- 1Laboratory of Applied Genetics, Socio-Environmental and Water Resources Institute, Federal Rural University of the Amazon, Belém, Brazil
- 2Laboratory of Genomics and Biotechnology, Biological Sciences Institute, Federal University of Pará, Belém, Brazil
- 3Comparative Immunogenetics Laboratory, Department of Veterinary Pathobiology, College of Veterinary Medicine and Biomedical Sciences, Texas A&M University, College Station, TX, United States
- 4Center for Technological Innovation, Evandro Chagas Institute, Belém, Brazil
- 5Center of Biodiversity Advanced Studies, Biological Sciences Institute, Federal University of Pará, Belém, Brazil
Sirenians share with cetaceans and pinnipeds several convergent traits selected for the aquatic lifestyle. Living in water poses new challenges not only for locomotion and feeding but also for combating new pathogens, which may render the immune system one of the best tools aquatic mammals have for dealing with aquatic microbial threats. So far, only cetaceans have had their class II Major Histocompatibility Complex (MHC) organization characterized, despite the importance of MHC genes for adaptive immune responses. This study aims to characterize the organization of the marine mammal class II MHC using publicly available genomes. We located class II sequences in the genomes of one sirenian, four pinnipeds and eight cetaceans using NCBI-BLAST and reannotated the sequences using local BLAST search with exon and intron libraries. Scaffolds containing class II sequences were compared using dotplot analysis and introns were used for phylogenetic analysis. The manatee class II region shares overall synteny with other mammals, however most DR loci were translocated from the canonical location, past the extended class II region. Detailed analysis of the genomes of closely related taxa revealed that this presumed translocation is shared with all other living afrotherians. Other presumptive chromosome rearrangements in Afrotheria are the deletion of DQ loci in Afrosoricida and deletion of DP in E. telfairi. Pinnipeds share the main features of dog MHC: lack of a functional pair of DPA/DPB genes and inverted DRB locus between DQ and DO subregions. All cetaceans share the Cetartiodactyla inversion separating class II genes into two subregions: class IIa, with DR and DQ genes, and class IIb, with non-classic genes and a DRB pseudogene. These results point to three distinct and unheralded class II MHC structures in marine mammals: one canonical organization but lacking DP genes in pinnipeds; one bearing an inversion separating IIa and IIb subregions lacking DP genes found in cetaceans; and one with a translocation separating the most diverse class II gene from the MHC found in afrotherians and presumptive functional DR, DQ, and DP genes. Future functional research will reveal how these aquatic mammals cope with pathogen pressures with these divergent MHC organizations.
Introduction
The transition from terrestrial to aquatic habitat has occurred in several terrestrial vertebrate lineages. In mammals, early after the Cretaceous period, independent ancestral lineages of afrotherian, cetartiodactyl, and carnivore would begin their path to return to aquatic environments which would lead to current sirenian, cetacean, and pinniped species of marine mammals. Those three lineages have further undergone adaptive radiation and their descendants are found in both oceanic and freshwater habitats.
The order Sirenia is represented by one species of dugong (Dugong dugon) and three species of manatees (Trichechus manatus, T. senegalensis, and T. inunguis), all of them exclusively herbivorous and whose closest living relatives are the elephants. The order Cetacea have approximately 89 species divided into two suborders: Odontoceti (toothed whales) and Mysticeti (baleen whales), and is closely related to hippopotamuses. Pinnipedia comprises a carnivore suborder with three families (Otaridae, Phocidae, and Odobenidae) of around 34 species of aquatic fin-footed mammals (seal, sea-lions, and walrus), closely related to bears and musteloids (e.g., raccoons and skunks), which are still dependent on the land to live, in contrast to sirenians and cetaceans, which are totally adapted to the aquatic environment. Aquatic mammals share several convergent traits selected for fresh water and marine habitats, including morphologic and genetic traits (1–4).
Living in water poses new challenges not only for locomotion and feeding but also for combating new pathogens. How the three major independent aquatic mammal lineages just detailed dealt with the genetic constraints of their ancestry and to what extent their recent history in similar habitats led to convergent evolution in their immune system is not clear. Several marine mammals lack major predators in the adult phase, so infectious disease may be an important cause of mortality (5). This may make the immune system of these aquatic lineages particularly important for their fitness and fecundity. Compared to their terrestrial relatives, marine mammals face distinct diversity of pathogens, disease ecology, and epidemiology (6–8), which may create distinct selective pressures on immune genetic systems, including the Major Histocompatibility Complex (MHC).
The MHC encodes many immune (and many non-immune) genes, canonically divided in class I, II, and III regions in vertebrates. The class II region includes classical (e.g., DR, DQ, DP) MHC, non-classical (e.g., DO, DM) MHC, antigen processing (e.g., TAP, PSMB) and other genes. Classical class II alpha and beta genes encode a protein heterodimer that presents antigens for T lymphocytes to detect infections and other danger signals. Classical MHC genes are highly polymorphic, confer resistance or susceptibility to diseases, and may be used as genetic markers for species conservation (9, 10). Several studies have reported the diversity of class II genes in cetaceans (11–19) and pinnipeds (5, 20–25). Past evidence also showed that class II MHC genes may be important genetic markers for survival in a seal species (5). Despite its proposed importance for marine mammals, so far only a representative of cetacean has had their class II MHC organization characterized (26). Therefore, we aimed to compare the genomic organization and evolution of the MHC class II region in sirenians, cetaceans, and pinnipeds, using genome assemblies from representatives of these groups available in public databases. We also included other mammals from different eutherian lineages for a better understanding of the evolution of marine mammals and the eutherian class II MHC region.
Materials and Methods
MHC Class II Genes Identification and Reannotation
The marine mammals investigated in this research were: the sirenian Florida manatee (Trichechus manatus latirostris); the cetaceans minke whale (Balaenoptera acutorostrata scammoni), sperm whale (Physeter catodon), baiji (Lipotes vexillifer), beluga whale (Delphinapterus leucas), finless porpoise (Neophocaena asiaeorientalis), bottlenose dolphin (Tursiops truncatus), Pacific white-sided dolphin (Lagenorhynchus obliquens), and killer whale (Orcinus orca); and the pinnipeds walrus (Odobenus rosmarus), Northern fur seal (Callorhinus ursinus), Hawaiian monk seal (Neomonachus schauinslandi), and Weddell seal (Leptonychotes wedelli). We included in the analysis other mammals as outgroups and representatives of other major eutherian branches. A summary of the assembly reports from each analyzed species is provided in Supplementary File S1.
Preliminary search on the NCBI database identified annotated MHC class II genes in the genomes of cetaceans, afrotherians, and pinnipeds. All predicted mRNA gene sequences were aligned to their human homologs. We selected presumptive well-annotated classical genes based on the presence of full-length sequences, presence of all exons, and no evidence of pseudogene misidentification (presence of stop codons and lack of homology in any exons). Those predicted genes and their human homologs were used to perform megablast and discontiguous megablast searches in the genomes of marine mammals and other mammals representative of the main eutherian branches. Gene references used were: DMA, NM_006120.3; DMB, NM_002118.4; DOA, NM_002119.3; DOB, NM_002120.3; DRA, NM_019111.4 and XM_007951302.1; DRB, NM_002124.3 and XM_003423461.2; DPA, NM_001242525.1 and XM_006882197.1; DPB, NM_002121.5 and XM_012559980.1; DQA, NM_002122.3 and XM_003421050.1; DQB, NM_001243961.1.
We checked all predicted class II gene and pseudogene sequences for proper annotation using Geneious 9 (27). MAFFT (28) alignments with the predicted coding sequence were used to check for missing or poorly annotated exons. We constructed local BLAST libraries containing exons and introns for each gene and performed blast on scaffolds containing class II sequences. Nomenclature used for class II genes of non-model species included a prefix formed by the first two letters of the genus and species (i.e., Loxodonta africana, Loaf; Trichechus manatus, Trma; Orycteropus afer, Oraf; Elephantulus edwardii, Eled; Chrysochloris asiatica, Chas; Echinops telfairi, Ecte; Dasypus novemcintus, Dano; B. acutorostrata, Baac; D. leucas; Dele; L. vexillifer, Live; N. asiaeorientalis, Neas; O. orca, Oror; L. obliquidens, Laob; T. truncates, Tutr; P. catodon, Phca; C. ursinus, Caur; N. schauinslandi, Nesc; L. weddelli, Lewe; O. rosmarus, Odro; Pteropus alecto, Ptal; Equus caballus, Eqca) (29, 30); BoLA, DLA, H2 and HLA were used for bovine, dog, mouse and human genes, respectively.
Predicted coding sequences with no stop codons or frameshift mutations were presumed to be functional and annotated as genes (including incomplete coding sequence due to assembly gaps); sequences with at least one stop codon or frameshift mutations were annotated as pseudogenes. Thus, for clarity, in this manuscript “locus/loci” will be used when broadly referring to sequences from a gene family, including both presumed genes and pseudogenes; “gene” will be used when referring only to presumed functional sequences; and “pseudogene” will be used for presumed non-functional sequences. New annotations and reannotation CDS, as well as the summary of the loci used in this study are provided here as Supplementary Files S2,S3.
Comparative Genomics Analysis
To construct dot plot graphs, the manatee, Northern fur seal and sperm whale were chosen as representatives of the main marine mammal branches. Scaffolds containing class II genes were first submitted to RepeatMasker (31) and resulting masking files were used along with sequence and gene annotations on PipMaker (32). For the manatee, scaffolds covering the class II MHC region were concatenated. Part of the annotations and sequences from the extended class II region were removed to provide a better view of the identity of key regions.
Phylogenetic Analysis
We constructed phylogenetic trees using the exons and introns from classical MHC class II genes and pseudogenes (when they could be accurately determined). We chose to use only loci located in scaffolds that allowed us to determine their location. Introns were separately aligned using MAFFT online service (33) and the alignments cleaned in GBlocks (34) under default settings and allowing gaps in all sequences. Intron alignments were then concatenated for the rest of the analysis. Exons were also aligned using MAFFT. Best-fit partition scheme and corresponding nucleotide substitution model was checked on PartitionFinder (35); each intron was discriminated for the search of all possible partition schemes and each codon position was treated as a partition. Maximum likelihood trees were constructed in CIPRES (36) using RAxML (37), with 1,000 bootstrap iterations. All phylogenetic tests were performed in triplicate. Trees were constructed on iTOL (38). Exon phylogenies did not change the main conclusions of this study, therefore we present only intron phylogenies since they are more comprehensive.
Results
The Marine Mammal Genomes and Class II MHC Synteny
All marine mammal genomes in our analysis were de novo assembled using varying assembly methods (Supplementary File S1). The manatee genome was made using Illumina Hi-seq technology with a 150x coverage; pinniped genomes were also made using Illumina reads with coverage ranging from 27.44x to 200x; and cetacean genomes were made using Illumina or BGISEQ-500 technology, with coverage ranging from 35.68x to 248x (Supplementary File S1).
The manatee class II sequences were distributed over eight scaffolds, while other marine mammals had their class II MHC distributed on 1–3 scaffolds (Table 1). Overall, we were able to locate one copy of each non-classical gene in all marine mammals, whereas the classical genes varied across taxa. MHC class II genes showed conservation in sequence length and number of exons when compared to human homologs, although many entries were only partial due to gaps in the genome or difficulty in determining exon boundaries.
We chose a representative of each marine mammal lineage to construct dot plot graphs against the human MHC. The manatee class II region maintain the overall synteny compared to human, but all DRB loci are located after the extended class II region (Figure 1A). The Northern fur seal class II region also have the main features of the human MHC class II organization; however it lacks conservation in the DP subregion and possesses an inverted DRB pseudogene between its DQ and DO subregions (Figure 1B). The sperm whale class II region is divided in two subregions due to an inversion separating the DR and DQ genes from the non-classic genes (Figure 1C); the cetacean also lacks identity in the DP subregion and possesses a DRB pseudogene between DOB and GCLC (Figure 1C).
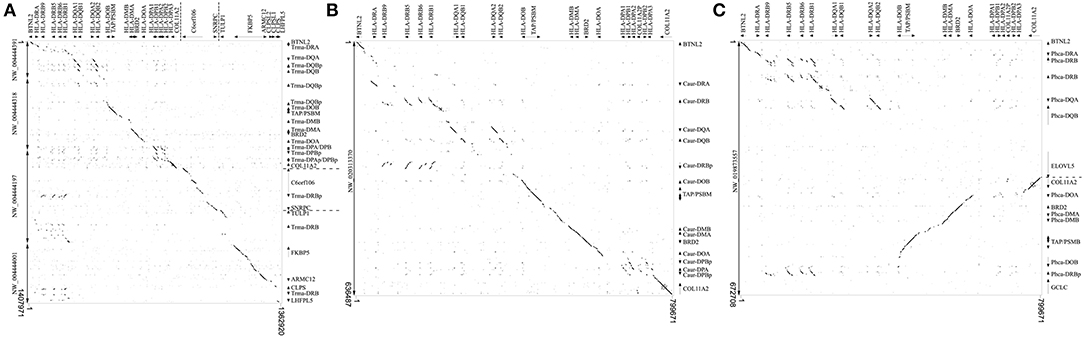
Figure 1. Dot plot analyses of (A) Trichechus manatus, (B) Callorhinus ursinus, and (C) Physeter catodon scaffolds containing MHC class II genes, compared to the same region of the human genome. Human gene annotations are shown on the top of the graphs. For clarity purposes, extended class II region was reduced to include only key regions containing class II sequences; dashed lines on the annotation represent gaps. T. manatus sequences were in different scaffolds and were therefore concatenated for this analysis.
Main Features of the Marine Mammal MHC Class II Region
Due to the fragmentation of the manatee class II region in distinct scaffolds, we turned our attention to other afrotherians to understand their organization. The genomes investigated here were all sequenced and assembled by the Broad Institute. L. africana was the first sequenced afrotherian genome, assembled with Sanger reads; other afrotherian genomes were sequenced by NGS Illumina Hi-seq technology. All genomes were de novo assembled, with coverage ranging from 44x to 150x for the Illumina assembled genomes (Supplementary File S1). C. asiatica and E. edwardii have all class II loci in the same scaffold, evidence that class II sequences lie in the same chromosome. All analyzed afrotherian share the presumptive translocation of DR loci (Figure 2); this translocation was not found in other boreoeutherian or xenarthran genomes analyzed here (data not shown). Other presumptive chromosomal rearrangements were the deletion of DQ in the ancestor of Afrosoricida (i.e., C. asiatica and E. telfairi) and deletion of DP in E. telfairi (Figure 2).
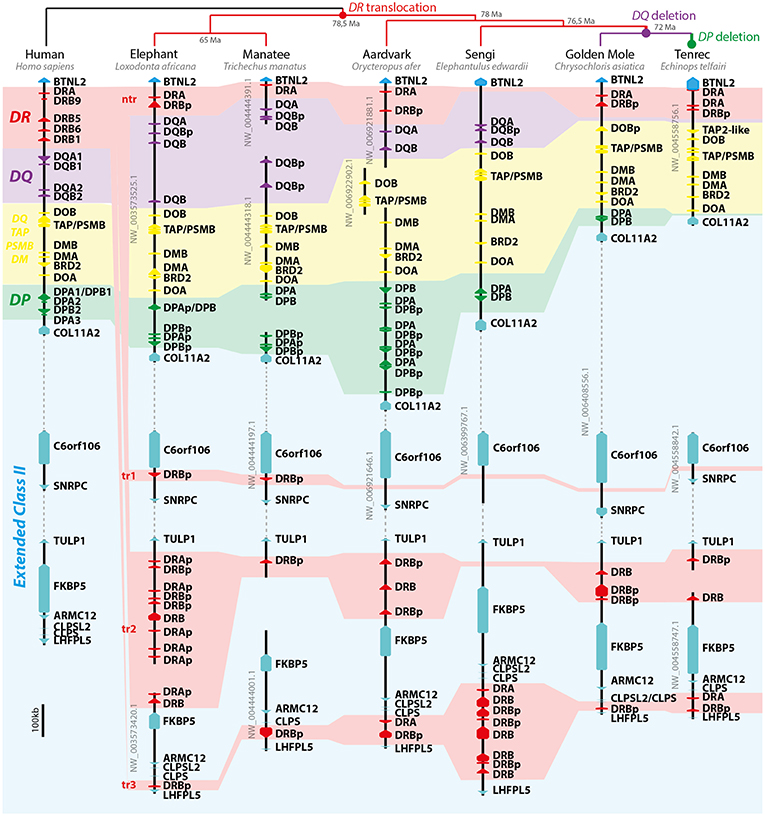
Figure 2. Model of afrotherian class II MHC evolution. On the top of image, the afrotherian phylogeny and divergence time (Ma, million years before present) proposed by Springer et al. (39) and presumptive evolutionary events leading to current afrotherian MHC structure. On the left the human class II MHC region is depicted as an outgroup and model of the mammalian genome organization. Dashed lines represent regions of the scaffolds excluded for clarity purposes, which are not to scale. Arrows represent genes and pseudogenes (shown as “p” in the end of the gene's name). Only informative scaffolds of the MHC structure are displayed. In color, a schematic view of class II loci helps to understand the evolution of class II loci (DR—red; DQ—purple; DP—green; DO/TAP/PSMB/DM —yellow). TAP/PSMB represents TAP1, TAP2, PSMB8, PSMB9.
The pinniped class II region has the same composition found in the dog DLA (Figure 3). Like the Northern fur seal, walrus and Weddell seal also possess DRB loci between DOB and DQB. Pinniped genomes have varied numbers of DR loci but a single pair of presumed DQ genes. Most DP loci seem to be pseudogenes in pinnipeds (Figure 3).
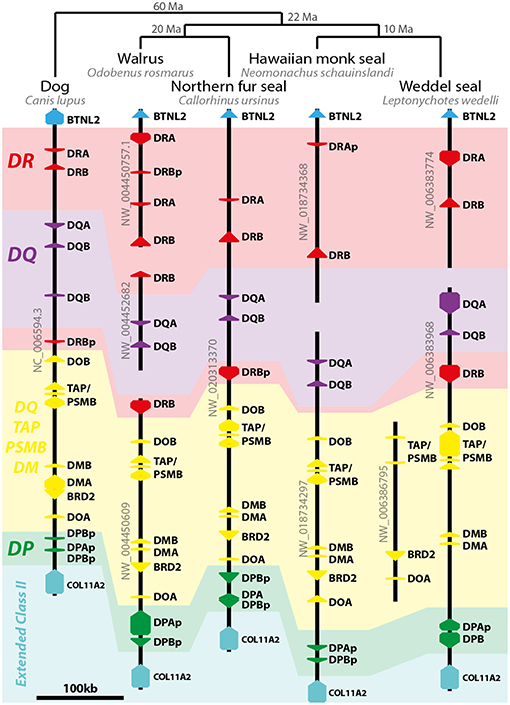
Figure 3. Model of pinniped class II MHC evolution. On the top of image, the pinniped phylogeny and divergence time (Ma, million years before present) proposed by Nyakatura and Bininda-Emonds (40). On the left, dog class II MHC region is depicted as an outgroup and model of the Carnivora genome organization. Arrows represent genes and pseudogenes (shown as “p” in the end of the gene's name). Only informative scaffolds of the MHC structure are displayed. In color, a schematic view of class II loci helps to understand the evolution of class II loci (DR—red; DQ—purple; DP—green; DO/TAP/PSMB/DM—yellow). TAP/PSMB represents TAP1, TAP2, PSMB8, PSMB9.
All cetaceans share with terrestrial Cetartiodactyla the inversion separating class II genes in two subregions: IIa, including DR and DQ loci; and IIb, including non-classic genes (Figure 4). Cetaceans have one DRA gene and up to three DRB loci in class IIa and a presumed DRB pseudogene next to DOB on class IIb region. Despite lying in the same location occupied by DYB and DSB in cattle, those sequences share a higher homology with DRB exons and introns and therefore were annotated as such. Most cetaceans have only one pair of DQ genes, whereas no DQ loci was found in the minke whale genome. Like cattle, cetaceans seem to have lost DP loci altogether, with only remnants of a DPB pseudogene found in the minke whale class IIb region. No DY loci was found in any cetacean (Figure 4).
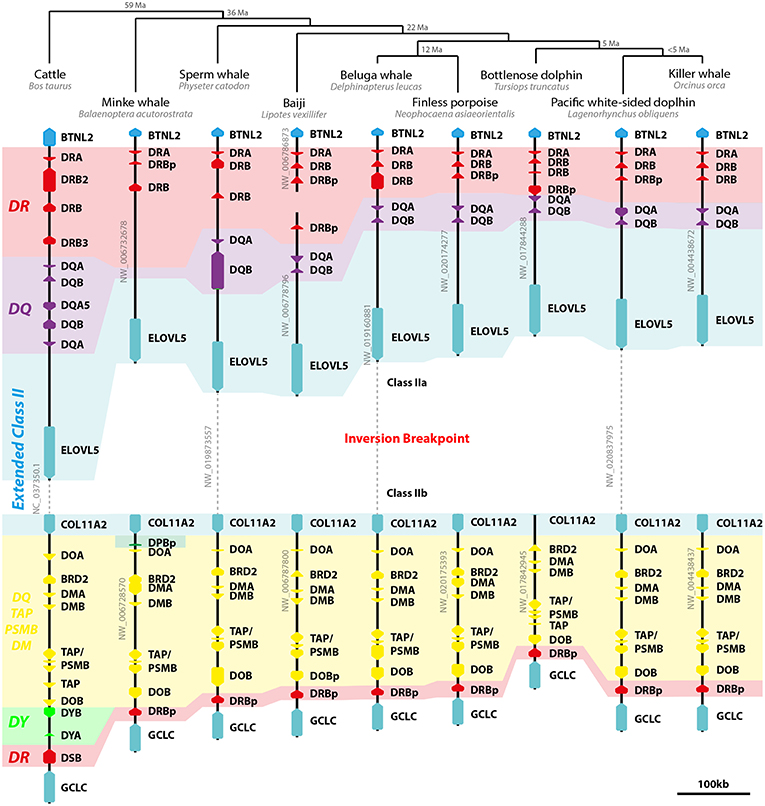
Figure 4. Model of cetacean class II MHC evolution. On the top of image, the Cetartiodactyla phylogeny and divergence time (Ma, million years before present) proposed by Zurano et al. (41). On the left, cattle class II MHC region is depicted as an outgroup and model of the terrestrial Cetartiodactyla genome organization. Dashed lines represent regions of the scaffolds excluded for clarity purposes, which are not to scale. Arrows represent genes and pseudogenes (shown as “p” in the end of the gene's name). Only informative scaffolds of the MHC structure are displayed. In color, a schematic view of class II loci helps to understand the evolution of class II loci (DR—red; DQ—purple; DP—green; DY—light green; DO/TAP/PSMB/DM—yellow). TAP/PSMB represents TAP1, TAP2, PSMB8, PSMB9.
Non-classical Class II and Antigen Processing Genes
Overall, non-classical genes were already annotated in the genomes analyzed here, but some entries needed a refined prediction of exon boundaries. The gene content and organization across marine mammals is highly conserved, as found in other mammals. Notably, D. leucas and E. telfairi DOB had to be separated from the TAP2 gene annotations. L. vexillifer and C. asiatica DOB have a stop codon at exon 5, therefore were annotated as presumed pseudogene despite being the only predicted DOB locus in their genomes. Protein alignments showed conservation of exon sizes, with most differences related to missing exons due to gaps in assembly (Supplementary Files S4–S7).
Classical Class II Genes
DR Loci
Most DR loci had to be reannotated, especially the small exons 5 and 6 from DRB. Protein alignments of DR genes are provided in Supplementary Files S8, S9. In the three orders of marine mammals we were able to find DRA and DRB genes, despite manatee having most of its sequences outside the canonical class II region. The translocation of DR loci in Afrotheria split sequences into four subregions (Figure 2): within the canonical class II region (not translocated, “nt”), between C6orf106 and SNRPC (translocation 1, “tr1”; ~2.3 Mb distant from nt), between TULP1 and FKBP5 (tr2; mean ~3.2 Mb distant from nt), and between CLPS and LHFPL5 (tr3; mean ~3.9Mb distant from nt). The manatee has presumptive functional DRA and DRB genes at the nt and tr2 region, respectively, this DRB gene has a 16 codon gap in exon 3 but was considered a functional gene since no stop codons were found. The DR subregion in manatee have several assembly gaps, which hinders a clearer definition of number of genes. Tr1 was only found in Paenungulata (i.e., L. africana and T. manatus). All afrotherians seem to have lost functional DRB from nt, whereas E. edwardii lack all DR loci in the region (Figure 2). Afrotherian species seem to maintain only one subregion with presumed functional DRB genes, either tr2 (Paenungulata, O. afer, and Afrosoricida) or tr3 (E. edwardii).
Pinnipeds possess DRB and DRA loci; walrus have an in tandem duplication of DR loci in the nt. The only DRA locus from Hawaiian monk seal has a 1-bp deletion in exon 2 leading to several stop codons, and therefore was annotated as a pseudogene. However, this species has a presumptive functional DRB gene. Pinnipeds (except the Hawaiian monk seal) also share with dog a DRB locus between DQB and DOB (termed “nt2” region) that seems to be functional in walrus and Weddell seal (Figure 3).
Cetaceans have one bona fide DRA gene and one to three DRB loci (Figure 4). The cetaceans and cattle share a one codon deletion on the first exon of DRA genes (Supplementary File S8). The cetacean DRB pseudogene in the class IIb region lies in a location similar to DSB in cattle (Figure 4). The position and direction of class IIb DRB pseudogenes are compatible to that of nt2 DRB in the non-inverted class II region of other mammals.
DRA phylogenies formed well-supported clusters separating Carnivora, Cetartiodactyla and Afrotheria loci (Figure 5A). The afrotherian translocated loci from different locations did not form a well-supported cluster on the phylogenies; overall, DR loci grouped by species and not by genomic position on the phylogenetic trees. The only evidence of orthology from different afrotherian species occurred in the Paenungulata DRA nt genes (Figure 5). Carnivora formed 2 well-supported clusters in DRB phylogeny separating nt from nt2 sequences, although horse nt2 loci did not cluster with the Carnivora nt2 loci (Figure 5B). Similarly, cetacean IIb loci formed a well-supported cluster apart from nt2 loci from horse and carnivores (Figure 5B). Cetacean IIa loci formed two clusters separating most DRB pseudogenes from the genes, and therefore the ancestor of cetaceans probably had one DRB gene and one pseudogene.
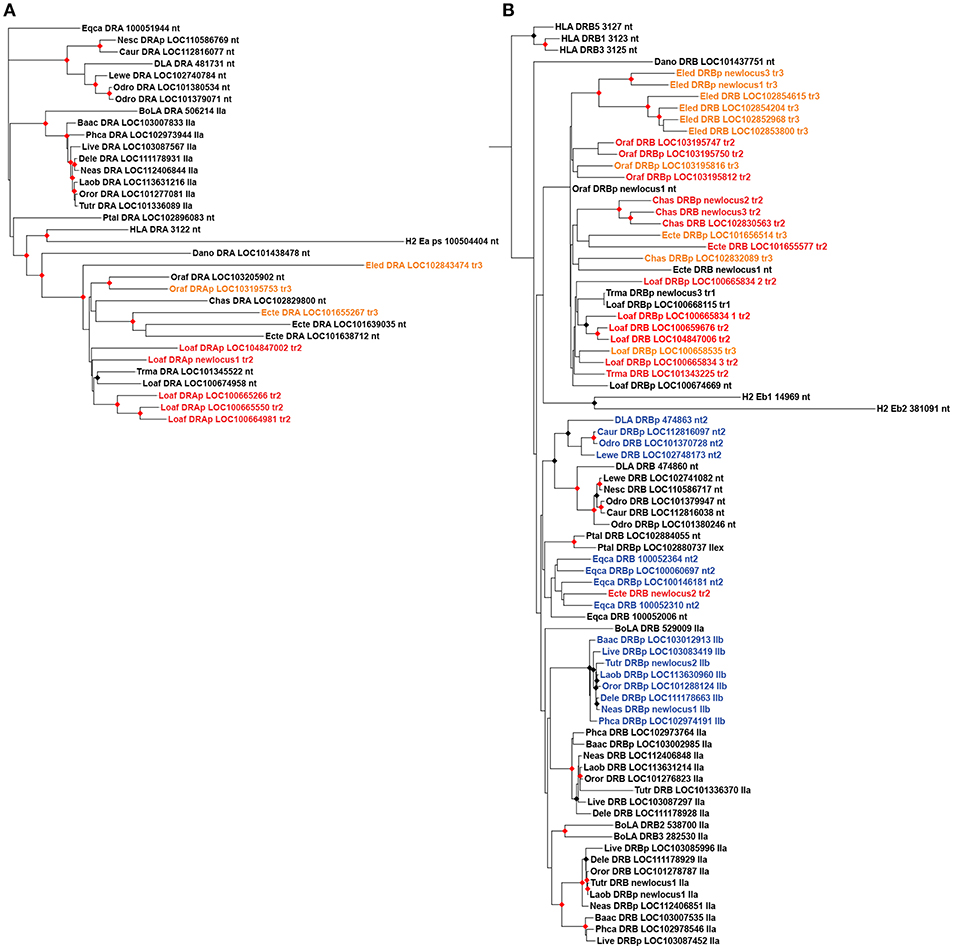
Figure 5. Maximum likelihood phylogenetic trees of DR MHC class II genes and pseudogenes. (A) DRA introns 1, 2, and 3 phylogeny; (B) DRB introns 1, 2, 3, 4, and 5 phylogeny. On the sequence positions: “nt” refers to not translocated; in blue, "nt2" refers to genes between DQ and DO, and IIb to genes located on class IIb region in Cetartiodactyla, in blue; “tr1” refers to translocated between C6orf106 and SNRPC; “tr2” between TULP1 and FKBP5, in red; “tr3” between CLPS and LHFPL5, in orange; “p” refers to pseudogenes. Black and red circles indicates >80 and >95% support values, respectively. L. africana, Loaf; T. manatus, Trma; O. afer, Oraf; El edwardii, Eled; C. asiatica, Chas; E. telfairi, Ecte; D. novemcintus, Dano; B. acutorostrata, Baac; D. leucas; Dele; L. vexillifer, Live; N. asiaeorientalis, Neas; O. orca, Oror; L. obliquidens, Laob; T. truncates, Tutr; P. catodon, Phca; C. ursinus, Caur; N. schauinslandi, Nesc; L. weddelli, Lewe; O. rosmarus, Odro; P. alecto, Ptal; E. caballus, Eqca; B. taurus, BoLA; H. sapiens, HLA; M. musculus, H2; C. l. familiaris, DLA.
DQ Loci
The marine mammals have a similar DQ subregion, with at least a pair of DQA and DQB functional genes annotated in most species analyzed here. The manatee genome has one DQA gene and four DQB loci, although only one seems to be functional. Most afrotherians also have a single DQA gene, while the number of DQB loci varied across taxa. The only species analyzed with multiple presumptive functional DQB genes is L. africana. We could not find any DQ loci in the genome of C. asiatica and E. telfairi. Pinnipeds and cetaceans have a pair of DQA and DQB genes, however, no DQ loci was located in the minke whale genome, possibly due to the abundance of assembly gaps in the DQ subregion. DQ genes maintained overall conservation of exon length, with differences only in exon 1 of DQB (Supplementary Files S10, S11). Most manatee loci clustered with elephant sequences in the phylogenies (Figure 6), but one manatee DQB pseudogene clustered with P. alecto pseudogene, suggesting this pseudogene was present in the ancestor of eutherians (Figure 6B). Carnivora and Cetartiodactyla DQA and DQB genes clustered inside their groups; cetacean DQA is orthologous to BoLA-DQA2 (Figure 6A).
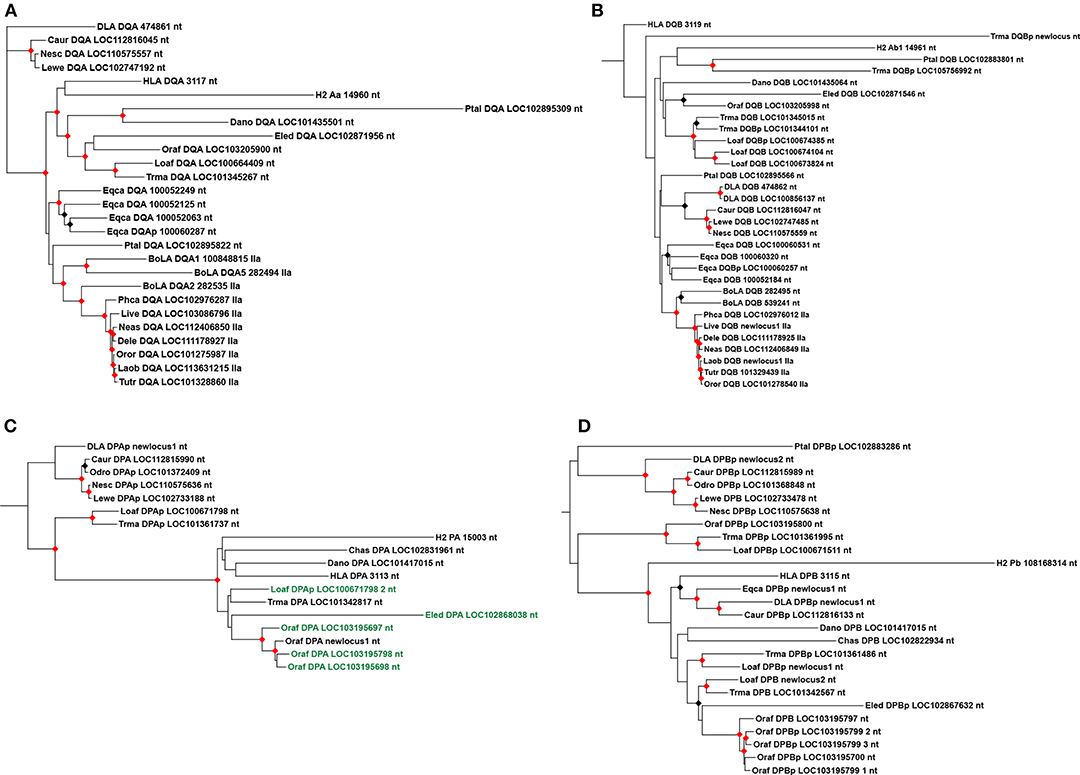
Figure 6. Maximum likelihood phylogenetic trees of DQ and DP MHC class II genes and pseudogenes. (A) DQA introns 1, 2, and 3 phylogeny; (B) DQB introns 1, 2, 3, 4, and 5 phylogeny; (C) DPA introns 1, 2, and 3 phylogeny; (D) DPB introns 1, 2, 3, and 4 phylogeny. In (C), sequences with a 3-codon insertion on exon 3 are in green. “p” refers to pseudogenes. Black and red circles indicates >80 and >95% support values, respectively. L. africana, Loaf; T. manatus, Trma; O. afer, Oraf; El edwardii, Eled; C. asiatica, Chas; E. telfairi, Ecte; D. novemcintus, Dano; B. acutorostrata, Baac; D. leucas; Dele; L. vexillifer, Live; N. asiaeorientalis, Neas; O. orca, Oror; L. obliquidens, Laob; T. truncates, Tutr; P. catodon, Phca; C. ursinus, Caur; N. schauinslandi, Nesc; L. weddelli, Lewe; O. rosmarus, Odro; P. alecto, Ptal; E. caballus, Eqca; B. taurus, BoLA; H. sapiens, HLA; M. musculus, H2; C. l. familiaris, DLA.
DP Loci
We reannotated most DP loci, mainly due to difficulty in assigning exon 1 for DPA and exon 5 for DPB. Most DPA loci were annotated with a small exon 1, because the start codon seemed to have mutated (coding for a valine instead of methionine). Protein alignments are provided in Supplementary Files S12, S13. Among the marine mammals, the only species with a pair of presumptive functional DPA and DPB genes is the manatee (Supplementary File S3, Table 1); the manatee possesses three DPA and four DPB loci, but only two DPA and one DPB are presumptive genes. Inside Afrotheria, O. afer possesses four in tandem duplications of the DP loci, while E. telfairi lost all DP loci (Figure 2). Most pinniped's DP loci are pseudogenes, except one DPB gene in Weddell seal and one DPA in Northern fur seal (Figure 3); Caur-DPA lacks homology in the end of exon 4 and Lewe-DPB is a partial sequence including only exons three and four. Cetaceans lack DP loci altogether, with the exception of a remnant of a DPB pseudogene (homology only to the exon 3) found in minke whale (Figure 4).
Four DPA genes (three from O. afer and one from E. edwardii) and two pseudogenes (one from O. afer and one from L. africana) possess a distinctive three codon insertion in exon 3, which may be evidence of an ancestral form of DPA in the afrotherian lineage (Supplementary Files S3, S12). However, sequences with this insertion did not form a well-supported cluster in the phylogenies (Figure 6C). DPA showed signs of orthology in Paenungulata; DPB pseudogenes showed signs of orthology between Paenungulata and O. afer (Figure 6). The ancestor of eutherians seems to have had two in tandem duplications of DP loci, one with functional genes and the other with pseudogenes; the manatee has loci from both clusters (Figure 6). Most Carnivora loci grouped inside pseudogene clusters; the presence of Caur-DPA and Lewe-DPB inside this cluster of Carnivora pseudogenes suggests both may not be functional (Figure 6).
Discussion
Here we report the organization of the marine mammal class II MHC and the first model for the evolution of this region in afrotherians, sirenians and pinnipeds, including species from the families Otaridae, Phocidae, and Odobenidae. We also expanded the number of class II MHC organization reports in cetaceans, including species from Mysticeti and Odontoceti lineages. We found that the manatee MHC includes the main classical mammalian class II genes while most DR loci were translocated—a feature manatee shares with the other afrotherians analyzed here. Both pinnipeds and cetaceans have presumptive functional DQ and DR genes, probably lost functional DP genes, and have one DRB locus lying next to DOB. These findings fill a gap in the study of marine mammal immunogenetics and eutherian MHC evolution, providing evidence of new chromosomal rearrangements events that led to changes in the organization of the mammalian MHC.
The afrotherian MHC is poorly studied as a whole—to our knowledge, the only reports on afrotherian MHC are two studies on the DQA polymorphisms in elephant and wooly mammoth (42, 43). Based on genomic resources analyzed here, the manatees share with other afrotherians a unique DR translocation separating it from the core class II region. Despite manatee genes being distributed over four main scaffolds, all class II MHC sequences, including translocated DR loci, presumably lie on the same chromosome, based on other afrotherian class II regions and data from chromosome painting in manatee (44). The similarity between the manatee and elephant class II organization suggests that elephant may serve as a model for understanding the manatee MHC function. Antigen presentation in manatee presumably uses DR, DQ, and DP, with evidence of DQ duplications. Future research addressing the expression and polymorphism of class II genes in both species is needed. Afrotherians also have other unique features: a three-codon insertion on exon 3 in some of the DPA loci and deletion of DQ and DP loci during Afrosoricida (tenrec and golden mole) evolution. It is important to notice that tenrec (E. telfairi) seem to have one of the simplest mammalian MHC class II regions. We found three DRA genes and only one DRB gene on the tenrec assembly (Figure 2; Supplementary File S3), which may represent a mammalian “minimal essential” MHC class II, like in chickens—with only two classical class II genes, coding the alpha and beta peptides (45).
Despite several reports on class II gene polymorphisms in pinnipeds, to our knowledge this is the first analysis of MHC structure in this clade. The class II MHC of pinnipeds is similar to the DLA organization (46). The lack of a pair of presumed functional DPA and DPB genes in pinnipeds suggests their MHC may function similarly to cetaceans—using primarily DR and DQ molecules—and may provide opportunities to investigate convergence in class II evolution in both clades. Most pinnipeds also have an inverted DRB locus between DQB and DOB, which is not only present in carnivores such as dogs (46) and cats (47) but also in horses (48). This inversion event is thought to have occurred in the ancestor of Laurasitheria (48) and this DRB may be functional in walrus and Weddell seal.
Despite several studies focused on class II gene polymorphism in cetaceans, their MHC structure was only recently characterized (26). Most cetaceans analyzed here and the previously reported Yangtze finless porpoise MHC (26) have one DRA and two DRB loci in class IIa, a DRB pseudogene in class IIb, a single DQ pair, and lack DP and DY genes. On the other hand, cattle have DYA, DYB, DSB, and duplicated DQ genes; therefore using cattle as a model for cetacean immunogenetics should be cautionary until further characterization of expression and MHC haplotype variation in cetaceans. Notably, the only Mysticeti species analyzed here lacks DQ loci in its assembly, probably due to the large assembly gaps in this region, since there are reports of DQ polymorphisms in baleen whales (16, 19).
The difficulties of assembling the MHC region is widely known, due to extensive variation in gene sequence and haplotype composition of multigene families. However, due to increasing availability of good non-model species genomes, researchers have started using publicly available genomes to analyze the MHC region (49–56). Despite the challenges, to our knowledge, there are no reports that such difficulties resulted in artifactual chromosomal rearrangements of MHC loci, such as the translocations seen in Afrotheria. This mutation event is supported by the fact that two different sequencing technologies (long-reads from Sanger and short-reads from Illumina) and two different de novo assembly algorithms [ARACHNE and ALLSPATH (57, 58)] resulted in the same translocated subregions across the afrotherian genomes. Thus, if the translocation was an assembly artifact, the same misassembly would have to be repeated six times independently with datasets from different species, generated with different sequencing methodologies and different assembly algorithms. It is important to note that no other mammals analyzed here or elsewhere had similar events. The MHC organization of the other analyzed marine mammals were highly consistent with the organization of their eutherian lineages. Similarly, the deletion of DQ in Afrosoricida is supported by the evolutionary relationship of C. asiatica and E. telfairi—a deletion shared by two independent assemblies of animals from the same lineage and by the overall reduction in MHC size in the species scaffolds especially in the DQ/DP subregion. Another way to provide physical evidence for the translocation or deletion would be to use fluorescent in situ hybridization or sequence BAC libraries containing MHC genes, which was beyond the scope of the present study.
The DR phylogenies clustered sequences by species, although we expected that all translocated loci across afrotherians would form a well-supported cluster in the phylogenies, separated from non-translocated sequences. DRB loci in the nt2-IIb region and DPA with a three-codon insertion also did not cluster in the phylogenies. Previous research, using class II MHC genes from laurasitherians, also had similar results (48). Orthologous relationships were particularly observed inside Cetacea and Carnivora in which species diverged < 60 Ma (40, 41). A better resolution of orthology among translocated loci would probably be achieved using less divergent taxa, since the clades analyzed here diverged early in afrotherian evolution. For instance, one of the closest pair of species studied here is the African elephant and the Florida manatee, with estimates of 70~65 million years of divergence (39, 59). The non-translocated DRA from manatee indeed clustered with the non-translocated elephant homolog, but the lack of other sequences (i.e., translocated Trma-DRA and non-translocated Trma-DRB) presently hinders a more comprehensive analysis.
In a simplistic scenario of the ancestral Afrotheria MHC, the translocated loci would diverge, and a phylogenetic analysis would separate loci from both regions (Figure 7A). However, in a realistic scenario, birth-and-death evolution (see below), natural selection, occasional gene conversion-like events and recombination may blur the evolutionary relationship among loci (Figure 7B). Those evolutionary processes may result in no true orthologs for MHC genes between distantly related taxa (60, 61). Similar processes may also explain why nt2 DRB loci and DPA loci with a three-codon insertion did not form a well-supported group in the phylogeny (since it is unlikely that both are cases of homoplasy).
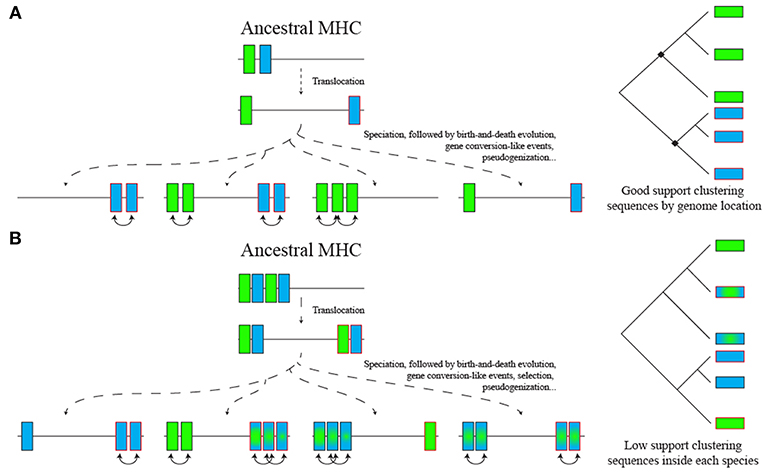
Figure 7. Two scenarios for ancestral MHC class II region evolution and impact on phylogenetic analysis. (A) A simple model with only two paralogs separated by a translocation event. (B) A complex model with in tandem duplication of two loci separated by a translocation event. In the first scenario, translocated genes from current species would cluster together in the phylogeny, while in the second scenario a birth-and-death model of evolution, including gene conversion-like events and differential loss of tandemly duplicated loci, results in no true orthologs across distantly related taxa. Selection acting on both translocated and non-translocated regions can also blur the phylogenetic signal in both scenarios both for exonic and intronic sequences.
The birth-and-death model of evolution for gene families—in which duplication, deletion and pseudogenization of genes lead to expansion and contraction of gene families (61)—affects both MHC class I and II genes but is more pronounced in the former, which usually results in lack of orthology when comparing animals from different families/orders (60). It has been proposed that the class I region evolves faster in eutherians due to its separation from the antigen processing genes (62); in addition, teleost classical class II genes are separated from the rest of the MHC and evolve similarly to eutherian class I genes (62). The variation in the number of DR loci in the afrotherian translocated regions suggests that the separation of DR loci from the class II region may have allowed genes to evolve faster, which could account for the loss of orthology seen in the phylogenies. Even though the translocation separated two DR subregions, the coded proteins still must form a functional heterodimeric class II protein that can interact with the TCR/CD4 complex of T lymphocytes. Thus, alpha and beta DR genes may coevolve and converge irrespective of their position in the genome, which again may impact phylogenies.
The clustering of genes related to antigen processing and presentation in the MHC and their conserved organization in eutherians is thought to be of functional importance (63). In mammals, early evidence of disruption in this organization was found in ruminants, in which an inversion split their class II region into two subregions (64, 65), an event now known to have taken place in the Cetartiodactyla ancestor (26). Since then, other studies revealed additional events disrupting this seemingly conserved organization: an inversion on distal class I region and loss of functional DQ and DP in felines (66); loss of DR in mole rats (67); disruption of the MHC organization and pseudoautosomal localization in monotremes (68); and several rearrangements in class I and II regions in wallaby (69). Those reports, including ours, provide opportunities to investigate how the MHC function evolves in different genomic landscapes and may challenge the functional importance of conserving the MHC organization (70). We also note that no afrotherian or xenarthran mammals had their entire MHC organization characterized, therefore our results show that the separation of class I and class II genes took place in the ancestor of all living eutherians after the split with marsupials [~170 million years ago (59, 71)], as suggested by Belov et al. (72).
The comparative study of the MHC in marine mammals may address how each lineage dealt with unique pathogen pressures in marine environments with the proposed distinct MHC class II genomic organization: the sirenians, with three classical gene families and the presumptive afrotherian translocated DR loci; the pinnipeds, with two classical gene families and inverted DRB loci; and the cetaceans, with two classical gene families and the cetartiodactyl inversion separating class IIa and IIb. Due to the lack of gene expression studies, the presumed annotation of genes and pseudogenes presented here should be interpreted with caution. Therefore, our results mandate future studies focusing upon in depth characterization of the structure, function and expression of the MHC as well as other important immunogenetic systems—such as TLR, Ig, and TCR, already in progress for some lineages and genes (73–78)—in the three marine mammal lineages. Direct sequencing and transcriptomic data will help clarify which sequences are functional, the degree of polymorphisms and any functional specialization of duplicated or translocated loci. Future research may use data provided here to carefully design amplification schemes that target canonical, translocated, inverted or IIb DRB loci.
Taken together, our results indicate a unique class II MHC architecture in each major marine mammal lineage. The evidence presented here also shows a sequential loss of two classical class II genes during Afrosoricida evolution, which may have resulted in the simplification of the class II region in E. telfairi, with only one classical class II protein encoded. Those results point to the separation of MHC class I and II regions in the ancestor of all living eutherians and reiterates the challenges to uncovering evolutionary relationships between MHC genes in distantly-related taxa. The occurrence of rearrangements in the mammalian MHC suggests this highly conserved system may be more malleable than once thought.
Author Contributions
AS, LS, MC, and MS designed the study. AS performed all analysis and prepared figures. AS, BB, and TD reannotated the class II genes and performed dot plot analysis. AS and TB performed the phylogenetic analysis. LS, MC, and MS supervised all analysis. AS, BB, LS, and MC prepared the manuscript. All authors reviewed and approved the manuscript.
Funding
This work was supported by: Coordenação de Aperfeiçoamento de Pessoal de Nível Superior, Brazil (CAPES) and Texas A&M University Collaborative Research Grant Program (CAPES/TAMU 001/2014). MC, BB, and TD were supported by US National Science Foundation award IOS-16568790 to MC. MS was supported by Conselho Nacional de Desenvolvimento Científico e Tecnológico (CNPq). LS was supported by Programa de Apoio à Cooperação Internacional (PACI) from Pró-Reitoria de Pós-Graduação and Fundação de Amparo à Pesquisa da UFPA (UFPA-PROPESP/FADESP).
Conflict of Interest Statement
The authors declare that the research was conducted in the absence of any commercial or financial relationships that could be construed as a potential conflict of interest.
Supplementary Material
The Supplementary Material for this article can be found online at: https://www.frontiersin.org/articles/10.3389/fimmu.2019.00696/full#supplementary-material
References
1. Uhen MD. Evolution of marine mammals: back to the sea after 300 million years. Anat Rec. (2007) 290:514–22. doi: 10.1002/ar.20545
2. Foote AD, Liu Y, Thomas GWC, Vinař T, Alföldi J, Deng J, et al. Convergent evolution of the genomes of marine mammals. Nat Genet. (2015) 47:272–5. doi: 10.1038/ng.3198
3. Kelley NP, Pyenson ND. Evolutionary innovation and ecology in marine tetrapods from the Triassic to the Anthropocene. Science. (2015) 348:a3716. doi: 10.1126/science.aaa3716
4. Chikina M, Robinson JD, Clark NL. Hundreds of genes experienced convergent shifts in selective pressure in marine mammals. Mol Biol Evol. (2016) 33:2182–92. doi: 10.1093/molbev/msw112
5. De Assunção-Franco M, Hoffman JI, Harwood J, Amos W. MHC genotype and near-deterministic mortality in grey seals. Sci Rep. (2012) 2:1–3. doi: 10.1038/srep00659
6. McCallum HI, Kuris A, Harvell CD, Lafferty KD, Smith GW, Porter J. Does terrestrial epidemiology apply to marine systems? Trends Ecol Evol. (2004) 19:585–91. doi: 10.1016/j.tree.2004.08.009
7. Suttle C A. Marine viruses–major players in the global ecosystem. Nat Rev Microbiol. (2007) 5:801–12. doi: 10.1038/nrmicro1750
8. Lafferty KD. Marine infectious disease ecology. Annu Rev Ecol Evol Syst. (2017) 48:473–96. doi: 10.1146/annurev-ecolsys-121415-032147
9. Ujvari B, Belov K. Major histocompatibility complex (MHC) markers in conservation biology. Int J Mol Sci. (2011) 12:5168–86. doi: 10.3390/ijms12085168
10. Trowsdale J, Knight JC. Major histocompatibility complex genomics and human disease. Annu Rev Genomics Hum Genet. (2013) 14:301–23. doi: 10.1146/annurev-genom-091212-153455
11. Pagán HJT, Ferrer T, O'Corry-Crowe G. Positive selection in coding regions and motif duplication in regulatory regions of bottlenose dolphin MHC class II genes. PLoS ONE. (2018) 13:1–27. doi: 10.1371/journal.pone.0203450
12. Zhang X, Lin W, Zhou R, Gui D, Yu X, Wu Y. Low major histocompatibility complex class ii variation in the endangered indo-pacific humpback dolphin (Sousa chinensis): inferences about the role of balancing selection. J Hered. (2016) 107:143–52. doi: 10.1093/jhered/esv138
13. Moreno-Santillán DD, Lacey EA, Gendron D, Ortega J. Genetic variation at exon 2 of the MHC class II DQB locus in blue whale (Balaenoptera musculus) from the Gulf of California. PLoS ONE. (2016) 11:e0141296. doi: 10.1371/journal.pone.0141296
14. Cammen KM, Wilcox LA, Rosel PE, Wells RS, Read AJ. From genome-wide to candidate gene: an investigation of variation at the major histocompatibility complex in common bottlenose dolphins exposed to harmful algal blooms. Immunogenetics. (2015) 67:125–33. doi: 10.1007/s00251-014-0818-x
15. Gillett RM, Murray BW, White BN. Characterization of class i- and class ii-like major histocompatibility complex loci in pedigrees of north atlantic right whales. J Hered. (2014) 105:188–202. doi: 10.1093/jhered/est095
16. Nigenda-Morales S, Flores-Ramírez S, Urbán-RJ, Vázquez-Juárez R. MHC DQB-1 polymorphism in the Gulf of California fin whale (Balaenoptera physalus) population. J Hered. (2008) 99:14–21. doi: 10.1093/jhered/esm087
17. Munguia-Vega A, Esquer-Garrigos Y, Rojas-Bracho L, Vazquez-Juarez R, Castro-Prieto A, Flores-Ramirez S. Genetic drift vs. natural selection in a long-term small isolated population: Major histocompatibility complex class II variation in the Gulf of California endemic porpoise (Phocoena sinus). Mol Ecol. (2007) 16:4051–65. doi: 10.1111/j.1365-294X.2007.03319.x
18. Martínez-Agüero M, Flores-Ramírez S, Ruiz-García M. First report of major histocompatibility complex class II loci from the Amazon pink river dolphin (genus Inia). Genet Mol Res. (2006) 5:421–31.
19. Baker CS, Vant MD, Dalebout ML, Lento GM, O'Brien SJ, Yuhki N. Diversity and duplication of DQB and DRB-like genes of the MHC in baleen whales (suborder: Mysticeti). Immunogenetics. (2006) 58:283–96. doi: 10.1007/s00251-006-0080-y
20. Lau Q, Chow N, Gray R, Gongora J, Higgins DP. Diversity of MHC DQB and DRB genes in the endangered Australian sea lion (Neophoca cinerea). J Hered. (2015) 106:395–402. doi: 10.1093/jhered/esv022
21. Gao XG, Han JB, Lu ZC, Zhang PJ, He CB. Sequence variation and gene duplication at the MHC DRB loci of the spotted seal Phoca largha. Genet Mol Res. (2015) 14:2055–62. doi: 10.4238/2015.March.20.15
22. Osborne AJ, Zavodna M, Chilvers BL, Robertson BC, Negro SS, Kennedy MA, et al. Extensive variation at MHC DRB in the New Zealand sea lion (Phocarctos hookeri) provides evidence for balancing selection. Heredity. (2013) 111:44–56. doi: 10.1038/hdy.2013.18
23. Cammen K, Hoffman JI, Knapp LA, Harwood J, Amos W. Geographic variation of the major histocompatibility complex in Eastern Atlantic grey seals (Halichoerus grypus). Mol Ecol. (2011) 20:740–52. doi: 10.1111/j.1365-294X.2010.04975.x
24. Weber DS, Stewart BS, Schienman J, Lehman N. Major histocompatibility complex variation at three class II loci in the northern elephant seal. Mol Ecol. (2004) 13:711–8. doi: 10.1046/j.1365-294X.2004.02095.x
25. Decker DJ, Stewart BS, Lehman N. Major histocompatibility complex class II DOA sequences from three Antarctic seal species verify stabilizing selection on the DO locus. Tissue Antigens. (2002) 60:534–38. doi: 10.1034/j.1399-0039.2002.600610.x
26. Ruan R, Ruan J, Wan X-L, Zheng Y, Chen M-M, Zheng J-S, et al. Organization and characteristics of the major histocompatibility complex class II region in the Yangtze finless porpoise (Neophocaena asiaeorientalis asiaeorientalis). Sci Rep. (2016) 6:22471. doi: 10.1038/srep22471
27. Kearse M, Moir R, Wilson A, Stones-Havas S, Cheung M, Sturrock S, et al. Geneious basic: an integrated and extendable desktop software platform for the organization and analysis of sequence data. Bioinformatics. (2012) 28:1647–9. doi: 10.1093/bioinformatics/bts199
28. Katoh K, Misawa K, Kuma K, Miyata T. MAFFT: a novel method for rapid multiple sequence alignment based on fast Fourier transform. Nucl Acids Res. (2002) 30:3059–66. doi: 10.1093/nar/gkf436
29. Ballingall KT, Bontrop RE, Ellis SA, Grimholt U, Hammond JA, Ho C, et al. Comparative MHC nomenclature : report from the ISAG / IUIS-VIC committee 2018 (2018)
30. Maccari G, Robinson J, Bontrop RE, Otting N, Groot NG De, Ho C, et al. IPD-MHC : nomenclature requirements for the non-human major histocompatibility complex in the next-generation sequencing era. Immunogenetics. (2018) 70:619–23. doi: 10.1007/s00251-018-1072-4
31. Smit A, Hubley R, Green P. RepeatMasker Open-4.0. (2013) Available online at: http://www.repeatmasker.org (accessed March 22, 2019).
32. Schwartz S, Zhang Z, Frazer KA, Smit A, Riemer C, Bouck J, et al. PipMaker–a web server for aligning two genomic DNA sequences. Genome Res. (2000) 10:577–86. doi: 10.1101/GR.10.4.577
33. Katoh K, Rozewicki J, Yamada KD. MAFFT online service : multiple sequence alignment, interactive sequence choice and visualization. Brief Bioinform. (2017) bbx108. doi: 10.1093/bib/bbx108
34. Talavera G, Castresana J. Improvement of phylogenies after removing divergent and ambiguously aligned blocks from protein sequence alignments. Syst Biol. (2007) 56:564–77. doi: 10.1080/10635150701472164
35. Lanfear R, Frandsen PB, Wright AM, Senfeld T, Calcott B. PartitionFinder 2: new methods for selecting partitioned models of evolution for molecular and morphological phylogenetic analyses. Mol Biol Evol. (2016) 34:msw260. doi: 10.1093/molbev/msw260
36. Miller MA, Pfeiffer W, Schwartz T. Creating the CIPRES Science Gateway for inference of large phylogenetic trees. In: 2010 Gateway Computing Environments Workshop (GCE) IEEE (2010). p. 1–8. doi: 10.1109/GCE.2010.5676129
37. Stamatakis A. RAxML-VI-HPC: maximum likelihood-based phylogenetic analyses with thousands of taxa and mixed models. Bioinformatics. (2006) 22:2688–90. doi: 10.1093/bioinformatics/btl446
38. Letunic I, Bork P. Interactive tree of life (iTOL) v3: an online tool for the display and annotation of phylogenetic and other trees. Nucl Acids Res. (2016) 1:1–4. doi: 10.1093/nar/gkw290
39. Springer MS, Signore AV, Paijmans JLA, Vélez-Juarbe J, Domning DP, Bauer CE, et al. Interordinal gene capture, the phylogenetic position of Steller's sea cow based on molecular and morphological data, and the macroevolutionary history of Sirenia. Mol Phylogenet Evol. (2015) 91:178–93. doi: 10.1016/j.ympev.2015.05.022
40. Nyakatura K, Bininda-Emonds ORP. Updating the evolutionary history of Carnivora (Mammalia): A new species-level supertree complete with divergence time estimates. BMC Biol. (2012) 10:1–31. doi: 10.1186/1741-7007-10-12
41. Zurano JP, Magalhães FM, Asato AE, Silva G, Bidau CJ, Mesquita DO, Costa GC. Cetartiodactyla: updating a time-calibrated molecular phylogeny. Mol Phylogenet Evol. (2019) 133:256–62. doi: 10.1016/J.YMPEV.2018.12.015
42. Pečnerová P, Díez-Del-Molino D, Vartanyan S, Dalén L. Changes in variation at the MHC class II DQA locus during the final demise of the woolly mammoth. Sci Rep. (2016) 6:1–11. doi: 10.1038/srep25274
43. Archie EA, Henry T, Maldonado JE, Moss CJ, Poole JH, Pearson VR, et al. Major histocompatibility complex variation and evolution at a single, expressed DQA locus in two genera of elephants. Immunogenetics. (2010) 62:85–100. doi: 10.1007/s00251-009-0413-8
44. Kellogg ME, Burkett S, Dennis TR, Stone G, Gray BA, McGuire PM, et al. Chromosome painting in the manatee supports Afrotheria and Paenungulata. BMC Evol Biol. (2007) 7:1–7. doi: 10.1186/1471-2148-7-6
45. Kaufman J, Milne S, Göbel TWF, Walker BA, Jacob JP, Auffray C, et al. The chicken B locus is a minimal essential major histocompatibility complex. Nature. (1999) 401:923–25. doi: 10.1038/44856
46. Debenham SL, Hart EA, Ashurst JL, Howe KL, Quail MA, Ollier WER, et al. Genomic sequence of the class II region of the canine MHC: comparison with the MHC of other mammalian species. Genomics. (2005) 85:48–59. doi: 10.1016/j.ygeno.2004.09.009
47. Yuhki N, Beck T, Stephens RM, Nishigaki Y, Newmann K, O'Brien SJ. Comparative genome organization of human, murine, and feline MHC Class II region. Genome Res. (2003) 13:1169–79. doi: 10.1101/gr.976103
48. Viłuma A, Mikko S, Hahn D, Skow L, Andersson G, Bergström TF. Genomic structure of the horse major histocompatibility complex class II region resolved using PacBio long-read sequencing technology. Sci Rep. (2017) 7:1–12. doi: 10.1038/srep45518
49. Ng JHJ, Tachedjian M, Wang LF, Baker ML. Insights into the ancestral organisation of the mammalian MHC class II region from the genome of the pteropid bat, Pteropus alecto. BMC Genomics. (2017) 18:19–21. doi: 10.1186/s12864-017-3760-0
50. Ng JHJ, Tachedjian M, Deakin J, Wynne JW, Cui J, Haring V, et al. Evolution and comparative analysis of the bat MHC-I region. Sci Rep. (2016) 6:1–18. doi: 10.1038/srep21256
51. Braasch I, Gehrke AR, Smith JJ, Kawasaki K, Manousaki T, Pasquier J, et al. The spotted gar genome illuminates vertebrate evolution and facilitates human-teleost comparisons. Nat Genet. (2016) 48:427–37. doi: 10.1038/ng.3526
52. Venkatesh B, Lee AP, Ravi V, Maurya AK, Lian MM, Swann JB, et al. Elephant shark genome provides unique insights into gnathostome evolution. Nature. (2014) 505:174–9. doi: 10.1038/nature12826
53. Saha NR, Ota T, Litman GW, Hansen J, Parra Z, Hsu E, et al. Genome complexity in the coelacanth is reflected in its adaptive immune system. J Exp Zool Part B Mol Dev Evol. (2014) 322:438–63. doi: 10.1002/jez.b.22558
54. Dijkstra JM, Grimholt U, Leong J, Koop BF, Hashimoto K. Comprehensive analysis of MHC class II genes in teleost fish genomes reveals dispensability of the peptide-loading DM system in a large part of vertebrates. BMC Evol Biol. (2013) 13:260. doi: 10.1186/1471-2148-13-260
55. Star B, Nederbragt AJ, Jentoft S, Grimholt U, Malmstrøm M, Gregers TF, et al. The genome sequence of Atlantic cod reveals a unique immune system. Nature. (2011) 477:207–10. doi: 10.1038/nature10342
56. Sambrook JG, Figueroa F, Beck S. A genome-wide survey of major histocompatibility complex (MHC) genes and their paralogues in zebrafish. BMC Genomics. (2005) 6:1–10. doi: 10.1186/1471-2164-6-152
57. Batzoglou S, Jaffe DB, Stanley K, Butler J, Gnerre S, Mauceli E, et al. ARACHNE: a whole-genome shotgun assembler. Genome Res. (2002) 12:177–89. doi: 10.1101/gr.208902
58. Gnerre S, MacCallum I, Przybylski D, Ribeiro FJ, Burton JN, Walker BJ, et al. High-quality draft assemblies of mammalian genomes from massively parallel sequence data. Proc Natl Acad Sci USA. (2011) 108:1513–8. doi: 10.1073/pnas.1017351108
59. Foley NM, Springer MS, Teeling EC. Mammal madness: is the mammal tree of life not yet resolved? Philos Trans R Soc B Biol Sci. (2016) 371. doi: 10.1098/rstb.2015.0140
60. Nei M, Rooney AP. Concerted and birth-and-death evolution of multigene families. Annu Rev Genet. (2005) 39:121–52. doi: 10.1146/annurev.genet.39.073003.112240
61. Eirín-López JM, Rebordinos L, Rooney AP, Rozas J. The birth-and-death evolution of multigene families revisited. Genome Dyn. (2012) 7:170–96. doi: 10.1159/000337119
62. Flajnik MF, Kasahara M, Street WB. Comparative genomics of the MHC : glimpses into the evolution review university of maryland at Baltimore. Immunity. (2001) 15:351–62. doi: 10.1016/S1074-7613(01)00198-4
63. Trowsdale J. The gentle art of gene arrangement: the meaning of gene clusters. Genome Biol. (2002) 3:comment2002.1. doi: 10.1186/gb-2002-3-3-comment2002
64. Andersson L, Lundén A, Sigurdardottir S, Davies CJ, Rask L. Linkage relationships in the bovine MHC region. High recombination frequency between class II subregions. Immunogenetics. (1988) 27:273–80.
65. van Eijk MJ, Russ I, Lewin HA. Order of bovine DRB3, DYA, and PRL determined by sperm typing. Mamm Genome. (1993) 4:113–8.
66. Beck TW, Menninger J, Murphy WJ, Nash WG, O'Brien SJ, Yuhki N. The feline major histocompatibility complex is rearranged by an inversion with a breakpoint in the distal class I region. Immunogenetics. (2005) 56:702–9. doi: 10.1007/s00251-004-0742-6
67. Nizetic D, Figueroa F, Dembic Z, Nevo E, Klein J. Major histocompatibility complex gene organization in the mole rat Spalax ehrenbergi: Evidence for transfer of function between class II genes. Proc Natl Acad Sci USA. (1987) 84:5828–32. doi: 10.1073/pnas.84.16.5828
68. Dohm JC, Tsend-Ayush E, Reinhardt R, Grützner F, Himmelbauer H. Disruption and pseudoautosomal localization of the major histocompatibility complex in monotremes. Genome Biol. (2007) 8:1–16. doi: 10.1186/gb-2007-8-8-r175
69. Siddle HV, Deakin JE, Coggil P, Whilming L, Harrow J, Kaufman J, et al. The tammar wallaby major histocompatibility complex shows evidence of past genomic instability. BMC Genomics. (2011) 12:421. doi: 10.1186/1471-2164-12-421
70. Miller HC, O'Meally D, Ezaz T, Amemiya C, Marshall-Graves JA, Edwards S. Major histocompatibility complex genes map to two chromosomes in an evolutionarily ancient reptile, the tuatara sphenodon punctatus. G3 (Bethesda). (2015) 5:1439–51. doi: 10.1534/g3.115.017467
71. Tarver JE, Dos Reis M, Mirarab S, Moran RJ, Parker S, O'Reilly JE, et al. The interrelationships of placental mammals and the limits of phylogenetic inference. Genome Biol Evol. (2016) 8:330–44. doi: 10.1093/gbe/evv261
72. Belov K, Deakin JE, Papenfuss AT, Baker ML, Melman SD, Siddle HV, et al. Reconstructing an ancestral mammalian immune supercomplex from a marsupial major histocompatibility complex. PLoS Biol. (2006) 4:0317–28. doi: 10.1371/journal.pbio.0040046
73. Villanueva-Noriega MJ, Baker CS, Medrano-González L. Evolution of the MHC-DQB exon 2 in marine and terrestrial mammals. Immunogenetics. (2013) 65:47–61. doi: 10.1007/s00251-012-0647-8
74. Breaux B, Deiss TC, Chen PL, Cruz-Schneider MP, Sena L, Hunter ME, et al. The Florida manatee (Trichechus manatus latirostris) immunoglobulin heavy chain suggests the importance of clan III variable segments in repertoire diversity. Dev Comp Immunol. (2017) 72:57–68. doi: 10.1016/J.DCI.2017.01.022
75. Breaux B, Hunter ME, Cruz-Schneider MP, Sena L, Bonde RK, Criscitiello MF. The Florida manatee (Trichechus manatus latirostris) T cell receptor loci exhibit V subgroup synteny and chain-specific evolution. Dev Comp Immunol. (2018) 85:71–85. doi: 10.1016/J.DCI.2018.04.007
76. Li L, Rong X, Li G, Wang Y, Chen B, Ren W, Yang G, Xu S. Genomic organization and adaptive evolution of IGHC genes in marine mammals. Mol Immunol. (2018) 99:75–81. doi: 10.1016/j.molimm.2018.04.011
77. Woodman S, Gibson AJ, García AR, Contreras GS, Rossen JW, Werling D, Offord V. Structural characterisation of Toll-like receptor 1 (TLR1) and Toll-like receptor 6 (TLR6) in elephant and harbor seals. Vet Immunol Immunopathol. (2016) 169:10–14. doi: 10.1016/J.VETIMM.2015.11.006
Keywords: molecular evolution, genomics, marine mammals, manatee, MHC, immunogenetics, pinnipeds, cetaceans
Citation: Sá ALA, Breaux B, Burlamaqui TCT, Deiss TC, Sena L, Criscitiello MF and Schneider MPC (2019) The Marine Mammal Class II Major Histocompatibility Complex Organization. Front. Immunol. 10:696. doi: 10.3389/fimmu.2019.00696
Received: 30 November 2018; Accepted: 13 March 2019;
Published: 04 April 2019.
Edited by:
Brian Dixon, University of Waterloo, CanadaReviewed by:
Unni Grimholt, Norwegian Veterinary Institute, NorwayPierre Boudinot, Institut National de la Recherche Agronomique (INRA), France
Ben F. Koop, University of Victoria, Canada
Copyright © 2019 Sá, Breaux, Burlamaqui, Deiss, Sena, Criscitiello and Schneider. This is an open-access article distributed under the terms of the Creative Commons Attribution License (CC BY). The use, distribution or reproduction in other forums is permitted, provided the original author(s) and the copyright owner(s) are credited and that the original publication in this journal is cited, in accordance with accepted academic practice. No use, distribution or reproduction is permitted which does not comply with these terms.
*Correspondence: André Luiz Alves de Sá, YW5kcmUubHVpei5zYUBob3RtYWlsLmNvbQ==
Michael Frederick Criscitiello, bWNyaXNjaXRpZWxsb0Bjdm0udGFtdS5lZHU=