- 1PARCC (Paris-Cardiovascular Research Center), INSERM U970, Paris, France
- 2UFR Science du Vivant, Université Paris Diderot, Sorbonne Paris Cité, Paris, France
- 3Faculté de Médecine, Université Paris Descartes, Sorbonne Paris Cité, Paris, France
Considering the high importance of immune surveillance and immune escape in the evolution of cancer, the development of immunotherapeutic strategies has become a major field of research in recent decades. The considerable therapeutic breakthrough observed when targeting inhibitory immune checkpoint molecules has highlighted the need to find approaches enabling the induction and proper activation of an immune response against cancer. In this context, therapeutic vaccination, which can induce a specific immune response against tumor antigens, is an important approach to consider. However, this strategy has its advantages and limits. Considering its low clinical efficacy, approaches combining therapeutic cancer vaccine strategies with other immunotherapies or targeted therapies have been emphasized. This review will list different cancer vaccines, with an emphasis on their targets. We highlight the results and limits of vaccine strategies and then describe strategies that combine therapeutic vaccines and antiangiogenic therapies or immune checkpoint blockade. Antiangiogenic therapies and immune checkpoint blockade are of proven clinical efficacy for some indications, but are limited by toxicity and the development of resistance. Their combination with therapeutic vaccines could be a way to improve therapeutic outcome by specifically stimulating the immune system and considering a global approach to tumor microenvironment remodeling.
Introduction
Therapeutic cancer vaccines are based on specific stimulation of the immune system using tumor antigens to elicit an antitumor response. Nevertheless, therapeutic cancer vaccines are still considered as a strategy that fails to demonstrate clinical benefits. Indeed, when compared to other newly developed immunotherapies such as immune checkpoint blockade (ICB) or CAR T-cell therapies, therapeutic vaccines still show very few outcomes in the establishment of clinical responses in advanced cancer patients.
Numerous improvements have been made in recent decades in therapeutic vaccination protocols that enhance the immune response elicited by the vaccination. FDA approval of the first therapeutic vaccine in 2010, the DC-based vaccine sipuleucel-T (Provenge®) in the treatment of prostate cancer (1), brings to the force possible success for therapeutic vaccination strategies.
In this mini review, we discuss the results and limitations of vaccine approaches tested in clinical trials targeting different tumor antigens. After describing the rationale for combining therapeutic vaccines with either antiangiogenic therapies or ICB, we present examples of combinations in preclinical mouse models and in human clinical trials.
Therapeutic Cancer Vaccines
Targeted Antigens
In the context of antitumor vaccine improvement, significant efforts have been focused on the choice of tumor antigen to target. Although numerous cancer vaccine strategies have been studied, targeted antigens remain at the heart of the discussions. They are classified into two main categories.
Tumor-associated antigens (TAAs) are expressed by tumor cells and also by normal cells such as overexpressed antigens (Her2/neu, survivin, MUC-1 …), cancer testis antigens (MAGE-3, NY-ESO-1 …), or differentiation antigens (Mart1, PSA, PAP …). Although TAAs are expressed at a certain level by normal cells, their immunogenicity induces specific T-cell responses (2–4). However, a certain degree of self-tolerance can be applied to TAAs.
In the case of tumor-specific antigens (TSAs), such as oncogenic viral proteins in virally induced cancers or neoantigens generated by non-silent somatic mutations of normal proteins, such central thymic tolerance is bypassed, being regarded as foreign antigens by the immune system. Neoantigen-based vaccine strategies have shown specific anti-tumor immunity in numerous preclinical models and have been tested in early (Phase I) human clinical trials with very promising results (5–10).
Therapeutic Vaccine Strategies
Different therapeutic vaccine strategies have been developed including whole tumor cell, tumor-cell lysates or gene-modified tumor cells, protein- or peptide-based vaccines, RNA and DNA vaccines, viral vector engineered to express tumor antigen and DC-based vaccines loaded with DNA, RNA or peptides. These strategies are further detailed in specialized reviews (10–15).
Many preclinical and clinical trials using these different strategies have been performed and we will focus here on those that have reached phase II/III clinical trials (16, 17). Some examples are provided below and in Table 1 (18–29).
Cancer vaccine strategies based on autologous dendritic cells (DCs) pulsed with tumor antigens have been widely studied (30, 31). For example, a phase III clinical trial testing peptide-pulsed DCs as a first-line treatment in advanced melanoma patients has been performed, but DC vaccination was ineffective compared to chemotherapy (18). A randomized phase II/III clinical trial is currently ongoing in glioblastoma patients to test a DC-based vaccine (NCT03548571). Many parameters such as DC-based vaccine administration, maintenance of DC viability and maturation as well as standardization of ex vivo generation can be limiting (32–34).
Improvement of vaccine platforms has also led to the use of viral vectors with modified viruses engineered to express both targeted antigens and immunomodulatory molecules. In this context, modified vaccinia virus of the Ankara strain (MVA) has been studied. A vaccine containing MVA expressing tumor antigen MUC-1 and immunostimulatory cytokine IL-2 (TG4010 vaccine) was tested in a phase II clinical trial in patients with metastatic renal cell carcinoma (mRCC) (19). Induction of an immunological response against MUC-1 was observed and safety established. Nevertheless, the trial showed no clinical benefit following vaccination. In the TIME clinical trial (phase II), TG4010 was tested in NSCLC patients with or without chemotherapy. Patients who showed a MUC-1-specific response (n = 16) had an improved clinical outcome with a median overall survival (OS) of 32.1 months vs. 12.7 months in non-responders (n = 6) (20).
Other vaccines have also emerged using this modified virus strategy, such as TroVax, an MVA expressing fetal oncogene 5T4 (MVA-5T4) studied in a phase III clinical trial in the treatment of renal cancer, though no clinical benefit was found (21). PROSTVAC (or PSA-TRICOM), a poxviral-based prostate-specific antigen (PSA) vaccine has been assessed in a phase II clinical trial in the treatment of metastatic castration-resistant prostate cancer (mCRPC) and was associated with a 44% reduction in the death rate and an 8.5 months improvement in median OS (22).
Thus, therapeutic vaccine strategies take many forms and importance is now also given to vaccine administration routes to enhance efficacy (35) as well as work done on vehicle delivery and adjuvants (12, 15, 36–38).
Therapeutic Vaccines in the Era of Combination Strategies
However, even though cancer vaccines have been greatly improved, overall they still fail to provide any clinical benefit as monotherapy in patients with advanced cancers. During cancer progression, tumors develop several mechanisms of immune escape such as tumor angiogenesis, recruitment of immunosuppressive cells, and over-expression of inhibitory molecules, all leading to an ineffective antitumor immune response (39, 40).
Due to their lack of migration and/or progressive exhaustion, tumor-specific T cells generated by vaccination do not act effectively against the tumor. Combination with strategies that counteract such immune escape mechanisms is therefore essential.
Therapeutic vaccine approaches have a major place in the arsenal of weapons developed to fight cancer and in many published studies have been combined with radio- or chemotherapy, with promising results, as reviewed elsewhere (41, 42).
In this mini review, we will concentrate on combinations of therapeutic vaccines and antiangiogenic treatments (AATs) or ICB, highlighting their synergistic potential and providing an update of preclinical and clinical results.
Therapeutic Cancer Vaccines and Antiangiogenic Treatments Combinations
Rationale for Vaccine Combination With AATs
During carcinogenesis, tumors will increase the expression of pro-angiogenic molecules such as vascular endothelial growth factor (VEGF), which is involved in tumor angiogenesis. This process fosters access to nutrient and oxygen supplies for the tumor and allows proliferation and metastatic dissemination even though vasculature development is abnormal (43). On the other hand, the aberrant tumor vasculature actively suppresses anti-tumor responses by providing a physical barrier to T cell infiltration and limits therapeutic drug efficacy due to poor delivery to the tumor (40). Tumor angiogenesis also contributes to immune escape with its immunosuppressive roles (44–47). For example, VEGF is involved in inhibition of DC maturation, development of tumor-associated macrophages, increase in regulatory T cells (Tregs), accumulation of myeloid-derived suppressor cells (MDSCs), and expression of inhibitory checkpoints such as PD-1 on CD8+ T cells (48).
Within this framework, numerous antiangiogenic molecules have been developed to repress tumor angiogenesis. AATs target many components of the tumor microenvironment (endothelial cells, tumor cells, DC, MDSC, Tregs….) resulting in a shift of cytokine and chemokine production favoring antitumor activities. These therapies lead to transient vascular normalization while dampening immunosuppression (40, 47, 49, 50). In this review, we will focus on drugs targeting the VEGF/VEGF receptor (VEGFR) pathway, such as bevacizumab (an anti-VEGFA antibody), tyrosine kinase inhibitors such as sunitinib, sorafenib or axitinib, which target VEFGR but also other pathways, and anti-VEGFR antibodies. These antiangiogenic drugs are currently the most used in the clinical setting. However, the benefits provided by these treatments are still limited and acquired resistance can appear (51, 52).
Considering the extensive tumor microenvironment remodeling induced by AATs, a combination of such strategies with therapeutic vaccines could help to enhance the immune response against tumors. By improving in quantity and quality the infiltration of T lymphocytes activated by the vaccine and by decreasing immunosuppression, AATs might act in synergy with therapeutic cancer vaccines.
Preclinical Studies Combining AATs And Vaccines
Several preclinical studies have sought to define the best timing of administration of therapeutic vaccines and AATs for an optimal synergy of action. These studies have yielded conflicting results.
In a first study, sunitinib was combined with a DC-based vaccine expressing IL-12 and pulsed with OVA-peptide (DC-IL12-OVA) in a B16-OVA tumor model. This combination improved therapeutic efficacy, increased type-1 antitumor T-cell recruitment in the tumor microenvironment and decreased immunosuppressive cells (MDSCs, Tregs) (53). Similar results in terms of efficacy were obtained using the tyrosine kinase inhibitor axitinib instead of sunitinib (54).
Bose et al. showed that the best therapeutic efficacy was achieved when sunitinib was administered alongside vaccine priming or boosting, whereas starting sunitinib treatment after vaccination did not lead to optimal efficacy (53).
Farsaci et al. studied the combination of sunitinib with a modified virus-based vaccine using rMVA containing transgenes for co-stimulatory molecules (B7-1, ICAM-1, and LFA-3) as well as for the carcinoembryogenic antigen (CEA) administered to CEA-transgenic mice bearing MC38-CEA tumors (55). Sunitinib was administered for 4 weeks followed by 2 weeks without treatment (comparable to schedules in cancer patients). In this context, vaccine was administered before, alongside or after the start of sunitinib treatment. Only situations where sunitinib preceded vaccination were associated with increased therapeutic efficacy when compared to sunitinib alone. The authors concluded that synergy between sunitinib and therapeutic vaccination happened after sunitinib preconditioned the immune system.
Finally, a study combining sunitinib with a protein-based vaccine using recombinant α-lactalbumin in a 4T1 mammary tumor-bearing mouse model highlighted the fact that sunitinib inhibited the priming phase of the active immunization protocol by reducing the number of CD11b+ CD11c+ antigen-presenting cells in draining lymph nodes and spleen when sunitinib is administered alongside vaccination (56).
Besides the importance of the timing of administration, the dose of antiangiogenic treatment is a key factor for vessel normalization in the tumor (47, 49). In a preclinical model of breast cancer, only low doses of anti-VEGFR2 antibody (DC101) enabled vascular normalization, and a combination of low-dose DC101 with irradiated tumor cell vaccine enhanced tumor control compared to vaccine alone (57).
Although there seems to be no consensus regarding treatment scheduling, some patterns do emerge. The nature of the vaccine strategy should be taken into account, as should the antiangiogenic drug properties, considering the large spectrum of immune responses considered.
Clinical Trials Combining AATs And Vaccines
Based on positive results in preclinical studies, the combination of AATs and therapeutic vaccines in human subjects is currently being assessed. In a phase II clinical study involving newly diagnosed intermediate and low-risk mRCC patients, sunitinib was combined with a personalized DC-based vaccine AGS-003 consisting of monocyte-derived DCs transfected with total autologous tumor RNAs and CD40L RNA. Vaccine administration was started at the beginning of the second cycle of sunitinib. Combination treatment induced immunological responses and prolonged survival (58). These encouraging results led to a phase III clinical trial, but AGS-003 failed to improve OS (NCT01582672).
The same lack of clinical response has been reported in a phase II clinical trial combining the multipeptide IMA901 with sunitinib in the treatment of mRCC (59).
Although progress has been made in the understanding of the impact of AATs, combinatorial approaches with cancer vaccines have failed to demonstrate clinical efficacy. To improve clinical outcomes, before considering vaccination we need a better understanding of immunological remodeling induced by antiangiogenic therapies and an assessment of the immunological stage of patients already on antiangiogenic drugs in the context of standard of care treatment. On the other hand, as mentioned above, attention should be paid to the treatment schedule and the doses of AAT. Clinical trials are usually not design to address this issue. To date, the combination of vaccines and antiangiogenic therapies has been less studied than other combinations, although numerous clinical trials are ongoing (some are listed in Table 2A) and hopefully will provide new insights into how such strategies might optimize synergistic effects.
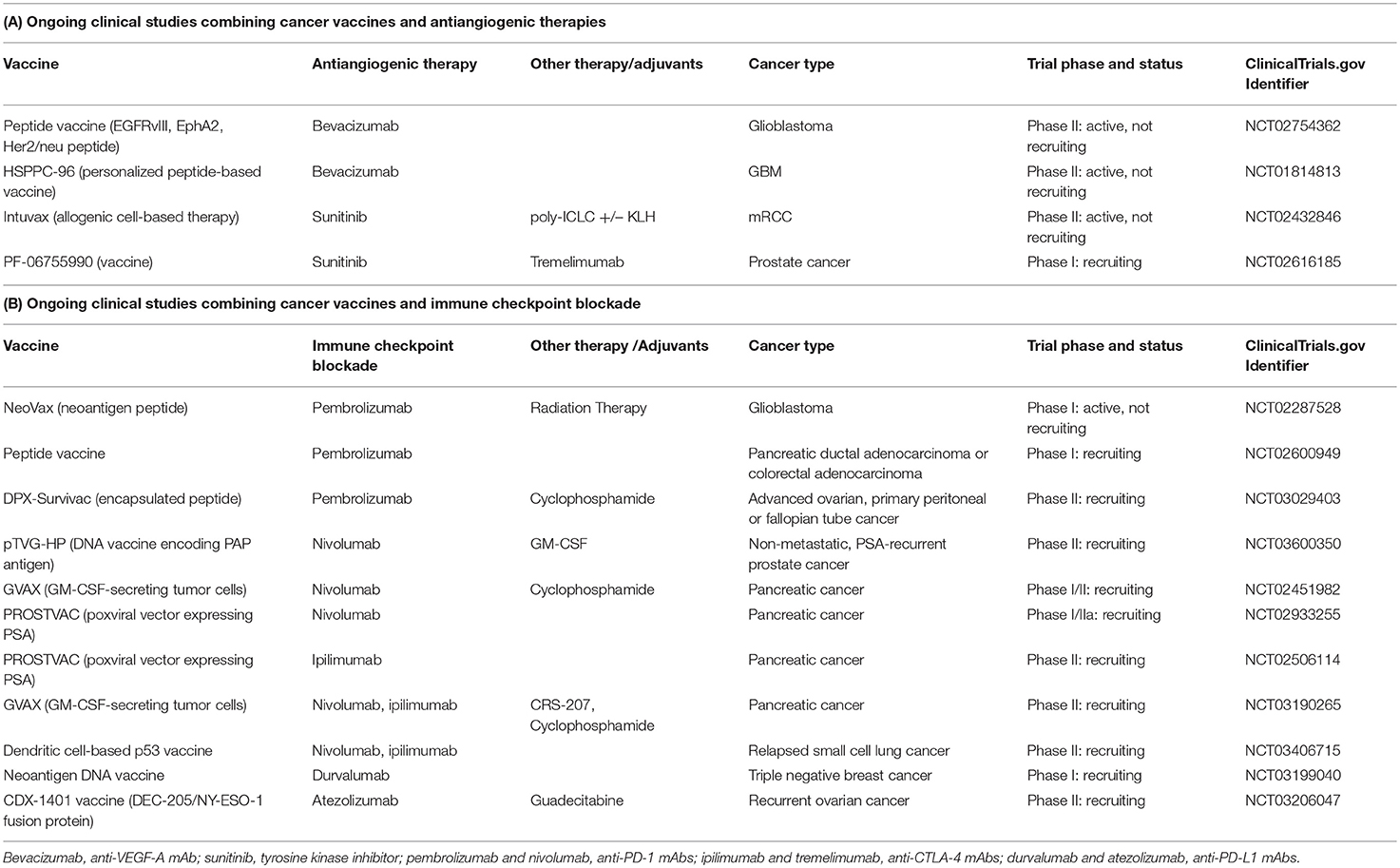
Table 2. Some examples of ongoing clinical trials combining cancer vaccines and antiangiogenic therapies (A) or immune checkpoint blockade (B).
Therapeutic Cancer Vaccines and Immune Checkpoint Blockade Combinations
Rationale for Vaccine Combination With ICB
As described before, the acquisition of immune checkpoint molecules by effector T cells in the tumor microenvironment render them progressively exhausted and unable to kill tumor cells. This has led in recent years to the development of ICB, which is now used in the treatment of many types of cancer.
Monoclonal antibodies (mAbs) directed against co-inhibitory molecules involved in T cell exhaustion or Treg cell function such as CTLA-4 (cytotoxic T-lymphocyte-associated antigen 4) and the PD-1/PD-L1 axes (programmed cell death 1/programmed death-ligand 1) have revolutionized the treatment of an increasing number of cancers, including melanoma, lung cancer, renal cancer, bladder cancer, and Hodgkin's lymphoma (60).
Although success stories such as anti-CTLA-4 and anti-PD-1 mAbs have emerged, these strategies seem to work as monotherapies in a restricted number of patients and some limitations have emerged, with the development of acquired resistance (61, 62). It is important to highlight that poorly immunogenic tumors (also referred to as “cold” tumors), like pancreatic and prostate cancers, are not sensitive to checkpoint blockade (63). Accordingly, the rationale of combining ICB with therapies that increase the number of infiltrating tumor-specific T cells, such as vaccination, is often underlined (63, 64).
Preclinical Studies Combining ICB And Vaccines
Anti-CTLA-4 mAbs in combination with cancer vaccines have been tested in some preclinical studies. For instance, a GM-CSF-producing tumor cell vaccine combined with CTLA-4 blockade has important synergistic effects in reducing tumor size and increasing the antitumor immune response in a melanoma model (65) and in a prostate cancer model (66).
Wada et al. have demonstrated the importance of timing with the combination of anti-CTLA-4 mAbs and GM-CSF gene-transfected tumor cell (GVAX) vaccine in the prostate cancer model Pro-TRAMP (67), by showing that anti-CTLA-4 mAbs should be administered after vaccination to produce additive effects. They hypothesized that delayed CTLA-4 blockade could avoid compensatory expansion of the Treg compartment, which might affect generation of an effective antitumor response.
To improve treatment of pancreatic ductal adenocarcinoma in which checkpoint inhibitor monotherapies seem to be ineffective, Soares et al. have shown that combining anti-PD-1 with GVAX vaccine improved survival and enhanced T-cell activity in mice (68).
Similar conclusions have been reached with another non-immunogenic tumor model. The combination of DC tumor lysate-based vaccine with PD-1 mAbs resulted in long-term survival in mice bearing large established glioma tumors, while neither treatment alone improved survival (69).
In addition, a preclinical study revealed that PD-1 checkpoint inhibition combined with an adenoviral-based vaccine targeting HPV-E6/E7 protein in the context of E6+/E7+ tumor-bearing mice resulted in a more effective antitumor response (70). The authors also showed that treatment with vaccine alone upregulated the expression of several inhibitory immune checkpoint molecules, including PD-1 and LAG-3 on CD8+ tumor-infiltrating lymphocytes. Another study reported increased PD-1 expression on CD8+ tumor-infiltrating lymphocytes after vaccination and significantly enhanced tumor growth inhibition when vaccine was combined with anti-PD-L1 (71). These results strengthen the rationale for vaccine and ICB combination and suggest that multiple checkpoint inhibition could help enhance the synergy of action with vaccine strategies.
Following this idea, Duraiswamy et al. showed that dual blockade of PD-1 and CTLA-4 combined with GVAX vaccine resulted in the rejection of CT26 colorectal tumor in 100% of mice and of ID8-VEGF ovarian carcinoma in 75% (72). Murine studies therefore support the concept that anti-CTLA-4 and anti-PD-1 mAbs increase the frequency of activated T cells and the effector T cell to Treg ratio in vaccinated tumors. While studies have focused on the scheduling of anti-CTLA-4 mAbs with vaccination, this issue has been less studied for the combination of anti-PD-1 mAbs and vaccine.
Clinical Trials Combining ICB And Vaccines
Following preclinical studies, clinical trials have tested the efficacy of such combinatorial approaches in cancer patients.
In melanoma patients, peptide vaccines have been tested in combination with ipilimumab (anti-CTLA-4), but were not associated with improved outcomes compared to ipilimumab alone (73–75). Nevertheless, some of these peptide vaccines have not shown great immunogenicity when tested alone in preclinical trials.
But combinatorial trials have also yielded promising results. In a phase Ib study, ipilimumab was tested in combination with GVAX vaccine in patients with pancreatic adenocarcinoma. The combination of the vaccine with anti-CTLA-4 therapy improved OS compared to ipilimumab alone (76). In another study, the authors showed that antibody-mediated CTLA-4 blockade increases tumor immunity in some patients who were previously vaccinated with GVAX (77).
In a phase I clinical trial, MART-1 peptide-pulsed DCs combined with tremelimumab (anti-CTLA-4) resulted in objective and durable tumor responses in melanoma patients (78). Of 16 patients, 4 had an objective response, 2 had a partial response, and 2 a complete response. TriMixDC-MEL, autologous monocyte-derived DCs electroporated with synthetic mRNA, combined with ipilimumab in advanced melanoma has shown an encouraging rate (38%) of highly durable tumor responses in a phase II trial, including 8 complete responses and 7 partial responses (79).
Viral vector-based vaccines combined with ICB have also shown great promise in human studies. The PROSTVAC vaccine combined with ipilimumab in treatment of mCRPC in a phase I dose-escalation trial proved safe, but clinical outcome has not been studied (80) and a randomized phase II trial is currently recruiting (NCT02506114). Aside from CTLA-4 blockade, PROSTVAC efficacy is also being assessed when combined with nivolumab (anti-PD-1) (phase I/II recruiting) (NCT02933255).
Clinical studies of a combination of anti-PD-1 antibodies and vaccines are still limited, but some early trials show encouraging results.
In a phase I study, patients with advanced solid cancers received p53MVA vaccine combined with pembrolizumab (anti-PD-1) (81). Clinical responses were observed in 3/11 patients, in whom disease remained stable for 30, 32, and 49 weeks, associated for two of them with an increased frequency and persistence of p53-reactive CD8+ T cells.
In a single-arm, phase II clinical trial, 24 patients with incurable HPV-16–positive cancer were vaccinated with ISA 101, a synthetic long-peptide vaccine composed of overlapping HPV E6 and E7 peptides in combination with nivolumab (82). The overall response rate of 33% and median OS of 17.5 months are promising compared to the 16–22% overall response rate and median survival of ~9 months with PD-1 inhibitors alone in similar patients (63).
Two phase I studies have shown that nivolumab in combination with peptide vaccines targeting differentiation antigens is safe and produces an immunological response in melanoma patients (83, 84).
Although clinical data on the combination of anti-CTLA-4 or anti-PD-1 mAbs and vaccines are still limited, some phase I or II clinical trials are ongoing (85). Table 2B lists ongoing clinical trials combining those mAbs but also involving PD-L1 blockade with therapeutic vaccines with or without more conventional therapies (radiotherapy, chemotherapy).
Regarding the complexity of assessing real clinical improvement in such combinatorial trials, an effort should be made in terms of trial design to determine whether the efficacy of vaccine-induced immune responses is improved when combined with immune checkpoint inhibition. It will also be necessary to evaluate immune mechanisms involved in the response to treatment in patients. Those parameters will then allow better scheduling for combination therapy, depending on the nature of the therapeutic vaccine and inhibitory molecules used.
Conclusions and Perspectives
Although therapeutic cancer vaccines have been associated with past failures, the era of combinatorial strategies in the treatment of cancer prompts their reconsideration. Strategies have been optimized and immunologic enhancement due to vaccines is now accepted. The overwhelming immunosuppressive tumor microenvironment that reduces the clinical efficacy of vaccines can now be modified by different approaches. Combinations of cancer vaccines and antiangiogenic therapies or ICB have emerged and shown promising results. To date, very impressive results for those combinations described in mice have not yet been recapitulated in humans. However, studies in mice have mainly used sub-cutaneous tumor grafts growing rapidly and representing an early stage of the disease. Conversely, clinical trials mainly concern patients with advanced cancers, i.e., at a late phase of the disease when immunosuppressive mechanisms are induced. Consequently, we currently lack clinical data showing any breakthrough, a better understanding of the tumor microenvironment will allow us to consider new combinations. Questions remain concerning the timing of treatments, adjuvants, immunization routes, optimal immunogenic vaccines, and tumor remodeling. There is also a need to set up clinical trials in patients at early disease stages. Combinations including newly developed ICB or costimulatory pathways as well as other antiangiogenic strategies such as vaccines directly targeting angiogenic compounds could also bring new hope and lead to clinical success. Finally, in the near future, multiple therapies involving distinct but complementary aspects of antitumor responses may be considered as the combination of vaccines, antiangiogenic therapies and ICB.
Author Contributions
All authors listed have made a substantial, direct and intellectual contribution to the work, and approved it for publication.
Conflict of Interest Statement
The authors declare that the research was conducted in the absence of any commercial or financial relationships that could be construed as a potential conflict of interest.
References
1. Kantoff PW, Higano CS, Shore ND, Berger ER, Small EJ, Penson DF, et al. Sipuleucel-T immunotherapy for castration-resistant prostate cancer. N Engl J Med. (2010) 363:411–22. doi: 10.1056/NEJMoa1001294
2. Romero P, Valmori D, Pittet MJ, Zippelius A, Rimoldi D, Lévy F, et al. Antigenicity and immunogenicity of Melan-A/MART-1 derived peptides as targets for tumor reactive CTL in human melanoma. Immunol Rev. (2002) 188:81–96. doi: 10.1034/j.1600-065X.2002.18808.x
3. Gnjatic S, Nishikawa H, Jungbluth AA, Güre AO, Ritter G, Jäger E, et al. NY-ESO-1: review of an immunogenic tumor antigen. Adv Cancer Res. (2006) 95:1–30. doi: 10.1016/S0065-230X(06)95001-5
4. Hobo W, Strobbe L, Maas F, Fredrix H, Greupink-Draaisma A, Esendam B, et al. Immunogenicity of dendritic cells pulsed with MAGE3, Survivin and B-cell maturation antigen mRNA for vaccination of multiple myeloma patients. Cancer Immunol Immunother. (2013) 62:1381–92. doi: 10.1007/s00262-013-1438-2
5. Türeci Ö, Vormehr M, Diken M, Kreiter S, Huber C, Sahin U. Targeting the heterogeneity of cancer with individualized neoepitope vaccines. Clin Cancer Res. (2016) 22:1885–96. doi: 10.1158/1078-0432.CCR-15-1509
6. Ott PA, Hu Z, Keskin DB, Shukla SA, Sun J, Bozym DJ, et al. An immunogenic personal neoantigen vaccine for patients with melanoma. Nature. (2017) 547:217–21. doi: 10.1038/nature22991
7. Sahin U, Derhovanessian E, Miller M, Kloke B-P, Simon P, Löwer M, et al. Personalized RNA mutanome vaccines mobilize poly-specific therapeutic immunity against cancer. Nature. (2017) 547:222–6. doi: 10.1038/nature23003
8. Yarchoan M, Johnson BA, Lutz ER, Laheru DA, Jaffee EM. Targeting neoantigens to augment antitumour immunity. Nat Rev Cancer. (2017) 17:209–22. doi: 10.1038/nrc.2016.154
9. Guo Y, Lei K, Tang L. Neoantigen vaccine delivery for personalized anticancer immunotherapy. Front Immunol. (2018) 9:1499. doi: 10.3389/fimmu.2018.01499
10. Hu Z, Ott PA, Wu CJ. Towards personalized, tumour-specific, therapeutic vaccines for cancer. Nat Rev Immunol. (2018) 18:168–82. doi: 10.1038/nri.2017.131
11. van der Burg SH, Arens R, Ossendorp F, van Hall T, Melief CJM. Vaccines for established cancer: overcoming the challenges posed by immune evasion. Nat Rev Cancer. (2016) 16:219–33. doi: 10.1038/nrc.2016.16
12. Wong KK, Li WA, Mooney DJ, Dranoff G. Advances in therapeutic cancer vaccines. Adv Immunol. (2016) 130:191–249. doi: 10.1016/bs.ai.2015.12.001
13. Maeng H, Terabe M, Berzofsky JA. Cancer vaccines: translation from mice to human clinical trials. Curr Opin Immunol. (2018) 51:111–22. doi: 10.1016/j.coi.2018.03.001
14. Finn OJ. The dawn of vaccines for cancer prevention. Nat Rev Immunol. (2018) 18:183–94. doi: 10.1038/nri.2017.140
15. Zhang R, Billingsley MM, Mitchell MJ. Biomaterials for vaccine-based cancer immunotherapy. J Control Release. (2018) 292:256–76. doi: 10.1016/j.jconrel.2018.10.008
16. Hale DF, Vreeland TJ, Peoples GE. Arming the immune system through vaccination to prevent cancer recurrence. Am Soc Clin Oncol Educ Book. (2016) 35:e159–67. doi: 10.14694/EDBK_158946
17. McNeel DG. Therapeutic cancer vaccines: how much closer are we? BioDrugs. (2018) 32:1–7. doi: 10.1007/s40259-017-0257-y
18. Schadendorf D, Ugurel S, Schuler-Thurner B, Nestle FO, Enk A, Bröcker EB, et al. Dacarbazine (DTIC) versus vaccination with autologous peptide-pulsed dendritic cells (DC) in first-line treatment of patients with metastatic melanoma: a randomized phase III trial of the DC study group of the DeCOG. Ann Oncol. (2006) 17:563–70. doi: 10.1093/annonc/mdj138
19. Oudard S, Rixe O, Beuselinck B, Linassier C, Banu E, Machiels JP, et al. A phase II study of the cancer vaccine TG4010 alone and in combination with cytokines in patients with metastatic renal clear-cell carcinoma: clinical and immunological findings. Cancer Immunol Immunother. (2011) 60:261–71. doi: 10.1007/s00262-010-0935-9
20. Tosch C, Bastien B, Barraud L, Grellier B, Nourtier V, Gantzer M, et al. Viral based vaccine TG4010 induces broadening of specific immune response and improves outcome in advanced NSCLC. J Immunother Cancer. (2017) 5:70. doi: 10.1186/s40425-017-0274-x
21. Amato RJ, Hawkins RE, Kaufman HL, Thompson JA, Tomczak P, Szczylik C, et al. Vaccination of metastatic renal cancer patients with MVA-5T4: a randomized, double-blind, placebo-controlled phase III study. Clin Cancer Res. (2010) 16:5539–47. doi: 10.1158/1078-0432.CCR-10-2082
22. Kantoff PW, Schuetz TJ, Blumenstein BA, Glode LM, Bilhartz DL, Wyand M, et al. Overall survival analysis of a phase II randomized controlled trial of a Poxviral-based PSA-targeted immunotherapy in metastatic castration-resistant prostate cancer. J Clin Oncol. (2010) 28:1099–105. doi: 10.1200/JCO.2009.25.0597
23. Higano CS, Corman JM, Smith DC, Centeno AS, Steidle CP, Gittleman M, et al. Phase 1/2 dose-escalation study of a GM-CSF-secreting, allogeneic, cellular immunotherapy for metastatic hormone-refractory prostate cancer. Cancer. (2008) 113:975–84. doi: 10.1002/cncr.23669
24. Lutz E, Yeo CJ, Lillemoe KD, Biedrzycki B, Kobrin B, Herman J, et al. A Lethally irradiated allogeneic granulocyte-macrophage colony stimulating factor-secreting tumor vaccine for pancreatic adenocarcinoma: a phase II trial of safety, efficacy, and immune activation. Ann Surg. (2011) 253:328–35. doi: 10.1097/SLA.0b013e3181fd271c
25. Vansteenkiste JF, Cho BC, Vanakesa T, De Pas T, Zielinski M, Kim MS, et al. Efficacy of the MAGE-A3 cancer immunotherapeutic as adjuvant therapy in patients with resected MAGE-A3-positive non-small-cell lung cancer (MAGRIT): a randomised, double-blind, placebo-controlled, phase 3 trial. Lancet Oncol. (2016) 17:822–35. doi: 10.1016/S1470-2045(16)00099-1
26. Weller M, Butowski N, Tran DD, Recht LD, Lim M, Hirte H, et al. Rindopepimut with temozolomide for patients with newly diagnosed, EGFRvIII-expressing glioblastoma (ACT IV): a randomised, double-blind, international phase 3 trial. Lancet Oncol. (2017) 18:1373–85. doi: 10.1016/S1470-2045(17)30517-X
27. Walter S, Weinschenk T, Stenzl A, Zdrojowy R, Pluzanska A, Szczylik C, et al. Multipeptide immune response to cancer vaccine IMA901 after single-dose cyclophosphamide associates with longer patient survival. Nat Med. (2012) 18:1254–61. doi: 10.1038/nm.2883
28. Butts C, Socinski MA, Mitchell PL, Thatcher N, Havel L, Krzakowski M, et al. Tecemotide (L-BLP25) versus placebo after chemoradiotherapy for stage III non-small-cell lung cancer (START): a randomised, double-blind, phase 3 trial. Lancet Oncol. (2014) 15:59–68. doi: 10.1016/S1470-2045(13)70510-2
29. Lawson DH, Lee S, Zhao F, Tarhini AA, Margolin KA, Ernstoff MS, et al. Randomized, placebo-controlled, phase III trial of yeast-derived Granulocyte-Macrophage Colony-Stimulating Factor (GM-CSF) versus peptide vaccination versus GM-CSF plus peptide vaccination versus placebo in patients with no evidence of disease after complete surgical resection of locally advanced and/or stage IV melanoma: a trial of the Eastern Cooperative Oncology Group-American College of Radiology Imaging Network Cancer Research Group (E4697). J Clin Oncol. (2015) 33:4066–76. doi: 10.1200/JCO.2015.62.0500
30. Figdor CG, de Vries IJM, Lesterhuis WJ, Melief CJM. Dendritic cell immunotherapy: mapping the way. Nat Med. (2004) 10:475–80. doi: 10.1038/nm1039
31. Wilgenhof S, Corthals J, Van Nuffel AMT, Benteyn D, Heirman C, Bonehill A, et al. Long-term clinical outcome of melanoma patients treated with messenger RNA-electroporated dendritic cell therapy following complete resection of metastases. Cancer Immunol Immunother. (2015) 64:381–8. doi: 10.1007/s00262-014-1642-8
32. Palucka K, Banchereau J. Cancer immunotherapy via dendritic cells. Nat Rev Cancer. (2012) 12:265–77. doi: 10.1038/nrc3258
33. Pizzurro GA, Barrio MM. Dendritic cell-based vaccine efficacy: aiming for hot spots. Front Immunol. (2015) 6:91. doi: 10.3389/fimmu.2015.00091
34. Saxena M, Bhardwaj N. Re-emergence of dendritic cell vaccines for cancer treatment. Trends Cancer. (2018) 4:119–37. doi: 10.1016/j.trecan.2017.12.007
35. Sandoval F, Terme M, Nizard M, Badoual C, Bureau MF, Freyburger L, et al. Mucosal imprinting of vaccine-induced CD8+ T cells is crucial to inhibit the growth of mucosal tumors. Sci Transl Med. (2013) 5:172ra20. doi: 10.1126/scitranslmed.3004888
36. Banday AH, Jeelani S, Hruby VJ. Cancer vaccine adjuvants–recent clinical progress and future perspectives. Immunopharmacol Immunotoxicol. (2015) 37:1–11. doi: 10.3109/08923973.2014.971963
37. Bowen WS, Svrivastava AK, Batra L, Barsoumian H, Shirwan H. Current challenges for cancer vaccine adjuvant development. Expert Rev Vaccines. (2018) 17:207–15. doi: 10.1080/14760584.2018.1434000
38. Sokolowska O, Nowis D. STING signaling in cancer cells: important or not? Arch Immunol Ther Exp. (2018) 66:125–32. doi: 10.1007/s00005-017-0481-7
39. Schreiber RD, Old LJ, Smyth MJ. Cancer immunoediting: integrating immunity's roles in cancer suppression and promotion. Science. (2011) 331:1565–70. doi: 10.1126/science.1203486
40. Schaaf MB, Garg AD, Agostinis P. Defining the role of the tumor vasculature in antitumor immunity and immunotherapy. Cell Death Dis. (2018) 9:115. doi: 10.1038/s41419-017-0061-0
41. Beyranvand Nejad E, Welters MJP, Arens R, van der Burg SH. The importance of correctly timing cancer immunotherapy. Expert Opin Biol Ther. (2017) 17:87–103. doi: 10.1080/14712598.2017.1256388
42. van Gulijk M, Dammeijer F, Aerts JGJV, Vroman H. Combination strategies to optimize efficacy of dendritic cell-based immunotherapy. Front Immunol. (2018) 9:2759. doi: 10.3389/fimmu.2018.02759
43. Carmeliet P, Jain RK. Molecular mechanisms and clinical applications of angiogenesis. Nature. (2011) 473:298–307. doi: 10.1038/nature10144
44. Melder RJ, Koenig GC, Witwer BP, Safabakhsh N, Munn LL, Jain RK. During angiogenesis, vascular endothelial growth factor and basic fibroblast growth factor regulate natural killer cell adhesion to tumor endothelium. Nat Med. (1996) 2:992–7. doi: 10.1038/nm0996-992
45. Voron T, Colussi O, Marcheteau E, Pernot S, Nizard M, Pointet A-L, et al. VEGF-A modulates expression of inhibitory checkpoints on CD8+ T cells in tumors. J Exp Med. (2015) 212:139–48. doi: 10.1084/jem.20140559
46. Hendry SA, Farnsworth RH, Solomon B, Achen MG, Stacker SA, Fox SB. The role of the tumor vasculature in the host immune response: implications for therapeutic strategies targeting the tumor microenvironment. Front Immunol. (2016) 7:621. doi: 10.3389/fimmu.2016.00621
47. Fukumura D, Kloepper J, Amoozgar Z, Duda DG, Jain RK. Enhancing cancer immunotherapy using antiangiogenics: opportunities and challenges. Nat Rev Clin Oncol. (2018) 15:325–40. doi: 10.1038/nrclinonc.2018.29
48. Lapeyre-Prost A, Terme M, Pernot S, Pointet AL, Voron T, Tartour E, et al. Immunomodulatory activity of VEGF in cancer. Int Rev Cell Mol Biol. (2017) 330:295–342. doi: 10.1016/bs.ircmb.2016.09.007
49. Jain RK. Normalization of tumor vasculature: an emerging concept in antiangiogenic therapy. Science. (2005) 307:58–62. doi: 10.1126/science.1104819
50. Vasudev NS, Reynolds AR. Anti-angiogenic therapy for cancer: current progress, unresolved questions and future directions. Angiogenesis. (2014) 17:471–94. doi: 10.1007/s10456-014-9420-y
51. Bergers G, Hanahan D. Modes of resistance to anti-angiogenic therapy. Nat Rev Cancer. (2008) 8:592–603. doi: 10.1038/nrc2442
52. van Beijnum JR, Nowak-Sliwinska P, Huijbers EJM, Thijssen VL, Griffioen AW. The great escape; the hallmarks of resistance to antiangiogenic therapy. Pharmacol Rev. (2015) 67:441–61. doi: 10.1124/pr.114.010215
53. Bose A, Taylor JL, Alber S, Watkins SC, Garcia JA, Rini BI, et al. Sunitinib facilitates the activation and recruitment of therapeutic anti-tumor immunity in concert with specific vaccination. Int J Cancer. (2011) 129:2158–70. doi: 10.1002/ijc.25863
54. Bose A, Lowe DB, Rao A, Storkus WJ. Combined vaccine+axitinib therapy yields superior antitumor efficacy in a murine melanoma model. Melanoma Res. (2012) 22:236–43. doi: 10.1097/CMR.0b013e3283538293
55. Farsaci B, Higgins JP, Hodge JW. Consequence of dose scheduling of sunitinib on host immune response elements and vaccine combination therapy. Int J Cancer. (2012) 130:1948–59. doi: 10.1002/ijc.26219
56. Jaini R, Rayman P, Cohen PA, Finke JH, Tuohy VK. Combination of sunitinib with anti-tumor vaccination inhibits T cell priming and requires careful scheduling to achieve productive immunotherapy. Int J Cancer. (2014) 134:1695–705. doi: 10.1002/ijc.28488
57. Huang Y, Yuan J, Righi E, Kamoun WS, Ancukiewicz M, Nezivar J, et al. Vascular normalizing doses of antiangiogenic treatment reprogram the immunosuppressive tumor microenvironment and enhance immunotherapy. Proc Natl Acad Sci USA. (2012) 109:17561–6. doi: 10.1073/pnas.1215397109
58. Amin A, Dudek AZ, Logan TF, Lance RS, Holzbeierlein JM, Knox JJ, et al. Survival with AGS-003, an autologous dendritic cell-based immunotherapy, in combination with sunitinib in unfavorable risk patients with advanced renal cell carcinoma (RCC): phase 2 study results. J Immunother Cancer. (2015) 3:14. doi: 10.1186/s40425-015-0055-3
59. Rini BI, Stenzl A, Zdrojowy R, Kogan M, Shkolnik M, Oudard S, et al. IMA901, a multipeptide cancer vaccine, plus sunitinib versus sunitinib alone, as first-line therapy for advanced or metastatic renal cell carcinoma (IMPRINT): a multicentre, open-label, randomised, controlled, phase 3 trial. Lancet Oncol. (2016) 17:1599–611. doi: 10.1016/S1470-2045(16)30408-9
60. Sharma P, Allison JP. The future of immune checkpoint therapy. Science. (2015) 348:56–61. doi: 10.1126/science.aaa8172
61. Sharma P, Hu-Lieskovan S, Wargo JA, Ribas A. Primary, adaptive, and acquired resistance to cancer immunotherapy. Cell. (2017) 168:707–23. doi: 10.1016/j.cell.2017.01.017
62. Seidel JA, Otsuka A, Kabashima K. Anti-PD-1 and Anti-CTLA-4 therapies in cancer: mechanisms of action, efficacy, and limitations. Front Oncol. (2018) 8:86. doi: 10.3389/fonc.2018.00086
63. Curran MA, Glisson BS. New hope for therapeutic cancer vaccines in the era of immune checkpoint modulation. Annu Rev Med. (2018) 70:409–24. doi: 10.1146/annurev-med-050217-121900
64. Karaki S, Anson M, Tran T, Giusti D, Blanc C, Oudard S, et al. Is there still room for cancer vaccines at the era of checkpoint inhibitors. Vaccines. (2016) 4:E37. doi: 10.3390/vaccines4040037
65. van Elsas A, Hurwitz AA, Allison JP. Combination immunotherapy of B16 melanoma using anti-cytotoxic T lymphocyte-associated antigen 4 (CTLA-4) and granulocyte/macrophage colony-stimulating factor (GM-CSF)-producing vaccines induces rejection of subcutaneous and metastatic tumors accompanied by autoimmune depigmentation. J Exp Med. (1999) 190:355–66.
66. Hurwitz AA, Foster BA, Kwon ED, Truong T, Choi EM, Greenberg NM, et al. Combination immunotherapy of primary prostate cancer in a transgenic mouse model using CTLA-4 blockade. Cancer Res. (2000) 60:2444–8.
67. Wada S, Jackson CM, Yoshimura K, Yen HR, Getnet D, Harris TJ, et al. Sequencing CTLA-4 blockade with cell-based immunotherapy for prostate cancer. J Transl Med. (2013) 11:89. doi: 10.1186/1479-5876-11-89
68. Soares KC, Rucki AA, Wu AA, Olino K, Xiao Q, Chai Y, et al. PD-1/PD-L1 blockade together with vaccine therapy facilitates effector T-cell infiltration into pancreatic tumors. J Immunother. (2015) 38:1–11. doi: 10.1097/CJI.0000000000000062
69. Antonios JP, Soto H, Everson RG, Orpilla J, Moughon D, Shin N, et al. PD-1 blockade enhances the vaccination-induced immune response in glioma. JCI Insight. (2016) 1:e87059. doi: 10.1172/jci.insight.87059
70. Rice AE, Latchman YE, Balint JP, Lee JH, Gabitzsch ES, Jones FR. An HPV-E6/E7 immunotherapy plus PD-1 checkpoint inhibition results in tumor regression and reduction in PD-L1 expression. Cancer Gene Ther. (2015) 22:454–62. doi: 10.1038/cgt.2015.40
71. Badoual C, Hans S, Merillon N, Van Ryswick C, Ravel P, Benhamouda N, et al. PD-1-expressing tumor-infiltrating T cells are a favorable prognostic biomarker in HPV-associated head and neck cancer. Cancer Res. (2013) 73:128–38. doi: 10.1158/0008-5472.CAN-12-2606
72. Duraiswamy J, Kaluza KM, Freeman GJ, Coukos G. Dual blockade of PD-1 and CTLA-4 combined with tumor vaccine effectively restores T-cell rejection function in tumors. Cancer Res. (2013) 73:3591–603. doi: 10.1158/0008-5472.CAN-12-4100
73. Hodi FS, O'Day SJ, McDermott DF, Weber RW, Sosman JA, Haanen JB, et al. Improved survival with ipilimumab in patients with metastatic melanoma. N Engl J Med. (2010) 363:711–23. doi: 10.1056/NEJMoa1003466
74. Sarnaik AA, Yu B, Yu D, Morelli D, Hall M, Bogle D, et al. Extended dose ipilimumab with a peptide vaccine: immune correlates associated with clinical benefit in patients with resected high-risk stage IIIc/IV melanoma. Clin Cancer Res. (2011) 17:896–906. doi: 10.1158/1078-0432.CCR-10-2463
75. Bjoern J, Iversen TZ, Nitschke NJ, Andersen MH, Svane IM. Safety, immune and clinical responses in metastatic melanoma patients vaccinated with a long peptide derived from indoleamine 2,3-dioxygenase in combination with ipilimumab. Cytotherapy. (2016) 18:1043–55. doi: 10.1016/j.jcyt.2016.05.010
76. Le DT, Lutz E, Uram JN, Sugar EA, Onners B, Solt S, et al. Evaluation of ipilimumab in combination with allogeneic pancreatic tumor cells transfected with a GM-CSF gene in previously treated pancreatic cancer. J Immunother. (2013) 36:382–9. doi: 10.1097/CJI.0b013e31829fb7a2
77. Hodi FS, Mihm MC, Soiffer RJ, Haluska FG, Butler M, Seiden MV, et al. Biologic activity of cytotoxic T lymphocyte-associated antigen 4 antibody blockade in previously vaccinated metastatic melanoma and ovarian carcinoma patients. Proc Natl Acad Sci USA. (2003) 100:4712–7. doi: 10.1073/pnas.0830997100
78. Ribas A, Comin-Anduix B, Chmielowski B, Jalil J, de la Rocha P, McCannel TA, et al. Dendritic cell vaccination combined with CTLA4 blockade in patients with metastatic melanoma. Clin Cancer Res. (2009) 15:6267–76. doi: 10.1158/1078-0432.CCR-09-1254
79. Wilgenhof S, Corthals J, Heirman C, van Baren N, Lucas S, Kvistborg P, et al. Phase II study of autologous monocyte-derived mRNA electroporated dendritic cells (TriMixDC-MEL) plus ipilimumab in patients with pretreated advanced melanoma. J Clin Oncol. (2016) 34:1330–8. doi: 10.1200/JCO.2015.63.4121
80. Madan RA, Mohebtash M, Arlen PM, Vergati M, Rauckhorst M, Steinberg SM, et al. Ipilimumab and a poxviral vaccine targeting prostate-specific antigen in metastatic castration-resistant prostate cancer: a phase 1 dose-escalation trial. Lancet Oncol. (2012) 13:501–8. doi: 10.1016/S1470-2045(12)70006-2
81. Chung V, Kos FJ, Hardwick N, Yuan Y, Chao J, Li D, et al. Evaluation of safety and efficacy of p53MVA vaccine combined with pembrolizumab in patients with advanced solid cancers. Clin Transl Oncol. (2018) 21:363–72. doi: 10.1007/s12094-018-1932-2
82. Massarelli E, William W, Johnson F, Kies M, Ferrarotto R, Guo M, et al. Combining immune checkpoint blockade and tumor-specific vaccine for patients with incurable human papillomavirus 16-related cancer: a phase 2 clinical trial. JAMA Oncol. (2018) 5:67–73. doi: 10.1001/jamaoncol.2018.4051
83. Weber JS, Kudchadkar RR, Yu B, Gallenstein D, Horak CE, Inzunza HD, et al. Safety, efficacy, and biomarkers of nivolumab with vaccine in ipilimumab-refractory or -naive melanoma. J Clin Oncol. (2013) 31:4311–8. doi: 10.1200/JCO.2013.51.4802
84. Gibney GT, Kudchadkar RR, DeConti RC, Thebeau MS, Czupryn MP, Tetteh L, et al. Safety, correlative markers, and clinical results of adjuvant nivolumab in combination with vaccine in resected high-risk metastatic melanoma. Clin Cancer Res. (2015) 21:712–20. doi: 10.1158/1078-0432.CCR-14-2468
Keywords: cancer vaccines, immunotherapies, combinatorial strategies, antiangiogenic treatments, immune checkpoint blockade
Citation: Mougel A, Terme M and Tanchot C (2019) Therapeutic Cancer Vaccine and Combinations With Antiangiogenic Therapies and Immune Checkpoint Blockade. Front. Immunol. 10:467. doi: 10.3389/fimmu.2019.00467
Received: 24 October 2018; Accepted: 21 February 2019;
Published: 14 March 2019.
Edited by:
Patrik Andersson, Massachusetts General Hospital and Harvard Medical School, United StatesReviewed by:
Viktor Umansky, German Cancer Research Center (DKFZ), GermanyYunlong Yang, Fudan University, China
Copyright © 2019 Mougel, Terme and Tanchot. This is an open-access article distributed under the terms of the Creative Commons Attribution License (CC BY). The use, distribution or reproduction in other forums is permitted, provided the original author(s) and the copyright owner(s) are credited and that the original publication in this journal is cited, in accordance with accepted academic practice. No use, distribution or reproduction is permitted which does not comply with these terms.
*Correspondence: Magali Terme, bWFnYWxpLnRlcm1lQGluc2VybS5mcg==
Corinne Tanchot, Y29yaW5uZS50YW5jaG90QGluc2VybS5mcg==