- 1Department of General, Visceral and Thoracic Surgery, University Medical Center Hamburg-Eppendorf, Hamburg, Germany
- 2Department of Biochemistry and Molecular Cell Biology, University Medical Center Hamburg-Eppendorf, Hamburg, Germany
- 3Immunology and Allergy Unit, Department of Medicine Solna, Karolinska Institute and University Hospital, Stockholm, Sweden
- 4Department of Medicine, University Medical Center Hamburg-Eppendorf, Hamburg, Germany
Dietary habits have a profound impact on intestinal homeostasis and in general on human health. In Western countries, high intake of calories derived from fried products, butter and processed meat is favored over dietary regimens rich in fruits and vegetables. This type of diet is usually referred to as Western-type diet (WTD) and it has been associated with several metabolic and chronic inflammatory conditions of the gastrointestinal tract. In this review, we describe how WTD promotes intestinal and extra-intestinal inflammation and alters mucosal immunity acting on CD4+ T cells in a microbiota-dependent or –independent fashion, ultimately leading to higher susceptibility to infectious and autoimmune diseases. Moreover, summarizing recent findings, we propose how dietary supplementation with fiber and vitamins could be used as a tool to modulate CD4+ T cell phenotype and function, ameliorating inflammation and restoring mucosal homeostasis.
Introduction
In the gastrointestinal (GI) tract, the immune system is constantly under great environmental pressure, continuously facing a wide variety of antigens derived both from intestinal microbiota and from food. Intestinal CD4+ T helper (TH) cells are key mediators of mucosal immunity, and according to their effector functions, they can be divided into different populations, namely TH1, TH2, and TH17, with the TH17 cells being relatively abundant within the GI tract (1–4). Here, different bacterial species dictate whether intestinal CD4+ T cells acquire pro- or anti-inflammatory effector phenotypes (5, 6), highlighting the crucial function of the intestinal microbiota in maintaining mucosal homeostasis. Pro-inflammatory responses driven by TH cells are controlled by different subsets of CD4+ T cells with regulatory capacities, namely Treg and TR1 cells, key players in promoting and maintaining mucosal tolerance to self- and food-related antigens (7–9). However, when mucosal tolerance fails to limit pro-inflammatory immune responses, this results in intestinal inflammation which can lead to the development of immune-mediated inflammatory diseases (IMIDs) such as inflammatory bowel diseases (IBDs). IBDs are among the leading diseases in Western countries (10, 11) and the observation that TH17 cells and TH17-associated cytokines such as IL-17A, IL-17F, and IL-22 are generally enriched in the inflamed mucosa of IBD patients, suggests that TH17 cells drive intestinal inflammation (12, 13). Interestingly, CD4+ T cells, especially TH17 cells, are highly susceptible to components of Western-type diet (WTD) (14, 15), and WTD has been associated with higher incidence of IBDs (16). Moreover, high intake of calories derived from processed meat, butter and fried products, all components of WTD, have been described to instantly alter the composition of the intestinal microbiota, a phenomenon called dysbiosis, toward a lower Bacteroidetes to Firmicutes ratio (17–19). Dysbiosis is also commonly found among patients suffering from IMIDs, including IBDs (20–23). However, despite these strong associations, a direct cause/effect link between WTD and development of IBDs has not yet been proven.
In this review, we discuss how changes in dietary habits favoring WTD affect intestinal immunity by altering composition of the intestinal microbiota and phenotype and functions of effector and regulatory CD4+ T cells. Furthermore, we propose that WTD leads both to higher susceptibility to infections and higher incidence of chronic autoimmune diseases, thus exacerbating intestinal and extra-intestinal inflammation. We support the hypothesis that supplementation of diets with defined products of bacterial or dietary origin can ameliorate WTD-induced inflammation, acting on the effector/regulatory T cell axis and, in turn, restoring intestinal homeostasis (Figure 1). The findings presented in this review are mostly based on murine experiments and are cross-validated in humans, where possible.
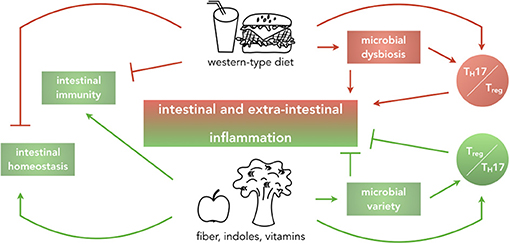
Figure 1. Impact of dietary habits on local and systemic homeostasis and immunity. Graphical abstract summarizing the main findings that this review will discuss. Western-type diets, rich in fat, cholesterol, sugar, and salt are reported to drive intestinal and extra-intestinal inflammation by causing microbial dysbiosis and alteration of the balance of pro- and anti-inflammatory T cells in the intestine, ultimately dampening intestinal immunity and affecting intestinal homeostasis. In contrast, diets enriched in fiber, indoles and vitamins implement beneficial effects on intestinal homeostasis by increasing microbial variety and inducing a regulatory environment.
The Intestinal Immune System and the Microbiota
The intestinal immune system promotes mucosal immunity and maintains tolerance to dietary and microbial antigens, both through its innate and adaptive components located within intestinal epithelia and lamina propria.
In addition to M cells and intraepithelial lymphocytes (IELs), goblet cells, Paneth cells and innate lymphoid type 3 cells (ILC3s) constitute the innate arm of the intestinal immune system. On the other hand, antibody-secreting plasma cells, CD4+ and CD8+ T cells represent the intestinal adaptive immune system. Mucins secreted by goblet cells form the single mucus layer of the small intestine and the two-layered mucus of the colon with the inner layer being impermeable to bacteria (24). ILC3s efficiently contribute to intestinal homeostasis through secretion of IL-17 and IL-22 (25, 26) that instruct Paneth cells to secrete antimicrobial peptides (AMPs) into the intestinal lumen. Although the innate components of the intestinal immune system are fundamental in providing a first line of protection from invading microbes, this review focuses on CD4+ TH cells given their unique role in orchestrating adaptive immune responses, protecting from infections.
Among the different CD4 TH cell subsets, TH17 cells are relatively abundant within the GI tract (27). They are characterized by the expression of the master transcription factor RORγt, the chemokine receptor CCR6 and the transcription factor aryl hydrocarbon receptor (AhR) (28, 29). TH17 cells secrete the highest amount of IL-17 and IL-22, contributing to protection against fungal and bacterial infections, ultimately maintaining mucosal immunity (1). However, the observation that high levels of IL-17 and IL-22 are found in the inflamed mucosa of patients suffering from IBDs, highlights their dualistic role in limiting or promoting intestinal inflammation (12, 13). Complete blockage of IL-17A failed to ameliorate intestinal inflammation in Crohn's disease, which might be explained by preventing the beneficial actions of IL-17A, such as promotion of AMP production that ultimately protects the host against invading microbes (30). In line with this, it has been also shown that IL17-secreting TCRγδ+ T cells mediate gut permeability and exert a protective function on epithelial barrier integrity (31). At the same time IL-17A-deficient T cells have been shown to induce a more aggressive disease outcome in a mouse model of transfer colitis (32). Taken together, these findings suggest that the cellular source of IL-17A production might determine the beneficial or detrimental role of the cytokine itself. Therefore, cell-specific targeting of IL-17A could open new therapeutic approaches. Furthermore, it has been described that TH17 cells are a highly plastic cell population, able to acquire properties typical of other CD4+ T cell subsets (33). Due to their high plasticity, TH17 cells can be either beneficial or detrimental to the host according to the cytokine profile they exhibit in response to inflammatory stimuli. While IL-12 and IL-23 drive the conversion of TH17 cells into pro-inflammatory TH1 cells, inducing acquisition of T-bet and CXCR3 and secretion of IFN-γ (33, 34), exposure to TGF-β and AhR ligands mediate the acquisition of IL-10 secretion from TH17, thus converting them into anti-inflammatory TR1exTH17 cells (35, 36). Characterized by lack of Foxp3 and expression of the co-inhibitory receptors CD49b and LAG-3 (37), both bona fide and TH17-derived TR1 cells (i.e., TR1exTH17) limit expansion of pro-inflammatory TH17 cells in an IL-10-dependent manner (38). In addition, pathogenicity of TH17 cells is also controlled by Foxp3+ Treg cells (38), which, similarly to TR1 and TH17 cells, are relatively abundant in the small intestine, where most of the dietary products are absorbed (9). In summary, pro- and anti-inflammatory CD4+ T cells co-exist within the GI tract being subject to a highly dynamic microenvironment.
An additional layer of complexity to this tight balance of pro- and anti-inflammatory cells is added by the microbiota, whose composition and abundance vary along the GI tract (39–41). It is increasingly recognized that the intestinal microbiota exerts non-redundant functions in the maintenance of homeostasis of the host, ranging from synthesis of nutrients to protection against invading pathogens and modulation of immune responses (42–44). Indeed, studies on germ-free (GF) mice have underlined a higher susceptibility to viral or bacterial infections of mice deprived of their intestinal microbiota as compared to mice housed under specific pathogen free (SPF) conditions (45, 46). This is probably due to the fact that bacterial species dictate the phenotype of CD4+ T cells. For example, Bacteroides fragilis favor differentiation of naïve CD4+ T cells toward IFN-γ-producing TH1 cells (6), while Segmented Filamentous Bacteria (SFB) drives differentiation toward IL-17-secreting TH17 cells (5). Of note, presence of SFB within the intestinal microbiota prevents the growth of pathogenic Citrobacter rodentium, probably due to TH17 induction, ameliorating colonic inflammation (5). These findings highlight once more the dualistic nature of TH17 cells in preventing or exacerbating intestinal inflammation.
Composition of the intestinal microbiota and in turn, intestinal CD4+ T cells are therefore key players in promoting mucosal homeostasis. On the one hand, bacterial species are able to shape intestinal immune functions by modulating CD4+ T cell responses. On the other hand, while TH17 cells mediate immunity to invading microbes, Foxp3+ Treg and TR1 cells maintain tolerance to self and dietary antigens, preventing, as well, uncontrolled TH17 cell-mediated immune responses. Failure to suppress uncontrolled CD4+ TH cell-mediated immune responses may lead to IBDs, as seen in mice lacking critical immunosuppression-associated genes, such as IL-10, which develop spontaneous colitis (47). In agreement, IBDs have been defined by aberrant CD4+ TH cell responses against the commensal microbiota in genetically susceptible hosts (48). How commensal-specific CD4+ TH cell responses develop has been reviewed elsewhere (49).
Dietary Habits in Western Countries
Dietary habits have a profound impact on the lifestyle of individuals. High lipid content in WTD often derived from saturated fatty acids and cholesterol, in addition to excess intake of sugar is linked to higher incidence of colorectal cancer and IMIDs (50–52). Additionally, elevated salt intake and consumption of medium (MCFA) and long chain fatty acids (LCFA), such as lauric and palmitic acids, induce or exacerbate inflammation, acting on the intestinal microbiota, as well as on the innate and adaptive components of the intestinal immune system (53–55).
WTD-favoring dietary habits are also in line with a reduced absorption of vitamins and intake of vegetables and fruits rich in fiber. Dietary fiber consists of non-starch polysaccharides, cellulose, lignin and other plant-derived oligo- or polysaccharides that are not digestible or absorbable in the small intestine (56). It is accepted that diets rich in fiber are beneficial to the host, and dietary regimens favoring consumption of fiber have been associated with a decreased risk of type 2 diabetes (T2D), cardiovascular diseases and intestinal inflammation (57–59). This suggests that fiber can potentially modulate intestinal related and unrelated immune responses. However, how the fiber ameliorates inflammation remains poorly understood. One possible mechanism could reside in its fermentation by bacteria within the colon, which results in the production of short-chain fatty acids (SCFAs) (60, 61). Indeed, acetate, butyrate and propionate, all SCFAs, mediate beneficial effects on the host by engagement of G protein–coupled receptors (GPRs) expressed by a variety of cells, including intestinal CD4+ T cells (62). In addition, recent evidences suggest that the beneficial effects of fiber consumption on the host might reside in the changes it induces in the composition of the intestinal microbiota itself (63).
In short, dietary habits greatly influence human health, modulating function of CD4+ T cells and composition of intestinal microbiota.
WTD Induces Mucosal Inflammation Altering Immunity
Lipids, Cholesterol, and Salt
In this part of the review, we describe the effects that high intake of lipids, cholesterol and salt have on the intestinal immune system, dissecting the complex interplay between adaptive immune cells and the intestinal microbiota. We then summarize recent findings on how WTD-favoring dietary regimens increase susceptibility to chronic autoimmune diseases and infections with commensal bacteria (Table 1). Ultimately, we propose how intestinal and extra-intestinal inflammation driven by WTD can be modulated by supplementation of defined bio-products of microbial or dietary origin, which in turn act on CD4+ T cells.
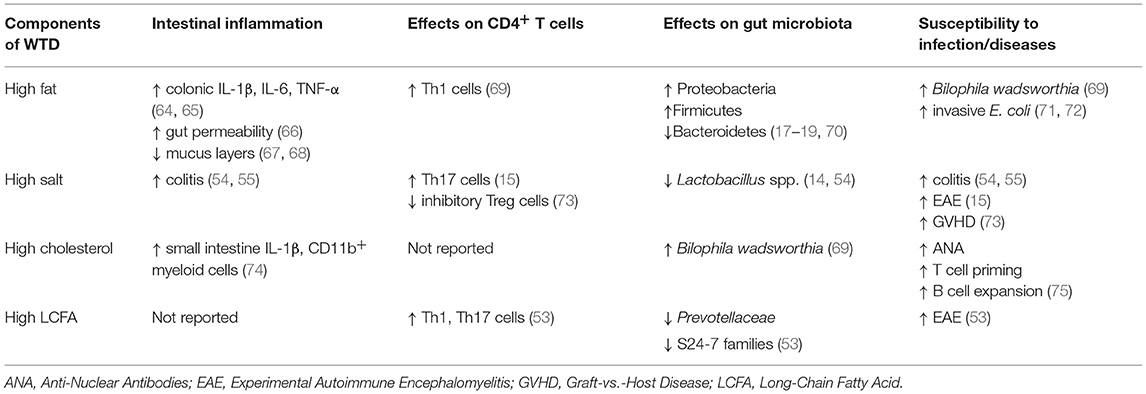
Table 1. Table showing how different components of WTD drive cellular and functional phenotypes associated with intestinal and extra-intestinal inflammation.
In addition to inducing systemic low-grade chronic inflammation typical of obesity (76, 77), WTD promotes local intestinal inflammation through a variety of mechanisms often linked to alteration of the intestinal microbiota composition (i.e., dysbiosis). Figure 2 provides a graphical summary.
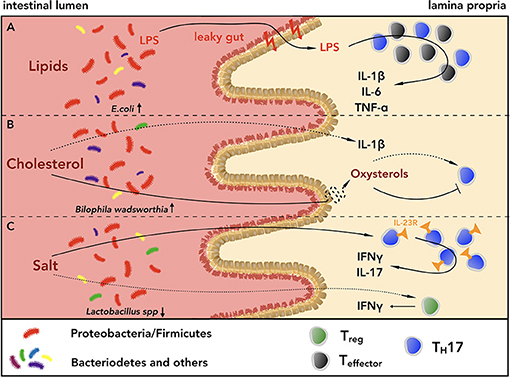
Figure 2. Lipids, cholesterol and salt shape the intestinal CD4+ T cell phenotype in a microbiota-dependent or independent-manner. (A) High intake of lipids induces dysbiosis, shifting the composition of the intestinal microbiota toward a higher ratio of Proteobacteria and Firmicutes to Bacteroidetes. This can lead to higher susceptibility to pathobiont infections, e.g. from invasive E. coli or Bilophila wadsworthia (BW). The mucus layers of both small intestine and colon get thinned, leading to higher gut permeability, which in turn favors the invasion of Gram-negative bacteria, and exacerbates intestinal inflammation. Furthermore, diets rich in saturated fatty acids increase the levels of pro-inflammatory cytokines, such as IL-1β, IL-6, TNF-α, within the gastrointestinal (GI) tract, contributing to the inflammatory state. (B) Diets rich in cholesterol alter the composition of bile acids and increase the levels of IL-1β in the small intestine, creating an inflammatory environment, which can lead to higher susceptibility to infections with BW, a pathogen known to require bile acids to outgrow. Cholesterol metabolites can also modulate intestinal inflammation inhibiting or promoting TH17 cell development through interaction with LXR or RORγt, respectively. (C) Within the WTD-driven intestinal inflammation, salt can alter the phenotype of CD4+ TH17 and Treg cells either directly or indirectly, worsening mucosal homeostasis. Via triggering of serum glucocorticoid kinase-1 (SGK1), salt drives the expression of IL-23R on TH17 cells, inducing their pathogenicity, and it promotes IFN-γ secretion from Treg cells, attenuating their suppressive capacities. Furthermore, high intake of salt can increase the frequencies of pathogenic TH17 cells reducing the amount of Lactobacillus spp. within the GI tract.
High intake of fat increases the levels of IL-1β, IL-6, TNF-α, and NF-κB in the colon (64, 65), resulting in higher concentration of lipocalin 2 (Lcn2) in the feces, a biomarker of intestinal inflammation (78). Thinning of the mucus layers of the small intestine and colon (67, 68) and higher gut permeability (66) lead to increased presence of invading Gram-negative bacteria and higher plasma levels of LPS (79, 80), exacerbating local and systemic inflammation. These findings indicate that the high lipid content of WTD affects mucosal homeostasis, inducing thinning of the protective intestinal mucus layers and thus, increasing gut permeability and levels of pro-inflammatory cytokines.
Similarly, high intake of cholesterol increases levels of IL-1β in the small intestine of mice and frequencies of CD11b+ and CD11c+ cells (74). Along the same line, liver X receptor (LXR)-deficient mice (LXRαβ−/−), which lack the receptor for oxysterols (i.e. cholesterol metabolites), fed for 8 or 16 weeks with WTD showed higher titers of antinuclear antibodies (ANA), increased B cell numbers and augmented TH cell priming, developing a lupus-like autoimmune disease (75). While these findings suggest that metabolism of cholesterol through LXR is crucial for preventing autoimmunity, the effect of excess intake of dietary cholesterol on TH cells still remains unclear. Indeed, oxysterols have been shown to both favor and inhibit TH17 cell differentiation via direct binding to RORγt (81) or engagement of LXR (82) respectively, ascribing a context-dependent beneficial or detrimental role to cholesterol. High cholesterol levels might also increase the production of bile acids (BAs) and high BA concentration in the colon, together with BA malabsorption, has been suggested as a possible cause of diarrhea (83), a condition that is commonly present in patients suffering from IBDs. However, the etiology of diarrhea in IBD patients is still under debate and it can be ascribed to a sum of factors, including intestinal inflammation and disruption of barrier integrity, rather than to one single factor.
In addition to direct effects on CD4+ T cells, diets rich in lipids and cholesterol have been shown to drastically alter the composition of the intestinal microbiota. Of note, phenotype and effector functions of intestinal CD4+ T cells are strictly associated with the different bacterial species of the microbiota, and the intestinal microbiota quickly responds to changes in dietary regimens. Indeed, long-term feeding of mice with WTD shifts the composition of intestinal microbiota toward a higher ratio of Proteobacteria and Firmicutes species over Bacteroidetes (19, 70), leading to higher susceptibility to pathobiont infections by invasive E. coli (71, 72). Similarly, diets rich in saturated milk-derived fat favor the growth of the pathobiont Bilophila wadsworthia (BW) in the colon of IL-10−/− mice, increasing the incidence of spontaneous colitis (69), and SPF mice colonized with BW present higher expression of IL-6 and Serum Amyloid A (SAA), exhibiting systemic inflammation (84). Of note, BW requires bile in its medium to be cultured (85), and diets rich in saturated milk-derived fat increased the amount of taurine conjugated bile acids (TCA) (69), indicating an as-of-yet undefined cross-talk between bile acid composition and intestinal microbiota.
These findings not only show that the intestinal microbiota is highly susceptible to perturbations of dietary regimes, but they also reveal a direct link between WTD, dysbiosis and increased susceptibility to infections and colitis. Further studies are needed to dissect how specific bacterial species act on the different CD4+ T cell populations.
Interestingly, dysbiosis is a common feature of patients suffering from IBDs, which is reflected by lower complexity of microbial species (86) and also exhibit increased frequencies of TH17 cells and amounts of IL-17 and IL-22 as compared to healthy individuals (12, 13).
It is however still unclear whether WTD first alters the composition of the intestinal microbiota that in turn induces pro-inflammatory TH17 cells and promotes intestinal inflammation, or vice versa. Moreover, the exact components of WTD able to target microbiota and/or TH17 cells still remain to be fully identified.
In the last years salt has been given a lot of attention. Wu et al. have shown that serum glucocorticoid kinase-1 (SGK1) drives the expression of IL-23R in TH17 cells via inactivation of Foxo1 (15), and it is known that IL-23R expression on TH17 cells defines their pathogenicity (87, 88). Interestingly, SGK1 has been shown to regulate salt sensing by different cell types, including epithelial colonic cells (89, 90). In their work, Wu et al. showed that mice fed with high salt diet (HSD) for 3 weeks exhibited higher frequencies of lamina propria (LP) TH17 cells as compared to normal chow-fed mice. Furthermore, HSD-fed mice were more susceptible to experimental autoimmune encephalomyelitis (EAE), showing prominent infiltration of TH17 in their central nervous system (CNS) (15). A similar phenotype has been described in mice fed with diets rich in lauric acid (53). Along the same line, HSD-fed mice presented increased intestinal inflammation in IL-10−/− mice (55) and increased severity of Dextran Sulfate Sodium (DSS)- and 2,4-Dinitrobenzene Sulfonic Acid (DNBS)-induced colitis (54). These studies not only indicate that dietary habits favoring WTD act locally inducing intestinal inflammation, but they also suggest a link between dietary regimes and extra-intestinal inflammation. Furthermore, recent evidence in a pilot human study showed that 14 days high salt challenge increased the number of circulating IL-17A- and TNF-α-secreting TH17 cells. This was associated with higher blood pressure, which is a risk factor for atherosclerosis (14). High salt consumption has also been described to induce IFN-γ secretion from human Treg cells, inhibiting their suppressive function both in vitro and in vivo in a SGK1-dependent fashion (73). Taken together, these studies show that salt directly alters phenotype and effector functions of CD4+ T cells in a microbiota-independent manner.
However, salt has also been reported to have a profound impact on the composition of intestinal microbiota, and only indirectly on the effector functions of intestinal TH cells (91).
Two research groups have independently reported that high salt intake decreases the levels of Lactobacillus spp, ultimately favoring inflammation (14, 54). HSD-driven depletion of Lactobacillus murinus (L. murinus) increased the frequencies of LP TH17 cells within small intestine and colon and L. murinus supplementation ameliorated EAE by reducing numbers of TH17 cells within the spinal cord of mice fed with HSD (14). Importantly, colonization of GF mice either with SFB alone or SFB and L. murinus resulted in high or low frequencies of LP TH17 cells, suggesting that L. murinus presence modulates TH17 cells. These findings suggest that dietary habits influence T cell phenotype and their effector functions both in a microbiota-dependent and –independent fashion, determining whether they exhibit protective or pathogenic roles in intestinal immunity.
In addition, HSD has been reported to decrease luminal levels of indole-3-lactic acid (ILA) and butyrate (14, 54). Butyrate promotes the expression of Foxp3, stabilizing the LP Treg phenotype, therefore, its reduction induced by HSD can alter intestinal homeostasis (92).
Taken together, favoring increased lipid, cholesterol and salt consumption leads to alterations of the composition of the intestinal microbiota that in turn affect phenotype and effector function of intestinal CD4+ T cells. This can ultimately result in higher susceptibility to both intestinal and extra-intestinal infections and an increased risk of developing chronic autoimmune diseases.
Fiber, Indoles, and Vitamins
In this part of the review, we describe the effects that WTD-associated low contents of fiber, indoles and vitamins have on adaptive components of the intestinal immune system. Then we propose how supplementation of diets with defined bio-products of bacterial and dietary origin can restore the perturbed intestinal homeostasis. These findings are briefly summarized in Table 2.
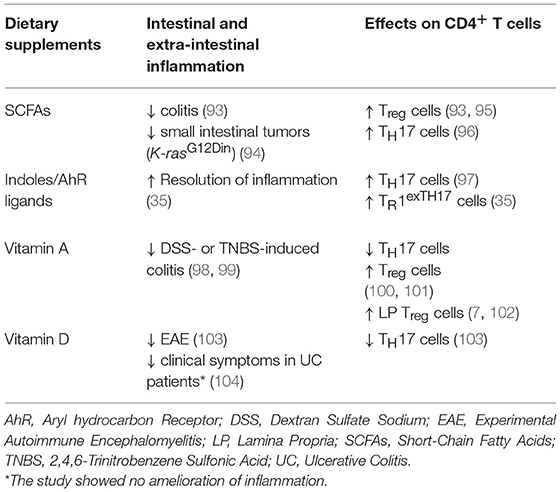
Table 2. Table showing effects that diet supplementation with defined bio-products can have on CD4+ T cell phenotype and on intestinal and extra-intestinal inflammation.
Individuals with low bacterial species diversity have been shown to exhibit higher body mass index (BMI), serum triglyceride, hemoglobin A1c (HbA1c) and C-reactive protein levels as compared to those with higher diversity, indicating a pivotal role of the intestinal microbiota in maintaining metabolic homeostasis (105, 106). Provision of fiber to the microbial community supports its species diversity (107) and diets low in dietary fiber have been associated with intestinal inflammation (108, 109). The beneficial effects of dietary fiber on mucosal homeostasis are graphically summarized in Figure 3. Deprivation of fiber induces proximity of intestinal bacteria to the epithelium by thinning of mucus layers (110), predisposing to pathogenic infections with Citrobacter rodentium (63). Along the same line, mice fed with fiber or inulin alone showed enrichment of SCFA-producing bacteria species, limiting Clostridium difficile growth, thus highlighting the therapeutic potential of fiber supplementation (111). In addition, Kim et al. showed that mice lacking the G-protein coupled receptor GPR43, one of the main receptors for SCFAs in the intestine, exhibited higher susceptibility to pathogenic infections, DSS-induced colitis and Azoxymethane (AOM)/DSS-induced carcinogenesis, all associated with increased frequencies of colonic LP TH17 and decreased frequencies of Treg cells (112, 113). Similarly, in a transfer-colitis model, SCFA supplementation ameliorated intestinal inflammation, increasing Treg cell population in a GPR43-dependent manner (93). Among SCFAs, butyrate has indeed been shown to increase the generation of extra-thymic Treg cells via promoting acetylation of the Foxp3 promoter and the conserved non-coding sequence 1 (CNS1), an enhancer element within Foxp3 locus (95). Butyrate supplementation protected also mice fed with HFD from developing spontaneous small intestinal tumors in the K-rasG12Din model (94). Similarly, acetate administration increased the frequencies of IL-17-producing cells during an active immune response to Citrobacter rodentium, resulting in augmented bacterial clearance (96).
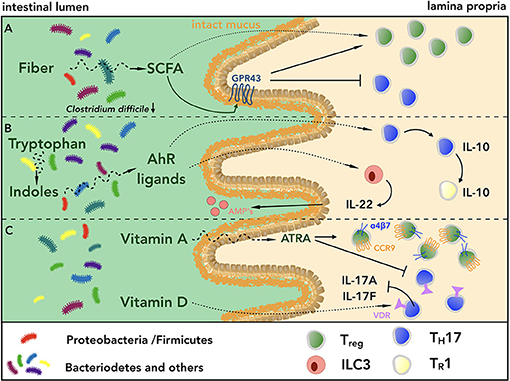
Figure 3. Fiber, indoles and vitamins act in a synergistic fashion promoting intestinal homeostasis. (A) Diets rich in fiber promote bacterial species diversity and short chain fatty acid (SCFA)-producing bacteria, which in turn limit pathogen growth, e.g. Clostridium difficile, and promote barrier integrity. SCFAs also support intestinal homeostasis upon engagement of GPR43 expressed on intestinal epithelial cells. Furthermore, SCFAs can directly interact with intestinal CD4+ T cells, shifting the balance of Treg/TH17 cells toward Treg cells, thus inducing a regulatory microenvironment. (B) Within the lumen of the GI tract, the conversion of indoles to biologically active ligands of AhR contributes to maintain intestinal homeostasis acting on innate and adaptive immune cells. On the one hand, engagement of AhR by its ligands induces secretion of IL-22 from ILC3s, which in turn promotes the production of antimicrobial peptides (AMPs) from Paneth cells. On the other hand, triggering of AhR axis on TH17 cells mediates their conversion into IL-10-secreting TR1 cells, which are in turn able to terminate immune responses. (C) The biologically active form all-trans retinoic acid (ATRA) of vitamin A induces the expression of the gut homing receptors CCR9 and α4β7 integrin on Treg cells, contributing to create a regulatory microenvironment within the GI tract, which is further augmented by the reduction of intestinal TH17 cells. Similarly, engagement of VDR by active vitamin D metabolites dampens IL-17 secretion by TH17 cells.
Taken together, these findings reveal not only the crucial role of SCFAs as mediators of intestinal immunity and mucosal homeostasis through their direct effect on CD4+ T cells, but they indirectly point out also the importance of the presence of SCFA-producing bacteria species within the intestinal microbiota.
Besides being characterized by a low content of fiber, WTD is also poor in fruits and vegetables, which have been shown to have a positive impact on human health (114). Green vegetables, especially belonging to the genus Brassica, contain indoles that are converted to biologically active ligands of AhR (115). AhR is expressed by various intestinal cell types, including IELs, ILC3s and TH17 cells (116, 117). Lack of AhR impairs expression of AMPs, increases gut permeability in DSS-induced colitis and exacerbates immune activation (115). Furthermore, AhR−/− mice exhibit reduction of IL-22-producing ILC3s, leading to higher fitness of SFB that in turn promotes intestinal TH17 cells (117, 118). At the same time, the observation that AhR supports TH17 cell differentiation through interaction with STAT1 (97), suggests that LP TH17 cell development can be mediated by an AhR dependent mechanism. Interestingly, engagement of AhR via 6-Formylindolo[3,2-b]carbazole (FICZ) in in vitro differentiated TH17 cells induces acquisition of IL-10, favoring their conversion into TR1 cells. This indicates that AhR ligands could promote resolution of immune responses (35). It is therefore interesting to speculate that diets rich in green vegetables could limit intestinal inflammation of patients suffering from IBDs via AhR-driven conversion of pro-inflammatory TH17 cells into regulatory TR1 cells. However, this remains to be proven.
Differentiation and stability of the TH17 cell phenotype can also be modulated by vitamins, especially A and D, the contents of which are reduced in WTD. Within the small intestine, dietary vitamin A is converted by CD103+ DCs into the biologically active form all-trans retinoic acid (ATRA) (119, 120). The detailed roles of ATRA in shaping intestinal immunity have been reviewed elsewhere (121). Administration of ATRA has been shown to ameliorate intestinal inflammation in mice suffering from DSS- or TNBS-induced colitis (98, 99), likely by shifting the balance Treg/TH17 in favor of Treg cells (100, 101). Addition of ATRA to TGF-β during Treg cell differentiation has also been shown to augment their capacity to migrate to the LP (7) and their in vivo suppressive capacities in a murine model of transfer-colitis (100). Of note, mice fed with vitamin A-deficient diet (VAD) showed a substantial decrease in the number of LP CD4+ T cells within the small intestine, due to the crucial role of vitamin A in mediating the induction of CCR9 and α4β7 integrin, key gut homing molecules (102). Along the same line, Tejon et al. showed that during intestinal inflammation, in vitro differentiated Treg cells were able to efficiently convert into TH17 cells when transferred into VAD-fed mice, suggesting an anti-inflammatory effect of ATRA (122).
On the one hand, inducing a more regulatory environment within the inflamed mucosa of patients suffering from IBDs via vitamin A supplementation could seem tempting. On the other hand, however, translating its effects in the clinics has been shown to be problematic, and as for now there is no evidence showing beneficial effects of vitamin A supplementation for the health of IBD patients.
Similar to vitamin A, vitamin D content is low in WTD and polymorphisms in the vitamin D receptor (VDR) gene have been associated with higher incidence of IBDs (123). Of note, TH17 cells can be sensitive to vitamin D levels given their expression of VDR. In line with this, high intake of vitamin D has been shown to dampen IL-17A and IL-17F secretion of TH17 cells, ultimately ameliorating clinical manifestations of EAE (103). Similarly, it has been recently reported that clinical disease activity of patients with active ulcerative colitis (UC) improved after weekly supplementation of cholecalciferol (104). However, no changes in intestinal and systemic inflammation were observed, and other clinical trials involving vitamin D supplementation to patients suffering from IBDs did not show substantial improvement of clinical parameters (124).
Taken together, while these findings question a possible therapeutic role of vitamin supplementation alone in ameliorating intestinal inflammation, they highlight the potential of dietary components in modulating the CD4+ T cell phenotype. Among them, SCFAs and AhR ligands could promote intestinal homeostasis and favor mucosal immunity. Translational studies are however required and will eventually shed the light on their efficacy in the clinics.
Conclusions and Perspectives
Evidences on the impact of biologically active dietary components in modulating mucosal immunity and homeostasis are starting to emerge. Western dietary habits favoring high intake of lipids, cholesterol and salt promote local intestinal and extra-intestinal inflammation shaping phenotype and effector functions of CD4+ T cells in a microbiota-dependent or –independent fashion. This can result in altered intestinal immunity, ultimately leading to higher susceptibility to infections caused by intestinal pathogens and increasing the risk for chronic inflammatory autoimmune diseases. The WTD-induced inflammatory state could, however, be potentially reverted by supplementing diets with food rich in fiber and indoles, which represent a promising therapeutic tool to modulate intestinal homeostasis by acting on the TH17/Treg cell axis and restoring SCFA-producing bacteria species.
Author Contributions
FS and NS wrote the manuscript and prepared tables and figures. EV and SH edited the manuscript. NG supervised and edited the manuscript.
Funding
This work was supported by the ERC (StG 715271 to NG and StG 337215 to SH).
Conflict of Interest Statement
The authors declare that the research was conducted in the absence of any commercial or financial relationships that could be construed as a potential conflict of interest.
References
1. Korn T, Bettelli E, Oukka M, Kuchroo VK. IL-17 and Th17 cells. Ann Rev Immunol. (2009) 27:485–517. doi: 10.1146/annurev.immunol.021908.132710
2. Luckheeram RV, Zhou R, Verma AD, Xia B. CD4(+) T cells: differentiation and functions. Clin Dev Immunol. (2012) 2012:925135. doi: 10.1155/2012/925135
3. Mosmann TR, Coffman RL. TH1 and TH2 cells: different patterns of lymphokine secretion lead to different functional properties. Ann Rev Immunol. (1989) 7:145–73. doi: 10.1146/annurev.iy.07.040189.001045
4. Romagnani S. Th1 and Th2 in human diseases. Clin Immunol Immunopathol. (1996) 80:225–35. doi: 10.1006/clin.1996.0118
5. Ivanov II, Atarashi K, Manel N, Brodie EL, Shima T, Karaoz U, et al. Induction of intestinal Th17 cells by segmented filamentous bacteria. Cell (2009) 139:485–98. doi: 10.1016/j.cell.2009.09.033
6. Mazmanian SK, Liu CH, Tzianabos AO, Kasper DL. An immunomodulatory molecule of symbiotic bacteria directs maturation of the host immune system. Cell (2005) 122:107–18. doi: 10.1016/j.cell.2005.05.007
7. Cassani B, Villablanca EJ, Quintana FJ, Love PE, Lacy-Hulbert A, Blaner WS, et al. Gut-tropic T cells that express integrin alpha4beta7 and CCR9 are required for induction of oral immune tolerance in mice. Gastroenterology (2011) 141:2109–18. doi: 10.1053/j.gastro.2011.09.015
8. Coombes JL, Siddiqui KR, Arancibia-Carcamo CV, Hall J, Sun CM, Belkaid Y, et al. A functionally specialized population of mucosal CD103+ DCs induces Foxp3+ regulatory T cells via a TGF-beta and retinoic acid-dependent mechanism. J Exp Med. (2007) 204:1757–64. doi: 10.1084/jem.20070590
9. Kim KS, Hong SW, Han D, Yi J, Jung J, Yang BG, et al. Dietary antigens limit mucosal immunity by inducing regulatory T cells in the small intestine. Science (2016) 351:858–63. doi: 10.1126/science.aac5560
10. El-Gabalawy H, Guenther LC, Bernstein CN. Epidemiology of immune-mediated inflammatory diseases: incidence, prevalence, natural history, and comorbidities. J Rheumatol Suppl. (2010) 85:2–10. doi: 10.3899/jrheum.091461
11. Teng MW, Bowman EP, McElwee JJ, Smyth MJ, Casanova JL, Cooper AM, et al. IL-12 and IL-23 cytokines: from discovery to targeted therapies for immune-mediated inflammatory diseases. Nat Med. (2015) 21:719–29. doi: 10.1038/nm.3895
12. Fujino S, Andoh A, Bamba S, Ogawa A, Hata K, Araki Y, et al. Increased expression of interleukin 17 in inflammatory bowel disease. Gut (2003) 52:65–70. doi: 10.1136/gut.52.1.65
13. Seiderer J, Elben I, Diegelmann J, Glas J, Stallhofer J, Tillack C, et al. Role of the novel Th17 cytokine IL-17F in inflammatory bowel disease (IBD): upregulated colonic IL-17F expression in active Crohn's disease and analysis of the IL17F p.His161Arg polymorphism in IBD. Inflamm Bowel Dis. (2008) 14:437–45. doi: 10.1002/ibd.20339
14. Wilck N, Matus MG, Kearney SM, Olesen SW, Forslund K, Bartolomaeus H, et al. Salt-responsive gut commensal modulates TH17 axis and disease. Nature (2017) 551:585–9. doi: 10.1038/nature24628
15. Wu C, Yosef N, Thalhamer T, Zhu C, Xiao S, Kishi Y, et al. Induction of pathogenic TH17 cells by inducible salt-sensing kinase SGK1. Nature (2013) 496:513–7. doi: 10.1038/nature11984
16. Chapman-Kiddell CA, Davies PS, Gillen L, Radford-Smith GL. Role of diet in the development of inflammatory bowel disease. Inflamm Bowel Dis. (2010) 16:137–51. doi: 10.1002/ibd.20968
17. Cani PD, Bibiloni R, Knauf C, Waget A, Neyrinck AM, Delzenne NM, et al. Changes in gut microbiota control metabolic endotoxemia-induced inflammation in high-fat diet-induced obesity and diabetes in mice. Diabetes (2008) 57:1470–81. doi: 10.2337/db07-1403
18. Turnbaugh PJ, Ley RE, Mahowald MA, Magrini V, Mardis ER, Gordon JI. An obesity-associated gut microbiome with increased capacity for energy harvest. Nature (2006) 444:1027–31. doi: 10.1038/nature05414
19. Zhang C, Zhang M, Pang X, Zhao Y, Wang L, Zhao L. Structural resilience of the gut microbiota in adult mice under high-fat dietary perturbations. ISME J. (2012) 6:1848–57. doi: 10.1038/ismej.2012.27
20. Cox AJ, West NP, Cripps AW. Obesity, inflammation, and the gut microbiota. Lancet Diabetes Endocrinol. (2015) 3:207–15. doi: 10.1016/S2213-8587(14)70134-2
21. Frank DN, Robertson CE, Hamm CM, Kpadeh Z, Zhang T, Chen H, et al. Disease phenotype and genotype are associated with shifts in intestinal-associated microbiota in inflammatory bowel diseases. Inflamm Bowel Dis. (2011) 17:179–84. doi: 10.1002/ibd.21339
22. Maeda Y, Kurakawa T, Umemoto E, Motooka D, Ito Y, Gotoh K, et al. Dysbiosis contributes to arthritis development via activation of autoreactive T cells in the intestine. Arthritis Rheumatol. (2016) 68:2646–61. doi: 10.1002/art.39783
23. Ott SJ, Musfeldt M, Wenderoth DF, Hampe J, Brant O, Folsch UR, et al. Reduction in diversity of the colonic mucosa associated bacterial microflora in patients with active inflammatory bowel disease. Gut (2004) 53:685–93. doi: 10.1136/gut.2003.025403
24. Pelaseyed T, Bergstrom JH, Gustafsson JK, Ermund A, Birchenough GM, Schutte A, et al. The mucus and mucins of the goblet cells and enterocytes provide the first defense line of the gastrointestinal tract and interact with the immune system. Immunol Rev. (2014) 260:8–20. doi: 10.1111/imr.12182
25. Cella M, Fuchs A, Vermi W, Facchetti F, Otero K, Lennerz JK, et al. A human natural killer cell subset provides an innate source of IL-22 for mucosal immunity. Nature (2009) 457:722–5. doi: 10.1038/nature07537
26. Takatori H, Kanno Y, Watford WT, Tato CM, Weiss G, Ivanov II, et al. Lymphoid tissue inducer-like cells are an innate source of IL-17 and IL-22. J Exp Med. (2009) 206:35–41. doi: 10.1084/jem.20072713
27. Ivanov II, Frutos Rde L, Manel N, Yoshinaga K, Rifkin DB, Sartor RB, et al. Specific microbiota direct the differentiation of IL-17-producing T-helper cells in the mucosa of the small intestine. Cell Host Microbe (2008) 4:337–49. doi: 10.1016/j.chom.2008.09.009
28. Annunziato F, Cosmi L, Liotta F, Maggi E, Romagnani S. The phenotype of human Th17 cells and their precursors, the cytokines that mediate their differentiation and the role of Th17 cells in inflammation. Int Immunol. (2008) 20:1361–8. doi: 10.1093/intimm/dxn106
29. Veldhoen M, Hirota K, Westendorf AM, Buer J, Dumoutier L, Renauld JC, et al. The aryl hydrocarbon receptor links TH17-cell-mediated autoimmunity to environmental toxins. Nature (2008) 453:106–9. doi: 10.1038/nature06881
30. Hueber W, Sands BE, Lewitzky S, Vandemeulebroecke M, Reinisch W, Higgins PD, et al. Secukinumab, a human anti-IL-17A monoclonal antibody, for moderate to severe Crohn's disease: unexpected results of a randomised, double-blind placebo-controlled trial. Gut (2012) 61:1693–700. doi: 10.1136/gutjnl-2011-301668
31. Lee JS, Tato CM, Joyce-Shaikh B, Gulen MF, Cayatte C, Chen Y, et al. Interleukin-23-Independent IL-17 Production Regulates Intestinal Epithelial Permeability. Immunity (2015) 43:727–38. doi: 10.1016/j.immuni.2015.09.003
32. O'Connor WJr, Kamanaka M, Booth CJ, Town T, Nakae S, Iwakura Y, et al. A protective function for interleukin 17A in T cell-mediated intestinal inflammation. Nat Immunol. (2009) 10:603–9. doi: 10.1038/ni.1736
33. Annunziato F, Cosmi L, Santarlasci V, Maggi L, Liotta F, Mazzinghi B, et al. Phenotypic and functional features of human Th17 cells. J Exp Med. (2007) 204:1849–61. doi: 10.1084/jem.20070663
34. Tanaka K, Martinez GJ, Yan X, Long W, Ichiyama K, Chi X, et al. Regulation of pathogenic T Helper 17 cell differentiation by steroid receptor coactivator-3. Cell Rep. (2018) 23:2318–29. doi: 10.1016/j.celrep.2018.04.088
35. Gagliani N, Amezcua Vesely MC, Iseppon A, Brockmann L, Xu H, Palm NW, et al. Th17 cells transdifferentiate into regulatory T cells during resolution of inflammation. Nature (2015) 523:221–5. doi: 10.1038/nature14452
36. Heinemann C, Heink S, Petermann F, Vasanthakumar A, Rothhammer V, Doorduijn E, et al. IL-27 and IL-12 oppose pro-inflammatory IL-23 in CD4+ T cells by inducing Blimp1. Nat Commun. (2014) 5:3770. doi: 10.1038/ncomms4770
37. Gagliani N, Magnani CF, Huber S, Gianolini ME, Pala M, Licona-Limon P, et al. Coexpression of CD49b and LAG-3 identifies human and mouse T regulatory type 1 cells. Nat Med. (2013) 19:739–46. doi: 10.1038/nm.3179
38. Huber S, Gagliani N, Esplugues E, O'Connor WJr, Huber FJ, Chaudhry A, et al. Th17 cells express interleukin-10 receptor and are controlled by Foxp3(-) and Foxp3+ regulatory CD4+ T cells in an interleukin-10-dependent manner. Immunity (2011) 34:554–65. doi: 10.1016/j.immuni.2011.01.020
39. Gill SR, Pop M, Deboy RT, Eckburg PB, Turnbaugh PJ, Samuel BS, et al. Metagenomic analysis of the human distal gut microbiome. Science (2006) 312:1355–9. doi: 10.1126/science.1124234
40. Li J, Jia H, Cai X, Zhong H, Feng Q, Sunagawa S, et al. An integrated catalog of reference genes in the human gut microbiome. Nat Biotechnol. (2014) 32:834–41. doi: 10.1038/nbt.2942
41. Sender R, Fuchs S, Milo R. Revised estimates for the number of human and bacteria cells in the body. PLoS Biol. (2016) 14:e1002533. doi: 10.1371/journal.pbio.1002533
42. Backhed F, Ley RE, Sonnenburg JL, Peterson DA, Gordon JI. Host-bacterial mutualism in the human intestine. Science (2005) 307:1915–20. doi: 10.1126/science.1104816
43. Belkaid Y, Hand TW. Role of the microbiota in immunity and inflammation. Cell (2014) 157:121–41. doi: 10.1016/j.cell.2014.03.011
44. Hill DA, Artis D. Intestinal bacteria and the regulation of immune cell homeostasis. Ann Rev Immunol. (2010) 28:623–67. doi: 10.1146/annurev-immunol-030409-101330
45. Inagaki H, Suzuki T, Nomoto K, Yoshikai Y. Increased susceptibility to primary infection with Listeria monocytogenes in germfree mice may be due to lack of accumulation of L-selectin+ CD44+ T cells in sites of inflammation. Infect Immunity (1996) 64:3280–7.
46. Zachar Z, Savage DC. Microbial interference and colonization of the murine gastrointestinal tract by Listeria monocytogenes. Infect Immunity (1979) 23:168–74.
47. Kuhn R, Lohler J, Rennick D, Rajewsky K, Muller W. Interleukin-10-deficient mice develop chronic enterocolitis. Cell (1993) 75:263–74. doi: 10.1016/0092-8674(93)80068-P
48. Peloquin JM, Goel G, Villablanca EJ, Xavier RJ. Mechanisms of pediatric inflammatory bowel disease. Ann Rev Immunol. (2016) 34:31–64. doi: 10.1146/annurev-immunol-032414-112151
49. Sorini C, Cardoso RF, Gagliani N, Villablanca EJ. Commensal bacteria-specific CD4+ T cell responses in health and disease. Front Immunol. (2018) 9:2667. doi: 10.3389/fimmu.2018.02667
50. Amre DK, D'Souza S, Morgan K, Seidman G, Lambrette P, Grimard G, et al. Imbalances in dietary consumption of fatty acids, vegetables, and fruits are associated with risk for Crohn's disease in children. Am J Gastroenterol. (2007) 102:2016–25. doi: 10.1111/j.1572-0241.2007.01411.x
51. Loftus EV Jr. (2004). Clinical epidemiology of inflammatory bowel disease: incidence, prevalence, and environmental influences. Gastroenterology 126, 1504–1517. doi: 10.1053/j.gastro.2004.01.063
52. Nystrom M, Mutanen M. Diet and epigenetics in colon cancer. World J Gastroenterol. (2009) 15:257–63. doi: 10.3748/wjg.15.257
53. Haghikia A, Jorg S, Duscha A, Berg J, Manzel A, Waschbisch A, et al. Dietary fatty acids directly impact central nervous system autoimmunity via the small intestine. Immunity (2015) 43:817–29. doi: 10.1016/j.immuni.2015.09.007
54. Miranda PM, De Palma G, Serkis V, Lu J, Louis-Auguste MP, McCarville JL, et al. High salt diet exacerbates colitis in mice by decreasing Lactobacillus levels and butyrate production. Microbiome (2018) 6:57. doi: 10.1186/s40168-018-0433-4
55. Tubbs AL, Liu B, Rogers TD, Sartor RB, Miao EA. Dietary salt exacerbates experimental colitis. J Immunol. (2017) 199, 1051–1059. doi: 10.4049/jimmunol.1700356
56. Harris PJ, Ferguson LR. Dietary fibre: its composition and role in protection against colorectal cancer. Mutation Res. (1993) 290:97–110. doi: 10.1016/0027-5107(93)90037-G
57. Hou JK, Abraham B, El-Serag H. Dietary intake and risk of developing inflammatory bowel disease: a systematic review of the literature. Am J Gastroenterol. (2011) 106:563–73. doi: 10.1038/ajg.2011.44
58. Meyer KA, Kushi LH, Jacobs DRJr, Slavin J, Sellers TA, Folsom AR. Carbohydrates, dietary fiber, and incident type 2 diabetes in older women. Am J Clin Nutr. (2000) 71:921–30. doi: 10.1093/ajcn/71.4.921
59. Wannamethee SG, Whincup PH, Thomas MC, Sattar N. Associations between dietary fiber and inflammation, hepatic function, and risk of type 2 diabetes in older men: potential mechanisms for the benefits of fiber on diabetes risk. Diabetes Care (2009) 32:1823–5. doi: 10.2337/dc09-0477
60. Flint HJ, Duncan SH, Scott KP, Louis P. Links between diet, gut microbiota composition and gut metabolism. Proc Nutr Soc. (2015) 74:13–22. doi: 10.1017/S0029665114001463
61. Miller TL, Wolin MJ. Pathways of acetate, propionate, and butyrate formation by the human fecal microbial flora. Appl Environ Microbiol. (1996) 62:1589–92.
62. Le Poul E, Loison C, Struyf S, Springael JY, Lannoy V, Decobecq ME, et al. Functional characterization of human receptors for short chain fatty acids and their role in polymorphonuclear cell activation. J Biol Chem. (2003) 278:25481–9. doi: 10.1074/jbc.M301403200
63. Desai MS, Seekatz AM, Koropatkin NM, Kamada N, Hickey CA, Wolter M, et al. A dietary fiber-deprived gut microbiota degrades the colonic mucus barrier and enhances pathogen susceptibility. Cell (2016) 167:1339–53.e1321. doi: 10.1016/j.cell.2016.10.043
64. Kim KA, Gu W, Lee IA, Joh EH, Kim DH. High fat diet-induced gut microbiota exacerbates inflammation and obesity in mice via the TLR4 signaling pathway. PLoS ONE (2012) 7:e47713. doi: 10.1371/journal.pone.0047713
65. Liu Z, Brooks RS, Ciappio ED, Kim SJ, Crott JW, Bennett G, et al. Diet-induced obesity elevates colonic TNF-alpha in mice and is accompanied by an activation of Wnt signaling: a mechanism for obesity-associated colorectal cancer. J Nutr Biochem. (2012) 23:1207–13. doi: 10.1016/j.jnutbio.2011.07.002
66. Murakami Y, Tanabe S, Suzuki T. High-fat diet-induced intestinal hyperpermeability is associated with increased bile acids in the large intestine of mice. J Food Sci. (2016) 81:H216–222. doi: 10.1111/1750-3841.13166
67. Gulhane M, Murray L, Lourie R, Tong H, Sheng YH, Wang R, et al. High fat diets induce colonic epithelial cell stress and inflammation that is reversed by IL-22. Sci Rep. (2016) 6:28990. doi: 10.1038/srep28990
68. Schroeder BO, Birchenough GMH, Stahlman M, Arike L, Johansson MEV, Hansson GC, et al. Bifidobacteria or fiber protects against diet-induced microbiota-mediated colonic mucus deterioration. Cell Host Microbe (2018) 23:27–40.e27. doi: 10.1016/j.chom.2017.11.004
69. Devkota S, Wang Y, Musch MW, Leone V, Fehlner-Peach H, Nadimpalli A, et al. Dietary-fat-induced taurocholic acid promotes pathobiont expansion and colitis in Il10-/- mice. Nature (2012) 487:104–8. doi: 10.1038/nature11225
70. Hildebrandt MA, Hoffmann C, Sherrill-Mix SA, Keilbaugh SA, Hamady M, Chen YY, et al. High-fat diet determines the composition of the murine gut microbiome independently of obesity. Gastroenterology (2009) 137:1716–24.e1711–1712. doi: 10.1053/j.gastro.2009.08.042
71. Agus A, Denizot J, Thevenot J, Martinez-Medina M, Massier S, Sauvanet P, et al. Western diet induces a shift in microbiota composition enhancing susceptibility to Adherent-Invasive E. coli infection and intestinal inflammation. Sci Rep. (2016) 6:19032. doi: 10.1038/srep19032
72. Martinez-Medina M, Denizot J, Dreux N, Robin F, Billard E, Bonnet R, et al. Western diet induces dysbiosis with increased E coli in CEABAC10 mice, alters host barrier function favouring AIEC colonisation. Gut (2014) 63:116–24. doi: 10.1136/gutjnl-2012-304119
73. Hernandez AL, Kitz A, Wu C, Lowther DE, Rodriguez DM, Vudattu N, et al. Sodium chloride inhibits the suppressive function of FOXP3+ regulatory T cells. J Clin Invest. (2015) 125:4212–22. doi: 10.1172/JCI81151
74. Progatzky F, Sangha NJ, Yoshida N, McBrien M, Cheung J, Shia A, et al. Dietary cholesterol directly induces acute inflammasome-dependent intestinal inflammation. Nat Commun. (2014) 5:5864. doi: 10.1038/ncomms6864
75. Ito A, Hong C, Oka K, Salazar JV, Diehl C, Witztum JL, et al. Cholesterol accumulation in CD11c(+) immune cells is a causal and targetable factor in autoimmune disease. Immunity (2016) 45:1311–26. doi: 10.1016/j.immuni.2016.11.008
76. Borst SE, Conover CF. High-fat diet induces increased tissue expression of TNF-alpha. Life Sci. (2005) 77:2156–65. doi: 10.1016/j.lfs.2005.03.021
77. Morin CL, Eckel RH, Marcel T, Pagliassotti MJ. High fat diets elevate adipose tissue-derived tumor necrosis factor-alpha activity. Endocrinology (1997) 138:4665–71. doi: 10.1210/endo.138.11.5519
78. Chassaing B, Srinivasan G, Delgado MA, Young AN, Gewirtz AT, Vijay-Kumar M. Fecal lipocalin 2, a sensitive and broadly dynamic non-invasive biomarker for intestinal inflammation. PLoS ONE (2012) 7:e44328. doi: 10.1371/journal.pone.0044328
79. Cani PD, Amar J, Iglesias MA, Poggi M, Knauf C, Bastelica D, et al. Metabolic endotoxemia initiates obesity and insulin resistance. Diabetes (2007) 56:1761–72. doi: 10.2337/db06-1491
80. Pendyala S, Walker JM, Holt PR. A high-fat diet is associated with endotoxemia that originates from the gut. Gastroenterology (2012) 142:1100–1101.e1102. doi: 10.1053/j.gastro.2012.01.034
81. Soroosh P, Wu J, Xue X, Song J, Sutton SW, Sablad M, et al. Oxysterols are agonist ligands of RORgammat and drive Th17 cell differentiation. Proc Natl Acad Sci USA. (2014) 111:12163–8. doi: 10.1073/pnas.1322807111
82. Cui G, Qin X, Wu L, Zhang Y, Sheng X, Yu Q, et al. Liver X receptor (LXR) mediates negative regulation of mouse and human Th17 differentiation. J Clin Invest. (2011) 121:658–70. doi: 10.1172/JCI42974
84. Feng Z, Long W, Hao B, Ding D, Ma X, Zhao L, et al. A human stool-derived Bilophila wadsworthia strain caused systemic inflammation in specific-pathogen-free mice. Gut Pathog. (2017) 9:59. doi: 10.1186/s13099-017-0208-7
85. Baron EJ, Summanen P, Downes J, Roberts MC, Wexler H, Finegold SM. Bilophila wadsworthia, gen. nov and sp nov, a unique gram-negative anaerobic rod recovered from appendicitis specimens and human faeces. J General Microbiol. (1989) 135:3405–11.
86. Manichanh C, Rigottier-Gois L, Bonnaud E, Gloux K, Pelletier E, Frangeul L, et al. Reduced diversity of faecal microbiota in Crohn's disease revealed by a metagenomic approach. Gut (2006) 55:205–11. doi: 10.1136/gut.2005.073817
87. Aggarwal S, Ghilardi N, Xie MH, de Sauvage FJ, Gurney AL. Interleukin-23 promotes a distinct CD4 T cell activation state characterized by the production of interleukin-17. J Biol Chem. (2003) 278:1910–4. doi: 10.1074/jbc.M207577200
88. Zhou L, Ivanov II, Spolski R, Min R, Shenderov K, Egawa T, et al. IL-6 programs T(H)-17 cell differentiation by promoting sequential engagement of the IL-21 and IL-23 pathways. Nat Immunol. (2007) 8:967–74. doi: 10.1038/ni1488
89. Kuntzsch D, Bergann T, Dames P, Fromm A, Fromm M, Davis RA, et al. The plant-derived glucocorticoid receptor agonist Endiandrin A acts as co-stimulator of colonic epithelial sodium channels (ENaC) via SGK-1 and MAPKs. PLoS ONE (2012) 7:e49426. doi: 10.1371/journal.pone.0049426
90. Wulff P, Vallon V, Huang DY, Volkl H, Yu F, Richter K, et al. Impaired renal Na(+) retention in the sgk1-knockout mouse. J Clin Invest. (2002) 110:1263–8. doi: 10.1172/JCI0215696
91. Yang Y, Torchinsky MB, Gobert M, Xiong H, Xu M, Linehan JL, et al. Focused specificity of intestinal TH17 cells towards commensal bacterial antigens. Nature (2014) 510:152–6. doi: 10.1038/nature13279
92. Furusawa Y, Obata Y, Fukuda S, Endo TA, Nakato G, Takahashi D, et al. Commensal microbe-derived butyrate induces the differentiation of colonic regulatory T cells. Nature (2013) 504:446–50. doi: 10.1038/nature12721
93. Smith PM, Howitt MR, Panikov N, Michaud M, Gallini CA, Bohlooly YM, et al. The microbial metabolites, short-chain fatty acids, regulate colonic Treg cell homeostasis. Science (2013) 341:569–73. doi: 10.1126/science.1241165
94. Schulz MD, Atay C, Heringer J, Romrig FK, Schwitalla S, Aydin B, et al. High-fat-diet-mediated dysbiosis promotes intestinal carcinogenesis independently of obesity. Nature (2014) 514:508–12. doi: 10.1038/nature13398
95. Arpaia N, Campbell C, Fan X, Dikiy S, van der Veeken J, deRoos P, et al. Metabolites produced by commensal bacteria promote peripheral regulatory T-cell generation. Nature (2013) 504:451–5. doi: 10.1038/nature12726
96. Park J, Kim M, Kang SG, Jannasch AH, Cooper B, Patterson J, et al. Short-chain fatty acids induce both effector and regulatory T cells by suppression of histone deacetylases and regulation of the mTOR-S6K pathway. Mucosal Immunol. (2015) 8:80–93. doi: 10.1038/mi.2014.44
97. Kimura A, Naka T, Nohara K, Fujii-Kuriyama Y, Kishimoto T. Aryl hydrocarbon receptor regulates Stat1 activation and participates in the development of Th17 cells. Proc Natl Acad Sci USA. (2008) 105:9721–6. doi: 10.1073/pnas.0804231105
98. Bai A, Lu N, Guo Y, Liu Z, Chen J, Peng Z. All-trans retinoic acid down-regulates inflammatory responses by shifting the Treg/Th17 profile in human ulcerative and murine colitis. J Leukocyte Biol. (2009) 86:959–69. doi: 10.1189/jlb.0109006
99. Hong K, Zhang Y, Guo Y, Xie J, Wang J, He X, et al. All-trans retinoic acid attenuates experimental colitis through inhibition of NF-kappaB signaling. Immunol Lett. (2014) 162:34–40. doi: 10.1016/j.imlet.2014.06.011
100. Mucida D, Park Y, Kim G, Turovskaya O, Scott I, Kronenberg M, et al. Reciprocal TH17 and regulatory T cell differentiation mediated by retinoic acid. Science (2007) 317:256–60. doi: 10.1126/science.1145697
101. Xiao S, Jin H, Korn T, Liu SM, Oukka M, Lim B, et al. Retinoic acid increases Foxp3+ regulatory T cells and inhibits development of Th17 cells by enhancing TGF-beta-driven Smad3 signaling and inhibiting IL-6 and IL-23 receptor expression. J Immunol. (2008) 181, 2277–84. doi: 10.4049/jimmunol.181.4.2277
102. Iwata M, Hirakiyama A, Eshima Y, Kagechika H, Kato C, Song SY. Retinoic acid imprints gut-homing specificity on T cells. Immunity (2004) 21:527–38. doi: 10.1016/j.immuni.2004.08.011
103. Chang SH, Chung Y, Dong C. Vitamin D suppresses Th17 cytokine production by inducing C/EBP homologous protein (CHOP) expression. J Biol Chem. (2010) 285:38751–5. doi: 10.1074/jbc.C110.185777
104. Garg M, Hendy P, Ding JN, Shaw S, Hold G, Hart A. The effect of vitamin D on intestinal inflammation and faecal microbiota in patients with ulcerative colitis. J Crohn's Colitis (2018) 12:963–972. doi: 10.1093/ecco-jcc/jjy052
105. Cotillard A, Kennedy SP, Kong LC, Prifti E, Pons N, Le Chatelier E, et al. Dietary intervention impact on gut microbial gene richness. Nature (2013) 500:585–8. doi: 10.1038/nature12480
106. Le Chatelier E, Nielsen T, Qin J, Prifti E, Hildebrand F, Falony G, et al. Richness of human gut microbiome correlates with metabolic markers. Nature (2013) 500:541–6. doi: 10.1038/nature12506
107. Bourquin LD, Titgemeyer EC, Fahey GCJr. Vegetable fiber fermentation by human fecal bacteria: cell wall polysaccharide disappearance and short-chain fatty acid production during in vitro fermentation and water-holding capacity of unfermented residues. J Nutr. (1993) 123:860–9. doi: 10.1093/jn/123.5.860
108. Burkitt DP. Epidemiology of large bowel disease: the role of fibre. Proc Nutr Soc. (1973) 32:145–9. doi: 10.1079/PNS19730032
109. Rodriguez-Cabezas ME, Galvez J, Camuesco D, Lorente MD, Concha A, Martinez-Augustin O, et al. Intestinal anti-inflammatory activity of dietary fiber (Plantago ovata seeds) in HLA-B27 transgenic rats. Clin Nutr. (2003) 22:463–71. doi: 10.1016/S0261-5614(03)00045-1
110. Earle KA, Billings G, Sigal M, Lichtman JS, Hansson GC, Elias JE, et al. Quantitative imaging of gut microbiota spatial organization. Cell Host Microbe (2015) 18:478–88. doi: 10.1016/j.chom.2015.09.002
111. Hryckowian AJ, Van Treuren W, Smits SA, Davis NM, Gardner JO, Bouley DM, et al. Microbiota-accessible carbohydrates suppress Clostridium difficile infection in a murine model. Nat Microbiol. (2018) 3:662–9. doi: 10.1038/s41564-018-0150-6
112. Kim M, Friesen L, Park J, Kim HM, Kim CH. Microbial metabolites, short-chain fatty acids, restrain tissue bacterial load, chronic inflammation, and associated cancer in the colon of mice. Eur J Immunol. (2018) 48:1235–47. doi: 10.1002/eji.201747122
113. Kim MH, Kang SG, Park JH, Yanagisawa M, Kim CH. Short-chain fatty acids activate GPR41 and GPR43 on intestinal epithelial cells to promote inflammatory responses in mice. Gastroenterology (2013) 145:396–406.e391–e310. doi: 10.1053/j.gastro.2013.04.056
114. D'Souza S, Levy E, Mack D, Israel D, Lambrette P, Ghadirian P, et al. Dietary patterns and risk for Crohn's disease in children. Inflamm Bowel Dis. (2008) 14:367–73. doi: 10.1002/ibd.20333
115. Li Y, Innocentin S, Withers DR, Roberts NA, Gallagher AR, Grigorieva EF, et al. Exogenous stimuli maintain intraepithelial lymphocytes via aryl hydrocarbon receptor activation. Cell (2011) 147:629–40. doi: 10.1016/j.cell.2011.09.025
116. Nakahama T, Hanieh H, Nguyen NT, Chinen I, Ripley B, Millrine D, et al. Aryl hydrocarbon receptor-mediated induction of the microRNA-132/212 cluster promotes interleukin-17-producing T-helper cell differentiation. Proc Natl Acad Sci USA. (2013) 110:11964–9. doi: 10.1073/pnas.1311087110
117. Qiu J, Heller JJ, Guo X, Chen ZM, Fish K, Fu YX, et al. The aryl hydrocarbon receptor regulates gut immunity through modulation of innate lymphoid cells. Immunity (2012) 36:92–104. doi: 10.1016/j.immuni.2011.11.011
118. Qiu J, Guo X, Chen ZM, He L, Sonnenberg GF, Artis D, et al. Group 3 innate lymphoid cells inhibit T-cell-mediated intestinal inflammation through aryl hydrocarbon receptor signaling and regulation of microflora. Immunity (2013) 39:386–99. doi: 10.1016/j.immuni.2013.08.002
119. Harrison EH. Mechanisms involved in the intestinal absorption of dietary vitamin A and provitamin A carotenoids. Biochim Biophys Acta (2012) 1821:70–7. doi: 10.1016/j.bbalip.2011.06.002
120. Villablanca EJ, Wang S, de Calisto J, Gomes DC, Kane MA, Napoli JL, et al. MyD88 and retinoic acid signaling pathways interact to modulate gastrointestinal activities of dendritic cells. Gastroenterology (2011) 141:176–85. doi: 10.1053/j.gastro.2011.04.010
121. Czarnewski P, Das S, Parigi SM, Villablanca EJ. Retinoic acid and its role in modulating intestinal innate immunity. Nutrients (2017) 9:68. doi: 10.3390/nu9010068
122. Tejon G, Manriquez V, De Calisto J, Flores-Santibanez F, Hidalgo Y, Crisostomo N, et al. Vitamin a impairs the reprogramming of Tregs into IL-17-producing cells during intestinal inflammation. BioMed Res Int. (2015) 2015:137893. doi: 10.1155/2015/137893
123. Simmons JD, Mullighan C, Welsh KI, Jewell DP. Vitamin D receptor gene polymorphism: association with Crohn's disease susceptibility. Gut (2000) 47:211–4. doi: 10.1136/gut.47.2.211
Keywords: inflammation, mucosal immunity, CD4 T cells, western diet, fat, salt, fiber, microbiota
Citation: Siracusa F, Schaltenberg N, Villablanca EJ, Huber S and Gagliani N (2019) Dietary Habits and Intestinal Immunity: From Food Intake to CD4+ TH Cells. Front. Immunol. 9:3177. doi: 10.3389/fimmu.2018.03177
Received: 15 June 2018; Accepted: 27 December 2018;
Published: 15 January 2019.
Edited by:
Marcela A. Hermoso, Universidad de Chile, ChileReviewed by:
Luca Pastorelli, University of Milan, ItalyPatricia Langjahr, Universidad Nacional de Asunción, Paraguay
Copyright © 2019 Siracusa, Schaltenberg, Villablanca, Huber and Gagliani. This is an open-access article distributed under the terms of the Creative Commons Attribution License (CC BY). The use, distribution or reproduction in other forums is permitted, provided the original author(s) and the copyright owner(s) are credited and that the original publication in this journal is cited, in accordance with accepted academic practice. No use, distribution or reproduction is permitted which does not comply with these terms.
*Correspondence: Nicola Gagliani, bi5nYWdsaWFuaUB1a2UuZGU=
†These authors have contributed equally to this work