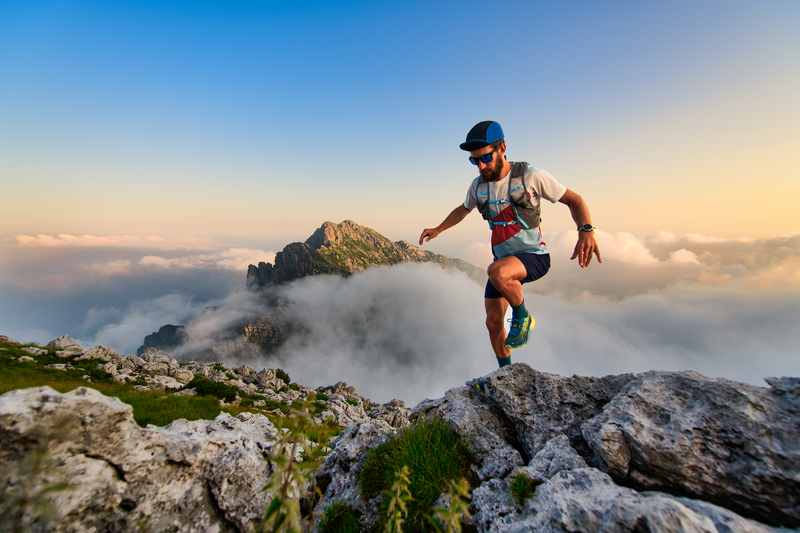
95% of researchers rate our articles as excellent or good
Learn more about the work of our research integrity team to safeguard the quality of each article we publish.
Find out more
ORIGINAL RESEARCH article
Front. Immunol. , 11 March 2019
Sec. Molecular Innate Immunity
Volume 10 - 2019 | https://doi.org/10.3389/fimmu.2019.00423
Neutrophil Extracellular Traps (NETs) are implicated in the development of auto-immunity in diseases such as rheumatoid arthritis (RA) and systemic lupus erythematosus (SLE) through the externalization of intracellular neoepitopes e.g., dsDNA and nuclear proteins in SLE and citrullinated peptides in RA. The aim of this work was to use quantitative proteomics to identify and measure NET proteins produced by neutrophils from healthy controls, and from patients with RA and SLE to determine if NETs can be differentially-generated to expose different sets of neoepitopes. Ultra-pure neutrophils (>99%) from healthy individuals (n = 3) and patients with RA or SLE (n = 6 each) were incubated ± PMA (50 nM, PKC super-activator) or A23187 (3.8 μM, calcium ionophore) for 4 h. NETs were liberated by nuclease digestion and concentrated onto Strataclean beads prior to on-bead digestion with trypsin. Data-dependent LC-MS/MS analyses were conducted on a QExactive HF quadrupole-Orbitrap mass spectrometer, and label-free protein quantification was carried out using Progenesis QI. PMA-induced NETs were decorated with annexins, azurocidin and histone H3, whereas A23187-induced NETs were decorated with granule proteins including CAMP/LL37, CRISP3, lipocalin and MMP8, histones H1.0, H1.4, and H1.5, interleukin-8, protein-arginine deiminase-4 (PADI4), and α-enolase. Four proteins were significantly different between PMA-NETs from RA and SLE neutrophils (p < 0.05): RNASE2 was higher in RA, whereas MPO, leukocyte elastase inhibitor and thymidine phosphorylase were higher in SLE. For A23187-NETs, six NET proteins were higher in RA (p < 0.05), including CAMP/LL37, CRISP3, interleukin-8, MMP8; Thirteen proteins were higher in SLE, including histones H1.0, H2B, and H4. This work provides the first, direct comparison of NOX2-dependent (PMA) and NOX2-independent (A23187) NETs using quantitative proteomics, and the first direct comparison of RA and SLE NETs using quantitative proteomics. We show that it is the nature of the stimulant rather than neutrophil physiology that determines NET protein profiles in disease, since stimulation of NETosis in either a NOX2-dependent or a NOX2-independent manner generates broadly similar NET proteins irrespective of the disease background. We also use our proteomics pipeline to identify an extensive range of post-translationally modified proteins in RA and SLE, including histones and granule proteins, many of which are known targets of auto-antibodies in each disease.
Neutrophil Extracellular Traps (NETs) are chromatin-derived extracellular “spider webs” that are expelled from neutrophils in response to infectious or inflammatory stimuli (1). They were first described as an alternative defense mechanism by which neutrophils trap and possibly kill microbes (1), with subsequent studies confirming this (2–6). Microbes including bacteria, fungi and viruses have varying susceptibility to NETs, either being trapped and/or killed, or having their growth inhibited (7). NET DNA structures are decorated with histones, myeloperoxidase (MPO), and other antimicrobial proteins such as neutrophil elastase. Some NET proteins, including histones, may be post-translationally modified with methylated, acetylated, and/or citrullinated residues (8–12). Many inducers of NET release (NETosis) have been identified, including physiological and non-physiological molecules, and micro-organisms including gram-positive and gram–negative bacteria and fungi. The most potent and commonly used non-physiological inducer of NETosis in vitro is phorbol 12-myristate 13-acetate (PMA) (13–15), a super-activator of protein kinase C (PKC). Calcium ionophores such as ionomycin and A23187 also induce the release of NETs containing, in particular, citrullinated histones (9–12, 16, 17). Many, more physiologically relevant inducers of NETs have been reported, including N-Formylmethionyl-leucyl-phenylalanine (fMLP), interleukin-8 (IL-8), lipopolysaccharide (LPS), Platelet toll-like receptor (TLR)-4, Nitric Oxide, and TNFα (18–21), although IL-8-induced NET formation may be sensitive to cell culture conditions(22).
In many cases, activation of the NADPH-oxidase (NOX2) and generation of reactive oxygen species (ROS) is required for NET formation (NOX2-dependent NETosis). ROS increase membrane permeability, leading to the release of neutrophil elastase into the nucleus, which first degrades linker H1 histones followed by core histones driving chromatin decondensation, a process enhanced by MPO (23). ROS also promote the morphological changes that occur during NETosis (24), inhibit apoptosis, and induce autophagy (23), with the level of intracellular ROS determining whether the autophagy reaction leads to NETosis (24). Many agonists, including PMA, induce NOX2-dependent NET production (15, 24), which is regulated by the Raf-MEK-ERK pathway (13, 25). There are also conflicting reports about the involvement of RIPK1, RIPK3, and MLKL signaling pathways in PMA-induced NETosis (26, 27). NETosis induced by calcium ionophores such as A23187 and activated platelets occurs in a different manner, independent of NOX2 activity and thus is often referred to as NOX2-independent NET formation (17, 28). NOX2-independent NETosis is dependent on intracellular calcium and activation of peptidylarginine deiminase (PAD) enzymes leading to hypercitrullination of histones (9, 17). Recent work suggests that activation of PAD induces citrullination of p47phox and p67phox proteins, preventing assembly of active NOX2 and production of NOX2-dependent ROS (29). NOX2-independent NETosis relies upon the production of mitochondrial ROS (mtROS) and activation of the calcium-activated small conductance potassium (SK) channel member SK3 (17). Elastase does not seem to be required for NOX2-independent NETosis (30), and neither are F-actin or histones cleaved (23, 31).
Neutrophils are implicated in the pathogenesis of several inflammatory diseases including rheumatoid arthritis (RA) and systemic lupus erythematosus (SLE) (32–36). Both diseases are characterized by a dysregulation of neutrophil activation, including cytokine and ROS production, gene expression and apoptosis (35, 37, 38). RA is a chronic, inflammatory disease affecting the joints, characterized by inflammation of synovial tissues and irreversible damage to cartilage and bone within synovial joints. In RA, neutrophil apoptosis is delayed, particularly within RA joints (39, 40), and activated neutrophils within synovial tissues and synovial fluid release cytotoxic proteases that damage cartilage. SLE is a heterogeneous auto-immune condition with multiple organ involvement, including the skin, kidneys, cardiovascular system, central nervous system, and joints (41, 42). In SLE, neutrophil apoptosis is enhanced leading to an increase in apoptotic burden associated with development of anti-nuclear auto-antibodies (43, 44). Neutrophils are implicated in the development of auto-immunity in both diseases through the production of NETs and externalization of intracellular neoepitopes e.g., dsDNA and nuclear proteins in SLE and citrullinated peptides in RA (8, 21, 45–50).
Increased spontaneous NETosis has been observed in several autoimmune conditions (51–53), including SLE (45, 46) and RA (21), and sera from SLE and RA patients activate neutrophils and cross-react with NET components (8, 21, 47–50). Serum levels of MPO-DNA complexes (NET remnants) are elevated in patients with RA, SLE, primary Sjögren's syndrome, dermatomyositis, and ankylosing spondylitis (54, 55). Analysis of NET remnants in the serum of patients with RA and SLE suggests NETs produced in both diseases originate via NOX2-independent NETosis (55). In RA, MPO-DNA levels are associated with increased neutrophil counts and positivity for rheumatoid factor (RF) and anti-citrullinated protein/peptide antibodies (ACPA) (54, 56). NETs are implicated in the activation of plasmacytoid dendritic cells in SLE (57), and may induce damage to endothelial tissues and organs (44, 45, 58, 59). NETs and NET-derived proteases activate coagulation, and provide a scaffolding for clot assembly, often associated with SLE (60, 61). NETs produced by both SLE neutrophils and SLE low-density granulocytes (LDGs) damage endothelial cells (44, 45, 58).
Previous proteomics studies have identified a number of proteins decorating NETs produced by both healthy and inflammatory neutrophils, including granule proteins (elastase, MPO, azurocidin, lactoferrin, gelatinase), histones (H1, H2A, H2B, H3, H4) and S100 family proteins (S100A8, S100A9, S100A10) (21, 62–64). However, these reports were descriptive and only semi-quantitative. The purpose of our study was to carry out the first fully quantitative proteomics analysis of NETs produced by RA and SLE neutrophils in response to NOX2-dependent and NOX2-independent activation, and to determine whether the protein composition of NETs is agonist and/or disease specific. We describe a comprehensive and quantitative proteomics approach to examining the composition of PMA- and A23187-induced NETs from ultrapure RA and SLE neutrophils, which has identified over 450 NET proteins. We have also analyzed these data to identify NET proteins for post-translational modifications on peptides, many of which correspond to known auto-antibody species in RA and SLE. We show that the mode of stimulation of NETosis (NOX2-dependent or NOX2-independent) generates broadly similar profiles of NET proteins irrespective of the disease background, and that it is the nature of the stimulant rather than the neutrophil physiology that determines NET protein profiles in disease. However, we also describe a number of proteins that are expressed at significantly higher levels on NETs in RA or SLE and which may have important contributions to disease pathology in these two very different inflammatory conditions.
All patients fulfilled ACR criteria for RA or SLE (65, 66) and were recruited from clinics at University Hospital Aintree. Clinical demographics for patients included in the study are shown in Table 1. All patients had active disease as determined by a clinician at the time of sample collection.
HetaSep solution and EasySep Human Neutrophil enrichment kit were from StemCell (Cambridge, UK); Ficoll-Paque was from GE Healthcare (Little Chalfont, UK); RPMI 1640 media plus without phenol red, L-glutamine, 25 mM HEPES and Hanks Balanced Salt Solution (HBSS), Annexin V-FITC, anti-rabbit AlexaFluor488, and anti-mouse AlexaFluor647 were from Life Technologies (Paisley, UK); Rapid Romanowsky stain was from TCS Biosciences (Botolph Claydon, UK); anti-CD16-FITC was from BD Biosciences (Oxford, UK); Propidium Iodide, A23187, phorbol 12-myristate 13-acetate (PMA), R848, LPS, DAPI, Mowiol 4–88, micrococcal nuclease, poly-L-lysine, human AB serum and PhastGel® Blue R Pre-measured tablets were from Sigma (Gillingham, UK); rabbit anti-neutrophil elastase antibody, mouse anti-myeloperoxidase antibody, rabbit anti-CRISP3 antibody, rabbit anti-thymidine phosphorylase antibody, rabbit anti-histone H2B, and mouse anti-PADI4 antibody were from Abcam (Cambridge, UK); goat anti-MMP8 antibody, mouse anti-CAMP (LL37) antibody, mouse anti-LCN1 antibody, and mouse anti-histone H1.0 antibody were from Biotechne (Abingdon, UK); Quantifluor dsDNA kit was from Promega (Southampton, UK); 96-well black plates, lithium-heparin vacutainers and Z-serum clot activator vacutainers were from Greiner (Stonehouse, UK); SYTOX® Green Nucleic Acid Stain and 0.5 M UltraPure EDTA pH8.0 was from ThermoFisher (Loughborough, UK); Strataclean beads were from Agilent (Cheadle, UK), cover slips were from Fischer Scientific (Loughborough, UK); MALP-2 was from Enzo Life Sciences (Exeter, UK); TNFα was from Merck (Watford, UK).
Blood was collected into lithium-heparin vacutainers. Ultra-pure neutrophils (>99%) were isolated from erythrocyte-depleted (HetaSep) whole blood as previously described using Ficoll-Paque followed by the EasySep Human Neutrophil enrichment kit (14, 67). Highly-pure (>99%) neutrophils were briefly centrifuged and resuspended in RPMI 1640 media containing L-glutamine, plus 25 mM HEPES, to a concentration of 5 × 106/mL. Neutrophil purity was confirmed by anti-CD16-FITC staining and morphology. Cytospins were prepared by centrifugation of 105 cells (in PBS with 10 mM EDTA) onto a glass slide at 30 g for 5 min using a Shandon 3 cytospin and immediately stained with Rapid Romanowsky stain.
RA SF from knee joints was aspirated into heparinized tubes and processed within 1 h. Aliquots of whole SF were centrifuged at >2,000 g for 5 min and cell-free SF was decanted and frozen at −80°C. RA serum was obtained by centrifuging blood drawn into Z serum clot activator tubes at 1,500 g for 10 min at RT, before freezing at −80°C.
Neutrophils were incubated at 37°C in 5% CO2 in RPMI 1640 in the absence or presence of human AB serum (0–2%) for 5 h. Following incubation, 2.5 × 104 cells were diluted in 50 μL of HBSS containing 0.5 μL Annexin V-FITC, and incubated in the dark at room temperature for 15 min. The total volume was then made up to 500 μL with HBSS containing propidium-iodide (PI, 1 μg/mL) before analysis by flow cytometry (>5,000 events analyzed) using a Guava EasyCyte flow cytometer.
Neutrophils (5 × 106/mL) were incubated with PMA (50 nM), A23187 (3.8 μM) or vehicle control (DMSO). Luminol-enhanced chemiluminescence (luminol, 10 μM) was measured continuously for 30 min using a Tecan plate reader at 37°C.
Neutrophils were seeded (at 2 × 105 cells/500 μL) in RPMI media plus 2% AB serum in duplicate wells (with or without a glass coverslip in the well) of a 24-well plate. Cells were allowed to adhere for 1 h prior to stimulation with PMA (50 nM), A23187 (3.8 μM) or vehicle control (DMSO). In preliminary experiments neutrophils were also stimulated with TNFα (100 ng/mL), LPS (100 ng/mL), MALP-2 (100 ng/mL), R848 (5 μM), soluble immune complexes (SIC, 10%), insoluble immune complexes (IIC, 10%) RA synovial fluid (SF, 10%), or autologous serum (10%). Immune complexes were prepared as previously described (68). Cells were incubated for a further 4 h to allow for NET production. Cells adhered to coverslips were fixed with 4% paraformaldehyde prior to immunofluorescent staining. Briefly, coverslips were removed from the plate and washed with PBS, permeabilised with 0.05% Tween 20 in TBS, fixed with TBS (2% BSA), and then stained for 30 min on drops of TBS (2% BSA) on parafilm stretched across a clean 24-well plate. Primary antibodies used were: rabbit anti-neutrophil elastase (1:200), mouse anti-myeloperoxidase (1:1,000), rabbit anti-citrullinated histone H3 (1:250), rabbit anti-CRISP3 (1:100), rabbit anti-thymidine phosphorylase (1:200), rabbit anti-histone H2B (1:100), mouse anti-PADI4 (1:100), goat anti-MMP8 (1:15), mouse anti-CAMP(LL37) (1:10), mouse anti-LCN1 (1:200), and mouse anti-histone H1.0 (1:200). Coverslips were washed three times with TBS prior to secondary antibody staining (anti-rabbit AlexaFluor488, 1:2,000, anti-mouse AlexaFluor647, 1:2,000, or anti-goat AlexaFluor647, 1:2,000) in TBS (+2% BSA) for 30 min. Coverslips were washed prior to staining with DAPI (1 μg/mL) for 3 min. Coverslips were washed a further 3 times and mounted onto glass slides using Mowiol 4–88. Slides were imaged on either an Epifluorescent microscope (Zeiss) or a confocal laser-scanning microscope (Leica DM2500) using a 20X, 40X, or 100X objective.
Images were analyzed using Fiji (69) with equal color balance. The DAPI channel of one image from each condition was used to train a machine learning pixel classifier in Ilastik v1.3.0 (70) to recognize three categories: background, compact nuclei, and NETs. Subsequently, all images in the dataset were processed to produce a simple segmentation count mask output. A Fiji (69) script was used to measure the area occupied by each label (available at https://bitbucket.org/snippets/davemason/5edXBB).
Two assays were used to measure the release of DNA during NETosis. In parallel experiments to those described above, neutrophils were seeded (5 × 105 cells/500 μL) in RPMI media plus 2% AB serum in a 24-well plate. Cells were allowed to adhere for 1 h prior to stimulation with PMA (50 nM), A23187 (3.8 μM), or vehicle control (DMSO). Cells were incubated for a further 4 h to allow for NET production. Following a total of 5 h incubation, 5 μM CaCl2 was added to culture supernatant followed by 500mU micrococcal nuclease and incubated for 10 min at 37°C. The nuclease reaction was stopped by the addition of 5 μL EDTA (0.5 M). Culture supernatants were removed from each well, centrifuged at 200 g for 5 min to remove cellular debris, and decanted into clean tubes prior to freezing at −80°C. DNA content of each supernatant was measured using the Quantifluor dsDNA kit in black 96-well plates using serially diluted lambda DNA as a calibration standard (0–2,000 ng/mL). Measurement was carried out at 485 nm excitation/535 nm emission on a Tecan plate reader. In addition, purified neutrophils (2 × 105 cells/200 μL) were seeded in 96-well black plates in the presence of 5 μM SYTOX Green Nucleic Acid Stain, a non-cell-permeable DNA binding dye. Cells were then stimulated as described above and incubated at 37°C. Plates were read every 30 min for 4 h on a Tecan plate reader. SYTOX green excites at 480/490 nm and emits at 520 nm [adapted from (16)].
Neutrophils were plated at 1.7 × 106 cells/mL in RPMI without phenol red, and supplemented with L-glutamine, 25 mM HEPES plus 2% AB serum in 12-well plates at 37°C in a 5% CO2 incubator. Cells were allowed to settle for 1 h, stimulated as described above and incubated for a further 4 h. After 4 h, the supernatant was removed and the cells were washed twice for 10 min with RPMI media (with no serum). Finally 1 mL RPMI media containing 1 μM CaCl2 and 50 mU micrococcal nuclease was added and incubated for 20 min to digest NET DNA. Five micromoles EDTA was used to stop the reaction. Digested NET material was removed by gently tilting the plate and removing the supernatant into a 1.5 mL Eppendorf tube. The supernatant was centrifuged at 400 g for 5 min at 4°C, carefully transferred to a clean tube and the centrifuged at 16,000 g for 5 min at 4°C. We have previously used protein absorption onto Strataclean beads to concentrate samples prior to proteomics (71). The supernatant fraction was carefully transferred to a clean tube, 10 μL Strataclean beads were added and the suspension was vortexed for 1 min at room temperature. The beads were then washed twice in 1 mL ice cold-PBS, by centrifuging at 2,000 g for 2 min at 4°C, before freezing at −80°C for later proteomics analysis. This protocol was developed and adapted from previous studies (62, 63).
The beads were re-suspended in 80 μL of 25 mM ammonium bicarbonate (ambic) and 5 μL of 1%(w/v) Rapigest (Waters) in 25 mM ambic, and the samples heated at 80°C for 10 min with shaking. Samples were reduced by the addition of 5 μL of 60 mM DTT in 25 mM ambic and heated at 60°C for 10 min. Samples were cooled and 5 μL of 180 mM iodoacetamide in 25 mM ambic was added, and samples incubated at room temp for 30 min in the dark. Trypsin (Promega Gold sequencing grade) (1 μg) was added and the samples incubated at 37°C overnight on a rotary mixer. The following day the digests were acidified (to remove Rapigest surfactant) by the addition of 1%(v/v) TFA (acidity checked using pH paper) and incubated at 37°C for 45 min. Samples were then centrifuged at 17,000 g for 30 min and the clarified supernatants transferred to 0.5 mL low-bind tubes. Samples were centrifuged for a further 30 min and 10 μL transferred to total recovery vials for LC-HRMS analysis.
For visualization of NET proteins by SDS-PAGE, Strataclean beads were first boiled for 5 min in 25 μL Laemmli buffer to liberate proteins. The samples were briefly centrifuged to sediment the Strataclean beads before loading the total volume of sample on a 10% SDS-PAGE and running at 100 V for 1 h. Gels were stained with PhastGel® Blue. One RA PMA sample was found to contain insufficient protein and was excluded from the analysis.
Data-dependent LC-MS/MS analyses were conducted on a QExactive HF quadrupole-Orbitrap mass spectrometer coupled to a Dionex Ultimate 3000 RSLC nano-liquid chromatograph (Hemel Hempstead, UK). Sample digest (4–8 μL) was loaded onto a trapping column (Acclaim PepMap 100 C18, 75 μm × 2 cm, 3 μm packing material, 100 Å) using a loading buffer of 0.1% (v/v) TFA, 2% (v/v) acetonitrile in water for 7 min at a flow rate of 9 μL/min. The trapping column was then set in-line with an analytical column (EASY-Spray PepMap RSLC C18, 75 μm × 50 cm, 2 μm packing material, 100 Å) and the peptides eluted using a linear gradient of 96.2% A (0.1% [v/v] formic acid):3.8% B [0.1% [v/v] formic acid in water:acetonitrile [80:20]] to 50% A:50% B over 30 min at a flow rate of 300 nL/min, followed by washing at 1% A:99% B for 5 min and re-equilibration of the column to starting conditions. The column was maintained at 40°C, and the effluent introduced directly into the integrated nano-electrospray ionization source operating in positive ion mode. The mass spectrometer was operated in DDA mode with survey scans between m/z 350–2,000 acquired at a mass resolution of 60,000 (FWHM) at m/z 200. The maximum injection time was 100 ms, and the automatic gain control was set to 3e6. The 10 most intense precursor ions with charges states of between 2+ and 5+ were selected for MS/MS with an isolation window of 2 m/z units. The maximum injection time was 100 ms, and the automatic gain control was set to 1e5. Fragmentation of the peptides was by higher-energy collisional dissociation using a normalized collision energy of 29%. Dynamic exclusion of m/z values to prevent repeated fragmentation of the same peptide was used with an exclusion time of 20 s.
Acquired MS data was searched in Mascot (v2.6.2, Matrix Science, London, UK) against the reviewed entries of the reference proteome set of H. sapiens from Uniprot (20,328 sequences) using a peptide mass tolerance of 10 ppm and a fragment ion tolerance of 0.01Da. Carbamidomethyl cysteine and oxidation of methionine were selected as fixed and variable modifications, respectively. Additional searches were conducted using PEAKs (v8.5, Bioinformatics Solutions Inc., Waterloo, Ontario, Canada) (72) to identify protein citrullination, acetylation, and methylation, which in addition to methionine oxidation were specified as variable modifications. A precursor mass tolerance of 10 ppm and a fragment ion mass tolerance of 0.01 Da was used. A peptide false discovery rate of 1% was set. Label-free protein quantification was performed using Progenesis QI for Proteomics v.2.0 (Waters Ltd., Newcastle-upon-Tyne, UK). Samples were automatically aligned according to retention time. Default peak picking parameters were applied and peptides with charges between 2+ and 7+ were retained. Database searching was performed using Mascot. A Mascot Generic File, created by Progenesis, was searched against the UniProt human reviewed database with modifications and mass tolerances as specified above. Search results were filtered to obtain a peptide false discovery rate of 1% before importing into back into Progenesis. Protein quantification was based on averaging the individual abundances for every unique peptide for each protein and comparing the relative abundance across sample runs and between experimental groups. The mass spectrometry proteomics data have been deposited to the ProteomeXchange Consortium via the PRIDE (73) partner repository with the dataset identifiers PXD011796 and doi: 10.6019/PXD011796.
In addition to analyzing the NET proteins using this methodology, we also measured the proteins present in a cell-free protein preparation (n = 4) from RPMI plus 2% AB serum to identify those proteins present in the culture media (n = 243 proteins, Supplementary Table 1). Any proteins detected in the NET samples that corresponded with this list were excluded from our analyses.
Experimental data were tested for normality using the Kolmogorov-Smirnov test, and then analyzed using Student's t-test or Mann-Whitney U-test as appropriate in GraphPad Prism (version 4). Proteomics data was analyzed using ANOVA in Progenesis and Pearson's correlation in R. Bespoke R scripts were used to carry out principal component analysis. Heatmaps were produced using MeV (v4) (74). Gene Ontology analysis was carried out using DAVID (v6.8) (75, 76) and is reported as a Benjamini-adjusted p-value (False Discovery Rate, FDR).
First we optimized our neutrophil culture conditions, as previous studies have demonstrated that NETosis is highly sensitive to pH and CO2 concentration (77), as well as serum concentration (15, 22, 78). In particular we wished to minimize the amount of serum used in our incubations, to prevent excessive carry-through of serum proteins into the proteomics analysis pipeline. Several recent studies (8, 21, 62, 63) have excluded serum from NETosis experiments; however we found that serum-free media caused high levels of cell death after 5 h (75.3 ± 10.3%, n = 3) and, in particular PI positive, necrotic cells (14.0 ± 5.8%, n = 3) in healthy, untreated neutrophils. Titration of serum in culture medium (0–2%) significantly decreased the amount of cell death after 5 h incubation (Figure 1A, 2% serum, 19.5 ± 4.5%, p < 0.01). The effect of serum supplementation on NETosis was confirmed in RA and SLE neutrophils using the SYTOX Green assay. Serum-free media increased the amount of DNA released by neutrophils over 4.5 h (Figures 1B,C). This was markedly increased in RA neutrophils, where the absence of serum caused a 2-fold increase in DNA release by 4.5 h (Figure 1C, n = 6, p < 0.05). These results mirrored the visible production of NETs in the absence or presence of up to 2% serum (Supplementary Figure 1). Based on these data we decided to use 2% AB serum in our experiments.
Figure 1. Optimization of incubation conditions for NET proteomics. (A) Titration of human AB serum (0–2%) in culture media significantly inhibited cell death detected by annexin V/PI in healthy neutrophils (n = 3 *p < 0.05, **p < 0.01). Supplementation of 2% AB inhibited externalization of DNA detected by SYTOX green assay in SLE (B) and RA (C) neutrophils (n = 6, *p < 0.05). (D) Optimization of wash steps to remove media containing serum proteins from NET preparations prior to proteomic analysis. (E) SDS-PAGE gel of NET proteins prepared from three healthy donors in response to PMA (50 nM) and A23187 (3.8 μM). (F) Detection of DNA in culture media of neutrophils treated with A23187, PMA, or DMSO (vehicle control). Significantly more NETosis was observed in response to PMA (n = 3, **p < 0.01). This was confirmed by immunofluorescent staining for DNA (DAPI,blue) and myeloperoxidase (red). Images taken on an Epifluorescent microscope (Zeiss) at X20 (G) and X100 (H) magnification.
Our next step was to minimize the carry-through of serum proteins to our proteomics pipeline. We found that inclusion of two gentle, 10 min wash steps significantly removed the amount of protein visible on a PhastGel® Blue stained SDS-PAGE gels (Figure 1D). We also titrated the concentration of micrococcal nuclease used during NET digestion, determining that 50 mU was sufficient to liberate NETs, as shown by the Quantifluor assay, Coomassie gel and immuno-fluorescent staining (Supplementary Figure 2). We observed differences in the quantity of NET proteins produced in response to PMA or A23187 by SDS-PAGE gel analysis (Figure 1E). These observations matched both measurements of NET DNA in culture supernatants, and immunofluorescent staining of NETs, prepared in parallel experiments (Figures 1F,G). Not only did A23187 produce fewer NETs, but also the NETs produced in response to A23187 and PMA were visually very different (Figures 1G,H). Whilst PMA-induced NETs tended to emanate from a neutrophil which had swollen to almost double its size before bursting, A23187-induced NETs appeared to emanate from cells which had condensed and ejected their DNA, while still partially retaining their nuclear structure (Figures 1G,H).
We validated the quantification of externalized DNA in culture supernatants by analyzing the immunofluorescent images. Characterization of the NET phenotype is a non-trivial problem given the high dynamic range of intensities involved and subtle changes in intensity distribution that occur during the process. To alleviate these issues, we turned to machine learning to more robustly characterize condensed nuclei and NETs (70). After training the model on a subset of the data, all images in the dataset were processed to produce a simple segmentation count mask output (Figures 2A,B). Quantification of the % NETs in each image using the Illastik pixel classifier (Figure 2C) showed good correlation with the picogreen assay of micrococcal nuclease-digested supernatants from parallel experiments with the same donor neutrophils (Figure 1F). Interestingly, when we measured NET material produced in response to A23187 using the picogreen assay (Figure 1F), this was not significantly different from untreated neutrophils despite visible NETs on coverslips (Figure 1G). However, NET production in response to A23187 was significant based on the immunofluorescent analysis (Figure 2C, p < 0.05).
Figure 2. Quantitative analysis of NET images using Ilastik. (A) Representative image of DAPI stained neutrophils stimulated with PMA, is shown with contrast enhanced to ~50% saturation to more clearly show the NETs. Image taken on an Epifluorescent microscope (Zeiss). (B) Ilastik machine learning pixel classifier recognizes three categories: background (black), compact nuclei (purple) and NETs (orange). (C) Quantification of NETs from healthy control neutrophils in response to PMA (50 nM) and A23187 (3.8 μM) using Ilastik pixel classifier (n = 3, *p < 0.05, **p < 0.01, Student's t-test).
Proteomics analysis of NETs produced by healthy control neutrophils in response to PMA (50 nM) and A23187 (3.8 μM) identified a total of 272 proteins with a minimum of 1 peptide. Filtering results to include proteins with n ≥ 2 peptides decreased this number to 197 (Supplementary Table 2). Of these, 97 proteins were present at significantly different amounts between the two conditions; 20 were higher in PMA-stimulated NETs and 77 were higher in A23187-stimulated NETs (Figures 3A,B, Supplementary Table 3, FDR < 0.05, fold-change ≥2, minimum 2 peptides). Gene Ontology analysis revealed that NET proteins externalized in response to PMA were associated with biological processes including: gene silencing, cellular metabolic processes, cell-cell adhesion and phagocytosis, whereas NET proteins externalized in response to A23187 were associated with glycolysis, cell-cell adhesion, movement of sub-cellular components and the pentose phosphate shunt (Figure 3B, FDR < 0.01). PMA-induced NET proteins were associated with cellular compartments including the nucleosome, exosomes, the membrane, cell-cell adherens junctions and azurophilic granules whereas A23187-induced NET proteins were associated with exosomes, the cytosol, cell-cell adherens junctions, the cytoskeleton, and specific granules (Figure 3B, FDR < 0.01). Histone 1 family proteins (H1.0, H1.4, H1.5) were significantly higher in A23187-induced NETs, whereas histone H2A and histone H3.1 were higher in PMA-induced NETs (Figure 3C, FDR < 0.05). Analysis of the distribution of neutrophil granule proteins, based on previously published proteomics analysis of sub-cellular neutrophil fractionations (79), revealed differences in types of granule proteins externalized on NETs, with azurophilic granule proteins cathepsin G (CTSG), neutrophil elastase (ELANE), and azurocidin (AZU1/CAP7) higher in PMA-induced NETs, and specific granule proteins cysteine-rich secretory protein 3 (CRISP3), cathelicidin anti-microbial peptide (CAMP/LL37), matrix metalloproteinases MMP8 and MMP9 higher in A23187-induced NETs (Figure 3C, FDR < 0.05). NETs stimulated by A23187 were also enriched with proteins from ficolin-1-rich granules (FG) and secretory vesicles (SV) (Figure 3D). Protein-arginine deiminase 4 (PADI4) was significantly higher in A23187-stimulated NETs (FDR = 0.012).
Figure 3. Analysis of NET proteins released in response to PMA and A23187 by healthy control neutrophils. Analysis of proteins significantly different (FDR < 0.05) between PMA- and A23187-induced NETs using (A) hierarchical clustering and (B) gene ontology. (C) Proteins significantly elevated in PMA-induced NETs included histone H3.1, ELANE, AZU1 ANXA11, whereas in A23187-induced NETs histone H1.5, CRISP3, CAMP, and MMP8 were significantly elevated (*FDR < 0.05, **FDR < 0.01). Y-axis represents normalized protein abundance. (D) Analysis of the distribution of neutrophil granule proteins revealed differences between the source of NET proteins in response to PMA and A23187 (AG, azurophilic granule; SG, specific granule; GG, gelatinase granule; FG, ficolin-1-rich granule; SV, secretory vesicles). Shading in (A) relates to log2 protein abundance: blue, low; black, median; red, high.
In order to determine whether more physiologically-relevant agonists could induce NET production in neutrophils from patients with inflammatory disease, we screened a range of agonists [including TLR- and FcγR-agonists and RA synovial fluid (SF)] using RA neutrophils (n = 3). We found that none of the TLR or FcγR agonists screened (LPS, MALP-2, R848, SIC, IIC) induced significant release of NET DNA into culture supernatants measured by micrococcal nuclease assay (Figure 4A), and whilst some MPO-positive NETs were visible by immunofluorescence in response to MALP-2 and R848, these were not statistically significant compared to untreated NET production when analyzed by our Ilastik algorithm (Figures 4B,C). RA SF induced the release of NETs, although this was highly donor dependent both in terms of donor SF (n = 3) and donor neutrophils (Figure 4A, p < 0.01). TNFα and autologous serum did not induce NETs in RA neutrophils. We did not detect significant levels of spontaneous NETosis in RA neutrophils (Figure 4C), in line with our previous observations (14). The most consistent stimulators of NETs in RA neutrophils were PMA and A23187, which produced significant amounts of NETs in all donors (Figure 4A, PMA 479.9 ± 19.6 ng, A23187 478.0 ± 44.8 ng, p < 0.01). NET production in response to A23187 was higher in RA patients compared to healthy controls (Figures 1F, 4A, p < 0.01).
Figure 4. Screening of NET agonists in neutrophils from patients with rheumatoid arthritis (RA). RA neutrophils (n = 3) were stimulated with PMA, A23187, TNF, LPS, MALP-2, R848, SIC, IIC, autologous serum (2%, AUTO-S), or RA synovial fluid (SF, n = 3 donors). NET production was measured using (A) quantification of DNA in culture supernatant following micrococcal nuclease digestion of DNA, and (B) quantification of DNA staining (DAPI) using Illastik machine-learning algorithm (*p < 0.05, **p < 0.01 compared to untreated). (C) Representative immunofluorescent images from n = 3 experiments. Images taken on an Epifluorescent microscope (Zeiss) at X20.
For our proteomics experiments using RA and SLE neutrophils (n = 6 each), we decided to induce NET production using PMA and A23187, as both these agonists produced consistent NETosis in every healthy and patient donor tested. We used ultra-pure neutrophils in all our experiments; this was confirmed by flow cytometric analysis of CD16+ cells and morphological assessment of cytospins (Supplementary Figure 3). We noted that CD16 staining was lower on some SLE neutrophils, with 3/6 patients having two populations of neutrophils: CD16bright and CD16dim. We did not observe any eosinophil contamination in these ultra-pure neutrophil preparations and believe the lower CD16 levels on SLE neutrophils may be an early marker of increased apoptosis, in line with previous reports (80).
In parallel experiments, we measured ROS production in response to PMA and A23187 (Figures 5A–C). Whilst PMA and A23187 induced significant production of ROS over 30 min by SLE neutrophils (Figure 5A, *p < 0.05, **p < 0.01) the increased levels of ROS produced by RA neutrophils did not reach statistical significance compared to untreated neutrophils (PMA p = 0.06, A23187 p = 0.31). We additionally noted that ROS production by RA neutrophils peaked around 1.5–2 min sooner than SLE neutrophils in response to both agonists (Figures 5B,C). This suggests that RA neutrophils have been “primed” and released ROS in vivo prior to isolation, in line with previous observations (33, 81). We measured the kinetics of NET production by RA and SLE neutrophils in response to PMA and A23187 using the SYTOX green assay. Significant release of NET DNA was observed by 2.5 h with both agonists (Figures 5D,E, *p < 0.05, **p < 0.01). We noted that A23187 induced higher levels of DNA release by SLE neutrophils compared to RA neutrophils, although this did not reach statistical significance (4 h p = 0.07). NET production in response to A23187 also occurred faster and was significant by 2 h in SLE neutrophils (p = 0.04) but not RA neutrophils (p = 0.09). We also noted that the absence or presence of serum greatly affected the amount of NET material produced in response to A23187, but not PMA. The amount of NET material produced in response to A23187 was lower in the presence of 2% serum, likely due to the presence of calcium-binding proteins and/or anti-oxidants in the serum (Supplementary Figure 4).
Figure 5. Production of ROS and externalization of DNA by RA and SLE neutrophils in response to PMA and A23187. (A) Total ROS production (area under the curve) over 30 min measured by luminol-enhanced chemiluminescence (n = 6 each RA and SLE, *p < 0.05, **p < 0.01). Representative traces are shown for RA neutrophils (B) and SLE neutrophils (C) (AU = arbitrary units). Externalization of DNA measured by SYTOX green assay by RA (D) and SLE (E) neutrophils (n = 6, *p < 0.05, **p < 0.01).
Quantitative proteomics analysis of RA and SLE NETs produced in response to PMA and A23187 identified 480 proteins (Figure 6A, n ≥ 1 peptide, Supplementary Table 4). Filtering results to include proteins with n ≥ 2 peptides decreased this number to 314 (Figure 6B). The number of proteins significantly different between the four experimental conditions (ANOVA p < 0.05) is shown in Figures 6A,B and detailed in Supplementary Table 5. Principal component analysis (PCA) of these proteins separated the samples based on both disease diagnosis and NET agonist, with tight clustering of biological replicates (Figures 6A,B). A number of interesting observations were made in relation to the proteins that were significantly different between the four treatments. Firstly, the identities of the proteins significantly different between PMA and A23187 treatments were similar, independent of whether RA or SLE neutrophils were stimulated, and echoed our observations in healthy control NETs. In general, PMA-induced NETs were decorated with proteins of the annexin family (ANXA1, ANXA4, ANXA5, ANXA6, ANXA7, ANXA11), azurocidin (AZU1/CAP7), and histone H3, whereas A23187-induced NETs were decorated with granule proteins such as CAMP/LL37, CRISP3, lipocalin (LCN2) and MMP8, histones H1.0, H1.4 and H1.5, interleukin-8 (CXCL8), PADI4, and α-enolase (ENO1).
Figure 6. Analysis of NET proteins produced by RA and SLE neutrophils in response to PMA and A23187. Venn diagrams show number of proteins above and below the FDR = 0.05 cut-off for each comparison. Principal component analysis (PCA) shows separation of samples based on significant proteins. Data are shown before (A) and after (B) filtering for n ≥ 2 peptides.
Secondly, a small number of significant differences in NET proteins were identified. When we compared NETs from RA and SLE patient neutrophils stimulated with PMA, four NET proteins were significantly different (Figure 6B, Supplementary Table 5). Non-secretory ribonuclease (RNASE2) was higher in RA neutrophils, whereas MPO, leukocyte elastase inhibitor (SERPINB1) and thymidine phosphorylase (TYMP) were higher in SLE neutrophils. In contrast, when we compared NETs from RA and SLE neutrophils stimulated with A23187, six NET proteins were higher in RA NETs, including CAMP, CRISP3, CXCL8, and MMP8, and 13 NET proteins were higher in SLE NETs, including histones H1.0, H2B (type 1-J), H2B (type 2-F), and H4 (Supplementary Table 5). The presence of a number of these differently expressed NET proteins was confirmed using immunofluorescent staining of NETs prepared and fixed on coverslips in parallel experiments from the same donors. Figure 7 shows the proteomics data (Figure 7A) alongside representative images of antibody-labeled NETs (Figure 7B). The abundance of SERPIN1B on PMA-induced NETs from RA patients correlated with RA disease activity (DAS28) scores (R2 = 0.84, p = 0.029). DAS28 also correlated significantly with phosphoglycerate kinase-1 (PGK1, R2 = 0.74, p = 0.028) and histone H1 family member 0 (H1F0, R2 = 0.68, p = 0.044) in A23187-induced RA NETs.
Figure 7. Immunofluorescent staining of NET proteins in RA and SLE NETs produced in response to A23187 or PMA. (A) Proteomics data is shown as box-and-whisker plots alongside (B) representative immunofluorescent images. Box-and-whisker plots also indicate the median (horizontal line) with dots representing each individual NET proteomics sample. DNA is stained with DAPI (blue), proteins are stained with antibodies as indicated on the figure (LL37/Cathelicidin, LCN2/Lipocalin, MMP8, Histone H1.0, PAD4 stained with AlexaFluor 647 and shown in red; CRISP3, Thymidine phosphorylase, Histone H2B stained with AlexaFluor 488 and shown in green). Images taken on a confocal laser-scanning microscope (Leica DM2500) at X20.
Several studies have reported the presence of post-translational modifications (PTMs), including citrullination, methylation and acetylation, on NET proteins from RA or SLE patients (47, 49, 55, 82). These PTMs may play a key role in the development of auto-immunity, for example the production of auto-antibodies to citrullinated peptides in RA and acetylated histones in SLE. Moreover, serum from RA and SLE patients cross-reacts with NET proteins containing PTMs (47, 83). We carried out analysis of PTMs in our proteomics data using the software PEAKS. Acetylation and methylation modifications were selected using standard PEAKS search terms, and citrullination (not a standard search term) was identified using a missed arginine cleavage together with an increase in MW of 0.98Da (84). All PTMs called by PEAKS were manually inspected for inclusion in the results, for example if a citrullination event was predicted in the C-terminal position of a peptide (i.e., a trypsin cleavage site) this was eliminated as an incorrect identification.
We initially searched for citrullinated peptides in the healthy control samples. PEAKS analysis identified two peptides with citrulline modifications in all three samples of A23187-induced NETs from histone H1.5, H1.3, or H1.4 and glial fibrilary acidic protein (Supplementary Table 6A). Four proteins were identified as having citrulline modifications in all three samples of PMA-induced NETs: azurocidin, neutrophil elastase, myeloid cell nuclear differentiation antigen (MNDA), and histidine decarboxylase (Supplementary Table 6A). Several other citrullinated peptides were identified but were not found to contain citrulline modifications in all samples (Supplementary Table 6A); for example citrullinated histone H3.1t peptide was found in 3/6 samples (two PMA-induced and one A23187-induced sample).
PEAKS identified histone H3 as the only citrullinated peptide all RA and SLE patient samples (Supplementary Table 6B). It is noteworthy that citrullinated histone H3 was present in all RA and SLE samples, but was only present in 3/6 healthy control samples, possibly indicating in vivo citrullination of histone H3 in disease. Eighteen peptides (from 11 proteins) were citrullinated in >50% of NET samples (irrespective of agonist), including MNDA, vimentin (VIM), actin, high mobility group protein B2 (HMGB2), non-histone chromosomal protein HMG-17 (HMGN2), lamin-B receptor (LBR), and histone H1.3/H1.4/H1.5 (Supplementary Table 6B). Nineteen peptides (from 11 proteins) were citrullinated in >50% A23187-induced NETs (6/12 samples), but <50% PMA-induced NETs (5/11 samples) (Supplementary Table 6C). These included histone H1.3/H1.4/H1.5, and HMGB1/2, as well as myosin 9/10/14. Nine peptides (from eight proteins) were citrullinated in >50% PMA-induced NETs, but <50% A23187-induced NET samples (Supplementary Table 6D). These included lactotransferrin and MPO. We did not observe any difference in citrullinated peptides between the RA and SLE samples.
In total, eighteen peptides (from 13 proteins) had acetylation modifications in at least 22/23 samples, including histones 1.0 1.2, 1.3, and 1.5, actin (cytoplasmic 1 or 2), thymosin beta-4 and annexin A1 and A3 (Supplementary Table 6E). Four thymosin beta peptides had the same acetylation site, but different trypsin cleavage profiles or other additional PTMs. Thirty-one peptides were acetylated in >50% A23187-induced NET samples but <50% PMA-induced NET samples (Supplementary Table 6F). Many of these were from histones, in particular histone H3 isoforms. Eleven peptides were acetylated in >50% PMA-induced NETs but <50% A23187-induced NETs (Supplementary Table 6G). Of note, we detected acetylated peptides from histone H2B and histone H4 in A23187-induced NETs but not in PMA-induced NETs. This could be because the acetylated tails of histone H2B are cleaved during NOX-dependent (i.e., PMA-induced) NETosis, as has been reported previously (55).
Methylation events were identified in three peptides (from two proteins) in at least 22/23 samples: actin (cytoplasmic 1 or 2) and histone H3.2 (Supplementary Table 6H). Nine methylated peptides were present in >50% A23187 samples and <50% PMA samples (Supplementary Table 6I) and four methylated peptides were present in >50% PMA samples and <50% A23187 samples (Supplementary Table 6J). The majority of the former were peptides from histone H3.1, H3.2, and H3.3.
Several proteins, in particular members of the histone family (histones H1.3/H1.4/H1.5, H2B, H3.1, and H3.3), had multiple PTMs including citrullinated, acetylated and methylated protein residues. Modifications of the N-terminal tails of histones detected in our study are summarized in Figure 8A. Immunofluorescent staining of citrullinated histone H3, and the presence of PADI4 on A23187-induced NETs, is shown in Figure 8B. A full list of all peptides with PTMs identified across all RA and SLE samples can be found in Supplementary Table 7. Example spectra from peptides with citrullination, acetylation and methylation modifications can be found in Supplementary Figures 5–7, respectively.
Figure 8. Post-translational modification of histones in NETs. (A) Summary of the post-translational modifications to the N-terminal tails of histones H1, H2A, H2B, H3, and H4 in RA and SLE neutrophil NETs (C, citrullination; A, acetylation; M, methylation; D, dimethylation). (B) Representative immunofluorescent staining of DNA (blue), citrullinated histone H3 (green) and PADI4 (red) in PMA- and A23187-induced NETs. Images taken on an Epifluorescent microscope (Zeiss).
In this study we have applied quantitative proteomics to investigate the protein content of NETs generated by healthy control, RA and SLE neutrophils in response to PMA (NOX2-dependent NETosis) and A23187 (NOX2-independent NETosis). We have identified significant differences in NET proteins released in response to these two agonists. We have also directly compared NETs from RA and SLE neutrophils, and show there are only a small number of proteins that are significantly different. In addition we have described a number of peptides containing citrullination, acetylation and/or methylation PTMs.
As part of this study we screened a wide-range of agonists, including TLR and FcγR agonists, for their ability to induce NETosis in RA neutrophils. Of note, LPS did not induce NETosis in our experimental system. This may be due to our use of ultra-pure neutrophils [important for the proteomics aspect of this study (85)], as LPS-induced NETosis has been shown to be critically dependent upon the presence of platelets (86, 87). We also noted lower production of NETs in response to A23187 than reported in other studies (16, 17), likely due to our inclusion of serum (containing calcium-binding proteins and anti-oxidants). In general we found that SLE neutrophils were more responsive to A23187-stimulation, both in terms of ROS production and NET release. We believe there could be two possible explanations for this. Firstly, RA peripheral blood neutrophils are primed in vivo and thus may have released ROS prior to isolation (33, 81). Secondly, the SLE patients in our study were significantly younger than the RA patients. Several neutrophil functions, including the production of ROS and NETosis, decline with age (88).
More than 100 different auto-antibodies have been identified in SLE (89). Antibodies against histones are particularly common, including auto-antibodies against histone H1, H2A, H2B, H2A-H2B, H3, and H4 (90–95). HMG-17 auto-antibodies correlate with both SLE disease activity and anti-dsDNA auto-antibody titres (96). Auto-antibodies that recognize catalase (97), lamin B1 and B2 (98–101), apolipoprotein A1 (102), annexin AI and α-enolase (103–105) have also been described in SLE, with annexin AI and α-enolase auto-antibodies being associated with skin and kidney involvement (104, 105). All of the aforementioned proteins were identified in SLE NETs our study.
MMP9 in SLE LDG NETs impairs aortic endothelium-dependent vasorelaxation and induces endothelial cell apoptosis (59). Cathelicidin (CAMP/LL37) has a strong association with the onset of SLE (106) and is elevated in both serum and skin of SLE patients (107, 108). However, CAMP/LL37 auto-antibody titres do not correlate with SLE disease activity (108). Interestingly we observed higher levels of CAMP/LL37 in RA NETs than we did in SLE NETs.
Many of the NET proteins identified in our analysis are elevated in RA synovial fluid, including cathepsins, MPO, MMP8, MMP9, LCN2, and PADI2 (109–113). These proteins contribute to cellular infiltration and degradation of collagen within synovial joints (114). Elevated serum MMP8 is a strong predictor of mortality in RA (115). RNASE2, elevated in RA NETs, is a potential gene biomarker of TNFi-refractory RA (67).
Patients with RA and Felty's syndrome (a complication of RA) also have auto-antibodies to histones (116, 117), with auto-antibodies in sera from patients with Felty's syndrome cross-reacting with citrullinated histones bound to NETs (49). RA sera, particularly those with high ACPA titres, stimulate NETosis and cross-react with citrullinated histone H4 derived from neutrophil NETs (50). Citrullinated histone H4 was previously identified in NETs (50), although we did not detect this in our study. B cell clones isolated from RA synovial tissue produce antibodies which strongly react with citrullinated forms of histones H2A/H2B, fibrinogen and vimentin (47). These antibodies also cross-react with NETs produced in vitro in response to PMA (47). Citrullinated histone H2B is arthrogenic in a mouse model of inflammatory arthritis (118) and we identified two citrullinated H2B peptides in some (but not all) RA patient samples.
Major findings in our proteomics data were (i) the significant differences in the externalization of histone family proteins in response to PMA and A23187, (ii) the differences in histone externalization between RA and SLE NETs, and (iii) the range of PTMs on histones. In general, we found that the core histones (H2A, H2B, H3, and H4) were expressed higher on PMA-induced NETs, whereas the linker histones (H1.0, H1.4, and H1.5) were expressed higher on A23187-induced NETs. We also observed fewer histone PTMs in response to PMA than A23187. Both these phenomenon are likely due to the activity of elastase which both rapidly degrades the linker histones (23) and cleaves the N-terminus of core histones during NOX2-dependent NETosis (55).
Histones H2B and H4 are major auto-antigens in SLE (119, 120), and interestingly we found these were significantly higher in A23187-induced NETs from SLE patients. Auto-antibodies against a number of histone PTMs have been found in SLE (8, 48, 120, 121). For example, acetylated histone H2B (K12/K20), citrullinated histone H3 and acetylated histone H4 are recognized by SLE serum auto-antibodies (8, 48), although the PTMs identified in our study were on different lysine residues from this earlier study. We did however, detect methylation of histone H3 lysine-27 (H3K27), a known auto-antigen in SLE (121).
Histone acetylation has been suggested to enhance the immuno-stimulatory potential of NETs in SLE (122). A recent study showed that histones from unstimulated SLE neutrophils are hypoacetylated and hypomethylated when compared to neutrophils from healthy donors. However, NETs from SLE patients were found to contain higher amounts of acetylated histone H4-K8, K12, K16, acetylated histone H2B-K12 and tri-methylated histone H3-K27 (123). Our study identified histone H4 acetylated at K8, K12, and K16. We also found acetylation of histone H4K5, which had previously been identified in NETs generated in response to H2O2 (8).
A number of proteins identified on NETs in our analysis contained one or more sites of citrullination. Again a high proportion of these proteins were histones (both core and linker histones), as well as actin, vimentin, MNDA, and HMGB2. Many citrullinated host proteins act as sources of autoantigens in RA; for example citrulline residues in aggrecan and vimentin are preferentially recognized by antigen-presenting cells in individuals carrying the HLA-DRB1*04:01/04 allele (which contains the “shared susceptibility epitope”) (124). A combination of the presence of ACPA auto-antibodies and the presence of the HLA-DRB1*04:01/04 allele is strongly associated with the development of RA (125). Citrullinated vimentin is the antigenic target of anti-Sa auto-antibodies, and is present in the sera and synovial tissue of RA patients (126). NETs containing citrullinated peptides are internalized by synovial fibroblasts via a RAGE-TLR9 pathway, and then presented via MHC Class II to antigen-specific T-cells (83). HLA-DRB1*04:01 transgenic mice develop auto-antibodies specific to citrullinated forms of NET peptides, including core histones (H2A, H2B, H3), α-enolase and vimentin (83).
A recent study suggested RA NETs produced in response to rheumatoid factor contain citrullinated azurocidin, catalase, histone H2A (type 2C), histone 2B, MPO, elastase, profilaggrin, protein S100-A12, and protein S100-A9 (83). However, many of the citrulline peptides identified in this recent study (83) were reported at the C-terminus of the peptide (trypsin cleavage site). As citrulline does not have the positive charge of arginine, the general assumption is that citrullination will result in a missed cleavage by trypsin (84), and therefore the assignment of these peptides is questionable. Indeed, we excluded any peptides with a reported C-terminal citrulline from our own analysis.
Recent analysis of the RA synovial citrullinome showed wide-spread protein citrullination within RA synovial fluid, and identified a number of citrullinated proteins that correspond with our dataset, such as actin (beta and gamma), cathepsin G, coronin, gelsolin, histone H1.3, histone H3.3, MNDA, MPO, myosin 9, and vimentin (110). Several of the PTMs identified corresponded to the arginine residue detected in our dataset, suggesting that NET products and/or NET-derived PAD enzymes may be responsible for the presence of these modified proteins (127, 128). Citrullination sites on histone H1 family proteins induced by PADI4 activity in response to A23187 have been detailed previously, and correspond to some of the citrulline PTMs identified in our dataset (82).
We detected both PADI4 and PADI2 in our datasets, although PADI2 was not significantly different between treatment conditions. PADI2 is the key enzyme involved in TNFα-induced protein citrullination in mouse models of RA (129). In this model, PADI4 did not induce citrullination of proteins in mouse ankles, but was essential for NET formation, and only PADI2 knockout improved clinical measurements of disease activity (129). RA patients with severe, erosive disease have auto-antibodies to PADI3/PADI4 which can increase the catalytic activity of PADI4 in a forward-feedback loop associated with high levels of disease activity (130). Indeed, PADI4 has emerged as a potential therapeutic target for the treatment of RA and has shown efficacy in some, but not all, PADI4 knock-out models of inflammatory arthritis (129, 131, 132).
It was recently shown that citrullinated histones, particularly citrullinated histone H3, mediate microvascular leakage, and endothelial barrier dysfunction (133). Though it is not entirely clear whether the same results would have been obtained with unmodified histones, this recent work (133) demonstrates the concept that PTM NET products are able to affect the physiology of other cells as well as being major auto-antigens. Citrullination of peptides in NETs may also alter their function, for example CAMP/LL37 can be citrullinated at three or five sites; CAMP/LL37 citrullinated at 5 residues is the most chemotactic to PBMCs and most pro-inflammatory (134).
We believe that our use of a global approach to NET proteomics rather than a hypothesis-driven, targeted approach highlights the fact that, with only a few exceptions, NET proteins are broadly similar irrespective of health or disease, and that the differences lie in the stimulus and mode of NET production (NOX2-dependent or NOX2-independent). Indeed, we believe that previous, hypothesis-driven research may have missed this important point. For example, there are a number of publications on the importance of NET-derived CAMP/LL37 in SLE and on the involvement of PADI4 activity in RA (45, 106–108, 130). However, we have shown that both CAMP/LL37 and PADI4 are presented on both RA and SLE NETs depending upon the agonist used. This raises two possibilities: first, that the stimuli leading to NET production in vivo in the respective diseases are different, or second it is the response of the adaptive immune system to the NET material that is the driver of disease manifestation. Fully understanding the drivers of NETosis in vivo is more important than ever. We agree with many others in the field that there are two different processes that are both often confusingly referred to as NETosis. Several groups are now making the distinction between NOX2-dependent and NOX2-independent NETosis (17, 25, 55). A recent publication even suggested referring to NOX2-independent NETosis as leukotrophic hypercitrullination (135).
Further subtleties in the signaling of physiological stimuli of NOX2-dependent and NOX2-independent NETosis may exist that are not captured by merely using PMA and A23187. We further echo the desire of others (136) to see the field become more standardized in the experimental approaches used decipher NETosis, including more quantitative and high through methodologies. We believe, that we have described both a high throughput proteomic and high throughput imaging technique. Analysis of NET remnants in serum of patients with RA and SLE suggests NETs produced in vivo in both diseases originate via NOX2-independent NETosis (55). Therefore, perhaps PMA is a poor model for future in vitro studies seeking to replicate NETosis in inflammatory disease.
The challenge in the field is to design experiments that recapitulate conditions in vivo e.g., inclusion of platelets, endothelial cells, and other immune cells, the use of biofluids such as sera and synovial fluid as agonists, and the measurement of NET proteins in tissue biopsies. However, this poses significant challenges particularly for proteomics studies, i.e., isolation of neutrophil and NET proteins from mixed-cell populations, and carry-through of proteins from sera and synovial fluid into proteomic analyses.
The importance of NETs and NET proteins has wide-reaching implications in medicine. It was recently shown that NET fragments promote innate immune responses that prevent lung transplant tolerance (137), and that NETs cause dendritic cell maturation and subsequent Th1 cell expansion (138). We must understand NETs further if we wish to suppress these unwanted immune responses. Future studies might also examine whether medication affects the protein contents of NETs, as suggested by a recent study showing clarithromycin increased CAMP/LL37 load on NETs (139).
This work provides the first, direct comparison of NOX2-dependent (PMA) and NOX2-independent (A23187) NETs using quantitative proteomics. We have also undertaken the first direct comparison of NETs from RA and SLE using proteomics, and show that whilst there are a small number of proteins that are significantly different between RA and SLE NETs, for example histone H2B is higher in SLE, it is the nature of the stimulant rather than the underlying neutrophil physiology that determines the NET protein patterns. We additionally identify an extensive range of post-translationally modified proteins in RA and SLE, many of which are known targets of auto-antibodies in each disease. This work provides important insight into how the regulation of NETosis, and exposure of intracellular antigens on NETs, may contribute to the pathophysiology of RA and SLE.
The datasets generated for this study can be found in PRIDE (73), doi: 10.6019/PXD011796.
This study was carried out in accordance with the recommendations the University of Liverpool Committee on Research Ethics (CORE) for healthy controls, and National Research Ethics Service (NRES) Committee Northwest (Greater Manchester West) for RA and SLE patients with written informed consent from all subjects. All subjects gave written informed consent in accordance with the Declaration of Helsinki. The protocol was approved by CORE and NRES.
EC designed the experiments, carried out the experiments, analyzed the data, and wrote the manuscript. ML carried out the experiments and analyzed the data and reviewed the manuscript. DS carried out the proteomics analysis and revised the manuscript. DM designed the image analysis macro, analyzed the data, and revised the manuscript. RB designed the proteomics experiments and revised the manuscript. RM provided patients and clinical advise, and revised the manuscript. HW designed the study, carried out the experiments, analyzed the data, and wrote the manuscript.
This work was funded by a Wellcome Trust Seed Award in Science (200605/Z/16/Z) and the University of Liverpool Technology Directorate. HW was funded by Arthritis Research UK (21430). ML was funded by the University of Liverpool MRes Clinical Sciences Research Support Fund. The Epifluorescent microscope at Liverpool Center for Cell Imaging was funded by MRC grant number MR/K015931/1.
The authors declare that the research was conducted in the absence of any commercial or financial relationships that could be construed as a potential conflict of interest.
We would like to thank the rheumatology nurses and consultants at University Hospital Aintree for their assistance in recruiting patients for this study. We thank Dr. Eva Caamano Gutierrez and Dr. Arturas Grauslys for bespoke R scripts used in statistical analysis.
The Supplementary Material for this article can be found online at: https://www.frontiersin.org/articles/10.3389/fimmu.2019.00423/full#supplementary-material
1. Brinkmann V, Reichard U, Goosmann C, Fauler B, Uhlemann Y, Weiss DS, et al. Neutrophil extracellular traps kill bacteria. Science. (2004) 303:1532–5. doi: 10.1126/science.1092385
2. Urban CF, Reichard U, Brinkmann V, Zychlinsky A. Neutrophil extracellular traps capture and kill Candida albicans yeast and hyphal forms. Cell Microbiol. (2006) 8:668–76. doi: 10.1111/j.1462-5822.2005.00659.x
3. Yipp BG, Petri B, Salina D, Jenne CN, Scott BN, Zbytnuik LD, et al. Infection-induced NETosis is a dynamic process involving neutrophil multitasking in vivo. Nat Med. (2012) 18:1386–93. doi: 10.1038/nm.2847
4. Parker H, Albrett AM, Kettle AJ, Winterbourn CC. Myeloperoxidase associated with neutrophil extracellular traps is active and mediates bacterial killing in the presence of hydrogen peroxide. J Leukoc Biol. (2012) 91:369–76. doi: 10.1189/jlb.0711387
5. Remijsen Q, Kuijpers TW, Wirawan E, Lippens S, Vandenabeele P, Vanden Berghe T. Dying for a cause: NETosis, mechanisms behind an antimicrobial cell death modality. Cell Death Differ. (2011) 18:581–8. doi: 10.1038/cdd.2011.1
6. Brinkmann V, Zychlinsky A. Neutrophil extracellular traps: is immunity the second function of chromatin? J Cell Biol. (2012) 198:773–83. doi: 10.1083/jcb.201203170
7. Lu T, Kobayashi SD, Quinn MT, Deleo FR. A NET Outcome. Front Immunol. (2012) 3:365. doi: 10.3389/fimmu.2012.00365
8. Liu CL, Tangsombatvisit S, Rosenberg JM, Mandelbaum G, Gillespie EC, Gozani OP, et al. Specific post-translational histone modifications of neutrophil extracellular traps as immunogens and potential targets of lupus autoantibodies. Arthritis Res Ther. (2012) 14:R25. doi: 10.1186/ar3707
9. Neeli I, Khan SN, Radic M. Histone deimination as a response to inflammatory stimuli in neutrophils. J Immunol. (2008) 180:1895–902. doi: 10.4049/jimmunol.180.3.1895
10. Wang Y, Li M, Stadler S, Correll S, Li P, Wang D, et al. Histone hypercitrullination mediates chromatin decondensation and neutrophil extracellular trap formation. J Cell Biol. (2009) 184:205–13. doi: 10.1083/jcb.200806072
11. Li P, Li M, Lindberg MR, Kennett MJ, Xiong N, Wang Y. PAD4 is essential for antibacterial innate immunity mediated by neutrophil extracellular traps. J Exp Med. (2010) 207:1853–62. doi: 10.1084/jem.20100239
12. Hemmers S, Teijaro JR, Arandjelovic S, Mowen KA. PAD4-mediated neutrophil extracellular trap formation is not required for immunity against influenza infection. PLoS ONE. (2011) 6:e22043. doi: 10.1371/journal.pone.0022043
13. Hakkim A, Fuchs TA, Martinez NE, Hess S, Prinz H, Zychlinsky A, et al. Activation of the Raf-MEK-ERK pathway is required for neutrophil extracellular trap formation. Nat Chem Biol. (2011) 7:75–7. doi: 10.1038/nchembio.496
14. Wright HL, Makki FA, Moots RJ, Edwards SW. Low-density granulocytes: functionally distinct, immature neutrophils in rheumatoid arthritis with altered properties and defective TNF signalling. J Leukoc Biol. (2017) 101:599–611. doi: 10.1189/jlb.5A0116-022R
15. Fuchs TA, Abed U, Goosmann C, Hurwitz R, Schulze I, Wahn V, et al. Novel cell death program leads to neutrophil extracellular traps. J Cell Biol. (2007) 176:231–41. doi: 10.1083/jcb.200606027
16. Barrientos L, Marin-Esteban V, de Chaisemartin L, Le-Moal VL, Sandre C, Bianchini E, et al. An improved strategy to recover large fragments of functional human neutrophil extracellular traps. Front Immunol. (2013) 4:166. doi: 10.3389/fimmu.2013.00166
17. Douda DN, Khan MA, Grasemann H, Palaniyar N. SK3 channel and mitochondrial ROS mediate NADPH oxidase-independent NETosis induced by calcium influx. Proc Natl Acad Sci USA. (2015) 112:2817–22. doi: 10.1073/pnas.1414055112
18. Rohrbach AS, Slade DJ, Thompson PR, Mowen KA. Activation of PAD4 in NET formation. Front Immunol. (2012) 3:360. doi: 10.3389/fimmu.2012.00360
19. Clark SR, Ma AC, Tavener SA, McDonald B, Goodarzi Z, Kelly MM, et al. Platelet TLR4 activates neutrophil extracellular traps to ensnare bacteria in septic blood. Nat Med. (2007) 13:463–9. doi: 10.1038/nm1565
20. Lim MB, Kuiper JW, Katchky A, Goldberg H, Glogauer M. Rac2 is required for the formation of neutrophil extracellular traps. J Leukoc Biol. (2011) 90:771–6. doi: 10.1189/jlb.1010549
21. Khandpur R, Carmona-Rivera C, Vivekanandan-Giri A, Gizinski A, Yalavarthi S, Knight JS, et al. NETs are a source of citrullinated autoantigens and stimulate inflammatory responses in rheumatoid arthritis. Sci Transl Med. (2013) 5:178ra140. doi: 10.1126/scitranslmed.3005580
22. Marcos V, Zhou Z, Yildirim AO, Bohla A, Hector A, Vitkov L, et al. Retraction: CXCR2 mediates NADPH oxidase-independent neutrophil extracellular trap formation in cystic fibrosis airway inflammation. Nat Med. (2011) 17:899. doi: 10.1038/nm0711-899a
23. Papayannopoulos V, Metzler KD, Hakkim A, Zychlinsky A. Neutrophil elastase and myeloperoxidase regulate the formation of neutrophil extracellular traps. J Cell Biol. (2010) 191:677–91. doi: 10.1083/jcb.201006052
24. Remijsen Q, Vanden Berghe T, Wirawan E, Asselbergh B, Parthoens E, De Rycke R, et al. Neutrophil extracellular trap cell death requires both autophagy and superoxide generation. Cell Res. (2011) 21:290–304. doi: 10.1038/cr.2010.150
25. Khan MA, Palaniyar N. Transcriptional firing helps to drive NETosis. Sci Rep. (2017) 7:41749. doi: 10.1038/srep41749
26. Desai J, Kumar SV, Mulay SR, Konrad L, Romoli S, Schauer C, et al. PMA and crystal-induced neutrophil extracellular trap formation involves RIPK1-RIPK3-MLKL signaling. Eur J Immunol. (201). 46:223–9. doi: 10.1002/eji.201545605
27. Amini P, Stojkov D, Wang X, Wicki S, Kaufmann T, Wong WW, et al. NET formation can occur independently of RIPK3 and MLKL signaling. Eur J Immunol. (2016) 46:178–84. doi: 10.1002/eji.201545615
28. Parker H, Dragunow M, Hampton MB, Kettle AJ, Winterbourn CC. Requirements for NADPH oxidase and myeloperoxidase in neutrophil extracellular trap formation differ depending on the stimulus. J Leukoc Biol. (2012) 92:841–9. doi: 10.1189/jlb.1211601
29. Zhou Y, An LL, Chaerkady R, Mittereder N, Clarke L, Cohen TS, et al. Evidence for a direct link between PAD4-mediated citrullination and the oxidative burst in human neutrophils. Sci Rep. (2018) 8:15228. doi: 10.1038/s41598-018-33385-z
30. Martinod K, Witsch T, Farley K, Gallant M, Remold-O'Donnell E, Wagner DD. Neutrophil elastase-deficient mice form neutrophil extracellular traps in an experimental model of deep vein thrombosis. J Thromb Haemost. (201). 14:551–8. doi: 10.1111/jth.13239
31. Metzler KD, Goosmann C, Lubojemska A, Zychlinsky A, Papayannopoulos V. A myeloperoxidase-containing complex regulates neutrophil elastase release and actin dynamics during NETosis. Cell Rep. (2014) 8:883–96. doi: 10.1016/j.celrep.2014.06.044
32. Wright HL, Moots RJ, Edwards SW. The multifactorial role of neutrophils in rheumatoid arthritis. Nat Rev Rheumatol. (2014) 10:593–601. doi: 10.1038/nrrheum.2014.80
33. Wright HL, Moots RJ, Bucknall RC, Edwards SW. Neutrophil function in inflammation and inflammatory diseases. Rheumatology. (2010) 49:1618–31. doi: 10.1093/rheumatology/keq045
34. Glennon-Alty L, Hackett AP, Chapman EA, Wright HL. Neutrophils and redox stress in the pathogenesis of autoimmune disease. Free Radic Biol Med. (2018) 125:25–35. doi: 10.1016/j.freeradbiomed.2018.03.049
35. Thieblemont N, Wright HL, Edwards SW, Witko-Sarsat V. Human neutrophils in auto-immunity. Sem Immunol. (2016) 28:159–73. doi: 10.1016/j.smim.2016.03.004
36. Apel F, Zychlinsky A, Kenny EF. The role of neutrophil extracellular traps in rheumatic diseases. Nat Rev Rheumatol. (2018) 14:467–75. doi: 10.1038/s41584-018-0039-z
37. Cross A, Bakstad D, Allen JC, Thomas L, Moots RJ, Edwards SW. Neutrophil gene expression in rheumatoid arthritis. Pathophysiology. (2005) 12:191–202. doi: 10.1016/j.pathophys.2005.07.006
38. Wright HL, Thomas HB, Moots RJ, Edwards SW. Interferon gene expression signature in rheumatoid arthritis neutrophils correlates with a good response to TNFi therapy. Rheumatology. (2015) 54:188–93. doi: 10.1093/rheumatology/keu299
39. Cross A, Barnes T, Bucknall RC, Edwards SW, Moots RJ. Neutrophil apoptosis in rheumatoid arthritis is regulated by local oxygen tensions within joints. J Leukoc Biol. (2006) 80:521–8. doi: 10.1189/jlb.0306178
40. Wright HL, Chikura B, Bucknall RC, Moots RJ, Edwards SW. Changes in expression of membrane TNF, NF-κB activation and neutrophil apoptosis during active and resolved inflammation. Ann Rheum Dis. (2011).70:537–43. doi: 10.1136/ard.2010.138065
41. Kaul A, Gordon C, Crow MK, Touma Z, Urowitz MB, van Vollenhoven R, et al. Systemic lupus erythematosus. Nat Rev Dis Primers. (2016) 2:16039. doi: 10.1038/nrdp.2016.39
42. Lisnevskaia L, Murphy G, Isenberg D. Systemic lupus erythematosus. Lancet. (2014) 384:1878–88. doi: 10.1016/S0140-6736(14)60128-8
43. Courtney PA, Crockard AD, Williamson K, Irvine AE, Kennedy RJ, Bell AL. Increased apoptotic peripheral blood neutrophils in systemic lupus erythematosus: relations with disease activity, antibodies to double stranded DNA, neutropenia. Ann Rheum Dis. (1999) 58:309–14. doi: 10.1136/ard.58.5.309
44. Kaplan MJ. Neutrophils in the pathogenesis and manifestations of SLE. Nat Rev Rheumatol. (2011) 7:691–9. doi: 10.1038/nrrheum.2011.132
45. Villanueva E, Yalavarthi S, Berthier CC, Hodgin JB, Khandpur R, Lin AM, et al. Netting neutrophils induce endothelial damage, infiltrate tissues, expose immunostimulatory molecules in systemic lupus erythematosus. J Immunol. (2011) 187:538–52 doi: 10.4049/jimmunol.1100450
46. Lood C, Blanco LP, Purmalek MM, Carmona-Rivera C, De Ravin SS, Smith CK, et al. Neutrophil extracellular traps enriched in oxidized mitochondrial DNA are interferogenic and contribute to lupus-like disease. Nat Med. (2016) 22:146–53. doi: 10.1038/nm.4027
47. Corsiero E, Bombardieri M, Carlotti E, Pratesi F, Robinson W, Migliorini P, et al. Single cell cloning and recombinant monoclonal antibodies generation from RA synovial B cells reveal frequent targeting of citrullinated histones of NETs. Ann Rheum Dis. (2016) 75:1866–75. doi: 10.1136/annrheumdis-2015-208356
48. Dieker J, Berden J H, Bakker M, Briand JP, Muller S, Voll R, et al. Autoantibodies against modified histone peptides in SLE patients are associated with disease activity and lupus nephritis. PLoS ONE. (2016) 11:e0165373. doi: 10.1371/journal.pone.0165373
49. Dwivedi N, Upadhyay J, Neeli I, Khan S, Pattanaik D, Myers L, et al. Felty's syndrome autoantibodies bind to deiminated histones and neutrophil extracellular chromatin traps. Arthritis Rheum. (2012) 64:982–92. doi: 10.1002/art.33432
50. Pratesi F, Dioni I, Tommasi C, Alcaro MC, Paolini I, Barbetti F, et al. Antibodies from patients with rheumatoid arthritis target citrullinated histone 4 contained in neutrophils extracellular traps. Ann Rheum Dis. (2014) 73:1414–22. doi: 10.1136/annrheumdis-2012-202765
51. Papayannopoulos V. Neutrophil extracellular traps in immunity and disease. Nat Rev Immunol. (2018) 18:134–47. doi: 10.1038/nri.2017.105
52. Gupta S, Kaplan MJ. The role of neutrophils and NETosis in autoimmune and renal diseases. Nat Rev Nephrol. (2016) 12:402–13. doi: 10.1038/nrneph.2016.71
53. Lee KH, Kronbichler A, Park DD, Park Y, Moon H, Kim H, et al. Neutrophil extracellular traps (NETs) in autoimmune diseases: A comprehensive review. Autoimmun Rev. (2017). 16:1160–73. doi: 10.1016/j.autrev.2017.09.012
54. Wang W, Jian Z, Guo J, Ning X. Increased levels of serum myeloperoxidase in patients with active rheumatoid arthritis. Life Sci. (2014) 117:19–23. doi: 10.1016/j.lfs.2014.09.012
55. Pieterse E, Rother N, Yanginlar C, Gerretsen J, Boeltz S, Munoz LE, et al. Cleaved N-terminal histone tails distinguish between NADPH oxidase (NOX)-dependent and NOX-independent pathways of neutrophil extracellular trap formation. Ann Rheum Dis. (2018) 77:1790–8. doi: 10.1136/annrheumdis-2018-213223
56. Wang W, Peng W, Ning X. Increased levels of neutrophil extracellular trap remnants in the serum of patients with rheumatoid arthritis. Int J Rheum Dis. (2018) 21:415–21. doi: 10.1111/1756-185X.13226
57. Lande R, Ganguly D, Facchinetti V, Frasca L, Conrad C, Gregorio J, et al. Neutrophils activate plasmacytoid dendritic cells by releasing self-DNA-peptide complexes in systemic lupus erythematosus. Sci Transl Med. (2011) 3:73ra19. doi: 10.1126/scitranslmed.3001180
58. Denny MF, Yalavarthi S, Zhao W, Thacker SG, Anderson M, Sandy AR, et al. A distinct subset of proinflammatory neutrophils isolated from patients with systemic lupus erythematosus induces vascular damage and synthesizes type I IFNs. J Immunol. (2010). 184:3284–97. doi: 10.4049/jimmunol.0902199
59. Carmona-Rivera C, Zhao W, Yalavarthi S, Kaplan MJ. Neutrophil extracellular traps induce endothelial dysfunction in systemic lupus erythematosus through the activation of matrix metalloproteinase-2. Ann Rheum Dis. (2015) 74:1417–24. doi: 10.1136/annrheumdis-2013-204837
60. Massberg S, Grahl L, von Bruehl ML, Manukyan D, Pfeiler S, Goosmann C, et al. Reciprocal coupling of coagulation and innate immunity via neutrophil serine proteases. Nat Med. (201). 16:887–96. doi: 10.1038/nm.2184
61. von Bruhl ML, Stark K, Steinhart A, Chandraratne S, Konrad I, Lorenz M, et al. Monocytes, neutrophils, platelets cooperate to initiate and propagate venous thrombosis in mice in vivo. J Exp Med. (2012) 209:819–35. doi: 10.1084/jem.20112322
62. Urban CF, Ermert D, Schmid M, Abu-Abed U, Goosmann C, Nacken W, et al. Neutrophil extracellular traps contain calprotectin, a cytosolic protein complex involved in host defense against Candida albicans. PLoS Pathog. (2009) 5:e1000639. doi: 10.1371/journal.ppat.1000639
63. O'Donoghue AJ, Jin Y, Knudsen GM, Perera NC, Jenne DE, Murphy JE, et al. Global substrate profiling of proteases in human neutrophil extracellular traps reveals consensus motif predominantly contributed by elastase. PLoS ONE. (2013) 8:e75141. doi: 10.1371/journal.pone.0075141
64. Chatfield SM, Grebe K, Whitehead LW, Rogers KL, Nebl T, Murphy JM, et al. Monosodium Urate crystals generate nuclease-resistant neutrophil extracellular traps via a distinct molecular pathway. J Immunol. (2018). 200:1802–16. doi: 10.4049/jimmunol.1701382
65. Aletaha D, Neogi T, Silman AJ, Funovits J, Felson DT, Bingham CO III, et al. 2010 Rheumatoid arthritis classification criteria, an American College of Rheumatology/European League Against Rheumatism collaborative initiative. Arthritis Rheum. (2010) 62:2569–81. doi: 10.1002/art.27584
66. Hochberg MC. Updating the American College of Rheumatology revised criteria for the classification of systemic lupus erythematosus. Arthritis Rheum. (1997) 40:1725. doi: 10.1002/art.1780400928
67. Wright HL, Cox T, Moots RJ, Edwards SW. Neutrophil biomarkers predict response to therapy with tumor necrosis factor inhibitors in rheumatoid arthritis. J Leukoc Biol. (2017) 101:785–95. doi: 10.1189/jlb.5A0616-258R
68. Fossati G, Bucknall RC, Edwards SW. Insoluble and soluble immune complexes activate neutrophils by distinct activation mechanisms: changes in functional responses induced by priming with cytokines. Ann Rheum Dis. (2002) 61:13–9. doi: 10.1136/ard.61.1.13
69. Schindelin J, Arganda-Carreras I, Frise E, Kaynig V, Longair M, Pietzsch T, et al. Fiji: an open-source platform for biological-image analysis. Nat Methods. (2012) 9:676–82. doi: 10.1038/nmeth.2019
70. Sommer C, Straehle C, Köthe U, Hamprecht FA. Ilastik, and Interactive learning and segmentation toolkit. In: 2011 IEEE International Symposium on Biomedical Imaging, and From Nano to Macro. Chicago, IL (2011). p. 230–3. doi: 10.1109/ISBI.2011.5872394
71. McLean L, Hurst JL, Gaskell CJ, Lewis JC, Beynon RJ. Characterization of cauxin in the urine of domestic and big cats. J Chem Ecol. (2007) 33:1997–2009. doi: 10.1007/s10886-007-9354-6
72. Zhang J, Xin L, Shan B, Chen W, Xie M, Yuen D, et al. PEAKS DB: de novo sequencing assisted database search for sensitive and accurate peptide identification. Mol Cell Proteomics. (2012) 11:M111 010587. doi: 10.1074/mcp.M111.010587
73. Vizcaino JA, Csordas A, del-Toro N, Dianes JA, Griss J, Lavidas I, et al. 2016 update of the PRIDE database and its related tools. Nucleic Acids Res. (2016) 44:D447–56. doi: 10.1093/nar/gkv1145
74. Saeed AI, Bhagabati NK, Braisted JC, Liang W, Sharov V, Howe EA, et al. TM4 microarray software suite. Methods Enzymol. (2006) 411:134–93. doi: 10.1016/S0076-6879(06)11009-5
75. Huang da W, Sherman BT, Lempicki RA. Systematic and integrative analysis of large gene lists using DAVID bioinformatics resources. Nat Protoc. (2009) 4:44–57. doi: 10.1038/nprot.2008.211
76. Huang da W, Sherman BT, Lempicki RA. Bioinformatics enrichment tools: paths toward the comprehensive functional analysis of large gene lists. Nucleic Acids Res. (2009) 37:1–13. doi: 10.1093/nar/gkn923
77. Maueroder C, Mahajan A, Paulus S, Gosswein S, Hahn J, Kienhofer D, et al. Menage-a-trois: the ratio of bicarbonate to CO2 and the pH regulate the capacity of neutrophils to form NETs. Front Immunol. (2016) 7:583. doi: 10.3389/fimmu.2016.00583
78. Kamoshida G, Kikuchi-Ueda T, Nishida S, Tansho-Nagakawa S, Kikuchi H, Ubagai T, et al. Spontaneous formation of neutrophil extracellular traps in serum-free culture conditions. FEBS Open Bio. (2017) 7:877–86. doi: 10.1002/2211-5463.12222
79. Rorvig S, Ostergaard O, Heegaard NH, Borregaard N. Proteome profiling of human neutrophil granule subsets, secretory vesicles, and cell membrane: correlation with transcriptome profiling of neutrophil precursors. J Leukoc Biol. (2013) 94:711–21. doi: 10.1189/jlb.1212619
80. Dransfield I, Buckle AM, Savill JS, McDowall A, Haslett C, Hogg N. Neutrophil apoptosis is associated with a reduction in CD16 (Fc gamma RIII) expression. J Immunol. (1994) 153:1254–63.
81. Mitchell TS, Moots RJ, Wright HL. Janus kinase inhibitors prevent migration of rheumatoid arthritis neutrophils towards interleukin-8, but do not inhibit priming of the respiratory burst or reactive oxygen species production. Clin Exp Immunol. (2017) 189:250–8. doi: 10.1111/cei.12970
82. Dwivedi N, Neeli I, Schall N, Wan H, Desiderio DM, Csernok E, et al. Deimination of linker histones links neutrophil extracellular trap release with autoantibodies in systemic autoimmunity. FASEB J. (2014) 28:2840–51. doi: 10.1096/fj.13-247254
83. Carmona-Rivera C, Carlucci PM, Moore E, Lingampalli N, Uchtenhagen H, James E, et al. Synovial fibroblast-neutrophil interactions promote pathogenic adaptive immunity in rheumatoid arthritis. Sci Immunol. (2017) 2:eaag3358. doi: 10.1126/sciimmunol.aag3358
84. Bennike T, Lauridsen K, Olesen M, Andersen V, Birkelund S, Stensballe A. Optimizing the identification of citrullinated peptides by mass spectrometry: utilizing the inability of trypsin to cleave after citrullinated amino acids. J Proteomics Bioinform. (2013) 6:288–95. doi: 10.4172/jpb.1000293
85. Calzetti F, Tamassia N, Arruda-Silva F, Gasperini S, Cassatella MA. The importance of being “pure” neutrophils. J Allergy Clin Immunol. (2017) 139:352–5 e356. doi: 10.1016/j.jaci.2016.06.025
86. Carestia A, Kaufman T, Rivadeneyra L, Landoni VI, Pozner RG, Negrotto S, et al. Mediators and molecular pathways involved in the regulation of neutrophil extracellular trap formation mediated by activated platelets. J Leukoc Biol. (2016) 99:153–62. doi: 10.1189/jlb.3A0415-161R
87. McDonald B, Davis RP, Kim SJ, Tse M, Esmon CT, Kolaczkowska E, et al. Platelets and neutrophil extracellular traps collaborate to promote intravascular coagulation during sepsis in mice. Blood. (2017) 129:1357–67. doi: 10.1182/blood-2016-09-741298
88. Hazeldine J, Harris P, Chapple IL, Grant M, Greenwood H, Livesey A, et al. Impaired neutrophil extracellular trap formation: a novel defect in the innate immune system of aged individuals. Aging Cell. (2014) 13:690–8. doi: 10.1111/acel.12222
89. Sherer Y, Gorstein A, Fritzler MJ, Shoenfeld Y. Autoantibody explosion in systemic lupus erythematosus: more than 100 different antibodies found in SLE patients. Semin Arthritis Rheum. (2004) 34:501–37. doi: 10.1016/j.semarthrit.2004.07.002
90. Gripenberg M, Helve T, Kurki P. Profiles of antibodies to histones, DNA and IgG in patients with systemic rheumatic diseases determined by ELISA. J Rheumatol. (1985) 12:934–9.
91. Wallace DJ, Lin HC, Shen GQ, Peter JB. Antibodies to histone (H2A-H2B)-DNA complexes in the absence of antibodies to double-stranded DNA or to (H2A-H2B) complexes are more sensitive and specific for scleroderma-related disorders than for lupus. Arthritis Rheum. (1994). 37:1795–7. doi: 10.1002/art.1780371213
92. Muller S, Bonnier D, Thiry M, Van Regenmortel MH. Reactivity of autoantibodies in systemic lupus erythematosus with synthetic core histone peptides. Int Arch Allergy Appl Immunol. (1989) 89:288–96. doi: 10.1159/000234962
93. Shoenfeld Y, Segol G, Segol O, Neary B, Klajman A, Stollar BD, et al. Detection of antibodies to total histones and their subfractions in systemic lupus erythematosus patients and their asymptomatic relatives. Arthritis Rheum. (1987) 30:169–75. doi: 10.1002/art.1780300207
94. Schett G, Steiner G, Smolen JS. Nuclear antigen histone H1 is primarily involved in lupus erythematosus cell formation. Arthritis Rheum. (1998) 41:1446–55. doi: 10.1002/1529-0131(199808)41:8<1446::AID-ART15>3.0.CO;2-6
95. Fritzler MJ, Tan EM. Antibodies to histones in drug-induced and idiopathic lupus erythematosus. J Clin Invest. (1978) 62:560–7. doi: 10.1172/JCI109161
96. Tzioufas AG, Boumba VA, Seferiadis K, Tsolas O, Moutsopoulos HM. Autoantibodies to HMG-17 nucleosomal protein in autoimmune rheumatic diseases. Correlation with systemic lupus erythematosus clinical activity and with antibodies to double-stranded DNA. Arthritis Rheum. (1993) 36:955–61. doi: 10.1002/art.1780360712
97. Mansour RB, Lassoued S, Gargouri B, El Gaid A, Attia H, Fakhfakh F. Increased levels of autoantibodies against catalase and superoxide dismutase associated with oxidative stress in patients with rheumatoid arthritis and systemic lupus erythematosus. Scand J Rheumatol. (2008) 37:103–8. doi: 10.1080/03009740701772465
98. Brito J, Biamonti G, Caporali R, Montecucco C. Autoantibodies to human nuclear lamin B2 protein. Epitope specificity in different autoimmune diseases. J Immunol. (1994) 153:2268–77.
99. Reeves WH, Chaudhary N, Salerno A, Blobel G. Lamin B autoantibodies in sera of certain patients with systemic lupus erythematosus. J Exp Med. (1987) 165:750–62. doi: 10.1084/jem.165.3.750
100. Senecal JL, Rauch J, Grodzicky T, Raynauld JP, Uthman I, Nava A, et al. Strong association of autoantibodies to human nuclear lamin B1 with lupus anticoagulant antibodies in systemic lupus erythematosus. Arthritis Rheum. (1999). 42:1347–53.
101. Lassoued K, Guilly MN, Danon F, Andre C, Dhumeaux D, Clauvel JP, et al. Antinuclear autoantibodies specific for lamins. Characterization and clinical significance. Ann Intern Med. (1988) 108:829–33. doi: 10.7326/0003-4819-108-6-829
102. Dinu AR, Merrill JT, Shen C, Antonov IV, Myones BL, Lahita RG. Frequency of antibodies to the cholesterol transport protein apolipoprotein A1 in patients with SLE. Lupus. (1998) 7:355–60. doi: 10.1191/096120398678920262
103. Bruschi M, Petretto A, Vaglio A, Santucci L, Candiano G, Ghiggeri GM. Annexin A1 and autoimmunity: from basic science to clinical applications. Int J Mol Sci. (2018) 19:E1348. doi: 10.3390/ijms19051348
104. Bruschi M, Sinico RA, Moroni G, Pratesi F, Migliorini P, Galetti M, et al. Glomerular autoimmune multicomponents of human lupus nephritis in vivo: alpha-enolase and annexin AI. J Am Soc Nephrol. (2014) 25:2483–98. doi: 10.1681/ASN.2013090987
105. Kretz CC, Norpo M, Abeler-Dorner L, Linke B, Haust M, Edler L, et al. Anti-annexin 1 antibodies: a new diagnostic marker in the serum of patients with discoid lupus erythematosus. Exp Dermatol. (2010) 19:919–21. doi: 10.1111/j.1600-0625.2010.01145.x
106. Kahlenberg JM, Kaplan MJ. Little peptide, big effects: the role of LL-37 in inflammation and autoimmune disease. J Immunol. (2013) 191:4895–901. doi: 10.4049/jimmunol.1302005
107. Sun CL, Zhang F Z. Li P, Bi LQ. LL-37 expression in the skin in systemic lupus erythematosus. Lupus. (2011) 20:904–11. doi: 10.1177/0961203311398515
108. Kienhofer D, Hahn J, Schubert I, Reinwald C, Ipseiz N, Lang SC, et al. No evidence of pathogenic involvement of cathelicidins in patient cohorts and mouse models of lupus and arthritis. PLoS ONE. (2014) 9:e115474. doi: 10.1371/journal.pone.0115474
109. Bhattacharjee M, Balakrishnan L, Renuse S, Advani J, Goel R, Sathe G, et al. Synovial fluid proteome in rheumatoid arthritis. Clin Proteomics. (2016) 13:12. doi: 10.1186/s12014-016-9113-1
110. van Beers JJ, Schwarte CM, Stammen-Vogelzangs J, Oosterink E, Bozic B, Pruijn GJ The rheumatoid arthritis synovial fluid citrullinome reveals novel citrullinated epitopes in apolipoprotein E, myeloid nuclear differentiation antigen, and beta-actin. Arthritis Rheum. (2013) 65:69–80. doi: 10.1002/art.37720
111. Wright HL, Bucknall RC, Moots RJ, Edwards SW. Analysis of SF and plasma cytokines provides insights into the mechanisms of inflammatory arthritis and may predict response to therapy. Rheumatology. (2012) 51:451–9. doi: 10.1093/rheumatology/ker338
112. Tchetverikov I, Ronday HK, Van El B, Kiers GH, Verzijl N, TeKoppele JM, et al. MMP profile in paired serum and synovial fluid samples of patients with rheumatoid arthritis. Ann Rheum Dis. (200). 63:881–3. doi: 10.1136/ard.2003.013243
113. Yoshihara Y, Nakamura H, Obata K, Yamada H, Hayakawa T, Fujikawa K, et al. Matrix metalloproteinases and tissue inhibitors of metalloproteinases in synovial fluids from patients with rheumatoid arthritis or osteoarthritis. Ann Rheum Dis. (2000) 59:455–61. doi: 10.1136/ard.59.6.455
114. Itoh T, Matsuda H, Tanioka M, Kuwabara K, Itohara S, Suzuki R. The role of matrix metalloproteinase-2 and matrix metalloproteinase-9 in antibody-induced arthritis. J Immunol. (2002) 169:2643–7. doi: 10.4049/jimmunol.169.5.2643
115. Mattey DL, Nixon NB, Dawes PT. Association of circulating levels of MMP-8 with mortality from respiratory disease in patients with rheumatoid arthritis. Arthritis Res Ther. (2012) 14:R204. doi: 10.1186/ar4042
116. Cohen MG, Webb J. Antihistone antibodies in rheumatoid arthritis and Felty's syndrome. Arthritis Rheum. (1989) 32:1319–24. doi: 10.1002/anr.1780321020
117. Tuaillon N, Muller S, Pasquali JL, Bordigoni P, Youinou P, Van Regenmortel MH. Antibodies from patients with rheumatoid arthritis and juvenile chronic arthritis analyzed with core histone synthetic peptides. Int Arch Allergy Appl Immunol. (1990) 91:297–305. doi: 10.1159/000235131
118. Sohn DH, Rhodes C, Onuma K, Zhao X, Sharpe O, Gazitt T, et al. Local Joint inflammation and histone citrullination in a murine model of the transition from preclinical autoimmunity to inflammatory arthritis. Arthritis Rheumatol. (2015) 67:2877–87. doi: 10.1002/art.39283
119. Burlingame RW, Boey ML, Starkebaum G, Rubin RL. The central role of chromatin in autoimmune responses to histones and DNA in systemic lupus erythematosus. J Clin Invest. (1994). 94:184–92. doi: 10.1172/JCI117305
120. Suzuki T, Burlingame RW, Casiano CA, Boey ML, Rubin RL. Antihistone antibodies in systemic lupus erythematosus: assay dependency and effects of ubiquitination and serum DNA. J Rheumatol. (1994). 21:1081–91.
121. van Bavel CC, Dieker JW, Kroeze Y, Tamboer WP, Voll R, Muller S, et al. Apoptosis-induced histone H3 methylation is targeted by autoantibodies in systemic lupus erythematosus. Ann Rheum Dis. (2011) 70:201–7. doi: 10.1136/ard.2010.129320
122. Rother N, Pieterse E, Lubbers J, Hilbrands L, van der Vlag J. Acetylated histones in apoptotic microparticles drive the formation of neutrophil extracellular traps in active lupus nephritis. Front Immunol. (2017) 8:1136. doi: 10.3389/fimmu.2017.01136
123. Pieterse E, Hofstra J, Berden J, Herrmann M, Dieker J, van der Vlag J. Acetylated histones contribute to the immunostimulatory potential of neutrophil extracellular traps in systemic lupus erythematosus. Clin Exp Immunol. (2015) 179:68–74. doi: 10.1111/cei.12359
124. Scally SW, Petersen J, Law SC, Dudek NL, Nel HJ, Loh KL, et al. A molecular basis for the association of the HLA-DRB1 locus, citrullination, and rheumatoid arthritis. J Exp Med. (2013) 210:2569–82. doi: 10.1084/jem.20131241
125. Berglin E, Padyukov L, Sundin U, Hallmans G, Stenlund H, Van Venrooij WJ, et al. A combination of autoantibodies to cyclic citrullinated peptide (CCP) and HLA-DRB1 locus antigens is strongly associated with future onset of rheumatoid arthritis. Arthritis Res Ther. (2004) 6:R303–8. doi: 10.1186/ar1187
126. Vossenaar ER, Despres N, Lapointe E, van der Heijden A, Lora M, Senshu T, et al. Rheumatoid arthritis specific anti-Sa antibodies target citrullinated vimentin. Arthritis Res Ther. (2004) 6:R142–150. doi: 10.1186/ar1149
127. Kinloch A, Lundberg K, Wait R, Wegner N, Lim NH, Zendman AJ, et al. Synovial fluid is a site of citrullination of autoantigens in inflammatory arthritis. Arthritis Rheum. (200). 58:2287–95.
128. Spengler J, Lugonja B, Ytterberg AJ, Zubarev RA, Creese AJ, Pearson MJ, et al. Release of active Peptidyl Arginine deiminases by neutrophils can explain production of extracellular citrullinated autoantigens in rheumatoid arthritis synovial fluid. Arthritis Rheumatol. (2015). 67:3135–45. doi: 10.1002/art.39313
129. Bawadekar M, Shim D, Johnson CJ, Warner TF, Rebernick R, Damgaard D, et al. Peptidylarginine deiminase 2 is required for tumor necrosis factor alpha-induced citrullination and arthritis, but not neutrophil extracellular trap formation. J Autoimmun. (2017). 80:39–47. doi: 10.1016/j.jaut.2017.01.006
130. Darrah E, Giles JT, Ols ML, Bull HG, Andrade F, Rosen A. Erosive rheumatoid arthritis is associated with antibodies that activate PAD4 by increasing calcium sensitivity. Sci Transl Med. (2013) 5:186ra165. doi: 10.1126/scitranslmed.3005370
131. Rohrbach AS, Hemmers S, Arandjelovic S, Corr M, Mowen KA. PAD4 is not essential for disease in the K/BxN murine autoantibody-mediated model of arthritis. Arthritis Res Ther. (2012) 14:R104. doi: 10.1186/ar3829
132. Seri Y, Shoda H, Suzuki A, Matsumoto I, Sumida T, Fujio K, et al. Peptidylarginine deiminase type 4 deficiency reduced arthritis severity in a glucose-6-phosphate isomerase-induced arthritis model. Sci Rep. (2015) 5:13041. doi: 10.1038/srep13041
133. Meegan JE, Yang X, Beard RS Jr, Jannaway M, Chatterjee V, Taylor-Clark TE, et al. Citrullinated histone 3 causes endothelial barrier dysfunction. Biochem Biophys Res Commun. (2018) 503:1498–502. doi: 10.1016/j.bbrc.2018.07.069
134. Kilsgard O, Andersson P, Malmsten M, Nordin SL, Linge HM, Eliasson M, et al. Peptidylarginine deiminases present in the airways during tobacco smoking and inflammation can citrullinate the host defense peptide LL-37, resulting in altered activities. Am J Respir Cell Mol Biol. (2012) 46:240–8. doi: 10.1165/rcmb.2010-0500OC
135. Konig MF, Andrade F. A critical reappraisal of neutrophil extracellular traps and NETosis mimics based on differential requirements for protein citrullination. Front Immunol. (2016) 7:461. doi: 10.3389/fimmu.2016.00461
136. Naccache PH, Fernandes MJ. Challenges in the characterization of neutrophil extracellular traps: the truth is in the details. Eur J Immunol. (2016) 46:52–5. doi: 10.1002/eji.201546022
137. Scozzi D, Wang X, Liao F, Zhiyi L, Zhu J, Pugh K, et al. Neutrophil extracellular trap fragments stimulate innate immune responses that prevent lung transplant tolerance. Am J Transplant. (2018). doi: 10.1111/ajt.15163. [Epub ahead of print].
138. Papadaki G, Kambas K, Choulaki C, Vlachou K, Drakos E, Bertsias G, et al. Neutrophil extracellular traps exacerbate Th1-mediated autoimmune responses in rheumatoid arthritis by promoting DC maturation. Eur J Immunol. (2016) 46:2542–54. doi: 10.1002/eji.201646542
139. Arampatzioglou A, Papazoglou D, Konstantinidis T, Chrysanthopoulou A, Mitsios A, Angelidou I, et al. Clarithromycin enhances the antibacterial activity and wound healing capacity in type 2 diabetes mellitus by increasing LL-37 load on neutrophil extracellular traps. Front Immunol. (2018) 9:2064. doi: 10.3389/fimmu.2018.02064
Keywords: neutrophil, neutrophil extracellular trap, NET, rheumatoid arthritis, systemic lupus erythematosus, citrullinated, histones
Citation: Chapman EA, Lyon M, Simpson D, Mason D, Beynon RJ, Moots RJ and Wright HL (2019) Caught in a Trap? Proteomic Analysis of Neutrophil Extracellular Traps in Rheumatoid Arthritis and Systemic Lupus Erythematosus. Front. Immunol. 10:423. doi: 10.3389/fimmu.2019.00423
Received: 29 November 2018; Accepted: 18 February 2019;
Published: 11 March 2019.
Edited by:
Liwu Li, Virginia Tech, United StatesReviewed by:
Yi Zhao, West China Hospital, Sichuan University, ChinaCopyright © 2019 Chapman, Lyon, Simpson, Mason, Beynon, Moots and Wright. This is an open-access article distributed under the terms of the Creative Commons Attribution License (CC BY). The use, distribution or reproduction in other forums is permitted, provided the original author(s) and the copyright owner(s) are credited and that the original publication in this journal is cited, in accordance with accepted academic practice. No use, distribution or reproduction is permitted which does not comply with these terms.
*Correspondence: Helen L. Wright, aGx3cmlnaHRAbGl2ZXJwb29sLmFjLnVr
Disclaimer: All claims expressed in this article are solely those of the authors and do not necessarily represent those of their affiliated organizations, or those of the publisher, the editors and the reviewers. Any product that may be evaluated in this article or claim that may be made by its manufacturer is not guaranteed or endorsed by the publisher.
Research integrity at Frontiers
Learn more about the work of our research integrity team to safeguard the quality of each article we publish.