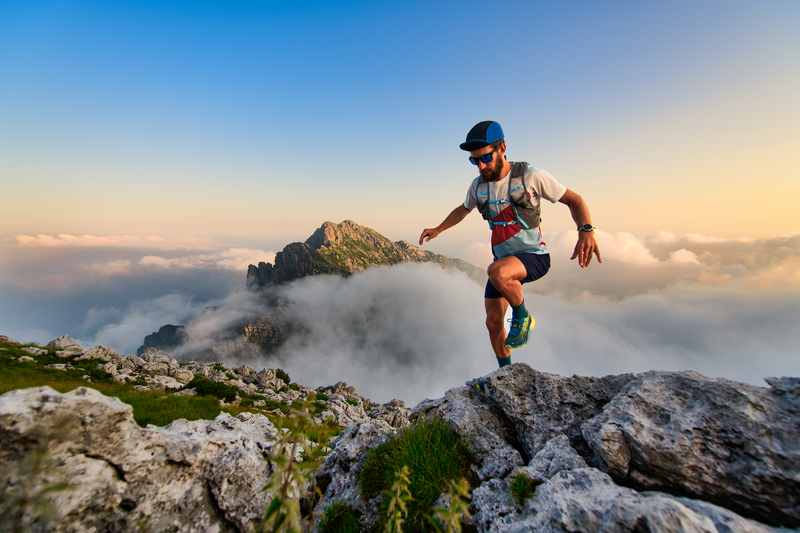
94% of researchers rate our articles as excellent or good
Learn more about the work of our research integrity team to safeguard the quality of each article we publish.
Find out more
ORIGINAL RESEARCH article
Front. Immunol. , 04 March 2019
Sec. Comparative Immunology
Volume 10 - 2019 | https://doi.org/10.3389/fimmu.2019.00160
This article is part of the Research Topic The Role of Red Blood Cells in the Immune Response of Fish View all 6 articles
Rock bream iridovirus (RBIV) causes severe mass mortality in Korean rock bream (Oplegnathus fasciatus) populations. To date, immune defense mechanisms of rock bream against RBIV are unclear. While red blood cells (RBCs) are known to be involved in the immune response against viral infections, the participation of rock bream RBCs in the immune response against RBIV has not been studied yet. In this study, we examined induction of the immune response in rock bream RBCs after RBIV infection. Each fish was injected with RBIV, and virus copy number in RBCs gradually increased from 4 days post-infection (dpi), peaking at 10 dpi. A total of 318 proteins were significantly regulated in RBCs from RBIV-infected individuals, 183 proteins were upregulated and 135 proteins were downregulated. Differentially upregulated proteins included those involved in cellular amino acid metabolic processes, cellular detoxification, snRNP assembly, and the spliceosome. Remarkably, the MHC class I-related protein pathway was upregulated during RBIV infection. Simultaneously, the regulation of apoptosis-related proteins, including caspase-6 (CASP6), caspase-9 (CASP9), Fas cell surface death receptor (FAS), desmoplakin (DSP), and p21 (RAC1)-activated kinase 2 (PAK2) changed with RBIV infection. Interestingly, the expression of genes within the ISG15 antiviral mechanism-related pathway, including filamin B (FLNB), interferon regulatory factor 3 (IRF3), nucleoporin 35 (NUP35), tripartite motif-containing 25 (TRIM25), and karyopherin subunit alpha 3 (KPNA3) were downregulated in RBCs from RBIV-infected individuals. Overall, these findings contribute to the understanding of RBIV pathogenesis and host interaction.
Rock bream iridovirus (RBIV) is a dsDNA virus that belongs to family Iridoviridae, genus Megalocytivirus (1). This virus causes severe mass mortality in Korean rock bream (Oplegnathus fasciatus) populations. RBIV was first reported in the summer of 1998 in southern coastal areas of Korea (2). Since then, high mortality resulting from RBIV occurs every year, causing important economic losses in rock bream aquaculture. RBIV is known to cause strong pathogenicity in rock bream individuals (3–7). To date, the immune response of rock bream with RBIV infection remains unclear, although it represents an important aquaculture health concern. Therefore, it is necessary to further detail the immune response mechanisms underlying the RBIV infection process in rock bream. Over the years, a considerable number of studies have investigated the immune response of rock bream at both physiological and molecular levels by transcriptomic and microarray analyses (8, 9). Recently, an increasing number of studies have been focused on the transcriptional immune responses of rock bream against RBIV (10–15). However, most have focused on kidney-mediated immune responses to determine the pathways responsible for fish mortality or survivability. Therefore, evaluation of the immune response or immune defense mechanisms in different organs is useful for the understanding host-RBIV interactions.
In contrast to mammalian red blood cells (RBCs) or erythrocytes, which lack a cell nucleus and organelles (16), nonmammalian RBCs are nucleated and contain organelles in their cytoplasm (17). Although the main physiological role for RBCs is the transportation of respiratory gases, their role in the antiviral response has recently been uncovered (18). Importantly, teleost RBCs can induce toll-like receptor (TLR) and peptidoglycan recognition protein (PGRP) receptor families (19), pathogen presentation to macrophages (20), and cytokine or interferon production (21–25). In addition, transcriptomic and proteomic studies of rainbow trout (Oncorhynchus mykiss) showed that nucleated RBCs contribute to several immune functions such as antigen presentation, leukocyte activation or immune cytokine production (26, 27).
To date, the impact of RBIV on rock bream RBCs in the global fish immune response has not been studied yet. In the present study, we aimed to investigate the differentially expressed proteins (DEPs) in rock bream RBCs upon RBIV in vivo infection in order to understand the molecular contribution of this cell type in the fish immune response against RBIV infection. Proteomic profiling of RBCs from RBIV-infected fish revealed upregulation of apoptosis, antigen processing, and presentation of peptide antigen via MHC class I (MHC-I) pathways. However, the ISG15 antiviral mechanism pathway appeared to be downregulated.
RBIV was obtained from naturally infected rock bream individuals as previously described (11). RBIV major capsid protein (MCP) gene copy number was quantified from supernatant preparations by quantitative real-time polymerase chain reaction (RT-qPCR). Virus titer was calculated as 1.1 × 107/100 μL MCP gene copies. Although some studies have demonstrated the use of cell lines to culture Megalocytivirus (28, 29), RBIV does not replicate well in in vitro cell culture conditions, so the TCID50 method was not used in this study.
RBIV-free rock bream individuals were obtained from a local farm. Thirty fish (11.2 ± 1.2 cm, 28.1 ± 3.2 g) were maintained at 23°C in an aquarium containing 250 L of UV-treated seawater. Fish were injected intraperitoneally (i.p.) with RBIV (100 μL/fish, 1.1 × 107 MCP gene copies) or phosphate-buffered saline (PBS) (100 μL/fish) as a control. Blood (200 μL/fish) and organs (spleen, kidney, and liver) were collected from RBIV-infected rock bream individuals at 1, 2, 4, 7, and 10 days post infection (dpi) (4 fish per time point). RBCs were isolated from blood (100 μL/fish) and purified by 2 consecutive density gradient centrifugations (7,206 g, Ficoll 1.007, Sigma-Aldrich). For RBIV copy number analysis, genomic DNA was isolated from the RBCs, blood, spleen, kidney, and liver of each fish using High Pure PCR Template Preparation Kit (Roche) following standard protocol. A standard curve was generated to determine RBIV MCP gene copy number by RT-qPCR as described previously (11). Virus copy number was determined from 100 μL of total genomic DNA. Statistical analyses were performed using GraphPad Prism software version 5.0 (GraphPad Software, USA). One-way analysis of variance (ANOVA) was performed between conditions, with Tukey's multiple comparison test. P < 0.05 were considered to indicate statistical significance.
Fish (11.0 ± 0.8 cm, 29.3 ± 4.7 g) were randomly divided into two groups (20 fish per group): a virus-injected group and a PBS-injected group. The experimental group was injected i.p. with RBIV (100 μL/fish) containing 1.1 × 107 MCP gene copies, and the control group was injected i.p. with PBS (100 μL/fish). Each group of fish were maintained at 23°C in the aquarium containing 250 L of UV-treated seawater. Blood (100 μL/fish) was collected from 8 fish at 7 dpi. Then, RBCs were purified by 2 consecutive density gradient centrifugations (7,206 g, Ficoll 1.007, Sigma-Aldrich). All rock bream experiments were carried out in strict accordance with the recommendations of the Institutional Animal Care and Use Committee of Chonnam National University (permit number: CNU IACUC-YS-2015-4).
Ficoll-purified RBCs from 5 fish in each group were pelletized by centrifugation (1,600 rpm). The cell pellet was washed with PBS, digested, cleaned-up/desalted, and pooled for each group (2 control groups and 2 RBIV-infected fish groups). Then, samples were subjected to liquid chromatography and mass spectrometry analysis (LC-MS) as previously described (26), except that the Pierce High pH Reversed-Phase Peptide Fractionation Kit (Thermo Fisher Scientific, Inc.) was used and 3 peptide fractions were collected. Progenesis QI v4.0 (Nonlinear Dynamics, Newcastle, UK) was used for protein differential expression analysis according to “between-subject design.” Log2 peptide ratios followed a normal distribution that was fitted using least squares regression. Mean and standard deviation values were derived from Gaussian fit and were used to estimate P-values and false discovery rates (FDRs). The confidence interval for protein identification was set to ≥95% (P ≤ 0.05). Only proteins having ≥2 quantitated peptides were considered. Peptides with an individual ion score above the 1% FDR threshold were considered correctly identified.
DEP pathway enrichment analysis was performed using ClueGO (30), CluePedia (31), and Cytoscape (32). The GO Biological Process, GO Immune Process, Kegg, Reactome, and Wikipathways databases were used. A P ≤ 0.05 and Kappa score of 0.4 were used as threshold values. Proteins were identified by sequence homology with Homo sapiens using Blast2GO version 4.1.9 (33).
For immune gene expression analysis, total RNA was extracted from RBCs using RNAiso Plus reagent (TaKaRa) following standard protocol. Total RNA was treated with DNase I (TaKaRa) and reverse transcribed using a ReverTra Ace qPCR RT Kit (Toyobo) according to manufacturer's protocol. Real-time PCR was carried out in an Exicycler 96 Real-Time Quantitative Thermal Block (Bioneer) using an AccuPre® 2x Greenstar qPCR Master Mix (Bioneer) as described previously (11). Each assay was performed in duplicate using β-actin genes as the endogenous control. The primers used are listed in Table 1. Relative gene expression was determined by the 2−ΔΔCt method (34). Statistical analyses were performed using GraphPad Prism software. Unpaired T-tests were performed between conditions. P < 0.05 were considered to indicate statistical significance. Data are represented as mean ± standard deviation.
RBIV copy number was quantified in RBC, blood, spleen, kidney, and liver samples. At 2, 4, 7, and 10 dpi, increased viral copy numbers were observed in the spleen, kidney, and liver. The maximum copy number for all samples was reached at 10 dpi (average value of 4.99 × 107 in the spleen, 2.56 × 107 in the kidney, and 2.44 × 107/100 μL in the liver) (Figures 1A–C).
Figure 1. RBIV MCP gene copy number in different rock bream organs. Fish i.p. injected with RBIV (1.1 × 107) were maintained at 23°C. Virus copy number in spleen (A), kidney (B), liver (C), blood (D), and RBCs (E) were analyzed at 1, 2, 4, 7, and 10 days post infection (dpi). One-way analysis of variance (ANOVA) was performed between conditions, with Tukey's multiple comparison test. Different superscript letters denote significant differences (P < 0.05). a≠ b. Data are represented as individual values. Line represents mean value.
In blood samples, the viral transcription level was 7.16 × 101/100 μL at 1 dpi, gradually increased to 3.81 × 102/100 μL at 2 dpi, and reached maximum values of 9.36 × 103/100 μL at 7 dpi and 2.04 × 104/100 μL at 10 dpi (Figure 1D). In Ficoll-purified RBCs from fish at 1, 2, 4, 7, and 10 dpi, virus copy numbers gradually increased with time; the average number of virus copies was 1.25 × 102, 2.31 × 102, 8.42 × 102, 9.22 × 103, and 3.54 × 104/100 μL, respectively (Figure 1E).
Cytoscape pathway enrichment analysis was performed in order to evaluate the functional pathways involved in the response of rock bream RBCs to RBIV (Figure 2). Proteins with a FDR < 0.001 and−1.5>log2 Fold Change (FC)>1.5 were selected for functional network analysis. A total of 318 proteins were differentially regulated at a significant level in RBCs from RBIV-infected individuals: 183 proteins were upregulated and 135 were downregulated. Upregulated pathways were categorized into 13 main categories, while downregulated pathways were categorized into 2 (Figures 2–6 and Tables 2–4). Within upregulated pathways, proteins were involved in synthesis of active ubiquitin, E1 and E2 enzymatic roles, pyridine-containing compound metabolic processes, RNA transport, the spliceosome, cytosolic tRNA aminoacylation, the vitamin B6 biosynthetic process, snRNP assembly, cellular detoxification, the cholesterol biosynthetic process, the cellular amino acid metabolic process, the Parkin-Ubiquitin proteasomal system pathway, apoptosis, and antigen processing and presentation of peptide antigen via MHC class I (Figures 2–4 and Tables 2, 3). Within downregulated pathways, proteins were mainly involved in the ISG15 antiviral mechanism and p130Cas linkage to MAPK signaling for integrins (Figures 2, 5, 6 and Table 4).
Figure 2. Cytoscape network analysis of differentially expressed protein (DEPs) in RBCs from RBIV-infected rock bream. DEPs in RBCs from RBIV-infected rock bream at 7 dpi, with −1.5 < log2FC < 1.5 and FDR P < 0.001. Overrepresented terms were identified by the Cytoscape ClueGo app, with GO Biological Process, Kegg, Reactome, and Wikipathways term databases. Red circles indicate upregulated/overrepresented terms, and green circles indicate downregulated/overrepresented terms. Gray circles indicate unspecific regulation. Color intensity represents the degree of overrepresentation.
Figure 3. Upregulated functional pathways in the proteome profile of RBIV-infected RBCs. Upregulated/overrepresented terms in DEPs of RBCs from RBIV-infected rock bream at 7 dpi, with −1.5 < log2FC < 1.5 and FDR P < 0.001. (A) Bar graph and (B) multilevel pie chart. Overrepresented terms were identified by the Cytoscape ClueGo app, with GO Biological Process, Kegg, Reactome, and Wikipathways term databases. Asterisks denote GO-term significance (*P < 0.05 and **P < 0.01).
Figure 4. GO Immune System Process terms in the proteome profile of RBIV-infected RBCs. Upregulated/overrepresented terms in DEPs of RBCs from RBIV-infected rock bream at 7 dpi, with −1.5<log2FC<1.5 and FDR P < 0.001. (A) Bar graph and (B) multilevel pie chart. Overrepresented terms were identified by the Cytoscape ClueGo app with the GO Immune System Process database. Asterisks denote GO-term significance (**P < 0.01).
Figure 5. Downregulated functional pathways in the proteome profile of RBIV-infected RBCs. Downregulated/overrepresented terms in DEPs of RBCs from RBIV-infected rock bream at 7 dpi, with −1.5 < log2FC < 1.5 and FDR P < 0.001. (A) Bar graph and (B) multilevel pie chart. Overrepresented terms were identified by the Cytoscape ClueGo app, with the GO Biological Process, Kegg, Reactome, and Wikipathways databases. Asterisks denote GO-term significance (*P < 0.05 and **P < 0.01).
Figure 6. Comparative protein levels in upregulated and downregulated overrepresented pathways in RBCs from RBIV-infected rock bream. Data represent the number of proteins represented in each pathway. Red bars indicate upregulated proteins and dashed bars indicate downregulated proteins.
Table 3. List of identified proteins related to antigen processing and presentation of peptide antigen via MHC class I.
A total of 36 apoptosis-related proteins were differentially regulated in RBCs from RBIV-infected individuals: 26 proteins were upregulated and 10 were downregulated (Figure 6). Among them, caspase-6 (CASP6), caspase-9 (CASP9), fas cell surface death receptor (FAS), and desmoplakin (DSP) were upregulated at 1.65, 5.35, 5.89, and 2.26 log2FC, respectively (Table 2). p21 (RAC1)-activated kinase 2 (PAK2) was downregulated at −2.39 log2FC (Table 2).
Ten spliceosome-related proteins were differentially regulated in RBCs from RBIV-infected individuals: 7 proteins were upregulated and 3 were downregulated (Figure 6 and Table 2). Moreover, 6 snRNP assembly-related proteins were differentially expressed: 5 proteins upregulated and 1 protein downregulated (Figure 6 and Table 2). Among upregulated proteins, the top-scored was small nuclear ribonucleoprotein polypeptide F (SNRPF), with 8.79 log2FC. In addition, small nuclear ribonucleoprotein D1 polypeptide (SNRPD1) and small nuclear ribonucleoprotein polypeptide G (SNRPG) were highly upregulated (Table 2).
A total of 28 DEPs in RBCs from RBIV-infected individuals were involved in cellular amino acid metabolic processes, including 22 upregulated and 6 downregulated proteins (Figure 6 and Table 2). Among upregulated proteins, histamine N-methyltransferase (HNMT), aldehyde dehydrogenase 9 family member A1 (ALDH9A1), glutamate-cysteine ligase catalytic subunit (GCLC), phosphoglycerate dehydrogenase (PHGDH), ribosome maturation factor (SBDS), and pyrroline-5-carboxylate reductase 3 (PYCR3) were highly upregulated with log2FC of 7.33, 7.08, 7.06, 5.96, 5.38, and 4.14, respectively (Table 2).
Of the 15 DEPs involved in cellular detoxification, 10 were upregulated (from 1.50 to 6.94 log2FC) and 5 were downregulated (from −2.90 to −5.96 log2FC) (Table 2). Of note, upregulated proteins included antioxidant enzymes such as glutathione S-transferase mu 3 (GSTM3), superoxide dismutase 1 (SOD1), and thioredoxin reductase 3 (TXNRD3).
Of 9 DEPs in RBCs from RBIV-infected individuals involved in antigen processing and presentation of peptide antigen via MHC class I (Figure 4), 7 were upregulated and 2 were downregulated (Figure 6 and Table 3). Among the upregulated proteins (with log2FC ranging from 1.85 to 4.08), were major histocompatibility complex class I-related protein (MR1), transporter 2 ATP binding cassette subfamily B member (TAP2), and 6 proteasome subunit proteins (proteasome 26S subunit non-ATPase 11 [PSMD11], proteasome subunit beta 6 [PSMB6], proteasome subunit beta 3 [PSMB3], proteasome 26S subunit non-ATPase 5 [PSMD5], and proteasome subunit beta 4 [PSMB4]).
The interferon-stimulated gene 15 (ISG15) antiviral mechanism pathway appeared to be mainly downregulated in RBCs from RBIV-infected rock bream (Figure 5). Within this pathway, 3 proteins were upregulated (signal transducer and activator of transcription 1 [STAT1], nucleoporin 93 [NUP93], and nucleoporin 98 [NUP98], with log2FC ranging from 2.73 to 6.57), and 5 were downregulated (filamin B [FLNB], nucleoporin 35 [NUP35], interferon regulatory factor 3 [IRF3], tripartite motif containing 25 [TRIM25], and karyopherin subunit alpha 3 [KPNA3], with log2FC ranging from −1.56 to −6.06) (Figure 6 and Table 4).
Representative proteins were selected from each overrepresented pathway for validation at the transcriptional level. The Fas and casp9 genes were selected as representatives of the apoptosis pathway, the mhcI gene was selected as a representative of antigen processing and presentation of peptide antigens via MHCI, and the irf3 gene was selected as a representative of the ISG15 antiviral mechanism. As shown in Figure 7, the expression levels of these proteins correlated with the RT-qPCR transcript levels.
Figure 7. Relative mRNA and protein expression analysis of IRF3, MHCI, FAS, and CASP9. RBCs from RBIV-infected rock bream compared to PBS-injected rock bream (control). (A) Gene expression analysis, relative to control individuals (red line), evaluated by means of RT-qPCR. The β-actin gene was used as an endogenous control. Bars represent the mean ± standard deviation (SD) (n = 4 individuals). Unpaired T-tests were performed between conditions.*P < 0.05. (B) Quantitative protein expression values of selected proteins for pathway validation from proteomic analysis. Bars indicate log2FC value. FDR values are indicated in Supplementary Table S1.
In this study, we report relevant findings in which RBIV, an economically important virus in rock bream aquaculture production, induce an immune response in RBCs. The spleen is one of the major target organs for RBIV replication (2–4, 7). However, we found similarities in RBIV level patterns in the spleen, kidneys, liver, blood, and RBCs. RBIV copy numbers were not as high as in RBCs as in other organs. Nonetheless, RBIV time-dependent increments were found in rock bream blood or Ficoll-purified RBCs.
Previous microarray analyses of kidney samples from RBIV-infected rock bream have shown that hemoglobin (α and β) expression gradually decreased after RBIV replication reached its maximum levels (around 106 to 107/μL) at 20 to 25 dpi (unpublished data). In contrast, high levels of hemoglobin expression were observed at 70 dpi when low viral loads were detected (below 102/μL) (unpublished data). On the other hand, rock bream individuals treated with poly (I:C) exhibited high expression levels of irf3, isg15, and protein kinase RNA-activated (pkr) genes in blood samples, whereas no significant upregulation was observed in the spleen or kidney (6). Furthermore, the highest mhcI constitutive gene expression was detected in the blood of rock bream compared to other tissues such as spleen or kidney (10). Together, these findings emphasize the importance of evaluating blood-mediated immune responses in rock bream against RBIV infection.
RBCs are the most common cell type in the blood, so understanding their immune response will be essential to identify future strategies for controlling RBIV infection. In the present study, we evaluated the proteome of RBCs from RBIV-infected rock bream. Among the upregulated proteins, the MHCI and apoptosis-related pathways were the most overrepresented in RBCs from RBIV-infected rock bream. MHCI plays a crucial role in the presentation of antigen peptides, which are produced by the degradation of intracellular pathogens. These antigen peptides then bind to MHCI molecules and are presented to CD8+ T lymphocytes to trigger cellular immune responses and induce the elimination of infected or apoptotic cells (35, 36). Apoptosis is a process of programmed cell death known to prevent the transmission of infection to uninfected healthy cells by killing infected cells (37). Cytotoxic lymphocytes (CTL) kill infected cells by 2 main pathways: i) releasing cytolytic granules such as pore-forming protein perforin and serine protease granzymes (38, 39) and ii) activating the caspase-dependent Fas ligand pathway (40, 41). In the present study, antigen processing and presentation of peptide antigen via MHCI was upregulated in RBCs from RBIV-infected rock bream. Simultaneously, FAS and CASP9, two proteins implicated in the caspase-dependent Fas ligand pathway, were upregulated in RBCs from RBIV-infected rock bream. Indeed, it has been reported that cytotoxic effector cells induce apoptosis in response to RBIV infection (11). In addition, perforin- and granzyme-related apoptosis initiation signals have been reported to be activated in the kidneys of RBIV-infected rock bream. However, the authors also reported that the Fas-induced, caspase-dependent apoptosis pathway was barely induced based on only slight increases in fas, casp3, casp8, and casp9 gene expression (11, 13). Conversely, based on our proteomic results, both FAS and CASP9 proteins were upregulated in RBCs from RBIV-infected individuals, indicating that RBIV-activated apoptosis in rock bream RBCs could occur via the caspase-dependent Fas ligand pathway. These results could also suggest that apoptosis-related genes may be differently expressed in kidneys and RBCs. Similarly, we have previously reported that a myristoylated membrane protein (MMP)-based DNA vaccine administered to rock bream triggered differential expression of apoptosis-related genes (including perforin, granzyme, Fas, Fas ligand, and caspases) depending on the tissue analyzed (spleen, kidney, liver, or muscle) (42). In addition, we have observed that other proteins involved in promoting or inducing apoptosis, such as DSP, PAK2, and heat shock protein family A (Hsp70) member 8 (HSPA8) proteins, were highly upregulated in rock bream RBCs upon RBIV infection. The induction of both the antigen processing and presentation via MHCI pathway and the apoptosis-related pathway against RBIV infection may indicate that RBCs attempt to activate CTLs and subsequently trigger them to induce apoptosis by perforin and granzyme production, which are critical factors for the inhibition of RBIV replication (13). Separately, MHCI-induced apoptosis has been also reported during differentiation and activation of certain hematopoietic cells (43).
Surprisingly, in the present study, proteins related to the ISG15 antiviral mechanism such as IRF3, NUP35, and TRIM25 were downregulated in RBCs from RBIV-infected individuals. In general, the first line of defense against viral infection is based on type I interferon (IFN) expression (44). ISG15 is known to play an antiviral role against different viral pathogens [reviewed in (45)]. In fish, the IFN-related immune response, as well as ISG15-related proteins, are known to exhibit an inhibitory effect on viral infections (46–53). In our previous studies, we have found that mx gene expression upregulation occurs soon after viral infection and is maintained in the kidneys of RBIV-infected rock bream at least till 10 dpi (15). However, the expression of the isg15 and pkr genes declined after 4 dpi. Therefore, type I IFN responses induced by RBIV infection seemed to be limited in time and were not able to maintain antiviral responses at later stages, leading to fish mortality (15). Many viruses have developed strategies to counteract the antiviral activity of ISG15 (54). In orange-spotted grouper (Epinephelus coioides) spleen cell line (GS), ISG15 was not significantly upregulated by Singapore grouper iridovirus (SGIV) infection, while it was overexpressed by grouper nervous necrosis virus (GNNV) (45). Moreover, SGIV infection could downregulate the expression of ISG15, IFN and Mx previously induced by poly I:C, suggesting that SGIV was able to counteract the cellular interferon-mediated antiviral activity. In this regard, the authors also speculated that SGIV encoded proteins could play vital roles in preventing ISG15 activity during SGIV infection. To our knowledge, nothing is known about the interactions between RBIV proteins and host innate immune responses, especially those related to IFN or ISG15 pathways proteins. Therefore, in light of evidences, further studies are needed to elucidate RBIV interactions and/or counteracting effects on rock bream innate immune response.
Finally, pathways related to the spliceosome, snRNP assembly, cellular amino acid metabolic processes, and cellular detoxification were differentially regulated in RBCs from RBIV-infected rock bream. In the same way, previous investigations by Nombela et al. have reported the regulation of proteins related to spliceosomal complex and antioxidant/antiviral response in RBCs exposed in vitro to VHSV (23). However, how these mechanisms contribute to rock bream immune response to RBIV remains to be studied.
In summary, we have demonstrated that rock bream RBCs are able to generate a response to RBIV infection. This response was characterized by the upregulation of apoptosis-, MHCI, cellular detoxification-, and spliceosome-related pathways and the downregulation of ISG15 antiviral mechanisms. We have therefore identified novel target proteins in RBCs that will be valuable tools for future studies on the elucidation of RBIV-rock bream interaction mechanisms. These relevant findings will contribute to mitigate an economically important viral disease affecting rock bream aquaculture.
M-HJ performed experiments, analyzed data, and wrote the manuscript. VC performed experiments. SC and MM performed proteomic sequencing. MO-V conceived ideas, analyzed data, oversaw the research, and wrote the manuscript. VC and S-JJ contributed to the preparation of the manuscript.
This research was supported by the European Research Council (ERC Starting Grant GA639249) and by the Basic Science Research Program through the National Research Foundation of Korea (NRF) funded by the Ministry of Science, ICT & Future Planning (2015R1C1A1A01053685).
The authors declare that the research was conducted in the absence of any commercial or financial relationships that could be construed as a potential conflict of interest.
We would like to thank Remedios Torres and Efren Lucas for their technical assistance. The proteomic analysis was performed in the Proteomics Facility of The Spanish National Center for Biotechnology (CNB-CSIC) that belongs to ProteoRed, PRB3-ISCIII, supported by grant PT17/0019. We would also like to thank the two reviewers for their valuable comments and suggestions.
The Supplementary Material for this article can be found online at: https://www.frontiersin.org/articles/10.3389/fimmu.2019.00160/full#supplementary-material
2. Jung SJ, Oh MJ. Iridovirus-like infection associated with high mortalities of striped beakperch, Oplegnathus fasciatus (Temminck et Schlegel), in southern coastal areas of the Korean peninsula. J Fish Dis. (2000) 23:223–6. doi: 10.1046/j.1365-2761.2000.00212.x
3. Jung MH, Jung SJ, Vinay TN, Nikapitiya C, Kim JO, Lee JH, et al. Effects of water temperature on mortality in Megalocytivirus-infected rock bream Oplegnathus fasciatus (Temminck et Schlegel) and development of protective immunity. J Fish Dis. (2015) 38:729–37. doi: 10.1111/jfd.12286
4. Jung MH, Lee J, Jung SJ. Low pathogenicity of FLIV (flounder iridovirus) and the absence of cross-protection between FLIV and RBIV (rock bream iridovirus). J Fish Dis. (2016) 39:1325–33. doi: 10.1111/jfd.12459
5. Jung MH, Lee JH, Ortega-Villaizan M, Perez L, Jung SJ. Protective immunity against Megalocytivirus infection in rock bream (Oplegnathus fasciatus) following CpG ODN administration. Vaccine. (2017) 35:3691–9. doi: 10.1016/j.vaccine.2017.05.073
6. Jung MH, Jung SJ. Protective immunity against rock bream iridovirus (RBIV) infection and TLR3-mediated type I interferon signaling pathway in rock bream (Oplegnathus fasciatus) following poly (I:C) administration. Fish Shellfish Immunol. (2017) 67:293–301. doi: 10.1016/j.fsi.2017.06.026
7. Jung MH, Nikapitiya C, Vinay TN, Lee J, Jung SJ. Rock bream iridovirus (RBIV) replication in rock bream (Oplegnathus fasciatus) exposed for different time periods to susceptible water temperatures. Fish Shellfish Immunol. (2017) 70:731–5. doi: 10.1016/j.fsi.2017.09.038
8. Umasuthan N, Whang I, Kim JO, Oh MJ, Jung SJ, Choi CY, et al. Rock bream (Oplegnathus fasciatus) serpin, protease nexin-1: transcriptional analysis and characterization of its antiprotease and anticoagulant activities. Dev Comp Immunol. (2011) 35:785–98. doi: 10.1016/j.dci.2011.03.013
9. Kwon MG, Kim JW, Park MA, Hwang JY, Choi HS, Kim MC, et al. Microarray analysis of gene expression in peripheral blood leucocytes from rock bream (Oplegnathus fasciatus) after stimulation by LPS, ConA/PMA, and poly I: C. Genes Genom. (2013) 35: 343–53. doi: 10.1007/s13258-012-0001-4
10. Nikapitiya C, Jung SJ, Jung MH, Song JY, Lee J, Lee JH, et al. Identification and molecular characterization of Z/ZE lineage MHC class I heavy chain homologue and β2-microglobulin from rock bream Oplegnathus fasciatus. Fish Pathol. (2014) 49:93–112. doi: 10.3147/jsfp.49.93
11. Jung MH, Nikapitiya C, Song JY, Lee JH, Lee JH, Oh MJ, et al. Gene expression of pro- and anti-apoptotic proteins in rock bream (Oplegnathus fasciatus) infected with Megalocytivirus. (family Iridoviridae). Fish Shellfish Immunol. (2014) 37:122–30. doi: 10.1016/j.fsi.2014.01.012
12. Hong S, Jin JW, Park JH, Kim JK, Jeong HD. Analysis of proinflammatory gene expression by RBIV infection in rock bream, Oplegnathus faciatus. Fish Shellfish Immunol. (2016) 50:317–26. doi: 10.1016/j.fsi.2015.09.002
13. Jung MH, Jung SJ. CpG ODN 1668 induce innate and adaptive immune responses in rock bream (Oplegnathus fasciatus) against rock bream iridovirus (RBIV) infection. Fish Shellfish Immunol. (2017) 69:247–57. doi: 10.1016/j.fsi.2017.08.030
14. Jung MH, Jung SJ. Gene expression regulation of the TLR9 and MyD88-dependent pathway in rock bream against rock bream iridovirus (RBIV) infection. Fish Shellfish Immunol. (2017) 70:507–14. doi: 10.1016/j.fsi.2017.09.036
15. Jung MH, Jung SJ. Innate immune responses against rock bream iridovirus (RBIV) infection in rock bream (Oplegnathus fasciatus) following poly (I:C) administration. Fish Shellfish Immunol. (2017) 71:171–6. doi: 10.1016/j.fsi.2017.10.002
16. Moras M, Lefevre SD, Ostuni MA. From erythroblasts to mature red blood cells: organelle clearance in mammals. Front Physiol. (2017) 8:1076. doi: 10.3389/fphys.2017.01076
17. Glomski CA, Tamburlin J, Chainani M. The phylogenetic odyssey of the erythrocyte. III. Fish, the lower vertebrate experience. Histol Histopathol. (1992) 7:501–28.>
18. Nombela I, Ortega-Villaizan M. Nucleated red blood cells: Immune cell mediators of the antiviral response. PLoS Pathog. (2018) 14:e1006910. doi: 10.1371/journal.ppat.1006910
19. Rodriguez MF, Wiens GD, Purcell MK, Palti Y. Characterization of Toll-like receptor 3 gene in rainbow trout (Oncorhynchus mykiss). Immunogenetics. (2005) 57:510–9. doi: 10.1007/s00251-005-0013-1
20. Passantino L, Altamura M, Cianciotta A, Patruno R, Tafaro A, Jirillo E, et al. Fish immunology. I. Binding and engulfment of Candida albicans by erythrocytes of rainbow trout (Salmo gairdneri Richardson). Immunopharmacol Immunotoxicol. (2002) 24:665–78. doi: 10.1081/IPH-120016050
21. Passantino L, Massaro MA, Jirillo F, Di Modugno D, Ribaud MR, Di Modugno G, et al. Antigenically activated avian erythrocytes release cytokine-like factors: a conserved phylogenetic function discovered in fish. Immunopharmacol Immunotoxicol. (2007) 29:141–52. doi: 10.1080/08923970701284664
22. Dahle MK, Wessel Ø, Timmerhaus G, Nyman IB, Jørgensen SM, Rimstad E, et al. Transcriptome analyses of Atlantic salmon (Salmo salar L.) erythrocytes infected with piscine orthoreovirus (PRV). Fish Shellfish Immunol. (2015) 45:780–90. doi: 10.1016/j.fsi.2015.05.049
23. Nombela I, Puente-Marin S, Chico V, Villena AJ, Carracedo B, Ciordia S, et al. Identification of diverse defense mechanisms in rainbow trout red blood cells in response to halted replication of VHS virus. F1000Research. (2018) 6:1958. doi: 10.12688/f1000research.12985.2
24. Nombela I, Carrion A, Puente-Marin S, Chico V, Mercado L, Perez L, et al. Infectious pancreatic necrosis virus triggers antiviral immune response in rainbow trout red blood cells, despite not being infective. F1000Research. (2017) 6:1968 doi: 10.12688/f1000research.12994.2
25. Workenhe ST, Kibenge MJ, Wright GM, Wadowska DW, Groman DB, et al. Infectious salmon anaemia virus replication and induction of alpha interferon in Atlantic salmon erythrocytes. Virol J. (2008) 5:36. doi: 10.1186/1743-422X-5-36
26. Puente-Marin S, Nombela I, Ciordia S, Mena MC, Chico V, Coll J, et al. In silico functional networks identified in fish nucleated red blood cells by means of transcriptomic and proteomic profiling. Genes. (2018) 9:202. doi: 10.3390/genes9040202
27. Chico V, Puente-Marin S, Nombela I, Ciordia S, Mena MC, Carracedo B, et al. Shape-shifted red blood cells: a novel red blood cell stage?. Cells. (2018) 7:31. doi: 10.3390/cells7040031
28. Nakajima K, Sorimachi M. Biological and physico-chemical properties of the iridovirus isolated from cultured red sea bream, Pagrus major. Fish Pathol. (1994) 29:29–33. doi: 10.3147/jsfp.29.29
29. Dong Y, Weng S, He J, Dong C. Field trial tests of FKC vaccines against RSIV genotype Megalocytivirus in cage-cultured mandarin fish (Siniperca chuatsi) in an inland reservoir. Fish Shellfish Immunol. (2013) 35:1598–603. doi: 10.1016/j.fsi.2013.09.005
30. Bindea G, Mlecnik B, Hackl H, Charoentong P, Tosolini M, Kirilovsky A, et al. ClueGO: a Cytoscape plug-in to decipher functionally grouped gene ontology and pathway annotation networks. Bioinformatics. (2009) 25:1091–3. doi: 10.1093/bioinformatics/btp101
31. Bindea G, Galon J, Mlecnik B. CluePedia Cytoscape plugin: pathway insights using integrated experimental and in silico data. Bioinformatics. (2013) 29:661–3. doi: 10.1093/bioinformatics/btt019
32. Shannon P, Markiel A, Ozier O, Baliga NS, Wang JT, Ramage D, et al. Cytoscape: a software environment for integrated models of biomolecular interaction networks. Genome Res. (2003) 13:2498–504. doi: 10.1101/gr.1239303
33. Gotz S, Garcia-Gomez JM, Terol J, Williams TD, Nagaraj SH, Nueda MJ, et al. High-throughput functional annotation and data mining with the Blast2GO suite. Nucleic Acids Res. (2008) 36:3420–35. doi: 10.1093/nar/gkn176
34. Livak KJ, Schmittgen TD. Analysis of relative gene expression data using real time quantitative PCR and the 2−ΔΔCt method. Methods. (2001) 25:402–8. doi: 10.1006/meth.2001.1262
36. Monac JJ. A molecular model of MHC class-I restricted antigen processing. Immunol Today. (1992) 13:173–9. doi: 10.1016/0167-5699(92)90122-N
37. Sun EW, Shi YF. Apoptosis: the quiet death silences the immune system. Pharmacol Ther. (2001) 92:135–45. doi: 10.1016/S0163-7258(01)00164-4
38. Smyth MJ, Trapani JA. Granzymes: exogenous proteinases that induce target cell apoptosis. Immunol Today. (1995) 16:202–6. doi: 10.1016/0167-5699(95)80122-7
39. Pardo J, Aguilo JI, Anel A, Martin P, Joeckel L, Borner C, et al. The biology of cytotoxic cell granule exocytosis pathway: granzymes have evolved to induce cell death and inflammation. Microbes Infect. (2009) 11:452–9. doi: 10.1016/j.micinf.2009.02.004
40. Suda T, Takahashi T, Golstein P, Nagata S. Molecular cloning and expression of the Fas ligand, a novel member of the tumor necrosis factor family. Cell. (1993) 75:1169–78. doi: 10.1016/0092-8674(93)90326-L
41. Ben-Hur H, Gurevich P, Ben-Arie A, Huszar M, Berman V, Tendler Y, et al. Apoptosis and apoptosis-related proteins (Fas, Fas ligand, bcl-2, p53) in macrophages of human ovarian epithelial tumors. Eur J Gynaecol Oncol. (2000) 21:141–5. doi: 10.3892/or.9.5.977
42. Jung MH, Nikapitiya C, Jung SJ. DNA vaccine encoding myristoylated membrane protein (MMP) of rock bream iridovirus (RBIV) induces protective immunity in rock bream (Oplegnathus fasciatus). Vaccine. (2018) 36:802–10. doi: 10.1016/j.vaccine.2017.12.077
43. Wallén-Ohman M, Larrick JW, Carlsson R, Borrebaeck CA. Ligation of MHC class I induces apoptosis in human pre-B cell lines, in promyelocytic cell lines and in CD40-stimulated mature B cells. Int Immunol. (1997) 9:599–606.>
44. Zhang YB, Gui JF. Molecular regulation of interferon antiviral response in fish. Dev Comp Immunol. (2012) 38:193–202. doi: 10.1016/j.dci.2012.06.003
45. Huang X, Huang Y, Cai J, Wei S, Ouyang Z, Qin Q. Molecular cloning, expression and functional analysis of ISG15 in orange-spotted grouper, Epinephelus coioides. Fish Shellfish Immunol. (2013) 34:1094–102. doi: 10.1016/j.fsi.2013.01.010
46. Chen YM, Su YL, Lin JHY, Yang HL, Chen TY. Cloning of an orange-spotted grouper (Epinephelus coioides) Mx cDNA and characterisation of its expression in response to nodavirus. Fish Shellfish Immunol. (2006) 20:58–71. doi: 10.1016/j.fsi.2005.04.001
47. Larsen R, Røkenes TP, Robertsen B. Inhibition of infectious pancreatic necrosis virus replication by Atlantic salmon Mx1 protein. J Virol. (2004) 78:7938–44. doi: 10.1128/JVI.78.15.7938-7944.2004
48. Lin CH, John JAC, Lin CH, Chang CY. Inhibition of nervous necrosis virus propagation by fish Mx proteins. Biochem Biophys Res Commun. (2006) 351:534–9. doi: 10.1016/j.bbrc.2006.10.063
49. Caipang CMA, Hirono I, Aoki T. In vitro inhibition of fish rhabdoviruses by Japanese flounder, Paralichthys olivaceus Mx. Virology. (2003) 317:373–82. doi: 10.1016/j.virol.2003.08.040
50. Avunje S, Kim WS, Park CS, Oh MJ, Jung SJ. Toll-like receptors and interferon associated immune factors in viral haemorrhagic septicaemia virus-infected olive flounder (Paralichthys olivaceus). Fish Shellfish Immunol. (2011) 31:407–14. doi: 10.1016/j.fsi.2011.06.009
51. Thanasaksiri K, Sakai N, Yamashita H, Hirono I, Kondo H. Influence of temperature on Mx gene expression profiles and the protection of sevenband grouper, Epinephelus septemfasciatus, against red-spotted grouper nervous necrosis virus (RGNNV) infection after poly (I:C) injection. Fish Shellfish Immunol. (2014) 40:441–5. doi: 10.1016/j.fsi.2014.07.035
52. Zhang J, Tang X, Sheng X, Xing J, Zhan W. The influence of temperature on viral replication and antiviral-related genes response in hirame rhabdovirus-infected flounder (Paralichthys olivaceus). Fish Shellfish Immunol. (2017) 68:260–5. doi: 10.1016/j.fsi.2017.07.029
53. Wang W, Zhang M, Xiao ZZ, Sun L. Cynoglossus semilaevis ISG15: a secreted cytokine-like protein that stimulates antiviral immune response in a LRGG motif-dependent manner. PLoS ONE. (2012) 7:e44884. doi: 10.1371/journal.pone.0044884
Keywords: rock bream, RBIV, red blood cells, erythrocyte, proteome, MHC class I, apoptosis, ISG15
Citation: Jung M-H, Chico V, Ciordia S, Mena MC, Jung S-J and Ortega-Villaizan MDM (2019) The Megalocytivirus RBIV Induces Apoptosis and MHC Class I Presentation in Rock Bream (Oplegnathus fasciatus) Red Blood Cells. Front. Immunol. 10:160. doi: 10.3389/fimmu.2019.00160
Received: 28 June 2018; Accepted: 17 January 2019;
Published: 04 March 2019.
Edited by:
Brian Dixon, University of Waterloo, CanadaReviewed by:
Stephanie DeWitte-Orr, Wilfrid Laurier University, CanadaCopyright © 2019 Jung, Chico, Ciordia, Mena, Jung and Ortega-Villaizan. This is an open-access article distributed under the terms of the Creative Commons Attribution License (CC BY). The use, distribution or reproduction in other forums is permitted, provided the original author(s) and the copyright owner(s) are credited and that the original publication in this journal is cited, in accordance with accepted academic practice. No use, distribution or reproduction is permitted which does not comply with these terms.
*Correspondence: Maria Del Mar Ortega-Villaizan, bW9ydGVnYS12aWxsYWl6YW5AdW1oLmVz
Disclaimer: All claims expressed in this article are solely those of the authors and do not necessarily represent those of their affiliated organizations, or those of the publisher, the editors and the reviewers. Any product that may be evaluated in this article or claim that may be made by its manufacturer is not guaranteed or endorsed by the publisher.
Research integrity at Frontiers
Learn more about the work of our research integrity team to safeguard the quality of each article we publish.