- 1Department of Immunology, Institute of Biomedical Sciences, University of São Paulo, São Paulo, Brazil
- 2Department of Preventive Veterinary Medicine and Animal Health, School of Veterinary Medicine and Animal Science, University of São Paulo, São Paulo, Brazil
- 3Department of Clinical Analysis, Toxicology and Food Science, School of Pharmaceutical Sciences of Ribeirao Preto, University of São Paulo, Ribeirao Preto, Brazil
- 4Laboratory of Molecular Virology, Carlos Chagas Institute, Fundação Oswaldo Cruz, Curitiba, Brazil
- 5Institute of Natural and Biological Sciences, Federal University of Triângulo Mineiro, Uberaba, Brazil
- 6Special Laboratory of Applied Toxinology, Butantan Institute, São Paulo, Brazil
- 7Department of Biochemistry and Immunology, School of Medicine of Ribeirao Preto, University of São Paulo, Ribeirao Preto, Brazil
- 8Department de Parasitology, Institute of Biomedical Sciences, University of São Paulo, São Paulo, Brazil
- 9National Institute of Science and Technology in Molecular Entomology, National Council of Scientific and Technological Development (INCT-EM/CNPq), Rio de Janeiro, Brazil
Amblyomma sculptum is an important vector of Rickettsia rickettsii, causative agent of Rocky Mountain spotted fever and the most lethal tick-borne pathogen affecting humans. To feed on the vertebrate host's blood, A. sculptum secretes a salivary mixture, which may interact with skin resident dendritic cells (DCs) and modulate their function. The present work was aimed at depicting the A. sculptum saliva-host DC network and the biochemical nature of the immunomodulatory component(s) involved in this interface. A. sculptum saliva inhibits the production of inflammatory cytokines by murine DCs stimulated with LPS. The fractionation of the low molecular weight salivary content by reversed-phase chromatography revealed active fractions eluting from 49 to 55% of the acetonitrile gradient. Previous studies suggested that this pattern of elution matches with that observed for prostaglandin E2 (PGE2) and the molecular identity of this lipid mediator was unambiguously confirmed by a new high-resolution mass spectrometry methodology. A productive infection of murine DCs by R. rickettsii was demonstrated for the first time leading to proinflammatory cytokine production that was inhibited by both A. sculptum saliva and PGE2, a result also achieved with human DCs. The adoptive transfer of murine DCs incubated with R. rickettsii followed by treatment with A. sculptum saliva or PGE2 did not change the cytokine profile associated to cellular recall responses while IgG2a-specific antibodies were decreased in the serum of these mice. Together, these findings emphasize the role of PGE2 as a universal immunomodulator of tick saliva. In addition, it contributes to new approaches to explore R. rickettsii-DC interactions both in vitro and in vivo.
Introduction
Ticks are hematophagous ectoparasites that transmit a broad range of pathogens, causing several diseases in humans and other animals (1). The physiology of the feeding process is different between the Argasidae (soft ticks) and the Ixodidae (hard ticks) families. While blood meal acquisition by Argasidae ticks is usually fast, taking from minutes to a few hours, Ixodidae ticks remain attached from days to weeks before dropping off the host (1). Blood feeding by ticks is a dynamic event and the salivary secretion has a pivotal role during the process, providing the control of the osmotic balance (2) and presenting a plethora of molecules with antihemostatic, anti-inflammatory, and immunomodulatory properties (3–5). As a consequence of modulating host hemostasis and immune responses, tick saliva may facilitate both transmission and acquisition of pathogens (3), which might correlate with the wide range of pathogens they transmit, including bacteria, viruses, protozoa and helminths (6–8). The phenomenon of pathogen infectivity enhancement caused by tick saliva was called saliva-activated transmission (SAT), a term originally coined to describe the promotion of Thogoto virus transmission by the salivary gland extract (SGE) of Rhipicephalus appendiculatus (9). Henceforward, several studies reported the SAT for many other viruses and bacteria, revealing the role of tick saliva in the increased infectivity of microorganisms in the blood-feeding context (3).
The most lethal among tick-borne diseases affecting humans is Rocky Mountain spotted fever, also known as Brazilian spotted fever, caused by Rickettsia rickettsii (10–14). In Brazil, the southeast region is the most affected (specifically the state of Sao Paulo) which contains the majority of the cases and the highest case-fatality rate (55%) (12, 14). In the Brazilian territory, the confirmed vectors of Rocky Mountain/Brazilian spotted fever, are Amblyomma sculptum [formely Amblyomma cajennense] (15) and Amblyomma aureolatum (12, 16).
During feeding, ticks insert their mouthparts into the skin of the host causing local tissue damage. Skin resident dendritic cells (DCs) work as sensors of the environment by interacting with commensal microorganisms and inflammatory stimuli (17–19). As a result, DCs promote tissue homeostasis (20), tolerance (21–23), and activation of T cell responses during infectious processes (24). The dynamics of tick saliva-DC interactions was first approached by studies showing that Langerhans cells—a major DC population from the epidermis—trap antigens from tick salivary glands (25, 26) and present them to lymphocytes in draining lymph nodes (27). These cells are also associated with tick resistance (28) and were found surrounding tick mouthparts in secondary infestations (29). More recently, a number of studies demonstrated that tick saliva affects the biology of DCs, typically inhibiting their differentiation, maturation, and function (30–35). Indeed, several molecules responsible for DC immunomodulation have been identified and characterized in salivary preparations of Ixodes scapularis (31, 36–39), Rhipicephalus sanguineus sensu lato (40), R. appendiculatus (41) and Ornithodoros moubata (42, 43). However, the identity of the putative molecule(s) present in A. sculptum saliva involved in DC modulation is elusive to date.
In the present work, we demonstrated the immunomodulatory effect of A. sculptum saliva on cytokine production by LPS-stimulated DCs. By employing bioassay-guided fractionation methods associated to a recently developed high-resolution mass spectrometry technique for target lipids, we ultimately characterized PGE2 as the molecule responsible for this biological activity in A. sculptum saliva. In addition, we showed for the first time that A. sculptum saliva and PGE2 inhibit the production of some proinflammatory cytokines induced by R. rickettsii in murine and human DCs. Our results also revealed that both saliva and PGE2 modulate adoptively transferred DCs to induce changes in humoral immune responses to R. rickettsii in vivo.
Materials and Methods
Ethics Statement
All procedures involving vertebrate animals were carried out in accordance with the Brazilian National Law number 11,794 and approved by the Institutional Animal Care and Use Committee from the University of Sao Paulo (protocol numbers 1423/2008, 128/2011 and 55/2015). All procedures involving humans were carried out in accordance with the recommendations of the Brazilian National Health Council, National Research Ethics Commission. The protocol was approved by the Human Research Ethics Committee from Fiocruz (CAAE: 60643816.6.0000.5248). All subjects gave written informed consent in accordance with the Declaration of Helsinki.
Animals
C3H/HePas female mice (Mus musculus), 6–12-week-old, were bred and maintained at the Isogenic Breeding Unit from the Department of Immunology, Institute of Biomedical Sciences, University of Sao Paulo, Sao Paulo, Brazil. New Zealand male rabbits (Oryctolagus cuniculus), 10–12-week-old, were purchased from ANILAB (Paulínia, SP, Brazil) and maintained at the Animal Facility from the Department of Preventive Veterinary Medicine and Animal Health, School of Veterinary Medicine and Animal Science, University of Sao Paulo, Sao Paulo, Brazil.
Ticks and Saliva Collection
Amblyomma sculptum ticks were obtained either from a laboratory colony started with adult ticks collected at Pedreira municipality, Sao Paulo State, Brazil or from the field, collected at Uberaba municipality, Minas Gerais State, Brazil. Larvae, nymphs, and adults were fed on rabbits as previously described (44). Off-host phases were held in an incubator at 25°C and 95% relative humidity. Unless otherwise indicated, adult A. sculptum females were removed from the vertebrate hosts after 7–9 days of attachment, washed in sterile phosphate-buffered saline (PBS), and salivation was induced by injection of pilocarpine (50 mg/mL in 0.7 M NaCl) or dopamine (0.2% in PBS) into the tick hemocoel using a 12.7 × 0.33 mm BD Ultra-Fine™ needle (Becton, Dickinson and Company, Franklin Lakes, NJ, United States) as previously described (45). The saliva was harvested every 10–15 min using a micropipette and transferred to a polypropylene tube kept on ice. Samples were stored at−80°C until use. The concentration of pilocarpine in the saliva samples was determined by mass spectrometry (Accela TSQ Quantum Max) at the Research Center Facility (CEFAP), Institute of Biomedical Sciences, University of Sao Paulo.
R. rickettsii Culture
R. rickettsii, Taiaçu strain (46) were grown until the 5th passage in VERO cells (ATCC™ CCL-81TM, Manassas, VA, United States). When 100% of VERO cells were infected, confirmed by Giménez stain (47), cells were collected, centrifuged at 4,500 g for 10 min and resuspended in sucrose-phosphate-glutamate buffer (48). Aliquots of 200 μL were transferred to cryovials and maintained in liquid nitrogen until use. For the experiments, the cryovials were immersed in water bath at 37°C until complete thawing followed by incubation in liquid nitrogen for 5 min, for cell disruption and bacteria release.
Fractionation of A. sculptum Saliva
Fifty microliters of A. sculptum saliva, collected after 7–9 days of host attachment, were diluted in 450 μL of PBS, and filtrated through a 3-kDa molecular weight cutoff microfilter (Vivaspin 500, Sartorius Biolab Products, Goettingen, NI, Germany), separating the saliva into a low molecular weight (LMW; < 3 kDa) and a high molecular weight (HMW; >3 kDa) fractions. The HMW fraction was diluted again in PBS and filtrated a second time. The resulting HMW and LMW fractions were pooled, sterilized through a 0.22 μm membrane (Millipore Corporation, Billerica, MA, United States), and used in DC cultures described later.
Two hundred microliters of the LMW fraction of A. sculptum saliva were applied onto analytical reversed phase C18 column (Shim-pack Shimadzu VP-ODS, size 250 mm × 4.6 mm, 5 μm), coupled to a ultra fast liquid chromatography (UFLC) system (LC-20AT Prominence, Shimadzu, Japan) previously equilibrated with 2% acetonitrile (ACN) in acidified water (0.05% trifluoroacetic acid). Salivary molecules were eluted with a linear gradient of 2–60% ACN in acidified water over 60 min, at a flow rate of 1 mL/min. Fractions were individually used in DC cultures described later.
Murine Bone Marrow-Derived DC Cultures
Bone marrow cells from C3H/HePas mice were collected from the femur and adjusted to 3 × 106 cells/mL in complete medium [RPMI 1640 medium supplemented with 10% heat-inactivated fetal bovine serum (FBS), 2 mM L-glutamine, 100 U/mL penicillin, 100 μg/mL streptomycin, 2.5 × 105 M 2-mercaptoethanol (all from GIBCO™, Grand Island, NE, United States)] in the presence of 20 ng/mL of murine GM-CSF (Biolegend, San Diego, CA, United States) to induce DC differentiation, as previously described (49). After 4 days, half of the medium was collected and replaced with fresh complete medium containing 40 ng/mL of GM-CSF. On the 7th day of culture, non-adherent cells were collected, washed, resuspended at 106 cells/mL in complete medium and distributed into 96-well plates at 105 cells/well.
For different experiments, cells were preincubated for 1 h with A. sculptum saliva collected after 7–9 days of host attachment (1:50, 1:100, 1:500, and 1:1,000 dilution), pilocarpine (0.5, 0.1, 0.05, 0.01 mM), LMW or HMW saliva fractions (equivalent to a 1:50 dilution of the whole saliva), or 100 nM (final concentration) of commercial PGE2 (Cayman Chemical, Ann Arbor, MI, United States), followed by incubation with 200 ng/mL of ultrapure lipopolysaccharide (LPS; InvivoGen, San Diego, CA, United States) or 106 R. rickettsii at 37°C under 5% CO2.
In another set of experiments, a cell suspension containing 106 cells/mL was prepared, distributed into 96-well plates at 105 cells/well, and preincubated for 1 h with UFLC fractions derived from A. sculptum saliva (equivalent to a 1:50 dilution of the whole saliva) followed by stimulation with LPS (200 ng/mL) at 37°C under 5% CO2.
Cytokines were evaluated in cell-free supernatants collected after 6 h (for TNF-α) or 18 h (for IL-6, IL-12p40 and IL-12p70) by enzyme-linked immunosorbent assay (ELISA) using BD OptEIA™ ELISA Sets (BD Biosciences, San Diego, CA, United States) according to manufacturer's instructions.
For flow cytometry analysis, DCs were preincubated for 1 h with medium only, A. sculptum saliva (1:50 dilution) or PGE2 (100 nM-final concentration), followed by incubation with R. rickettsii for 18 h, as described above. DCs were collected, washed twice in culture medium to remove unbound bacteria, suspended in PBS containing 1% heat-inactivated FBS and stained with fluorochrome-conjugated antibodies against murine CD11c, I-A/I-E (MHC class II), CD40, CD80, and CD86 (BD Biosciences), acquired in a FACSCanto II (BD Biosciences) and analyzed by FlowJo software, version 10.0.7 (Tree Star, Ashland, OR, United States).
Human Monocyte-Derived DC Cultures
Peripheral blood samples were obtained by venipuncture from three healthy donors. Peripheral blood mononuclear cells (PBMC) were obtained by density gradient separation with Ficoll-Paque PLUS, density 1.077 g/mL (GE Healthcare, Chicago, IL, United States). CD14+ cells were purified by positive selection with anti-human CD14 microbeads (Miltenyi Biotec, Auburn, CA, United States) in accordance with the manufacturer's recommendations. For DC differentiation, CD14+ cells were maintained in complete medium containing 25 ng/mL of human IL-4 and 12.5 ng/mL of human GM-CSF (both from PeproTech, Rocky Hill, NJ, United States) and incubated at 37°C under 5% CO2. After 3 days, fresh medium containing IL-4 and GM-CSF was added to the culture. On the 7th day of culture, non-adherent cells were collected, washed and suspended at 106 cells/mL in complete medium. DCs were distributed into 96-well plates at 105 cells/well and preincubated for 1 h with medium only, A. sculptum saliva (1:50 dilution) or commercial PGE2 (100 nM-final concentration), followed by incubation with 106 R. rickettsii at 37°C under 5% CO2. After 24 h, the cell-free supernatants were collected for TNF-α, IL-6, IL-12p70, IL-8, and IL-1β determination by cytometric bead array (CBA-BD Biosciences) according to manufacturer's instructions.
For flow cytometry analysis, cells were recovered with 100 μL of blocking buffer (PBS supplemented with 5% FBS and 1% AB human serum) and incubated for 20 min at room temperature. After centrifugation, cells were stained with fluorochrome-conjugated antibodies against human CD11c, HLA-DR, CD40, CD80, and CD86 (BD Biosciences), acquired in a FACSCanto II (BD Biosciences) and analyzed by FlowJo software, version 10.0.7 (Tree Star).
PGE2 Determination
PGE2 concentration in A. sculptum saliva collected after 3, 5, 7, 9, and 11 days of host attachment or in saliva fractions between 49 and 55% ACN was determined by a PGE2 EIA Kit (Cayman) following the manufacturer's instructions.
Target Lipids Mass Spectrometry
Eicosanoids from saliva and saliva fractions were purified by ACN extraction (liquid/liquid). The solvent was added to the sample (sample:ACN-1:10 v/v) and maintained overnight at −80°C. After, the samples were centrifuged, the organic phase was obtained, dried and resuspended in 50 μL of methanol:water (7:3 v/v). Aliquots of each extracted sample were injected into an LC-MS/MS system equipped with an ESI (electrospray source ionization) operated in negative mode on TripleTOF 5600® mass spectrometer (Sciex, Redwood City, CA, United States). Compounds separation employed a binary gradient with phase A (water:acetonitrile-7:3 v/v; pH 5.8) and phase B (acetonitrile:isopropanol-7:3 v/v) at a flow of 0.6 mL/min in an Ascentis Express C18 column (Supelco, St. Louis, MO, United States). The characterization of PGE2 was performed by the high-resolution multiple-reaction monitoring (MRMHR) method with specific m/z transitions for enabled proper alignment of the detected fragment ions with their respective precursor ions for accurate identification in high-resolution and matching with standards analytes (Cayman) as previously described (50).
Transmission Electron Microscopy
Murine DCs were distributed into 24-well plates at 106 cells/well and incubated for 18 h at 37°C under 5% of CO2 with 107 R. rickettsii, Taiaçu strain (46). Cells were collected and fixed at 4°C with 2% glutaraldehyde and 2% paraformaldehyde in 0.1 M cacodylate buffer, pH 7.4, post-fixed in 1% osmium tetroxide (OsO4) and embedded in Spurr resin. Ultrathin sections of 70 nm were gathered onto copper grids and stained with 2% uranyl acetate for 1 h, then washed in distilled water and stained in 0.5% lead citrate (51). Ultrastructure was examined in a TECNAI G20 200 KV electron microscope (FEI Company, Eindhoven, Netherlands).
Adoptive Transfer of DCs Incubated With R. rickettsii to Mice
Murine DCs were distributed into 24-well plates at 106 cells/well and preincubated with medium only (control), A. sculptum saliva (1:50 dilution) or PGE2 (100 nM-final concentration) for 1 h, followed by incubation with 107 R. rickettsii for 18 h at 37°C under 5% CO2. DCs of each group were collected and washed twice in culture medium to remove unbound bacteria and resuspended in PBS. Mice were injected subcutaneously with 106 DCs of each group in a 100 μL volume. During the first 5 days after DC transfer, animals of each group received a subcutaneous treatment (100 μL at the same site of DC inoculation) as follows: PBS (group “PBS”); A. sculptum saliva 1:50 dilution (group “Saliva”); and 100 nM PGE2 (group “PGE2”). After 30 days, mice were euthanized and the blood (serum separation) and spleen (lymphoproliferation and qPCR) were collected.
Lymphocyte Proliferation and Cytokine Production
Spleens from mice adoptively transferred with DCs were aseptically dissected after euthanasia and spleen cells were separated using a 40 μm cell strainer (Corning Cell Strainer, Corning Incorporated, Durham, NC, United States). A cell suspension was prepared and transferred to 96-well plates at 105 cells/well followed by stimulation with concanavalin A (Con A-0.5 μg/mL final concentration; Sigma-Aldrich, St Louis, MO, United States) or heat-killed R. rickettsii (HKRr−2 × 105 bacteria/well) and incubated at 37°C under 5% CO2. After 48 h, 25 μL of 0.01% resazurin (prepared in complete medium) were added to culture cells and after additional 24 h, the culture absorbance at 570 and 600 nm was determined and used to indirectly evaluate cell proliferation as previously described (31, 36, 49).
In another set of experiments, spleen cells were distributed into 24-well plates at 2.5 × 106 cells/well followed by stimulation with 5 × 106 cells of HKRr/well. After 72 h of incubation at 37°C under 5% CO2, the concentration of IFN-γ and IL-4 was evaluated in cell-free supernatants according to manufacturer's instructions (BD OptEIA™ ELISA Set-BD Biosciences).
IgG1 and IgG2a Determination
Specific IgG1 and IgG2a against R. rickettsii antigens were measured in the serum of mice receiving DC adoptive transfer under different conditions by an in-house ELISA assay. Briefly, 96-well plates were coated with HKRr (equivalent to 106 bacteria/well) diluted in 0.1 M sodium carbonate buffer (pH 9.5) and maintained overnight at 4°C, followed by blocking with PBS containing 1% heat-inactivated FBS for 1 h at room temperature. Serum samples (1:100 dilution) were added and incubated for 2 h. Peroxidase-labeled anti-IgG1 (Invitrogen, Waltham, MA, United States) and -IgG2a (BD Biosciences) detection antibodies (1:1,000 dilution) were added for 1 h and the reaction was developed by addition of the TMB substrate reagent set (BD Biosciences). The reaction was stopped by addition of phosphoric acid [(H3PO4)1 M] and the absorbance at 450 nm was determined and used to qualitatively estimate the amount of each antibody. The blank for each reaction consisted of the same dilutions of serum from mice injected with DCs only.
Statistical Analysis
For the comparison of the experimental groups, analysis of variance (ANOVA) followed by Tukey as a post-test was used. A p ≤ 0.05 was considered statistically significant.
Results
A. sculptum Saliva Inhibits LPS-Induced Proinflammatory Cytokine Production
In order to evaluate the effects of A. sculptum saliva on parameters associated with DC maturation, cells were preincubated with different dilutions of saliva (induced by pilocarpine) and stimulated with LPS. A significant inhibition of LPS-induced TNF-α and IL-6 production by DCs was achieved at all saliva dilutions employed (Figures 1A,B) whereas IL-12p40 production was significantly decreased only at 1:50 dilution (Figure 1C), and IL-12p70 production was decreased at 1:50 and 1:100 dilution (Figure 1D).
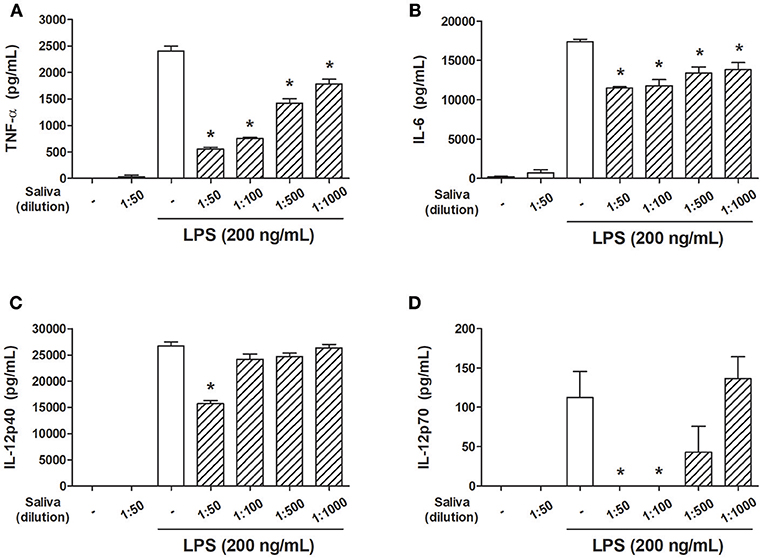
Figure 1. A. sculptum saliva inhibits the production of inflammatory cytokines by LPS-stimulated murine DCs. Bone marrow cells from C3H/HePas mice were differentiated into DCs, seeded at 105 cells/well in 96-well plates and preincubated for 1 h with medium only or with different dilutions of A. sculptum saliva (indicated in the figure) collected by pilocarpine stimulation from female ticks fed on host for 7–9 days. Cells were stimulated with ultrapure LPS (200 ng/mL) during 6 h for TNF-α (A) or 18 h for IL-6, IL-12p40, and IL-12p70 (B–D, respectively) determination in culture supernatants by ELISA. Results are expressed as the mean ± SEM. *p ≤ 0.05 vs. “LPS without saliva” group.
In order to determine whether the presence of pilocarpine in the saliva samples could influence the response of DCs, the concentration of the salivary agonist was determined by mass spectrometry. The quantification of five independent samples indicated a pilocarpine salivary concentration of 1,555.04 ± 513.01 μg/mL (~7.5 mM). In the presence of 0.5 mM pilocarpine (more than 3-fold the equivalent of saliva at 1:50 dilution, which is expected to be around 0.15 mM) we observed a small inhibition of TNF-α production by DCs but not of IL-6, IL-12p40, or IL-12p70. At lower concentrations (0.1, 0.05 and 0.01 mM) no changes in the production of these cytokines were observed in the presence of the agonist (Supplementary Figure S1). In addition, experiments performed in the presence of saliva induced by dopamine revealed a similar inhibitory pattern of all cytokines evaluated when compared with pilocarpine-induced saliva (Supplementary Figure S2). Therefore, both salivary preparations were considered equivalent in terms of their biological activity on dendritic cells, irrespective of the agonist used for salivation.
PGE2 Is the Major DC Inhibitor of A. sculptum Saliva
To characterize the salivary molecule(s) associated to DC immunomodulation, A. sculptum saliva components were initially separated into a LMW fraction (< 3 kDa) and a HMW fraction (> 3 kDa) by microfiltration. DCs were maintained in medium or preincubated with each fraction followed by LPS stimulation. The production of TNF-α was evaluated as a readout parameter of cell activation, since it was the cytokine most inhibited in the presence of saliva (Figure 1A). As observed in the Figure 2A, the LMW fraction significantly inhibited TNF-α production while the HMW fraction did not affect the production of the cytokine. Of note, the LMW fraction also inhibited the production of IL-6, IL-12p40, and IL-12p70 (data not shown).
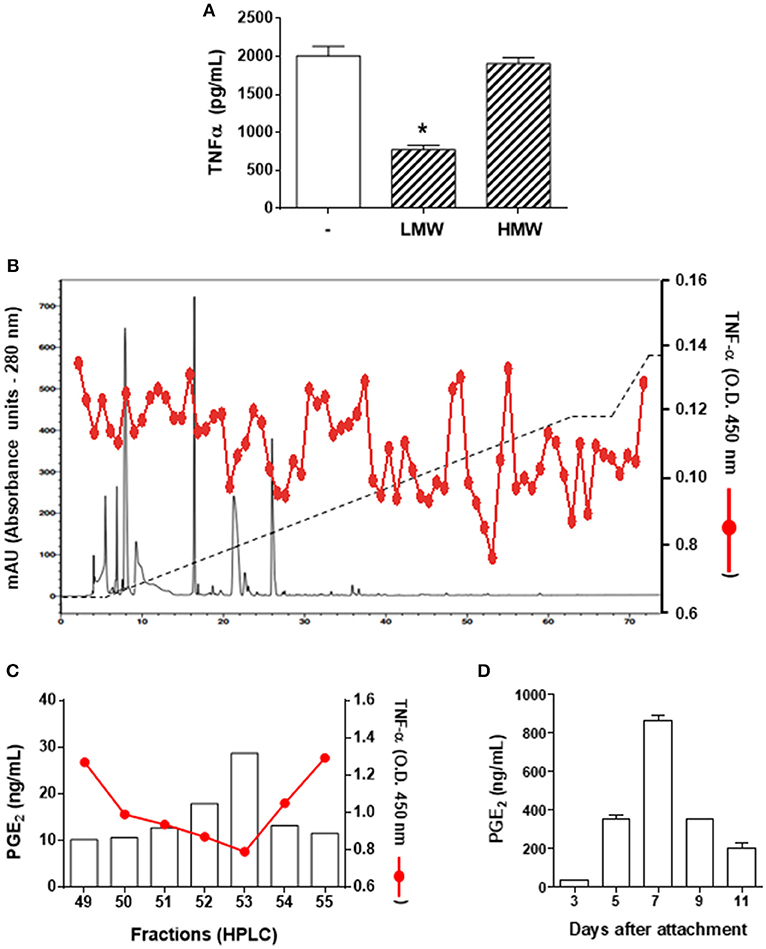
Figure 2. PGE2 is the DC modulatory molecule of A. sculptum saliva. Bone marrow cells from C3H/HePas mice were differentiated into DCs, seeded at 105 cells/well in 96-well plates and preincubated for 1 h with medium only, with a low molecular weight (LMW) or with a high molecular weight (HMW) fractions of A. sculptum saliva collected by pilocarpine stimulation from female ticks fed on host for 7–9 days. Cells were stimulated with ultrapure LPS (200 ng/mL) and after 6 h, the TNF-α production was evaluated in culture supernatants by ELISA (A). The LMW fraction was fractionated by UFLC (solid black line), eluted with a linear gradient of ACN (2–60% -dashed black line). Each fraction was tested for LPS-induced TNF-α production by DCs (solid red line) (B). PGE2 concentration was determined in the fractions presenting stronger inhibition of TNF-α (eluted between 49 to 55% of ACN) by ELISA (C). PGE2 concentration was determined in A. sculptum saliva collected by pilocarpine stimulation from female ticks fed on host for 3, 5, 7, 9, and 11 days (D). *p ≤ 0.05 vs. “LPS without fractions” group.
A. sculptum saliva components were fractionated by reversed phase UFLC and the activity of each fraction was screened on LPS-stimulated DCs, using the TNF-α production as a readout assay. The stronger inhibition of TNF-α production occurred in the presence of fractions eluted between 49 and 55% of ACN (Figure 2B). Previous fractionation approaches of I. scapularis and R. sanguineus saliva employing similar chromatographic conditions identified PGE2 as the DC immunomodulatory molecule in fractions eluted at comparable ACN concentrations (31, 40). Therefore, the presence of PGE2 was evaluated in the fractions presenting TNF-α inhibitory using a commercial PGE2 ELISA kit and confirmed a direct proportional association between the potency of the activity and the concentration of this lipid mediator in the respective fraction (Figure 2C). In addition, the concentration of PGE2 in A. sculptum saliva was increased along the feeding process, reaching the peak on the 7th day after attachment to the host, and decreasing thereafter (Figure 2D).
A recent study explored a new combinatory methodology involving analytical chemistry and molecular biology to describe a precise lipidomic quantitative dataset of eicosanoids in biological samples. This technique identified a cross-reaction of PGE2 and PGD2 when evaluated by commercial ELISA kits (50), thus revealing a potential artifact of previous studies that employed this assay to define the presence of PGE2 in tick saliva. In order to rule out any possible PGD2 contamination in our material (and perhaps in samples produced by similar fractionation methods), we performed a target lipidomics of our samples searching for the specific PGE2 signature. Bioactive fractions were analyzed in an eicosanomics platform using a MRMHR method by an advanced mass spectrometry approach. The information was combined in three dimensions to give a representation of the retention time, signal intensity, and m/z value for the analytes and by using a bioinformatics software for qualitative review of LC-/MS data files. The data processing usually proceeds through multiple steps, including feature detection, which is conducted to identify all signals caused by true ions and to avoid the detection of false positives, thereby interpolating theoretical information from the molecular data file and experimental fragments. In our study, alignment to confirm retention-time differences between runs was conducted through a comparison of the internal standard PGE2-d4 chromatographic peaks. Figure 3A showed the similarity of PGE2-d4 retention time and bioactive PGE2 from A. sculptum salivary fraction on the analytical chromatogram. Indeed, we confirmed the real structure of PGE2 in A. sculptum-saliva by virtual match with a high-resolution fragment ion of a commercial PGE2 standard (Figure 3B). Also, the LC-MS/MS data were in accordance with the biological assays and the PGE2 quantification by an ELISA kit demonstrated in this work.
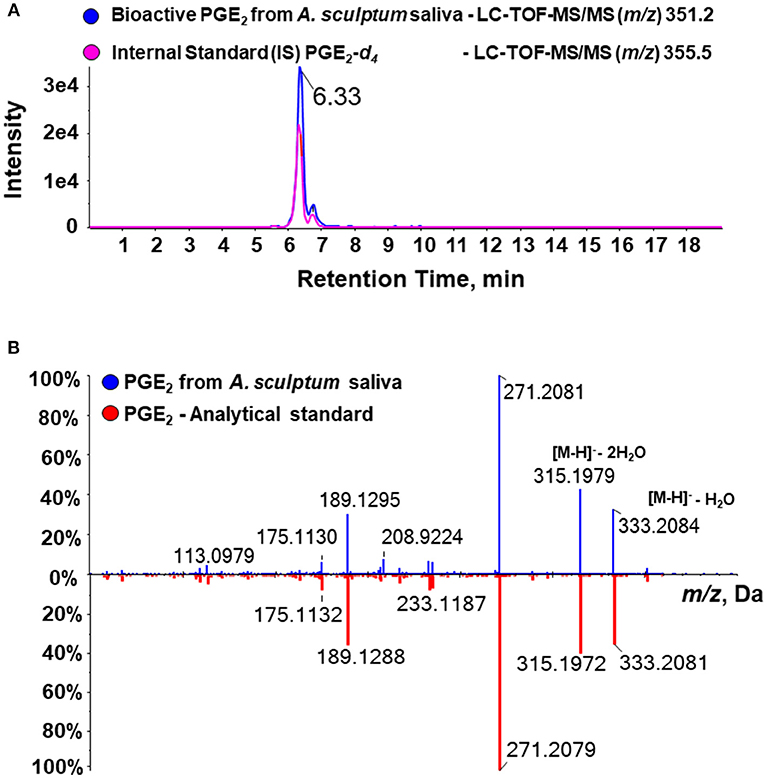
Figure 3. Target high-resolution sequential mass spectrometry for lipids confirms the molecular identity of PGE2 from A. sculptum saliva. Eicosanoids of A. sculptum saliva (collected by pilocarpine stimulation from female ticks fed on host for 7–9 days) and of saliva fractions were extracted and evaluated in an eisosanomics platform. (A) Chromatogram of LC-MS/MS (MRMHR mode) of an internal standard (PGE2-d4 – pink line) and the target bioactive PGE2 (blue line) showing the same retention time. (B) Comparative fragmental spectrum in high-resolution of PGE2 from A. sculptum saliva (blue line) and a commercial PGE2 standard (red line) showing nearly identical m/z profile.
A. sculptum Saliva and PGE2 Inhibit R. rickettsii-Induced Proinflammatory Cytokine Production but Not CD40, CD80, or CD86 Expression by Both Murine and Human DCS
Recent studies reported a murine model of R. rickettsii infection in C3H/HeN mice (52–57). We attempted to infect C3H/HePas (a related strain) with R. rickettsii through a subcutaneous route, but failed to observe susceptibility (data not shown). Thus, we decided to use this murine strain to evaluate immunological parameters associated with the resistance to R. rickettsii infection in vivo, and the putative role of A. sculptum saliva and PGE2 on these parameters. By using transmission electron microscopy, we visualized the ultrastructure of a resting DC maintained in medium only (Figure 4A). The bacteria in the cytosol of the cells confirmed, for the first time, that murine DCs can be infected by R. rickettsii in vitro (Figure 4B, white arrows), similarly to that reported for R. conorii (58).
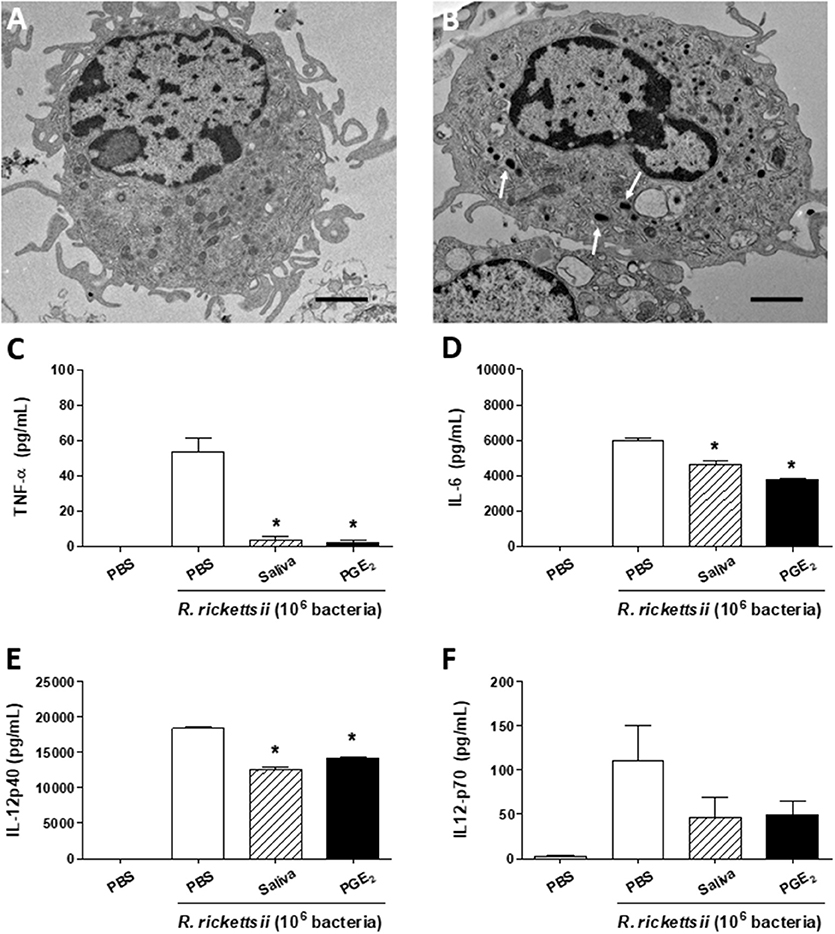
Figure 4. A. sculptum saliva and PGE2 downmodulate inflammatory cytokines produced by murine DCs incubated with R. rickettsii. Bone marrow cells from C3H/HePas mice were differentiated into DCs and used in the following assays. Transmission electron microscopy of a resting DC (A) and a R. rickettsii-infected DC (B), showing the bacteria in the cytosol (white arrows). Non-adherent cells were collected, seeded at 105 cells/well in 96-well plates and preincubated for 1 h with medium only, with A. sculptum saliva collected by pilocarpine stimulation from female ticks fed on host for 7–9 days (1:50 dilution) and PGE2 (100 nM). Cells were incubated with R. rickettsii (106 bacteria) during 6 h for TNF-α (C) or 18 h for IL-6, IL-12p40, and IL-12p70 (D–F, respectively) determination in culture supernatants by ELISA. Results are expressed as the mean ± SEM. *p ≤ 0.05 vs. “DC + R. rickettsii/PBS” group. Scale bar = 2 μM.
When incubated with R. rickettsii, murine DCs produced the proinflammatory cytokines TNF-α, IL-6 and IL-12p40 and IL-12p70 (Figures 4C–F, respectively). In the presence of A. sculptum saliva or PGE2, TNF-α, IL-6, and IL-12p40 were significantly inhibited (Figures 4C–E, respectively), similar to the response observed under LPS stimulation (Figure 1). For IL-12p70, however, the inhibition elicited by saliva and PGE2 did not reach statistical significance (Figure 4F).
The expression of CD40, CD80, and CD86—important maturation/activation markers of DCs and other antigen-presenting cells—was evaluated under similar conditions. DC incubation with R. rickettsii induced a significant expression of the three molecules when compared with cells incubated with medium only. However, the presence of A. sculptum saliva or PGE2 did not downmodulate the R. rickettsii-induced expression of neither of the surface marker on murine DCs (Supplementary Figure S3).
Because R. rickettsii causes an infection with high case-fatality rate (55%) in humans (12, 14), we next evaluate whether the above mentioned cytokine modulation by the bacteria also occurs in human DCs. When incubated with R. rickettsii, human DCs produced several proinflammatory cytokines: TNF-α, IL-6, IL-12p70, IL-8, and IL-1β (Figures 5A–E, respectively). In the presence of A. sculptum saliva or PGE2, only TNF-α (Figure 5A) and IL-12p70 (Figure 5C) were significantly inhibited while IL-6 (Figure 5B), IL-8 (Figure 5D), and IL-1β (Figure 5E) presented a slight inhibition that did not reach statistical significance. The expression of CD40, CD80, and CD86 was also evaluated in the same experimental conditions. For human DCs, the incubation with R. rickettsii, in the presence of absence of saliva and PGE2, did not change the expression of CD40. A mild upregulation of CD80 was observed in the presence of R. rickettsii, which was partially inhibited in the presence of tick saliva. The expression of CD86 was upregulated in the presence of the bacteria, but neither saliva nor PGE2 diminished the expression of this surface marker in human DCs (Supplementary Figure S4).
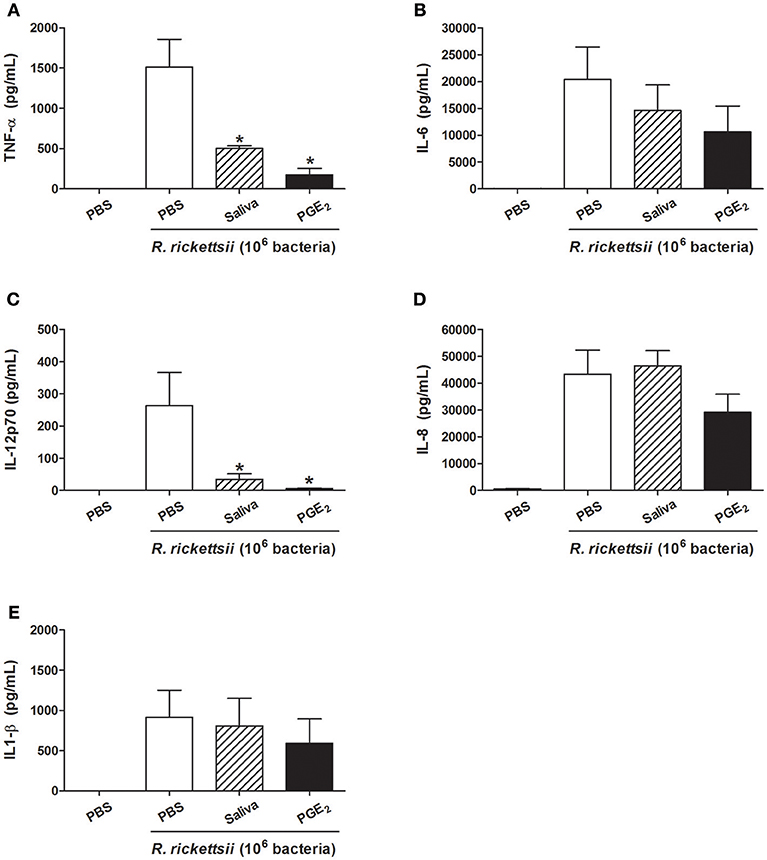
Figure 5. A. sculptum saliva and PGE2 downmodulate inflammatory cytokines produced by human DCs incubated with R. rickettsii. PBMC were differentiated into DCs, seeded at 105 cells/well in 96-well plates and preincubated for 1 h with medium only, with A. sculptum saliva collected by dopamine stimulation from field female ticks (1:50 dilution) and PGE2 (100 nM). After that, cells were incubated with R. rickettsii (106 bacteria) during 24 h for TNF-α (A), IL-6 (B), IL-12p70 (C), IL-8 (D), and IL1-β (E) determination in culture supernatants by CBA. Results are expressed as the mean ± SEM. *p ≤ 0.05 vs. “DC + R. rickettsii/PBS” group.
A. sculptum Saliva and PGE2 Change the Profile of Humoral but Not Cellular Immune Responses to R. rickettsii
Next, murine DC cultures maintained with medium only or treated with PGE2 or A. sculptum saliva were incubated with R. rickettsii and then transferred to mice. For five consecutive days the mice were treated with PBS, A. sculptum saliva or PGE2 as described in Material and Methods. After 30 days, R. rickettsii-specific cellular and humoral responses were evaluated.
When maintained in medium only, spleen cells of mice adoptively transferred with DCs incubated with R. rickettsii and submitted to different treatment protocols produced similar low basal levels of IFN-γ and IL-4 (Figures 6A,B). The in vitro stimulation of these cells with HKRr (recall response) induced a statistically significant increase of IFN-γ production in all three groups when compared to their respective controls, although no differences were observed among these groups (Figure 6A). The stimulation with HKRr also induced a slight increase in the IL-4 production by spleen cells of all groups receiving DCs incubated with R. rickettsii. In comparison with their respective controls, this increase only reached statistical significance in the group receiving DCs incubated with R. rickettsii and treated with PGE2; however, no differences were observed among the experimental groups (Figure 6B). In an attempt to estimate if the different treatments could induce any change in T cell polarization, the IFN-γ/IL-4 ratio was calculated for each group. Despite the increased polarization to the Th1 profile observed in cells stimulated in vitro with HKRr (over 200:1 ratio), no significant differences were observed among the three experimental groups (Figure 6C). Of note, qPCR analysis did not detect bacteria in spleen, suggesting that at this moment of the infection, no bacterial cell was present in mice spleens (data not shown).
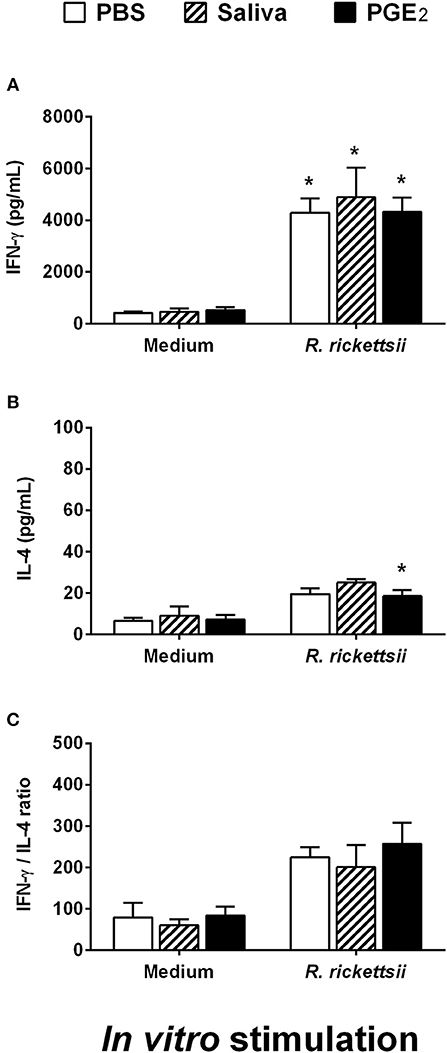
Figure 6. Adoptive transfer of murine DCs incubated with R. rickettsii and treated with A. sculptum saliva or PGE2 does not change IFN-γ or IL-4 recall response of spleen cells following antigenic restimulation in vitro. Bone marrow cells from C3H/HePas mice were differentiated into DCs, seeded at 106 cells/well in 24-well plates and preincubated for 1 h with medium only, A. sculptum saliva collected by pilocarpine stimulation from female ticks fed on host for 7–9 days (1:50 dilution) or PGE2 (100 nM). Cells were incubated with R. rickettsii (107 bacteria) during 18 h, collected and washed to remove unbound bacteria. Mice were injected subcutaneously with 106 DCs of each group in a 100 μL volume. During the first 5 days after DC transfer, animals of each group received a subcutaneous treatment (100 μL at the same site of DC inoculation) as follows: PBS, A. sculptum saliva (1:50 dilution) and PGE2 (100 nM). After 30 days of DC transference, the spleen was collected and the cells were restimulated with medium or HKRr for 72 h. The levels of IFN-γ (A) and IL-4 (B) were evaluated in cell-free supernatant of cultures by ELISA. The IFN-γ/IL-4 ratio is also presented (C). Results are expressed as the mean ± SEM. *p ≤ 0.05 vs. “DC + R. rickettsii/PBS” group.
The serum obtained from mice adoptively transferred with murine DCs incubated with R. rickettsii was evaluated for the presence of specific IgG2a and IgG1 antibodies. Figure 7A shows that specific IgG2a was significantly decreased in the serum of mice adoptively transferred with DCs incubated with R. rickettsii and treated with saliva or PGE2, when compared to the serum of mice treated with PBS (vehicle). On the other hand, specific IgG1 levels were similar among all groups (Figure 7B). The evaluation of IgG2a/IgG1 ratio revealed a negative balance toward IgG2a (below 0.5:1 ratio) in the groups that received DCs incubated with R. rickettsii followed by treatment with A. sculptum saliva or PGE2, when compared with the PBS-treated group (~1:1 ratio; Figure 7C).
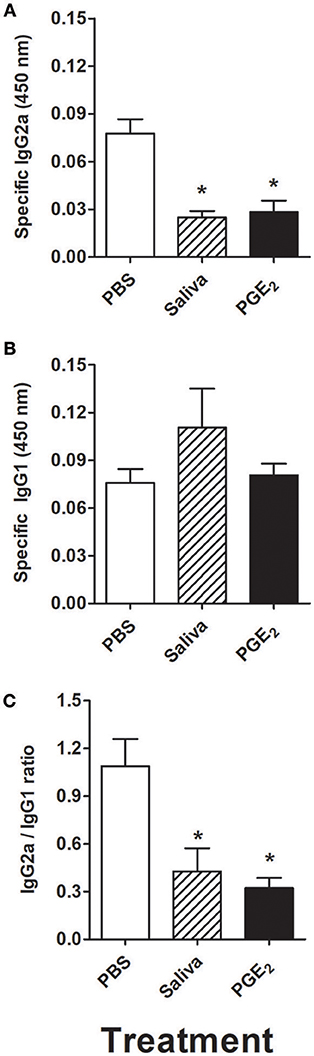
Figure 7. Adoptive transfer of murine DCs incubated with R. rickettsii and treated with A. sculptum saliva or PGE2 decreases specific IgG2a without changing IgG1 levels in serum. Bone marrow cells from C3H/HePas mice were differentiated into DCs, seeded at 106 cells/well in 24-well plates and preincubated for 1 h with medium only, A. sculptum saliva collected by pilocarpine stimulation from female ticks fed on host for 7–9 days (1:50 dilution) or PGE2 (100 nM). Cells were incubated with R. rickettsii (107 bacteria) during 18 h, collected and washed to remove unbound bacteria. Mice were injected subcutaneously with 106 DCs of each group in a 100 μL volume. During the first 5 days after DC transfer, animals of each group received a subcutaneous treatment (100 μL at the same site of DC inoculation) as follows: PBS, A. sculptum saliva (1:50 dilution) and PGE2 (100 nM). After 30 days of DC transference, a blood aliquot was collected and the levels of R. rickettsii-specific IgG2a (A) and IgG1 (B) antibodies were evaluated in serum samples by ELISA. The IgG2a/IgG1 ratio is also presented (C). Results are expressed as the mean ± SEM. *p ≤ 0.05 vs. “DC + R. rickettsii/PBS” group.
Discussion
Several DC populations are intimately associated to the skin immune system and present a number of biological, sometimes contradictory, specialized functions (18, 19, 59). In addition, in the last 40 years, a detailed interaction between DCs and tick saliva has been uncovered by both in vivo and in vitro studies (25–35). However, only two studies evaluated aspects related to the role of A. sculptum saliva in DCs up to date (34, 60), and none of them isolated or characterized the immunomodulatory molecule(s) responsible for the activities described.
In the present work, we demonstrated that A. sculptum saliva is able to decrease the production of inflammatory cytokines by LPS-stimulated DCs, regardless of the agonist employed for salivation (pilocarpine or dopamine). Reversed-phase UFLC fractionation showed that fractions eluting from 49 to 55% of ACN presented inhibitory activity on LPS-induced TNF-α production by DCs. The elution profile matches with PGE2 as demonstrated by previous studies (36, 40). In addition, an ELISA assay showed a direct proportional association between the potency of this activity and the PGE2 concentration in the fractions. However, a recent study on eicosanoid profiling of biological samples revealed that PGE2 and PGD2 cross-react in immunoenzymatic assays (50). In order to ascertain whether PGE2 really is the bioactive salivary molecule found in the present work (and perhaps all the other studies describing this lipid mediator in tick saliva), we employed a new high-resolution target mass spectrometry methodology recently described and validated to unambiguously characterize PGE2 as a major DC modulatory molecule in A. sculptum saliva. Although PGE2 and PGD2 are molecular isomers and present the same fragmentation profile and molecular mass characterization, both analytes can be separated by ultra-high-performance liquid chromatography system in a binary gradient (50) and, consequently, they can be identified and quantified as different lipid compounds in a complex biology mixture. Though a previous study identified the presence of PGE2 in I. scapularis saliva by a shotgun LC-MS approach (31), the method only detected the precursor ion in low resolution and this produced a bias on the presence of the target analyte considering the numbers of isomers in complex biological matrices. Herein, we used an advanced mass spectrometry assay (LC-TOF-MS/MS) in MRMHR for target lipidomics, which identify the molecular mass of precursor ion and all the fragment ions in high-resolution with specific retention time in LC, that give us no doubt about the molecular identity of PGE2 in the A. sculptum saliva.
Some protein modulators of DC biology have been described in tick saliva of different species. The production of inflammatory cytokines by DCs, as well as the expression of DC activation surface markers, among other activities, are inhibited in the presence of salivary Salp15, sialostatin L1 and sialostatin L2 from I. scapularis (36–39), OmC2 from O. moubata (42, 43), Japanin from R. appendiculatus (41), and DsCystatin from Dermacentor silvarum (61). In most cases, the concentration of these molecules employed in these published studies were in the micromolar range, a concentration far superior to the amount of any protein naturally found in tick saliva. In this regard, even considering theoretical differences in the potency of the activity between native and the recombinant proteins, the only DC immunomodulatory molecules identified in tick saliva and confirmed in the salivary cocktail at pharmacological levels are PGE2 and adenosine, which are non-protein molecules (36, 40). Cytokine binding proteins are not likely to influence our findings because all of such molecules described so far were much larger than 3 kDa (62–64). Confirming this assumption, there was a lack of activity of the HMW fraction in LPS-stimulated DCs. In addition, no anti-TNF-α activity was found in saliva of Amblyomma americanum, suggesting that Amblyomma spp. may be devoid of this activity (65). On the other hand, considering that R. rickettsii is transmitted to the host since the first days of tick attachment and that PGE2 concentration in the saliva is relatively low during this initial period, the role of the other DC salivary modulators cannot be ruled out. Thus, the present study reinforces an important role of PGE2 on DC-A. sculptum saliva interactions, acting either additively or synergistically with other protein immunomodulators.
The SAT phenomenon has been reviewed in the context of tick saliva for many pathogens (3, 66). Nonetheless, few studies evaluated the SAT effect through the Amblyomma-Rickettsia association (67–69) and none of them specifically addressed the R. rickettsii interaction with A. sculptum. Experimental models for rickettsial infections that closely reproduce human disease are largely lacking, particularly for R. rickettsii (70, 71), even though a murine model using the C3H/HeN has been successfully used in the last few years to trial potential vaccine candidates to R. rickettsii (52–57, 72). Our attempts to use the C3H/HePas strain failed in generating a model of susceptibility to our R. rickettsii isolate (data not show), although this murine strain is known to be closely related to the C3H/HeN.
Another aspect not appropriately covered by the literature is that few reports characterized the infection of murine DCs by Rickettsia spp. (58, 73–75). Here we showed, for the first time that R. rickettsii is able to infect murine DCs and induce a proinflammatory cytokine profile by these cells. This same proinflammatory phenotype was observed in human DCs incubated with R. rickettsii. We also demonstrated that A. sculptum saliva and PGE2 inhibit this profile in both murine and human DCs, reinforcing their modulatory activity on a rickettsial-DC context. Of note, the R. rickettsii-induced expression of CD40, CD80, and CD86 presented a particular profile for murine and human DCs and was not consistently downmodulated by the presence of saliva or PGE2. This aspect is not covered by the literature and more studies are needed to establish the differences and similarities in the DC responses to rickettsial infection between the two species.
Considering the role of DC as the most important antigen-presenting cell in the skin and other organs (23), the modulation of DC phenotype by tick saliva may have an impact on the in vivo immunity to R. rickettsii. Thus, we assessed whether the adoptive transfer of murine DCs incubated with R. rickettsii and treated with A. sculptum saliva or PGE2 would induce changes in humoral and cellular immune responses against R. rickettsii in C3H/HePas mice. The recall responses showed the production of high levels of IFN-γ and a marginal increase of IL-4 by spleen cells of DC-transferred mice stimulated with HKRr when compared to cells maintained in medium. In fact, IFN-γ is associated to host defenses against rickettsial infections (76, 77) and the IFN-γ-related response is reportedly associated with resistance to the infection in vaccination studies of mice against R. rickettsii (53–55). More recently, protective peptides derived from R. rickettsii antigens were shown to trigger a Th1 dominant response associated with IFN-γ production (56). On the other hand, tick infestation is known to increase the production of Th2 cytokines while inhibiting Th1 cytokines in mice (78, 79), but this polarization seems to be more effective in susceptible than in resistant mice (80), and under natural infection rather than through syringe inoculation (81). In addition, either DCs directly incubated with tick saliva in vitro or DCs isolated from mice treated with tick saliva induced a decrease of IFN-γ and stimulation of IL-4 production by CD4+ T cells (32). In the present study however, the treatment of DCs with A. sculptum saliva or PGE2 prior to incubation with R. rickettsii was not able to alter the IFN-γ/IL-4 ratio systemically developed by the mice injected with these cells.
Regarding the humoral immunity, R. rickettsii-specific IgG2a levels were diminished in the serum of mice receiving DCs incubated with the bacteria and treated with A. sculptum saliva or PGE2 while R. rickettsii-specific IgG1 levels remained unaltered. The role of IL-4 (originally described as a B cell stimulatory factor) in the B cell survival and switch to IgG1 was established more than 30 years ago (82, 83) and may help to explain the persistence of this isotype observed in the presence of saliva and PGE2. Earlier studies characterized murine monoclonal antibodies with protective activity against R. rickettsii and showed that most of them were of IgG2a isotype and none were of IgG1 isotype (84, 85). In addition, the protective properties of IgG2a antibodies against R. rickettsii infection in vivo, acting in synergy to other IFN-γ-dependent mechanisms, are supported by some studies (53–55). Furthermore, because mice were also treated with either saliva or PGE2 after DC transfer, a direct effect of the treatment on antibody class switch cannot be ruled out, as extensively demonstrated by many studies on B cells. For example, PGE2 was shown to promote the differentiation of B lymphocytes into IgG2a- (86), IgG1- and IgE-secreting cells (87–89), while IgM and IgG3 were inhibited in the same conditions (87). The basis of these differences may reside in the differentiation stage of B lymphocytes (e.g., resting vs. activated), the stimuli present in the environment (e.g., cytokines, pathogens or microbial products), the T helper population interacting with B cells and, ultimately, the set of PGE2 receptors expressed by these cells during a given immune response. In addition, there is an interesting hypothesis recently raised in that PGE2 from tick saliva would be the mediator responsible for allergy to red meat found in some individuals exposed to ticks. According to the authors, salivary PGE2 would trigger antibody class switch in type-A and -O individuals to induce anti-α-Gal IgE antibodies that break oral tolerance to food allergens (90). In line with this conjecture, specific IgG2a/IgG1 ratio was decreased following treatment with either A. sculptum saliva or PGE2, but whether these humoral changes are associated with increased susceptibility to R. rickettsii still remains to be determined.
In brief, the present work reinforces the anti-inflammatory role of tick saliva on murine and human DCs and shows that A. sculptum produces pharmacological levels of PGE2 in its salivary secretion. A new and robust mass spectrometry approach confirmed that tick saliva does not contain PGD2, which usually cross react with PGE2 in immunoenzymatic assays. Finally, we evidenced for the first time, the murine DC-R. rickettsii interactions and the ability of PGE2 to drive DC-dependent humoral changes to a rickettsial infection in vivo.
Author Contributions
EE, BB, CLS, and AS-N designed the experiments. EE, AR-H, RT, FC, and AS-N generated biological samples. EE, BB, AP, AC, PW, CAS, and AS-N performed the experiments. EE, BB, AP, AC, CLS, LF, AF, CAS, and AS-N analyzed data. EE, BB, and AS-N performed statistic data analysis. PW, ML, PS, CLS, LF, AF, CAS, AS-N contributed reagents, materials, and analysis tools. EE, BB, AC, PW, AF, CAS, and AS-N wrote the paper. All authors read and approved the final manuscript.
Funding
This work was supported by funds from the Sao Paulo Research Foundation [FAPESP; Grants # 2013/26450-2 (Thematic Project) and # 2015/00658-1 (Multiuser Equipment)], National Institute of Science and Technology in Molecular Entomology, National Council for Scientific and Technological Development (INCT-EM/CNPq; Grant # 465678/2014-9), the Coordination for the Improvement of Higher Education Personnel (CAPES; Finance code 001), and the Research Support Center on Bioactive Molecules from Arthropod Vectors, University of Sao Paulo (NAP-MOBIARVE/USP, Grant # 12.1.17661.1.7).
Conflict of Interest Statement
The authors declare that the research was conducted in the absence of any commercial or financial relationships that could be construed as a potential conflict of interest.
Acknowledgments
We would like to thank Dr. Cláudia Nunes Duarte dos Santos and Dr. Andréia Akemi Suzukawa (Carlos Chagas Institute/Fiocruz-PR) for technical assistance and access to the BSL-3 laboratory for the experiments with human cells and R. rickettsii; Sandra Alexandre (Department of Immunology, Institute of Biomedical Sciences, University of Sao Paulo); Fernando Gonçalves de Almeida and Thais Viggiani Santana (CEFAP, Universidade de Sao Paulo); and to Pedro Cesar Ferreira da Silva (Department of Preventive Veterinary Medicine and Animal Health, School of Veterinary Medicine and Animal Science, University of Sao Paulo) for their technical assistance; Dra. Sirlei Daffre (Department of Parasitology, Institute of Biomedical Sciences, University of Sao Paulo) for providing equipment and reagents used in this work; and Dr. Carlo José Freire de Oliveira for teaching how to collect tick saliva and for the critical reading of the manuscript. The authors also thank to the Program for Technological Development in Tools for Health-PDTIS-FIOCRUZ for the use of their facilities at the Carlos Chagas Institute/Fiocruz-PR.
Supplementary Material
The Supplementary Material for this article can be found online at: https://www.frontiersin.org/articles/10.3389/fimmu.2019.00118/full#supplementary-material
References
2. Bowman AS, Sauer JR. Tick salivary glands: function, physiology and future. Parasitology (2004) 129(Suppl.):S67–81. doi: 10.1017/S0031182004006468
3. Simo L, Kazimirova M, Richardson J, Bonnet SI. The essential role of tick salivary glands and saliva in tick feeding and pathogen transmission. Front Cell Infect Microbiol. (2017) 7:281. doi: 10.3389/fcimb.2017.00281
4. Kotal J, Langhansova H, Lieskovska J, Andersen JF, Francischetti IM, Chavakis T, et al. Modulation of host immunity by tick saliva. J Proteomics (2015) 128:58–68. doi: 10.1016/j.jprot.2015.07.005
5. Francischetti IM, Sa-Nunes A, Mans BJ, Santos IM, Ribeiro JM. The role of saliva in tick feeding. Front Biosci. (2009) 14:2051–88.
7. Dantas-Torres F, Chomel BB, Otranto D. Ticks and tick-borne diseases: a One Health perspective. Trends Parasitol. (2012) 28:437–46. doi: 10.1016/j.pt.2012.07.003
8. Otranto D, Dantas-Torres F, Brianti E, Traversa D, Petric D, Genchi C, et al. Vector-borne helminths of dogs and humans in Europe. Parasit Vectors (2013) 6:16. doi: 10.1186/1756-3305-6-16
9. Nuttall PA, Jones LD. Non-viraemic tickborne virus transmission: mechanism and significance. In: Dusbabek F, Bukva V, editors. Modern Acarology. České Budéjovice: Prague and The Hague, Academia and SPB Academic Publishing (1991). p. 3–6.
10. Dantas-Torres F. Rocky Mountain spotted fever. Lancet Infect Dis. (2007) 7:724–32. doi: 10.1016/S1473-3099(07)70261-X
11. Walker DH. Rickettsiae and rickettsial infections: the current state of knowledge. Clin Infect Dis. (2007) 45(Suppl. 1):S39–44. doi: 10.1086/518145
12. Labruna MB. Ecology of rickettsia in South America. Ann N Y Acad Sci. (2009) 1166:156–66. doi: 10.1111/j.1749-6632.2009.04516.x
13. Angerami RN, Resende MR, Feltrin AF, Katz G, Nascimento EM, Stucchi RS, et al. Brazilian spotted fever: a case series from an endemic area in southeastern Brazil: clinical aspects. Ann N Y Acad Sci. (2006) 1078:252–4. doi: 10.1196/annals.1374.044
14. de Oliveira SV, Guimaraes JN, Reckziegel GC, Neves BM, Araujo-Vilges KM, Fonseca LX, et al. An update on the epidemiological situation of spotted fever in Brazil. J Venom Anim Toxins Incl Trop Dis. (2016) 22:22. doi: 10.1186/s40409-016-0077-4
15. Nava S, Beati L, Labruna MB, Caceres AG, Mangold AJ, Guglielmone AA. Reassessment of the taxonomic status of Amblyomma cajennense (Fabricius, 1787) with the description of three new species, Amblyomma tonelliae n. sp., Amblyomma interandinum n. sp. and Amblyomma patinoi n. sp., and reinstatement of Amblyomma mixtum, and Amblyomma sculptum (Ixodida: Ixodidae). Ticks Tick Borne Dis. (2014) 5:252–76. doi: 10.1016/j.ttbdis.2013.11.004
16. Szabo MP, Pinter A, Labruna MB. Ecology, biology and distribution of spotted-fever tick vectors in Brazil. Front Cell Infect Microbiol. (2013) 3:27. doi: 10.3389/fcimb.2013.00027
17. Naik S, Bouladoux N, Linehan JL, Han SJ, Harrison OJ, Wilhelm C, et al. Commensal-dendritic-cell interaction specifies a unique protective skin immune signature. Nature (2015) 520:104–8. doi: 10.1038/nature14052
18. Pasparakis M, Haase I, Nestle FO. Mechanisms regulating skin immunity and inflammation. Nat Rev Immunol. (2014) 14:289–301. doi: 10.1038/nri3646
19. Malissen B, Tamoutounour S, Henri S. The origins and functions of dendritic cells and macrophages in the skin. Nat Rev Immunol. (2014) 14:417–28. doi: 10.1038/nri3683
20. Seneschal J, Clark RA, Gehad A, Baecher-Allan CM, Kupper TS. Human epidermal Langerhans cells maintain immune homeostasis in skin by activating skin resident regulatory T cells. Immunity (2012) 36:873–84. doi: 10.1016/j.immuni.2012.03.018
21. Hasegawa H, Matsumoto T. Mechanisms of tolerance induction by dendritic cells in vivo. Front Immunol. (2018) 9:350. doi: 10.3389/fimmu.2018.00350
22. Waithman J, Allan RS, Kosaka H, Azukizawa H, Shortman K, Lutz MB, et al. Skin-derived dendritic cells can mediate deletional tolerance of class I-Restricted self-reactive T cells. J Immunol. (2007) 179:4535–41. doi: 10.4049/jimmunol.179.7.4535
23. Worbs T, Hammerschmidt SI, Forster R. Dendritic cell migration in health and disease. Nat Rev Immunol. (2017) 17:30–48. doi: 10.1038/nri.2016.116
24. Lopez-Bravo M, Ardavin C. In vivo induction of immune responses to pathogens by conventional dendritic cells. Immunity (2008) 29:343–51. doi: 10.1016/j.immuni.2008.08.008
25. Allen JR, Khalil HM, Graham JE. The location of tick salivary antigens, complement and immunoglobulin in the skin of guinea-pigs infested with Dermacentor andersoni larvae. Immunology (1979) 38:467–72.
26. Allen JR, Khalil HM, Wikel SK. Langerhans cells trap tick salivary gland antigens in tick-resistant guinea pigs. J Immunol. (1979) 122:563–5.
27. Nithiuthai S, Allen JR. Langerhans cells present tick antigens to lymph node cells from tick-sensitized guinea-pigs. Immunology (1985) 55:157–63.
28. Nithiuthai S, Allen JR. Effects of ultraviolet irradiation on the acquisition and expression of tick resistance in guinea-pigs. Immunology (1984) 51:153–9.
29. Nithiuthai S, Allen JR. Significant changes in epidermal Langerhans cells of guinea-pigs infested with ticks (Dermacentor andersoni). Immunology (1984) 51:133–41.
30. Cavassani KA, Aliberti JC, Dias AR, Silva JS, Ferreira BR. Tick saliva inhibits differentiation, maturation and function of murine bone-marrow-derived dendritic cells. Immunology (2005) 114:235–45. doi: 10.1111/j.1365-2567.2004.02079.x
31. Sá-Nunes A, Bafica A, Lucas DA, Conrads TP, Veenstra TD, Andersen JF, et al. Prostaglandin E2 is a major inhibitor of dendritic cell maturation and function in Ixodes scapularis saliva. J Immunol. (2007) 179:1497–505. doi: 10.4049/jimmunol.179.3.1497
32. Skallova A, Iezzi G, Ampenberger F, Kopf M, Kopecky J. Tick saliva inhibits dendritic cell migration, maturation, and function while promoting development of Th2 responses. J Immunol. (2008) 180:6186–92. doi: 10.4049/jimmunol.180.9.6186
33. Slamova M, Skallova A, Palenikova J, Kopecky J. Effect of tick saliva on immune interactions between Borrelia afzelii and murine dendritic cells. Parasite Immunol. (2011) 33:654–60. doi: 10.1111/j.1365-3024.2011.01332.x
34. Carvalho-Costa T, Mendes M, da Silva M, da Costa T, Tiburcio M, Anhe A, et al. Immunosuppressive effects of Amblyomma cajennense tick saliva on murine bone marrow-derived dendritic cells. Parasit Vectors (2015) 8:22. doi: 10.1186/s13071-015-0634-7
35. Oliveira CJ, Cavassani KA, More DD, Garlet GP, Aliberti JC, Silva JS, et al. Tick saliva inhibits the chemotactic function of MIP-1α and selectively impairs chemotaxis of immature dendritic cells by down-regulating cell-surface CCR5. Int J Parasitol. (2008) 38:705–16. doi: 10.1016/j.ijpara.2007.10.006
36. Sá-Nunes A, Bafica A, Antonelli LR, Choi EY, Francischetti IM, Andersen JF, et al. The immunomodulatory action of sialostatin L on dendritic cells reveals its potential to interfere with autoimmunity. J Immunol. (2009) 182:7422–9. doi: 10.4049/jimmunol.0900075
37. Hovius JW, de Jong MA, den Dunnen J, Litjens M, Fikrig E, van der Poll T, et al. Salp15 binding to DC-SIGN inhibits cytokine expression by impairing both nucleosome remodeling and mRNA stabilization. PLoS Pathog (2008) 4:e31. doi: 10.1371/journal.ppat.0040031
38. Lieskovska J, Palenikova J, Langhansova H, Campos Chagas A, Calvo E, Kotsyfakis M, et al. Tick sialostatins L and L2 differentially influence dendritic cell responses to Borrelia spirochetes. Parasit Vectors (2015) 8:275. doi: 10.1186/s13071-015-0887-1
39. Lieskovska J, Palenikova J, Sirmarova J, Elsterova J, Kotsyfakis M, Campos Chagas A, et al. Tick salivary cystatin sialostatin L2 suppresses IFN responses in mouse dendritic cells. Parasite Immunol (2015) 37:70–8. doi: 10.1111/pim.12162
40. Oliveira CJ, Sa-Nunes A, Francischetti IM, Carregaro V, Anatriello E, Silva JS, et al. Deconstructing tick saliva: non-protein molecules with potent immunomodulatory properties. J Biol Chem. (2011) 286:10960–9. doi: 10.1074/jbc.M110.205047
41. Preston SG, Majtan J, Kouremenou C, Rysnik O, Burger LF, Cabezas Cruz A, et al. Novel immunomodulators from hard ticks selectively reprogramme human dendritic cell responses. PLoS Pathog (2013) 9:e1003450. doi: 10.1371/journal.ppat.1003450
42. Salat J, Paesen GC, Rezacova P, Kotsyfakis M, Kovarova Z, Sanda M, et al. Crystal structure and functional characterization of an immunomodulatory salivary cystatin from the soft tick Ornithodoros moubata. Biochem J. (2010) 429:103–12. doi: 10.1042/BJ20100280
43. Zavasnik-Bergant T, Vidmar R, Sekirnik A, Fonovic M, Salat J, Grunclova L, et al. Salivary tick cystatin OmC2 targets lysosomal Cathepsins S and C in human dendritic cells. Front Cell Infect Microbiol. (2017) 7:288. doi: 10.3389/fcimb.2017.00288
44. Pinter A, Labruna MB, Faccini JL. The sex ratio of Amblyomma cajennense (Acari: Ixodidae) with notes on the male feeding period in the laboratory. Vet Parasitol. (2002) 105:79–88. doi: 10.1016/S0304-4017(01)00650-1
45. Oliveira CJ, Anatriello E, de Miranda-Santos IK, Francischetti IM, Sa-Nunes A, Ferreira BR, et al. Proteome of Rhipicephalus sanguineus tick saliva induced by the secretagogues pilocarpine and dopamine. Ticks Tick Borne Dis. (2013) 4:469–77. doi: 10.1016/j.ttbdis.2013.05.001
46. Pinter A, Labruna MB. Isolation of Rickettsia rickettsii and Rickettsia bellii in cell culture from the tick Amblyomma aureolatum in Brazil. Ann N Y Acad Sci. (2006) 1078:523–9. doi: 10.1196/annals.1374.103
48. Ammerman NC, Beier-Sexton M, Azad AF. Laboratory maintenance of Rickettsia rickettsii. Curr Protoc Microbiol. (2008) Chapter 3:Unit 3A 5. doi: 10.1002/9780471729259.mc03a05s11
49. Bizzarro B, Barros MS, Maciel C, Gueroni DI, Lino CN, Campopiano J, et al. Effects of Aedes aegypti salivary components on dendritic cell and lymphocyte biology. Parasit Vectors (2013) 6:329. doi: 10.1186/1756-3305-6-329
50. Sorgi CA, Zarini S, Martin SA, Sanchez RL, Scandiuzzi RF, Gijon MA, et al. Dormant 5-lipoxygenase in inflammatory macrophages is triggered by exogenous arachidonic acid. Sci Rep. (2017) 7:10981. doi: 10.1038/s41598-017-11496-3
51. Silva JRMC, Staines NA, Parra OM, Hernandez-Blazquez FJ. Experimental studies on the response of the fish (Notothenia coriiceps Richardson, 1844) to parasite (Pseudoterranova decipiens Krabbe, 1878) and other irritant stimuli at Antarctic temperatures. Polar Biol. (1999) 22:417–24.
52. Gong W, Wang P, Xiong X, Jiao J, Yang X, Wen B. Enhanced protection against Rickettsia rickettsii infection in C3H/HeN mice by immunization with a combination of a recombinant adhesin rAdr2 and a protein fragment rOmpB-4 derived from outer membrane protein B. Vaccine (2015) 33:985–92. doi: 10.1016/j.vaccine.2015.01.017
53. Gong W, Qi Y, Xiong X, Jiao J, Duan C, Wen B. Rickettsia rickettsii outer membrane protein YbgF induces protective immunity in C3H/HeN mice. Hum Vaccin Immunother (2015) 11:642–9. doi: 10.1080/21645515.2015.1011572
54. Gong W, Wang P, Xiong X, Jiao J, Yang X, Wen B. Chloroform-methanol Residue of Coxiella burnetii markedly potentiated the specific immunoprotection elicited by a recombinant protein fragment rOmpB-4 derived from outer membrane protein B of Rickettsia rickettsii in C3H/HeN Mice. PLoS ONE (2015) 10:e0124664. doi: 10.1371/journal.pone.0124664
55. Gong W, Xiong X, Qi Y, Jiao J, Duan C, Wen B. Surface protein Adr2 of Rickettsia rickettsii induced protective immunity against Rocky Mountain spotted fever in C3H/HeN mice. Vaccine (2014) 32:2027–33. doi: 10.1016/j.vaccine.2014.02.057
56. Wang P, Xiong X, Jiao J, Yang X, Jiang Y, Wen B, et al. Th1 epitope peptides induce protective immunity against Rickettsia rickettsii infection in C3H/HeN mice. Vaccine (2017) 35:7204–12. doi: 10.1016/j.vaccine.2017.09.068
57. Riley SP, Cardwell MM, Chan YG, Pruneau L, Del Piero F, Martinez JJ. Failure of a heterologous recombinant Sca5/OmpB protein-based vaccine to elicit effective protective immunity against Rickettsia rickettsii infections in C3H/HeN mice. Pathog Dis. (2015) 73:ftv101. doi: 10.1093/femspd/ftv101
58. Fang R, Ismail N, Soong L, Popov VL, Whitworth T, Bouyer DH, et al. Differential interaction of dendritic cells with Rickettsia conorii: impact on host susceptibility to murine spotted fever rickettsiosis. Infect Immun. (2007) 75:3112–23. doi: 10.1128/IAI.00007-07
59. Nestle FO, Di Meglio P, Qin JZ, Nickoloff BJ. Skin immune sentinels in health and disease. Nat Rev Immunol. (2009) 9:679–91. doi: 10.1038/nri2622
60. Monteiro GE, Bechara GH, Franzin AM, de Miranda Santos IK. Antigen-presenting cells in draining lymph nodes of goats repeatedly infested by the Cayenne tick Amblyomma cajennense nymphs. Exp Appl Acarol. (2011) 53:63–9. doi: 10.1007/s10493-010-9380-x
61. Sun T, Wang F, Pan W, Wu Q, Wang J, Dai J. An immunosuppressive tick salivary gland protein DsCystatin interferes with toll-like receptor signaling by downregulating TRAF6. Front Immunol. (2018) 9:1245. doi: 10.3389/fimmu.2018.01245
62. Gillespie RD, Dolan MC, Piesman J, Titus RG. Identification of an IL-2 binding protein in the saliva of the Lyme disease vector tick, Ixodes scapularis. J Immunol. (2001) 166:4319–26. doi: 10.4049/jimmunol.166.7.4319
63. Konik P, Slavikova V, Salat J, Reznickova J, Dvoroznakova E, Kopecky J. Anti-tumour necrosis factor-α activity in Ixodes ricinus saliva. Parasite Immunol. (2006) 28:649–56. doi: 10.1111/j.1365-3024.2006.00899.x
64. Deruaz M, Frauenschuh A, Alessandri AL, Dias JM, Coelho FM, Russo RC, et al. Ticks produce highly selective chemokine binding proteins with antiinflammatory activity. J Exp Med. (2008) 205:2019–31. doi: 10.1084/jem.20072689
65. Rezkova M, Kopecky J. Anti-tumour necrosis factor activity in saliva of various tick species and its appearance during the feeding period. Folia Parasit (2017) 64:2017.032. doi: 10.14411/fp.2017.032
66. Nuttall PA, Labuda M. Tick-host interactions: saliva-activated transmission. Parasitology (2004) 129(Suppl.):S177–89. doi: 10.1017/S0031182004005633
67. Banajee KH, Embers ME, Langohr IM, Doyle LA, Hasenkampf NR, Macaluso KR. Amblyomma maculatum feeding augments Rickettsia parkeri infection in a rhesus macaque model: a pilot study. PLoS ONE (2015) 10:e0135175. doi: 10.1371/journal.pone.0135175
68. Banajee KH, Verhoeve VI, Harris EK, Macaluso KR. Effect of Amblyomma maculatum (Acari: Ixodidae) saliva on the acute cutaneous immune response to Rickettsia parkeri infection in a murine model. J Med Entomol. (2016) 53:1252–60. doi: 10.1093/jme/tjw125
69. Grasperge BJ, Morgan TW, Paddock CD, Peterson KE, Macaluso KR. Feeding by Amblyomma maculatum (Acari: Ixodidae) enhances Rickettsia parkeri (Rickettsiales: Rickettsiaceae) infection in the skin. J Med Entomol. (2014) 51:855–63. doi: 10.1603/ME13248
70. Osterloh A. Immune response against rickettsiae: lessons from murine infection models. Med Microbiol Immunol. (2017) 206:403–17. doi: 10.1007/s00430-017-0514-1
71. Bechach Y, Capo C, Mege JLaR D. Ricketsial diseases: from Rickettsia-arthopod relationships to pathophysiology and animal models. Future Microbiol. (2008) 3:223–36. doi: 10.2217/17460913.3.2.223
72. Meng Y, Xiong X, Qi Y, Duan C, Gong W, Jiao J, et al. Protective immunity against Rickettsia heilongjiangensis in a C3H/HeN mouse model mediated by outer membrane protein B-pulsed dendritic cells. Sci China Life Sci. (2015) 58:287–96. doi: 10.1007/s11427-014-4720-4
73. Jordan JM, Woods ME, Soong L, Walker DH. Rickettsiae stimulate dendritic cells through toll-like receptor 4, leading to enhanced NK cell activation in vivo. J Infect Dis. (2009) 199:236–42. doi: 10.1086/595833
74. Jordan JM, Woods ME, Feng HM, Soong L, Walker DH. Rickettsiae-stimulated dendritic cells mediate protection against lethal rickettsial challenge in an animal model of spotted fever rickettsiosis. J Infect Dis. (2007) 196:629–38. doi: 10.1086/519686
75. Bechelli J, Smalley C, Zhao X, Judy B, Valdes P, Walker DH, et al. MyD88 mediates instructive signaling in dendritic cells and protective inflammatory response during rickettsial infection. Infect Immun. (2016) 84:883–93. doi: 10.1128/IAI.01361-15
76. Valbuena G, Feng HM, Walker DH. Mechanisms of immunity against rickettsiae. New perspectives and opportunities offered by unusual intracellular parasites. Microbes Infect. (2002) 4:625–33. doi: 10.1016/S1286-4579(02)01581-2
77. Walker DH, Valbuena GA, Olano JP. Pathogenic mechanisms of diseases caused by Rickettsia. Ann N Y Acad Sci. (2003) 990:1–11. doi: 10.1111/j.1749-6632.2003.tb07331.x
78. Ferreira BR, Silva JS. Successive tick infestations selectively promote a T-helper 2 cytokine profile in mice. Immunology (1999) 96:434–9. doi: 10.1046/j.1365-2567.1999.00683.x
79. Mejri N, Franscini N, Rutti B, Brossard M. Th2 polarization of the immune response of BALB/c mice to Ixodes ricinus instars, importance of several antigens in activation of specific Th2 subpopulations. Parasite Immunol. (2001) 23:61–9. doi: 10.1046/j.1365-3024.2001.00356.x
80. Zeidner N, Mbow ML, Dolan M, Massung R, Baca E, Piesman J. Effects of Ixodes scapularis and Borrelia burgdorferi on modulation of the host immune response: induction of a TH2 cytokine response in Lyme disease-susceptible (C3H/HeJ) mice but not in disease-resistant (BALB/c) mice. Infect Immun (1997) 65:3100–6.
81. Christe M, Rutti B, Brossard M. Cytokines (IL-4 and IFN-gamma) and antibodies (IgE and IgG2a) produced in mice infected with Borrelia burgdorferi sensu stricto via nymphs of Ixodes ricinus ticks or syringe inoculations. Parasitol Res. (2000) 86:491–6.
82. Vitetta ES, Ohara J, Myers CD, Layton JE, Krammer PH, Paul WE. Serological, biochemical, and functional identity of B cell-stimulatory factor 1 and B cell differentiation factor for IgG1. J Exp Med. (1985) 162:1726–31.
83. Coffman RL, Ohara J, Bond MW, Carty J, Zlotnik A, Paul WE. B cell stimulatory factor-1 enhances the IgE response of lipopolysaccharide-activated B cells. J Immunol. (1986) 136:4538–41.
84. Lange JV, Walker DH. Production and characterization of monoclonal antibodies to Rickettsia rickettsii. Infect Immun. (1984) 46:289–94.
85. Anacker RL, List RH, Mann RE, Hayes SF, Thomas LA. Characterization of monoclonal antibodies protecting mice against Rickettsia rickettsii. J Infect Dis. (1985) 151:1052–60.
86. Stein SH, Phipps RP. Antigen-specific IGG2A production in response to prostaglandin E2, immune complexes, and IFN-gamma. J Immunol. (1991) 147:2500–6.
87. Roper RL, Conrad DH, Brown DM, Warner GL, Phipps RP. Prostaglandin E2 promotes IL-4-induced IgE and IgG1 synthesis. J Immunol. (1990) 145:2644–51.
88. Roper RL, Phipps RP. Prostaglandin E2 and cAMP inhibit B lymphocyte activation and simultaneously promote IgE and IgG1 synthesis. J Immunol. (1992) 149:2984–91.
89. Fedyk ER, Phipps RP. Prostaglandin E2 receptors of the EP2 and EP4 subtypes regulate activation and differentiation of mouse B lymphocytes to IgE-secreting cells. Proc Natl Acad Sci USA. (1996) 93:10978–83.
Keywords: Amblyomma sculptum, tick saliva, PGE2, dendritic cells, Rickettsia rickettsii, immunomodulation
Citation: Esteves E, Bizzarro B, Costa FB, Ramírez-Hernández A, Peti APF, Cataneo AHD, Wowk PF, Timóteo RP, Labruna MB, Silva Junior PI, Silva CL, Faccioli LH, Fogaça AC, Sorgi CA and Sá-Nunes A (2019) Amblyomma sculptum Salivary PGE2 Modulates the Dendritic Cell-Rickettsia rickettsii Interactions in vitro and in vivo. Front. Immunol. 10:118. doi: 10.3389/fimmu.2019.00118
Received: 13 August 2018; Accepted: 15 January 2019;
Published: 04 February 2019.
Edited by:
Peter M. Van Endert, Institut National de la Santé et de la Recherche Médicale (INSERM), FranceReviewed by:
Patricia Anne Nuttall, University of Oxford, United KingdomYochai Wolf, Weizmann Institute of Science, Israel
Copyright © 2019 Esteves, Bizzarro, Costa, Ramírez-Hernández, Peti, Cataneo, Wowk, Timóteo, Labruna, Silva Junior, Silva, Faccioli, Fogaça, Sorgi and Sá-Nunes. This is an open-access article distributed under the terms of the Creative Commons Attribution License (CC BY). The use, distribution or reproduction in other forums is permitted, provided the original author(s) and the copyright owner(s) are credited and that the original publication in this journal is cited, in accordance with accepted academic practice. No use, distribution or reproduction is permitted which does not comply with these terms.
*Correspondence: Anderson Sá-Nunes, c2FudW5lc0B1c3AuYnI=