,4, whose expression is also increased. In a disease such as cancer, HMW-HA contributes to a stem cell-like microenvironment that is immuno-suppressive and may protect tumor cells from DNA damage. (D) The continued accumulation of HMW HA polymers also provides a source for generating LMW-HA that accumulates in tumor microenvironments as shown in (A). These fragments can be targeted by peptide mimetics that bind and sequestering them, preventing their activation of pro-migration and proliferation signaling pathways.
The Hyaluronome
HA is uniquely synthesized by plasma membrane HAS. Polymers are initiated on the cytoplasmic face of these proteins, then extruded through pores created by aggregated HAS into the ECM (29–32). The three HAS isoforms are encoded on different chromosomes and exhibit distinct tissue distribution and enzymatic properties (31, 33, 34). HA polymers are degraded by three major hyaluronidases (HYAL1-3). HYAL1 and 3 are primarily located in the lysosome and, together with glucosaminidases and glucuronidases (35), degrade HA polymers into monomers. HYAL2 is located at the cell surface, which together with extracellular reactive oxygen/nitrative species (ROS/NOS), generate extracellular LMW-HA (36). These HMW- and LMW-HA polymers bind to CD44, RHAMM, LYVE1, TLR2/4, STAB2, and LAYILLIN (37). HA receptors lack kinase activity and signal by associating with growth factor receptors (e.g., EGFR, PDGFR, and TGFBR) (38, 39). One function of HA:HA receptor interactions is to regulate receptor clustering and membrane localization (40). These critical HA functions are mediated by polymer size and the size preference of HA receptors. For example, while CD44, RHAMM and TLR2,4 bind to a range of sizes, RHAMM and TLR2,4 preferentially bind to very low molecular-weight HA (<7 kDa) (4, 37). The functional complexity of HA polymer signaling is additionally generated by the extensive and often tissue/stimulus-specific alternative splicing of CD44 (41, 42), tissue-specific expression of LYVE1 (lymphatics), and STAB2 (liver) (43–46). The multi-compartmentalization, stress- and stem cell-specific expression of RHAMM, as well as cell context-dependent interaction of HA receptors with each other (e.g., RHAMM/CD44, RHAMM/TLR2,4) also influence HA-mediated signaling (37, 38).
How this HA signaling machinery functions in tumorigenesis can be complex and unpredictable. For example, CD44 can perform tumor-promoting or -suppressing functions depending on its association with protein partners (47). Despite its elevated expression on progenitor-like tumor-initiating cells, deletion of CD44 in a mouse model of luminal breast cancer susceptibility increases rather than decreases metastases. Similarly, either increasing or decreasing intracellular RHAMM expression will de-regulate its functions in centrosome and spindles, increasing the potential for generating genomic instability and enhancing tumorigenesis (48). Such dual properties make direct therapeutic targeting of HA receptors challenging. Nevertheless, de-regulated HA metabolism/signaling typifies many cancers and often predicts poor clinical outcome (49–56). Therefore, developing an understanding of HA metabolism/signaling as a microenvironmental factor that contributes to cancer susceptibility, initiation and progression is likely to identify therapeutic avenues for managing this disease.
Hyaluronan: a Double-Edged Sword in Cancer?
The tumor microenvironment is emerging as a critical factor in cancer progression and recurrence. HA production and accumulation in both the stroma and tumor parenchyma is characteristic of prostate, bladder, lung, breast and other cancers, and is linked to poor clinical outcome (49, 51, 52, 57–59). The majority of these studies do not report the molecular-weight ranges of HA present in the tumor samples. However, the increased expression of HA receptors that preferentially bind to LMW-HA (e.g., RHAMM), increased HYAL expression (e.g., HYAL1,2) (49, 50, 55, 57, 60, 61), and elevated ROS levels predict that LMW-HA is elevated. However, It is likely that both HMW and LMW-HA are increased in tumor microenvironments and that both contribute to tumor survival, growth and aggression (Figures 1B,C). Data mining using TCGA data sets in the cBioPortal for Cancer Genomics (www.cbioportal.org) provides unbiased evidence the association of increased HA production and fragmentation with cancer patient survival. For example, increases in hyaluronidases, HAS and HA receptors are significantly linked to reduced survival in colorectal (e.g., HAS2, HMMR, CD44, HYAL1, p = 0.013) (62), and breast [e.g., HAS2, HMMR, HYAL2, p = 0.023, (63) and p = 5.24e-3(64)] cancers.
Experimental studies provide direct evidence for the importance of elevated HA production in tumor initiation and progression. As examples, MMTV-Neu mice over-expressing a HAS2 transgene exhibit increased mammary tumor incidence and growth compared to wildtype animals (65). HAS2-mediated acceleration of tumor progression results in part from resistance to apoptosis caused by constitutive activation of PI3K/AKT signaling (65, 66). Conversely, HAS2 knockdown in metastatic breast cancer cell lines arrests tumor cells in G0/G1 as a result of reduced cyclin A, B, and cdc2 expression (67). Stable knockdown of this HAS in MDA-MB-231 breast cancer cells decreases their invasion, which is rescued by HAS2 re-expression (66). Conversely, cell sorting of breast tumor cell lines that bind to high levels of HA identifies highly invasive, metastatic subpopulations (68). Experimental evidence from these types of studies suggests that elevated HMW-HA accumulation in either the peri-tumor stroma or tumor parenchyma performs several key functions that favor tumor growth in addition to protection from apoptosis. These include reducing uptake of chemotherapeutic drugs by regulating drug transport proteins, reducing neo-angiogenesis, and reducing drug diffusion into the tumor by increasing local tissue hydrostatic pressure (69–71). HMW-HA reduces immune surveillance at least in part by blocking neo-angiogenesis. Importantly, abundant HMW-HA polymers also provide a stable source for generating LMW-HA.
An example of evidence supporting this latter function is provided by the enhanced invasion of tumor cells when they are transfected with both HAS2 and hyaluronidases (72). HYAL1 knockdown reduces tumorigenicity of breast cancer cell lines (73), while forced expression of HYAL1 promotes tumor cell growth and migration in culture and in vivo (74, 75). LMW-HA polymers promote neo-angiogenesis, tumor cell migration, invasion, and proliferation (16). LMW-HA also attracts macrophages, which polarize into subpopulations that protect tumor cells from adaptive immune cell killing (76). This fragment-specific signaling is mediated by HA receptors, the best studied of which are RHAMM and CD44. Tumor cell migration stimulated by LMW-HA is often associated with an epithelial-mesenchymal transition mediated by CD44 through activating PI3K/AKT and TGFβ signaling (65), while RHAMM is linked to increasing tumor cell migration via regulating ERK (77–79) and AURKA/beta-catenin pathways (80, 81). This signaling not only impacts the functional properties of tumor cells but also regulates stromal cells properties. Thus, LMW-HA/CD44/RHAMM binding promotes angiogenic capillary invasion into the tumor microenvironment. In MMTV-Neu mice, elevated LMW-HA production induces intra-tumoral microvessel formation through promoting angiogenic factor secretion, and enhanced type I collagen, fibronectin, bFGF, CD31 mRNA expression (65, 74). Further, bFGF-induced angiogenesis is accelerated in the presence of LMW-HA (74).
LMW-HA in the tumor microenvironment also promotes monocyte recruitment and differentiation of cytotoxic M1 subtypes into the M2 subtype by altering the Th1/Th2 cytokine balance, thereby enhancing local ROS level, which increases LMW-HA generation and M2 monocyte accumulation (76). This appears to have clinical relevance since both increased M2-like tumor-associated macrophages (TAMs) and LMW-HA accumulation correlate with tumor metastatic potential and poor prognosis in breast cancer patients (54). Both CD44 antibodies and LMW-HA binding peptides reduce monocyte activation and M2 polarization predict that these effects are modulated by LMW-HA:CD44 interactions(76). Moreover, tumor-derived LMW-HA promotes tolerance of tumor cells to infiltrating macrophages (82). This tumoricidal neutralization is suggested to involve IRAK-M (interleukin-1 receptor-associated kinase M), with LMW-HA as an extracellular modulator through both TLR2,4 and CD44 (82).
Collectively, clinical and experimental studies show the importance of tumor or peri-tumor stromal cell HA production and fragmentation for tumor progression. Targeting HA may therefore provide promising therapeutic approaches in cancer management and treatment.
Therapeutic Approaches for Reducing HA Production and Fragmentation to Control Tumorigenesis
Inhibiting HA Synthesis
4-Methylumbelliferone (4-MU) is an inhibitor that depletes one of the building blocks (glucuronic acid, GA) of HA synthesis (83, 84). In 4-MU treated mammalian cells, UDP-transferase catalyzes the transfer of GA onto 4-MU, thus depleting the pool of cytoplasmic UDP-GA and inhibiting HA synthesis (83, 85). 4-MU also decreases HAS2/3 expression (60–80% in cancer cell lines) (86). This suppression is accompanied by reduced CD44 and RHAMM expression, suggesting a feedback loop between HA synthesis and receptor expression (86). HA-mediated downstream signaling is therefore inhibited following 4-MU administration with a consequent reduction in proliferation, migration, and invasion of cancer cells. 4-MU reduces metastasis in skin, lung, osteosarcoma, and breast cancer xenograft models(85, 87–91). Dietary incorporation of 4-MU is also associated with enhanced chemo-prevention: daily ingestion of 4-MU (450 mg/kg) for 28 weeks abrogated prostate tumor initiation and metastasis in experimental models (91). Nevertheless, this approach is not specific for tumor-associated HA and would also block the desirable functions of HMW-HA in normal tissues.
Targeting LMW-HA
Blocking Hyaluronidase Activity
Natural derivatives (i.e., heparin, glycyrrhizic acid, and sodium aurothiomalate) and drugs (i.e., fenoprofen) targeting HYAL isoforms have been investigated as therapeutic agents (26, 85, 88). Originally described as urinary HYAL inhibitors, O-sulfated HA derivatives (sHA) exhibit potent inhibition of HYAL1 activity (IC50: 0.0083–0.019 μM) through binding to allosteric sites (92, 93). Moreover, sHA exhibits more effective non-competitive than competitive inhibition, suggesting its efficacy would not be impeded by elevated HA concentration in tumor tissues. In a prostate cancer animal model, sHA-application inhibited HYAL1 activity and triggered apoptosis through the extrinsic pathway (70). The downregulation of HYAL1 correlated with reduced CD44 and RHAMM expression, and PI3K/AKT signaling, suggesting a feedback mechanism between HA degradation and signaling in tumor cells. The proliferative and invasive capacities of prostate cancer cells are also decreased significantly after sHA-treatment (69).
Conversely, increased degradation of stromal HA by PEGPH20 (a pegylated hyaluronidase) in pancreatic cancer degrades the HA capsule that accumulates around these tumor cells to allow better exposure of tumor cells to chemotherapy and release suppression of neo-angiogenesis (94). PEGPH20 efficacy in facilitating a response of pancreatic tumor cells to chemotherapy is currently being assessed in Phase 3 clinical trials for metastatic pancreatic ductal adenocarcinoma (ClinicalTrials.gov) (94). PEGPH20 administration in animal models of pancreatic cancer induced vascular collapse in tumors and dramatically elevated the interstitial fluid pressure, enhancing perfusion, and delivery of chemotherapeutic drugs into the solid tumor (71). Thus, combined administration of PEGPH20 and other chemotherapy (i,e: gemcitabine) promoted survival and reduced tumor growth in mouse models through inducing apoptosis and suppressing proliferation (71).
Sequestering HA Fragments
The use of peptides or small molecules that bind to the HA binding regions of HA receptors, such as CD44 and RHAMM, is another therapeutic approach that attempts to sequester LMW-HA, thus preventing these from interacting with receptors to abrogate signal activation. To date, extensive use of peptides to block tumorigenesis has not yet been reported. However, RHAMM- or HA-binding peptides can inhibit invasion and survival of prostate and breast cancer cells in culture and in vivo (95, 96). LMW-HA binding peptides have been isolated by screening phage display libraries (97, 98) and by designing RHAMM-like peptides that bind to LMW-HAs with nM affinity (95). These have been shown to block inflammation and fibrosis in both cell culture and animal models (95). Since some cancers originate from progenitor-like cancer-initiating cells, which commonly express CD44, small molecule inhibitors of CD44:HA binding are also being developed (99). However, polysaccharide:protein interactions in general and HA-CD44 binding in particular occur over large surfaces, making design of small molecule inhibitors challenging (95). The amounts of LMW-HAs that accumulate in the peri-tumor stroma is surprisingly small (Figure 1D). These low amounts predict the use of LMW-HA binding peptides may be one of the most effective approaches for targeting the tumorigenic properties tumor microenvironments.
HMW-HA, Hyaluronidase, and CD44 as Tumor Prevention Mechanisms
HMW-HA can also suppress tumor initiation in cancer-prone species by inhibiting proliferation, migration/invasion and ROS-mediated DNA damage. HMW-HA blocks proliferation by signaling through CD44 to promote G1/G0 arrest (100) and, as noted above, alter tumor growth kinetics by suppressing neo-angiogenesis and immune responses (101). Consistent with these reported functions, HMW-HA suppresses growth of murine astrocytoma cell lines, glioma and colon carcinoma xenografts. HMW-HA has also been reported to reduce the migratory and invasive capacity of aggressive cancer cells (23, 102), which could occur by several mechanisms. These include: (1) HMW-HA strengthens cell-cell junctions and decreases the permeability of ECM, thus preventing invasion (103); (2) HMW-HA promotes tight junction formation and myosin polymerization in lymphatics by displacing LMW-HA from the LYVE-1 receptor, which restricts invasion into lymph nodes (103); (3) HMW-HA blocks migration by enhancing NF2 and CD44 co-association, which activates the tumor suppressor properties of CD44 (24); and (4) HMW-HA modifies the motogenic effects of growth factor signaling. As an example, bFGF-treatment decreases sarcoma cell migration as a result of increased HAS1, HAS2, and CD44 expression combined with a reduced expression of HYAL2 (74), which favors accumulation of HMW-HA(55). Further, the addition of HMW-HA reduced fibrosarcoma cell migration, which can be overridden by exogenous LMW-HA or HAS1 knockdown (74). In addition to these growth- and migratory-suppressing effects, HMW-HA also reduces ROS-mediated DNA damage, which lessens mutational burden and thereby reduces the risk of neoplastic transformation (36, 104).
While the above studies predict functions of HMW-HA that could contribute to tumor resistance in cancer-prone species, such as mice and humans, the cancer-resistant naked mole-rat (Heterocephalus glaber) provided the first direct evidence for HMW-HA in suppressing carcinogen-induced tumorigenesis(24, 105). In H. glaber, the combination of HAS2 overexpression, strongly reduced hyaluronidase activity, and signaling through CD44 provides resistance to several carcinogenic insults, including UVB and activated RAS (24). Interestingly, HMW-HA accumulates in some tissues, notably skin, and remains high throughout the lifetime of this animal (24). In contrast, HMW-HA declines in skin and most tissues of human and mouse with age (106–110) and after chronic exposure to UVB, which is the predominant carcinogen causing keratinocyte tumors.
The naked mole-rat utilizes many tumor resistance mechanisms, including the HMW-HA-regulated mechanism termed “early contact inhibition” (ECI), a robust form of contact inhibition that involves expression of a novel isoform of p16INK4A (105). HA induces early expression at the p16INK4A locus through CD44 binding, resulting in blocked phosphorylation of Rb and attenuation of cell cycle. Naked mole-rat fibroblasts cultured in the presence of hyaluronidase display no ECI and downregulate p16INK4A expression (16). This effect of HMW-HA is mediated by CD44:NF2 signaling (24, 105) (Figure 2A).The growth-inhibiting non-phosphorylated form of NF2 is predominant in cultured naked mole-rat cells, but the addition of hyaluronidase stimulates NF2 phosphorylation, which promotes cell growth (24).
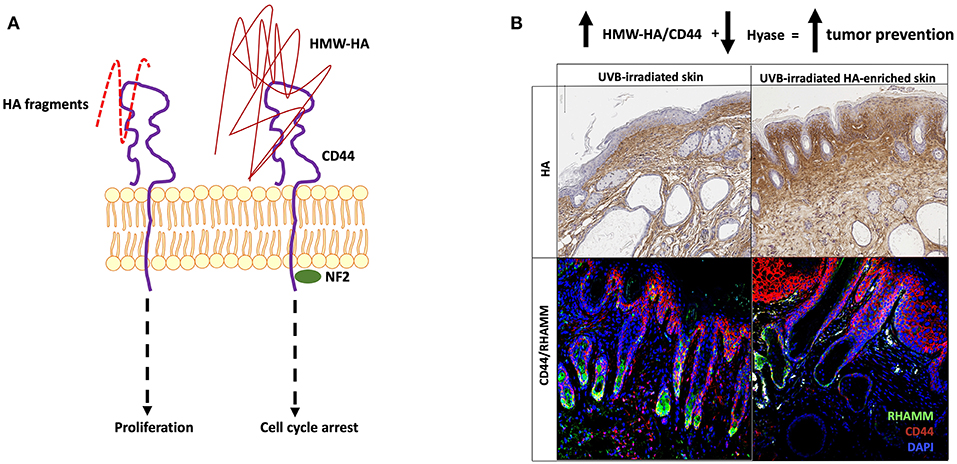
Figure 2. HMW-HA functions in tumor prevention. (A) LMW-HA:CD44 interactions promote proliferation while HMW-HA:CD44 interactions suppress proliferation, which can be mediated by an association of CD44 with the tumor suppressor, Merlin (NF2). HMW-HA:CD44 signals result in cell cycle arrest and are one mechanism for reducing susceptibility to cancer. (B) Dorsal skin of UVB-exposed keratinocyte tumor-susceptible mice treated topically with HMW-hyaluronan-phosphatidylethanolamine (HA-enriched) do not form tumors, accumulate higher levels of HMW-HA (top panels) and CD44 (bottom panels), and lower levels of hyaluronidases than UVB-irradiated controls. Epidermal HA (brown) is detected via an HA-binding biotinylated protein on paraffin-embedded tissue counterstained with hematoxylin (blue). CD44 (red) and RHAMM (green) are detected using pan-antibodies in dorsal skin of mice counterstained with DAPI (blue).
HMW-HA as a Chemoprevention Strategy
To date, HMW-HA has not been used as a chemoprevention strategy, although oral consumption has been shown to restrict tissue inflammation, notably in the bowel (111). The barrier to topical application of HMW-HA has historically been its restricted passage through the outer cornified layer of the epidermis. However, the recent development of high molecular-weight hyaluronan-phosphatidylethanolamine polymers [E. Turley (2010), Patent number: US20130059769A1] that cross the epidermis and form coats around keratinocytes and dermal cells (112) will permit an assessment of whether or not increasing skin HMW-HA can reduce the predisposition of skin to tumorigenesis in cancer-susceptible species (Figure 2B).
Conclusions
In summary, LMW-HA augments the proliferative and migratory capacities of tumor cells, while HMW-HA reduces tumorigenicity and confers cancer resistance by restricting proliferation, limiting inflammation, neo-angiogenesis, and possibly DNA damage. Further research is required to harvest the full therapeutic potential of targeting LMW-HA polymers and utilizing the tumor resistance properties of HMW-HA. Improved understanding of the mechanisms augmenting the size-dependent biological effects of HA is likely to advance new therapeutic development to limit tumorigenesis.
Author Contributions
ML provided the first draft of the manuscript, which was edited by ET and CT. CT and ML provided the data for figures. ET assembled all figures presented in this review.
Conflict of Interest Statement
The authors declare that the research was conducted in the absence of any commercial or financial relationships that could be construed as a potential conflict of interest.
Acknowledgments
This work was supported by a Cancer Research Society Grant (#22491) to ET. ET and ML were supported by the Translational Breast Cancer Research Unit through the Breast Cancer Society of Canada. ML was also supported by the Interdisciplinary Development Initiative in Stem Cells and Regenerative Medicine Scholarship (R3170A15) through Western University.
References
1. Bray F, Ferlay J, Soerjomataram I, Siegel RL, Torre LA, Jemal A. Global cancer statistics 2018: GLOBOCAN estimates of incidence and mortality worldwide for 36 cancers in 185 countries. CA Cancer J Clin. (2018) 68:394–424. doi: 10.3322/caac.21492
2. Hanahan D, Weinberg RA. The hallmarks of cancer. Cell. (2000) 100:57–70. doi: 10.1016/S0092-8674(00)81683-9
3. Hanahan D, Weinberg RA. Hallmarks of cancer: the next generation. Cell. (2011) 144:646–74. doi: 10.1016/j.cell.2011.02.013
4. Tolg C, McCarthy JB, Yazdani A, Turley EA. Hyaluronan and Rhamm in wound repair and the “cancerization” of stromal tissues. Biomed Res Int. (2014) 2014:103923. doi: 10.1155/2014/103923
5. Martincorena I, Roshan A, Gerstung M, Ellis P, Van Loo P, McLaren S, et al. High burden and pervasive positive selection of somatic mutations in normal human skin. Science. (2015) 348:880–6. doi: 10.1126/science.aaa6806
6. Bhat R, Bissell MJ. Of plasticity and specificity: dialectics of the microenvironment and macroenvironment and the organ phenotype. Wiley Interdiscip Rev Dev Biol. (2014) 3:147–63. doi: 10.1002/wdev.130
7. Weaver VM, Gilbert P. Watch thy neighbor: cancer is a communal affair. J Cell Sci. (2004) 117:1287–90. doi: 10.1242/jcs.01137
8. Barcellos-Hoff MH. It takes a tissue to make a tumor: epigenetics, cancer and the microenvironment. J Mammary Gland Biol Neoplasia. (2001) 6:213–21. doi: 10.1023/A:1011317009329
9. Risques RA, Kennedy SR. Aging and the rise of somatic cancer-associated mutations in normal tissues. PLOS Genetics. (2018) 14:e1007108. doi: 10.1371/journal.pgen.1007108
10. Illmensee K, Mintz B. Totipotency and normal differentiation of single teratocarcinoma cells cloned by injection into blastocysts. Proc Natl Acad Sci USA. (1976) 73:549–53. doi: 10.1073/pnas.73.2.549
11. Weaver VM, Petersen OW, Wang F, Larabell CA, Briand P, Damsky C, et al. Reversion of the malignant phenotype of human breast cells in three-dimensional culture and in vivo by integrin blocking antibodies. J Cell Biol. (1997) 137:231–45. doi: 10.1083/jcb.137.1.231
12. Wang F, Hansen RK, Radisky D, Yoneda T, Barcellos-Hoff MH, Petersen OW, et al. Phenotypic reversion or death of cancer cells by altering signaling pathways in three-dimensional contexts. J Natl Cancer Inst. (2002) 94:1494–503. doi: 10.1093/jnci/94.19.1494
13. Hall CL, Turley EA. Hyaluronan: Rhamm mediated cell locomotion and signaling in tumorigenesis. J Neurooncol. (1995) 26:221–9. doi: 10.1007/BF01052625
14. Cyphert JM, Trempus CS, Garantziotis S. Size matters: molecular weight specificity of hyaluronan effects in cell biology. Int J Cell Biol. (2015) 2015:563818. doi: 10.1155/2015/563818
15. Monslow J, Govindaraju P, Puré E. Hyaluronan - A Functional and Structural Sweet Spot in the Tissue Microenvironment. Front Immunol. (2015) 6:231. doi: 10.3389/fimmu.2015.00231
16. Schwertfeger KL, Cowman MK, Telmer PG, Turley EA, McCarthy JB. Hyaluronan, inflammation, and breast cancer progression. Front Immunol. (2015) 6:236. doi: 10.3389/fimmu.2015.00236
17. Ellis SL, Williams B, Asquith S, Bertoncello I, Nilsson SK. An innovative triple immunogold labeling method to investigate the hemopoietic stem cell niche in situ. Microsc Microanal. (2009) 15:403–14. doi: 10.1017/S1431927609990924
18. Gesteira TF, Sun M, Coulson-Thomas YM, Yamaguchi Y, Yeh L-K, Hascall V, et al. Hyaluronan rich microenvironment in the limbal stem cell niche regulates limbal stem cell differentiation. Invest Ophthalmol Vis Sci. (2017) 58:4407–21. doi: 10.1167/iovs.17-22326
19. Simpson RML, Hong X, Wong MM, Karamariti E, Bhaloo SI, Warren D, et al. Hyaluronan is crucial for stem cell differentiation into smooth muscle lineage. Stem Cells. (2016) 34:1225–38. doi: 10.1002/stem.2328
20. Solis MA, Chen Y-H, Wong TY, Bittencourt VZ, Lin Y-C, Huang LLH. Hyaluronan regulates cell behavior: a potential niche matrix for stem cells. Biochem Res Int. (2012) 2012:346972. doi: 10.1155/2012/346972
21. Cowman MK, Lee H-G, Schwertfeger KL, McCarthy JB, Turley EA. The content and size of hyaluronan in biological fluids and tissues. Front Immunol. (2015) 6:261. doi: 10.3389/fimmu.2015.00261
22. Liu Y-Y, Lee C-H, Dedaj R, Zhao H, Mrabat H, Sheidlin A, et al. High-molecular-weight hyaluronan–a possible new treatment for sepsis-induced lung injury: a preclinical study in mechanically ventilated rats. Crit Care. (2008) 12:R102. doi: 10.1186/cc6982
23. Mueller BM, Schraufstatter IU, Goncharova V, Povaliy T, DiScipio R, Khaldoyanidi SK. Hyaluronan inhibits postchemotherapy tumor regrowth in a colon carcinoma xenograft model. Mol Cancer Ther. (2010) 9:3024–32. doi: 10.1158/1535-7163.MCT-10-0529
24. Tian X, Azpurua J, Hine C, Vaidya A, Myakishev-Rempel M, Ablaeva J, et al. High molecular weight hyaluronan mediates the cancer resistance of the naked mole-rat. Nature. (2013) 499:346–9. doi: 10.1038/nature12234
25. D'Agostino A, Stellavato A, Busico T, Papa A, Tirino V, Papaccio G, et al. In vitro analysis of the effects on wound healing of high- and low-molecular weight chains of hyaluronan and their hybrid H-HA/L-HA complexes. BMC Cell Biol. (2015) 16:19. doi: 10.1186/s12860-015-0064-6
26. Ghazi K, Deng-Pichon U, Warnet JM, Rat P. Hyaluronan fragments improve wound healing on in vitro cutaneous model through P2X7 purinoreceptor basal activation: role of molecular weight. PLoS ONE. (2012) 7:e48351. doi: 10.1371/journal.pone.0048351
27. Litwiniuk M, Krejner A, Speyrer MS, Gauto AR, Grzela T. Hyaluronic acid in inflammation and tissue regeneration. Wounds. (2016) 28:78–88.
28. Toole BP. Hyaluronan: from extracellular glue to pericellular cue. Nat Rev Cancer. (2004) 4:528–39. doi: 10.1038/nrc1391
29. Markovitz A, Cifonelli JA, Dorfman A. The biosynthesis of hyaluronic acid by group A Streptococcus. VI. Biosynthesis from uridine nucleotides in cell-free extracts. J Biol Chem. (1959) 234:2343–50.
30. Sayo T, Sugiyama Y, Takahashi Y, Ozawa N, Sakai S, Ishikawa O, et al. Hyaluronan synthase 3 regulates hyaluronan synthesis in cultured human keratinocytes. J Invest Dermatol. (2002) 118:43–8. doi: 10.1046/j.0022-202x.2001.01613.x
31. Törrönen K, Nikunen K, Kärnä R, Tammi M, Tammi R, Rilla K. Tissue distribution and subcellular localization of hyaluronan synthase isoenzymes. Histochem Cell Biol. (2014) 141:17–31. doi: 10.1007/s00418-013-1143-4
32. Weigel PH. Hyaluronan synthase: the mechanism of initiation at the reducing end and a pendulum model for polysaccharide translocation to the cell exterior. Int J Cell Biol. (2015) 2015:367579. doi: 10.1155/2015/367579
33. Itano N, Sawai T, Yoshida M, Lenas P, Yamada Y, Imagawa M, et al. Three isoforms of mammalian hyaluronan synthases have distinct enzymatic properties. J Biol Chem. (1999) 274:25085–92. doi: 10.1074/jbc.274.35.25085
34. Makkonen KM, Pasonen-Seppänen S, Törrönen K, Tammi MI, Carlberg C. Regulation of the hyaluronan synthase 2 gene by convergence in cyclic AMP response element-binding protein and retinoid acid receptor signaling. J Biol Chem. (2009) 284:18270–81. doi: 10.1074/jbc.M109.012492
35. Csoka AB, Frost GI, Stern R. The six hyaluronidase-like genes in the human and mouse genomes. Matrix Biol. (2001) 20:499–508. doi: 10.1016/S0945-053X(01)00172-X
36. Monzon ME, Fregien N, Schmid N, Falcon NS, Campos M, Casalino-Matsuda SM, et al. Reactive oxygen species and hyaluronidase 2 regulate airway epithelial hyaluronan fragmentation. J Biol Chem. (2010) 285:26126–34. doi: 10.1074/jbc.M110.135194
37. Misra S, Hascall VC, Markwald RR, Ghatak S. Interactions between hyaluronan and its receptors (CD44, Rhamm) regulate the activities of inflammation and cancer. Front Immunol. (2015) 6:201. doi: 10.3389/fimmu.2015.00201
38. Turley EA, Wood DK, McCarthy JB. Carcinoma cell hyaluronan as a “portable” cancerized prometastatic microenvironment. Cancer Res. (2016) 76:2507–12. doi: 10.1158/0008-5472.CAN-15-3114
39. Moustakas A, Heldin P. TGFβ and matrix-regulated epithelial to mesenchymal transition. Biochim Biophys Acta. (2014) 1840:2621–34. doi: 10.1016/j.bbagen.2014.02.004
40. Yang C, Cao M, Liu H, He Y, Xu J, Du Y, et al. The high and low molecular weight forms of hyaluronan have distinct effects on CD44 clustering. J Biol Chem. (2012) 287:43094–107. doi: 10.1074/jbc.M112.349209
41. Ghatak S, Hascall VC, Markwald RR, Misra S. Stromal hyaluronan interaction with epithelial CD44 variants promotes prostate cancer invasiveness by augmenting expression and function of hepatocyte growth factor and androgen receptor. J Biol Chem. (2010) 285:19821–32. doi: 10.1074/jbc.M110.104273
42. Misra S, Hascall VC, De Giovanni C, Markwald RR, Ghatak S. Delivery of CD44 shRNA/nanoparticles within cancer cells: perturbation of hyaluronan/CD44v6 interactions and reduction in adenoma growth in Apc Min/+ MICE. J Biol Chem. (2009) 284:12432–46. doi: 10.1074/jbc.M806772200
43. Lawrance W, Banerji S, Day AJ, Bhattacharjee S, Jackson DG. Binding of hyaluronan to the native lymphatic vessel endothelial receptor LYVE-1 is critically dependent on receptor surface clustering and hyaluronan organisation. J Biol Chem. (2016) 291:8014–30. doi: 10.1074/jbc.M115.708305
44. Pandey MS, Weigel PH. Hyaluronic acid receptor for endocytosis (HARE)-mediated endocytosis of hyaluronan, heparin, dermatan sulfate, and acetylated low density lipoprotein (AcLDL), but not chondroitin sulfate types A, C, D, or E, Activates NF-κB-regulated gene expression. J Biol Chem. (2014) 289:1756–67. doi: 10.1074/jbc.M113.510339
45. Sleeman J, Rudy W, Hofmann M, Moll J, Herrlich P, Ponta H. Regulated clustering of variant CD44 proteins increases their hyaluronate binding capacity. J Cell Biol. (1996) 135:1139–50. doi: 10.1083/jcb.135.4.1139
46. van der Voort R, Manten-Horst E, Smit L, Ostermann E, van den Berg F, Pals ST. Binding of cell-surface expressed CD44 to hyaluronate is dependent on splicing and cell type. Biochem Biophys Res Commun. (1995) 214:137–44. doi: 10.1006/bbrc.1995.2267
47. Lopez JI, Camenisch TD, Stevens MV, Sands BJ, McDonald J, Schroeder JA. CD44 attenuates metastatic invasion during breast cancer progression. Cancer Res. (2005) 65:6755–63. doi: 10.1158/0008-5472.CAN-05-0863
48. Tolg C, Hamilton SR, Morningstar L, Zhang J, Zhang S, Esguerra KV, et al. Rhamm promotes interphase microtubule instability and mitotic spindle integrity through MEK1/ERK1/2 activity. J Biol Chem. (2010) 285:26461–74. doi: 10.1074/jbc.M110.121491
49. Bertrand P, Girard N, Duval C, d'Anjou J, Chauzy C, Ménard JF, et al. Increased hyaluronidase levels in breast tumor metastases. Int J Cancer. (1997) 73:327–31. doi: 10.1002/(SICI)1097-0215(19971104)73:3<327::AID-IJC4>3.0.CO;2-1
50. Bouga H, Tsouros I, Bounias D, Kyriakopoulou D, Stavropoulos MS, Papageorgakopoulou N, et al. Involvement of hyaluronidases in colorectal cancer. BMC Cancer. (2010) 10:499. doi: 10.1186/1471-2407-10-499
51. Pirinen R, Tammi R, Tammi M, Hirvikoski P, Parkkinen JJ, Johansson R, et al. Prognostic value of hyaluronan expression in non-small-cell lung cancer: Increased stromal expression indicates unfavorable outcome in patients with adenocarcinoma. Int J Cancer. (2001) 95:12–7. doi: 10.1002/1097-0215(20010120)95:1<12::AID-IJC1002>3.0.CO;2-E
52. Rangel MP, de Sá VK, Martins V, Martins JRM, Parra ER, Mendes A, et al. Tissue hyaluronan expression, as reflected in the sputum of lung cancer patients, is an indicator of malignancy. Braz J Med Biol Res. (2015) 48:557–67. doi: 10.1590/1414-431x20144300
53. Ropponen K, Tammi M, Parkkinen J, Eskelinen M, Tammi R, Lipponen P, et al. Tumor cell-associated hyaluronan as an unfavorable prognostic factor in colorectal cancer. Cancer Res. (1998) 58:342–7.
54. Tiainen S, Tumelius R, Rilla K, Hämäläinen K, Tammi M, Tammi R, et al. High numbers of macrophages, especially M2-like (CD163-positive), correlate with hyaluronan accumulation and poor outcome in breast cancer. Histopathology. (2015) 66:873–83. doi: 10.1111/his.12607
55. McAtee CO, Barycki JJ, Simpson MA. Emerging roles for hyaluronidase in cancer metastasis and therapy. Adv Cancer Res. (2014) 123:1–34. doi: 10.1016/B978-0-12-800092-2.00001-0
56. Wu M, Cao M, He Y, Liu Y, Yang C, Du Y, et al. A novel role of low molecular weight hyaluronan in breast cancer metastasis. FASEB J. (2015) 29:1290–8. doi: 10.1096/fj.14-259978
57. Auvinen P, Rilla K, Tumelius R, Tammi M, Sironen R, Soini Y, et al. Hyaluronan synthases (HAS1-3) in stromal and malignant cells correlate with breast cancer grade and predict patient survival. Breast Cancer Res Treat. (2014) 143:277–86. doi: 10.1007/s10549-013-2804-7
58. Golshani R, Hautmann SH, Estrella V, Cohen BL, Kyle CC, Manoharan M, et al. HAS1 expression in bladder cancer and its relation to urinary HA test. Int J Cancer. (2007) 120:1712–20. doi: 10.1002/ijc.22222
59. Yoshida T, Matsuda Y, Naito Z, Ishiwata T. CD44 in human glioma correlates with histopathological grade and cell migration. Pathol Int. (2012) 62:463–70. doi: 10.1111/j.1440-1827.2012.02823.x
60. Franzmann EJ, Schroeder GL, Goodwin WJ, Weed DT, Fisher P, Lokeshwar VB. Expression of tumor markers hyaluronic acid and hyaluronidase (HYAL1) in head and neck tumors. Int J Cancer. (2003) 106:438–45. doi: 10.1002/ijc.11252
61. Lokeshwar VB, Rubinowicz D, Schroeder GL, Forgacs E, Minna JD, Block NL, et al. Stromal and epithelial expression of tumor markers hyaluronic acid and hyal1 hyaluronidase in prostate cancer. J Biol Chem. (2001) 276:11922–32. doi: 10.1074/jbc.M008432200
62. Cancer Genome Atlas N. Comprehensive molecular characterization of human colon and rectal cancer. Nature. (2012) 487:330–7. doi: 10.1038/nature11252
63. Ciriello G, Gatza ML, Beck AH, Wilkerson MD, Rhie SK, Pastore A, et al. Comprehensive molecular portraits of invasive lobular breast cancer. Cell. (2015) 163:506–19. doi: 10.1016/j.cell.2015.09.033
64. Pereira B, Chin SF, Rueda OM, Vollan HK, Provenzano E, Bardwell HA, et al. The somatic mutation profiles of 2,433 breast cancers refines their genomic and transcriptomic landscapes. Nat Commun. (2016) 7:11479. doi: 10.1038/ncomms11479
65. Koyama H, Hibi T, Isogai Z, Yoneda M, Fujimori M, Amano J, et al. Hyperproduction of hyaluronan in neu-induced mammary tumor accelerates angiogenesis through stromal cell recruitment: possible involvement of versican/PG-M. Am J Pathol. (2007) 170:1086–99. doi: 10.2353/ajpath.2007.060793
66. Bernert B, Porsch H, Heldin P. Hyaluronan synthase 2 (HAS2) promotes breast cancer cell invasion by suppression of tissue metalloproteinase inhibitor 1 (TIMP-1). J Biol Chem. (2011) 286:42349–59. doi: 10.1074/jbc.M111.278598
67. Li Y, Li L, Brown TJ, Heldin P. Silencing of hyaluronan synthase 2 suppresses the malignant phenotype of invasive breast cancer cells. Int J Cancer. (2007) 120:2557–67. doi: 10.1002/ijc.22550
68. Veiseh M, Kwon DH, Borowsky AD, Tolg C, Leong HS, Lewis JD, et al. Cellular heterogeneity profiling by hyaluronan probes reveals an invasive but slow-growing breast tumor subset. Proc Natl Acad Sci USA. (2014) 111:E1731–9. doi: 10.1073/pnas.1402383111
69. Sato N, Cheng XB, Kohi S, Koga A, Hirata K. Targeting hyaluronan for the treatment of pancreatic ductal adenocarcinoma. Acta Pharm Sin B. (2016) 6:101–5. doi: 10.1016/j.apsb.2016.01.002
70. Benitez A, Yates TJ, Lopez LE, Cerwinka WH, Bakkar A, Lokeshwar VB. Targeting hyaluronidase for cancer therapy: antitumor activity of sulfated hyaluronic acid in prostate cancer cells. Cancer Res. (2011) 71:4085–95. doi: 10.1158/0008-5472.CAN-10-4610
71. Provenzano PP, Cuevas C, Chang AE, Goel VK, Von Hoff DD, Hingorani SR. Enzymatic targeting of the stroma ablates physical barriers to treatment of pancreatic ductal adenocarcinoma. Cancer Cell. (2012) 21:418–29. doi: 10.1016/j.ccr.2012.01.007
72. Simpson MA. Concurrent expression of hyaluronan biosynthetic and processing enzymes promotes growth and vascularization of prostate tumors in mice. Am J Pathol. (2006) 169:247–57. doi: 10.2353/ajpath.2006.060032
73. Tan J-X, Wang X-Y, Li H-Y, Su X-L, Wang L, Ran L, et al. HYAL1 overexpression is correlated with the malignant behavior of human breast cancer. Int J Cancer. (2011) 128:1303–15. doi: 10.1002/ijc.25460
74. Berdiaki A, Nikitovic D, Tsatsakis A, Katonis P, Karamanos NK, Tzanakakis GN. bFGF induces changes in hyaluronan synthase and hyaluronidase isoform expression and modulates the migration capacity of fibrosarcoma cells. Biochim Biophys Acta. (2009) 1790:1258–65. doi: 10.1016/j.bbagen.2009.06.013
75. Tan JX, Wang XY, Su XL, Li HY, Shi Y, Wang L, et al. Upregulation of HYAL1 expression in breast cancer promoted tumor cell proliferation, migration, invasion and angiogenesis. PLoS ONE. (2011) 6:e22836. doi: 10.1371/journal.pone.0022836
76. Kuang DM, Wu Y, Chen N, Cheng J, Zhuang S-M, Zheng L. Tumor-derived hyaluronan induces formation of immunosuppressive macrophages through transient early activation of monocytes. Blood. (2007) 110:587–95. doi: 10.1182/blood-2007-01-068031
77. Tolg C, Hamilton SR, Nakrieko KA, Kooshesh F, Walton P, McCarthy JB, et al. Rhamm-/- fibroblasts are defective in CD44-mediated ERK1,2 motogenic signaling, leading to defective skin wound repair. J Cell Biol. (2006) 175:1017–28. doi: 10.1083/jcb.200511027
78. Hamilton SR, Fard SF, Paiwand FF, Tolg C, Veiseh M, Wang C, et al. The hyaluronan receptors CD44 and Rhamm (CD168) form complexes with ERK1,2 that sustain high basal motility in breast cancer cells. J Biol Chem. (2007) 282:16667–80. doi: 10.1074/jbc.M702078200
79. Wang Z, Wu Y, Wang H, Zhang Y, Mei L, Fang X, et al. Interplay of mevalonate and Hippo pathways regulates Rhamm transcription via YAP to modulate breast cancer cell motility. Proc Natl Acad Sci USA. (2014) 111:E89–98. doi: 10.1073/pnas.1319190110
80. Kouvidi K, Nikitovic D, Berdiaki A, Tzanakakis GN. Hyaluronan/Rhamm interactions in mesenchymal tumor pathogenesis: role of growth factors. Adv Cancer Res. (2014) 123:319–49. doi: 10.1016/B978-0-12-800092-2.00012-5
81. Chen H, Mohan P, Jiang J, Nemirovsky O, He D, Fleisch MC, et al. Spatial regulation of Aurora A activity during mitotic spindle assembly requires Rhamm to correctly localize TPX2. Cell Cycle. (2014) 13:2248–61. doi: 10.4161/cc.29270
82. del Fresno C, Otero K, Gómez-García L, González-León MC, Soler-Ranger L, Fuentes-Prior P, et al. Tumor cells deactivate human monocytes by up-regulating IL-1 receptor associated kinase-M expression via CD44 and TLR4. J Immunol. (2005) 174:3032–40. doi: 10.4049/jimmunol.174.5.3032
83. Nagy N, Kuipers HF, Frymoyer AR, Ishak HD, Bollyky JB, Wight TN, et al. 4-methylumbelliferone treatment and hyaluronan inhibition as a therapeutic strategy in inflammation, autoimmunity, and cancer. Front Immunol. (2015) 6:123. doi: 10.3389/fimmu.2015.00123
84. Kakizaki I, Kojima K, Takagaki K, Endo M, Kannagi R, Ito M, et al. A Novel Mechanism for the inhibition of hyaluronan biosynthesis by 4-methylumbelliferone. J Biol Chem. (2004) 279:33281–9. doi: 10.1074/jbc.M405918200
85. Lokeshwar VB, Mirza S, Jordan A. Targeting hyaluronic acid family for cancer chemoprevention and therapy. Adv Cancer Res. (2014) 123:35–65. doi: 10.1016/B978-0-12-800092-2.00002-2
86. Kultti A, Pasonen-Seppänen S, Jauhiainen M, Rilla KJ, Kärnä R, Pyöriä E, et al. 4-Methylumbelliferone inhibits hyaluronan synthesis by depletion of cellular UDP-glucuronic acid and downregulation of hyaluronan synthase 2 and 3. Exp Cell Res. (2009) 315:1914–23. doi: 10.1016/j.yexcr.2009.03.002
87. Arai E, Nishida Y, Wasa J, Urakawa H, Zhuo L, Kimata K, et al. Inhibition of hyaluronan retention by 4-methylumbelliferone suppresses osteosarcoma cells in vitro and lung metastasis in vivo. Br J Cancer. (2011) 105:1839–49. doi: 10.1038/bjc.2011.459
88. Balazs EA, Högberg B, Laurent TC. The biological activity of hyaluron sulfuric acid. Acta Physiologica Scandinavica. (1951) 23:168–78. doi: 10.1111/j.1748-1716.1951.tb00806.x
89. Bhattacharyya SS, Paul S, Mandal SK, Banerjee A, Boujedaini N, Khuda-Bukhsh AR. A synthetic coumarin (4-methyl-7 hydroxy coumarin) has anti-cancer potentials against DMBA-induced skin cancer in mice. Eur J Pharmacol. (2009) 614:128–36. doi: 10.1016/j.ejphar.2009.04.015
90. Urakawa H, Nishida Y, Knudson W, Knudson CB, Arai E, Kozawa E, et al. Therapeutic potential of hyaluronan oligosaccharides for bone metastasis of breast cancer. J Orthop Res. (2012) 30:662–72. doi: 10.1002/jor.21557
91. Yates TJ, Lopez LE, Lokeshwar SD, Ortiz N, Kallifatidis G, Jordan A, et al. Dietary supplement 4-methylumbelliferone: an effective chemopreventive and therapeutic agent for prostate cancer. J Natl Cancer Inst. (2015) 107:85. doi: 10.1093/jnci/djv085
92. Isoyama T, Thwaites D, Selzer MG, Carey RI, Barbucci R, Lokeshwar VB. Differential selectivity of hyaluronidase inhibitors toward acidic and basic hyaluronidases. Glycobiology. (2006) 16:11–21. doi: 10.1093/glycob/cwj036
93. Toida T, Ogita Y, Suzuki A, Toyoda H, Imanari T. Inhibition of hyaluronidase by fully O-sulfonated glycosaminoglycans. Arch Biochem Biophys. (1999) 370:176–82. doi: 10.1006/abbi.1999.1395
94. Hendifar A, Bullock A. Breaking the barrier-PEGylated recombinant human hyaluronidase (PEGPH20)-A new therapeutic approach to the treatment of pancreatic ductal adenocarcinoma. breaking the barrier-pegylated recombinant human hyaluronidase (pegph20)-a new therapeutic approach to the treatment of pancreatic ductal adenocarcinoma. Oncology. (2017) 13:107–11. doi: 10.17925/OHR.2017.13.02.107
95. Hauser-Kawaguchi A, Luyt LG, Turley E. Design of peptide mimetics to block pro-inflammatory functions of HA fragments. Matrix Biol. (2018). doi: 10.1016/j.matbio.2018.01.021
96. Liu N, Xu XM, Chen J, Wang L, Yang S, Underhill CB, et al. Hyaluronan-binding peptide can inhibit tumor growth by interacting with Bcl-2. Int J Cancer. (2004) 109:49–57. doi: 10.1002/ijc.11636
97. Tolg C, Hamilton SR, Zalinska E, McCulloch L, Amin R, Akentieva N, et al. A Rhamm mimetic peptide blocks hyaluronan signaling and reduces inflammation and fibrogenesis in excisional skin wounds. Am J Pathol. (2012) 181:1250–70. doi: 10.1016/j.ajpath.2012.06.036
98. Mummert ME. Immunologic roles of hyaluronan. Immunol Res. (2005) 31:189–206. doi: 10.1385/IR:31:3:189
99. Liu L-K, Finzel B. High-resolution crystal structures of alternate forms of the human CD44 hyaluronan-binding domain reveal a site for protein interaction. Acta Crystallogr F Struct Biol Commun. (2014) 70(Pt 9):1155–61. doi: 10.1107/S2053230X14015532
100. Takabe P, Bart G, Ropponen A, Rilla K, Tammi M, Tammi R, et al. Hyaluronan synthase 3 (HAS3) overexpression downregulates MV3 melanoma cell proliferation, migration and adhesion. Exp Cell Res. (2015) 337:1–15. doi: 10.1016/j.yexcr.2015.07.026
101. Deed R, Rooney P, Kumar P, Norton JD, Smith J, Freemont AJ, et al. Early-response gene signalling is induced by angiogenic oligosaccharides of hyaluronan in endothelial cells. Inhibition by non-angiogenic, high-molecular-weight hyaluronan. Int J Cancer. (1997) 71:251–6. doi: 10.1002/(SICI)1097-0215(19970410)71:2<251::AID-IJC21>3.0.CO;2-J
102. Enegd B, King JAJ, Stylli S, Paradiso L, Kaye AH, Novak U. Overexpression of hyaluronan synthase-2 reduces the tumorigenic potential of glioma cells lacking hyaluronidase activity. Neurosurgery. (2002) 50:1311–8. doi: 10.1097/00006123-200206000-00023
103. Yu M, He P, Liu Y, He Y, Du Y, Wu M, et al. Hyaluroan-regulated lymphatic permeability through S1P receptors is crucial for cancer metastasis. Med Oncol. (2015) 32:381. doi: 10.1007/s12032-014-0381-1
104. Yagci A, Murk W, Stronk J, Huszar G. Spermatozoa bound to solid state hyaluronic acid show chromatin structure with high DNA chain integrity: an acridine orange fluorescence study. J Androl. (2010) 31:566–72. doi: 10.2164/jandrol.109.008912
105. Seluanov A, Hine C, Azpurua J, Feigenson M, Bozzella M, Mao Z, et al. Hypersensitivity to contact inhibition provides a clue to cancer resistance of naked mole-rat. Proc Natl Acad Sci USA. (2009) 106:19352–7. doi: 10.1073/pnas.0905252106
106. Meyer LJ, Stern R. Age-dependent changes of hyaluronan in human skin. J Invest Dermatol. (1994) 102:385–9. doi: 10.1111/1523-1747.ep12371800
107. Dai G, Freudenberger T, Zipper P, Melchior A, Grether-Beck S, Rabausch B, et al. Chronic ultraviolet B irradiation causes loss of hyaluronic acid from mouse dermis because of down-regulation of hyaluronic acid synthases. Am J Pathol. (2007) 171:1451–61. doi: 10.2353/ajpath.2007.070136
108. Röck K, Grandoch M, Majora M, Krutmann J, Fischer JW. Collagen fragments inhibit hyaluronan synthesis in skin fibroblasts in response to ultraviolet B (UVB) new insights into mechanisms of matrix remodeling. J Biol Chem. (2011) 286:18268–76. doi: 10.1074/jbc.M110.201665
109. Stern R, Maibach HI. Hyaluronan in skin: aspects of aging and its pharmacologic modulation. Clin Dermatol. (2008) 26:106–22. doi: 10.1016/j.clindermatol.2007.09.013
110. Papakonstantinou E, Roth M, Karakiulakis G. Hyaluronic acid: a key molecule in skin aging. Dermatoendocrinol. (2012) 4:253–8. doi: 10.4161/derm.21923
111. Asari A, Kanemitsu T, Kurihara H. Oral administration of high molecular weight hyaluronan (900 kDa) controls immune system via Toll-like receptor 4 in the intestinal epithelium. J Biol Chem. (2010) 285:24751–8. doi: 10.1074/jbc.M110.104950
Keywords: hyaluronan, hyaluronan receptors, tumor microenvironment, cancer resistance, tumor initiation, CD44, RHAMM
Citation: Liu M, Tolg C and Turley E (2019) Dissecting the Dual Nature of Hyaluronan in the Tumor Microenvironment. Front. Immunol. 10:947. doi: 10.3389/fimmu.2019.00947
Received: 04 December 2018; Accepted: 12 April 2019;
Published: 10 May 2019.
Edited by:
Hiroto Kawashima, Chiba University, JapanReviewed by:
Vincent Charles Hascall, Cleveland Clinic Lerner College of Medicine, United StatesSuniti Misra, Medical University of South Carolina, United States
Copyright © 2019 Liu, Tolg and Turley. This is an open-access article distributed under the terms of the Creative Commons Attribution License (CC BY). The use, distribution or reproduction in other forums is permitted, provided the original author(s) and the copyright owner(s) are credited and that the original publication in this journal is cited, in accordance with accepted academic practice. No use, distribution or reproduction is permitted which does not comply with these terms.
*Correspondence: Eva Turley, eva.turley@lhsc.on.ca