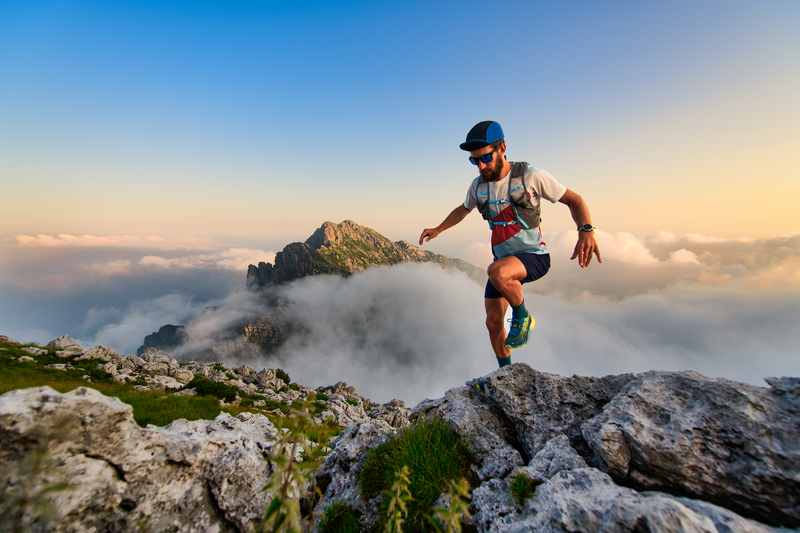
95% of researchers rate our articles as excellent or good
Learn more about the work of our research integrity team to safeguard the quality of each article we publish.
Find out more
REVIEW article
Front. Immunol. , 05 September 2018
Sec. Microbial Immunology
Volume 9 - 2018 | https://doi.org/10.3389/fimmu.2018.01926
This article is part of the Research Topic The Immunology of Sepsis – Understanding Host Susceptibility, Pathogenesis of Disease, and Avenues for Future Treatment View all 27 articles
A commentary has been posted on this article:
Commentary: Precision Immunotherapy for Sepsis
Decades of sepsis research into a specific immune system-targeting adjunctive therapy have not resulted in the discovery of an effective compound. Apart from antibiotics, source control, resuscitation and organ support, not a single adjunctive treatment is used in current clinical practice. The inability to determine the prevailing immunological phenotype of patients and the related large heterogeneity of study populations are regarded by many as the most important factors behind the disappointing results of past clinical trials. While the therapeutic focus has long been on immunosuppressive strategies, increased appreciation of the importance of sepsis-induced immunoparalysis in causing morbidity and mortality in sepsis patients has resulted in a paradigm shift in the sepsis research field towards strategies aimed at enhancing the immune response. However, similar to immunosuppressive therapies, precision medicine is imperative for future trials with immunostimulatory compounds to succeed. As such, identifying those patients with a severely suppressed or hyperactive immune system who will most likely benefit from either immunostimulatory or immunosuppressive therapy, and accurate monitoring of both the immune and treatment response is crucial. This review provides an overview of the challenges lying ahead on the path towards precision immunotherapy for patients suffering from sepsis.
Sepsis is defined as life-threatening organ dysfunction caused by a dysregulated host response to infection (1). It is the number one cause of death in the Intensive Care unit (ICU), and the worldwide incidence of sepsis is estimated to exceed 30 million cases per year (2, 3). Despite advances in ICU management and goal-directed interventions in the last decades, sepsis mortality rates remain as high as 30% (4). In the Western world alone, annually an estimated 6 million people die of sepsis, representing more deaths than lung, breast and colon cancer together (5). In addition, it is also one of the most expensive conditions encountered in hospitals, with annual costs exceeding 20 billion dollars in the US alone1. Despite these alarming facts, sepsis remains a relatively neglected condition that is unknown to the general public.
In May 2017, the WHO adopted a resolution aimed to improve the prevention, diagnosis, and management of sepsis2, illustrating the urgency of the problem. The search for a specific immune system-targeting adjunctive therapy has dominated the sepsis research field for more than 4 decades, with the disappointing result of dozens of negative trials and not a single adjunctive treatment in current clinical use. Experts agree that this is not due to the fact that the drugs tested are ineffective per se, but rather to the inability to restrict treatment to selected patient groups that may actually benefit from a specific type of therapy (6–9). Not surprisingly, it is therefore also agreed upon that precision medicine is imperative for future trials to succeed, but accurate and reliable immunomonitoring is currently not a reality (6, 8–10). For new therapies, it is paramount that we do not make the same mistake that was previously made for immunosuppressive treatments by advocating the use of compounds in all sepsis patients. Instead, we should only target patients who are truly hyperinflamed with immune suppressive drugs and immunoparalyzed patients with immunostimulatory compounds to avoid unnecessary risks of side effects in patient groups with a lower chance of a therapeutic effect and increase the chances of success in patient groups with a higher likelihood of benefit. In addition to immunomodulatory therapy, advocating personalized medicine is also relevant for other promising sepsis treatments, for instance those targeting the well-described metabolic dysfunction observed in these patients. However this is beyond the scope of this review. This review discusses whether we are targeting the right pathophysiological immunological mechanisms and emphasizes the challenges that lie ahead on the path towards precision immunotherapy for septic patients.
The proinflammatory response in sepsis is directed at eliminating invading pathogens and involves leukocyte activation, cytokine production, reactive oxygen species and protease release, and complement and coagulation activation (9). An overzealous hyperactive proinflammatory response may exert detrimental effects for the host by eliciting high fevers, hypotension, tachycardia, tachypnoea, coagulation disorders and organ failure, the latter resulting from collateral tissue damage. Examples of deleterious effects of hyperinflammation in various tissues are illustrated in Figure 1. Pulmonary hyperinflammation may lead to the development of acute respiratory distress syndrome (ARDS), a life-threatening condition characterized by unexplained respiratory failure through hypoxemia with bilateral infiltrates of noncardiac origin (11). In the circulating blood compartment hyperinflammation alters coagulation, which may result in a relatively uncommon but fulminant phenomenon called disseminated intravascular coagulation (DIC) (12), which is characterized by simultaneous widespread microvascular thrombosis and profuse bleeding. Capillary leak and development of interstitial oedema are unfortunately very common problems resulting from a systemic hyperinflammatory state, and have widespread cardiovascular effects such as hypotension, tachycardia and in severe cases even septic shock which results in more tissue damage. In the acute phase, hyperinflammation in the brain may cause headaches, nausea, apathy, somnolence or delirium. Furthermore it is suggested that long-term effects such as cognitive decline and behavioral changes can also be attributed to a hyperinflammatory state (13). Other organs that are often affected by hyperinflammation include the kidneys (acute kidney injury which frequently requires renal replacement therapy), the intestines (paralytic ileus), and the liver (liver failure with liver test abnormalities and altered glycemic control).
Figure 1. Clinical consequences of hyperinflammation and immunoparalysis to selected tissues. ARDS, acute respiratory distress syndrome; LSEC, liver sinusoidal endothelial cells; MDSC, myeloid-derived suppressor cells; RTEC, renal tubule epithelial cell; M-cell, microfold cell; TPN, total parenteral nutrition; HSPC, hematopoietic stem and progenitor cells.
From the 1970s until the turn of the century, it was commonly assumed that sepsis mortality resulted exclusively from this overzealous pro-inflammatory response. As a consequence, therapeutic research in the sepsis field was solely focused on dampening or preventing excessive inflammation. However, all clinical trials to date investigating immunosuppressive therapy in sepsis, including anti-endotoxin (signaling) molecules, TLR-receptor antagonists, anti-cytokine therapies (e.g., anti-TNF-α, IL-1RA etc.), and high dose corticosteroids(14–24), have convincingly demonstrated that inhibition of the immune response exerts no beneficial effects in an unselected heterogeneous group of sepsis patients.
In the last decade a paradigm shift in our understanding of the immune derangements in sepsis has taken place (25). It is increasingly recognized that, for many patients not excessive immune activation, but rather immunosuppression, also known as “sepsis-induced immunoparalysis,” is the overriding immune dysfunction associated with high mortality and morbidity (7, 25–29). The capacity of circulating leukocytes to release proinflammatory cytokines is impaired during immunoparalysis and apoptotic immune cell death is profoundly increased. In contrast to necrotic cell death, which generally causes stimulation of the immune system and enhanced defense against microbes, apoptotic cell death results in anti-inflammatory cytokine production, and cellular anergy (30–32). The majority of cells lost through apoptosis in septic patients are lymphocytes (33) and low absolute lymphocyte counts were shown to be associated with mortality (34). Sepsis-induced immunoparalysis renders patients unable to clear their primary infection and more likely to develop secondary infections with opportunistic bacteria or fungi (35) later on. This is in line with data that the vast majority of septic patients do not die from the initial pro-inflammatory hit, but at a later time point from secondary or opportunistic infections in an immunosuppressed state (35–38). More precisely: approximately a quarter of sepsis patients die within 4 days. Of the remaining three-quarter that survives, one third regains immunocompetence and the mortality in this group is 10%. Two-thirds develops immunoparalysis accounting for 65% of total mortality (10). The development of immunoparalysis appears to start simultaneously with the proinflammatory response. The clinical relevance of immunoparalysis is further illustrated by several observational findings: (i) in patients who died from sepsis or septic shock, a continuous septic focus was observed in 63 of the 71 patients (89%) who were treated with antibiotics for more than 7 days (39), (ii) during the late phase of sepsis, infections due to opportunistic bacteria increase from 9 to 18% and Candida-infections from 13 to 30% (35), and (iii) reactivation of latent viruses was found in 43% of critically ill patients (40). Of interest, detection rate of positive viral PCR results increased with ICU length of stay and was associated with the development of fungal and opportunistic bacterial infections. Importantly, Epstein Barr virus and CMV PCR-titers in patients with a bacterial sepsis was similar to those reported in stem-cell and organ transplant patients, indicative of clinically relevant immune suppression. These recent observational insights indicate that sepsis-induced immunoparalysis accounts for the majority of sepsis-related deaths. Although the importance of immunoparalysis is increasingly recognized (28), there is still lack of consensus that immunosuppression is a clinically important phenomenon (41, 42). Nevertheless, combined with the many disappointing trials on immunosuppressive strategies, appreciation of the detrimental role of sepsis-induced immunoparalysis (7, 25–29, 43) has led the sepsis research field to focus more and more on ways to restore the suppressed immune response through immunostimulatory treatment (7, 25, 28, 29). This concept is appealing and the treatments under investigation are most likely effective to reverse immunoparalysis. However, in light of previous sepsis trials, only patients with proven immunoparalysis should receive immunostimulatory treatment to avoid unnecessary risks and increase the chances of successful trials. However, methods to identify patients with immunoparalysis and subsequent therapeutic reversal of immunoparalysis are still in their infancy.
Many cytokines, chemokines or other proteins have been studied as potential biomarkers to characterize a hyperinflammatory state in sepsis patients. Three pro-inflammatory cytokines, namely tumor necrosis factor (TNF), interleukin-1β (IL-1β) and interleukin-6 (IL-6) play a pivotal role in the initial response of the innate immune system to injury or infection. These three cytokines are, among others, crucial for activation of endothelial cells, recruitment of leukocytes to the site, generation of fever and other systemic symptoms, production of acute phase reactants and induction of a shift in cell production in the bone marrow (44). Nevertheless neither TNF or IL-1β have emerged as reliable biomarker for hyperinflammation in sepsis patients, potentially because they are elevated only for a very short period of time in the initial phase of sepsis, when patients may not yet have been admitted to the ICU. IL-6 has been most extensively studied as a potential biomarker, with the advantages of being elevated for a longer period of time and the availability of commercial bedside immunoassays (45). Elevated levels of IL-6 in septic patients have been shown to be associated with increased mortality (46, 47). However this illustrates prognostic rather than diagnostic value and like most cytokines, IL-6 is not specific for sepsis as increased levels are observed in many inflammatory conditions. Moreover the chemokines interleukin-8 (IL-8) and monocyte chemoattractant protein-1 (MCP-1) have been shown to be superior to IL-6 for diagnosis of sepsis (48) and prediction of sepsis mortality (49) respectively. The acute phase protein CRP has a high sensitivity for detection of early onset sepsis (50), but its low specificity is a major drawback for its use as a biomarker to stratify the immune status in patients with sepsis. Procalcitonin (PCT) is elevated in patients with invasive bacterial infections (51). However it remains to be determined whether the detection of bacteraemia with PCT can accurately distinguish patients in a hyperinflammatiory state from patients with immunoparalysis. Research on monocyte activation markers as potential biomarkers of a hyperinflammatory state in sepsis has identified a possible role for the soluble form of the receptor for advanced glycation end-products (sRAGE). This molecule may be considered as a receptor for danger associated molecule patterns (DAMPs) and elevated levels were shown to be associated with poor survival in severe sepsis (52). Despite the numerous laboratory options illustrated above no accurate single biomarker for hyperinflammation in sepsis is currently used in clinical practice. As an alternative, a more clear clinical example for a severe hyperinflammatory state is the macrophage activation syndrome (MAS). This is defined as a fulminant cytokine storm concurrent with hepatosplenomegaly, liver dysfunction, hyperferritaemia, pancytopenia, and disseminated intravascular coagulation (53). MAS is a serious complication of sepsis and the clearly overriding hyperinflammatory state in these patients, may provide opportunities for targeted application of anti-inflammatory therapies, as discussed elsewhere in this review.
Identification of patients with immunoparalysis is currently based on HLA-DR expression on circulating monocytes and, to a lesser degree, cytokine production of leukocytes stimulated ex vivo with lipopolysaccharide (LPS, endotoxin) (54–57), although the accuracy of these markers still lacks solid evidence. There are no data on the predictive value on the individual patient level, meaning that precision immunostimulatory treatment for sepsis patients may not be feasible using the markers that are currently advocated. The inability to restrict immunostimulatory treatment to those patients who will actually most likely benefit from it may result in another series of failed clinical trials, because beneficial effects in immunoparalyzed patients will be offset by possible harmful effects of these compounds in immunocompetent patients. Several other markers of immunoparalysis are proposed (25), based on the increasing knowledge of the pathophysiology of immunoparalysis. These include expression of inhibitory receptors like programmed death-1 (PD-1) and its ligand PD-L1 (58), cytotoxic T lymphocyte antigen-4 (CTLA-4) (59), and B and T lymphocyte attenuator (BTLA) (60), molecules that play a role in the exhaustion of lymphocytes. Furthermore, several immunosuppressive lymphocyte subpopulations (including T-regulatory cells) have been identified in patients suffering from immunoparalysis (61). Moreover, epigenetic changes were shown to be involved in immunoparalysis (54), revealing that TLR-induced chromatin modifications are responsible for transient silencing of tolerizable (T) genes (including those encoding proinflammatory mediators), and for priming of non-tolerizable (NT) genes (including those encoding antimicrobial peptides). The T genes are transiently inactivated to prevent pathology associated with excessive inflammation, while the NT genes remain inducible to provide continuous protection from infection and tissue repair. In addition, it was demonstrated that negative TLR regulators such as IRAK-M and SHIP-1, might also participate in the development of immunoparalysis (62). However, the role of these relatively recently discovered mechanisms in the pathogenesis of immunoparalysis is currently unclear and clinical application as a biomarker is not yet feasible.
Understanding what causes the contrasting hyperinflammatory and immunoparalyzed phenotypes observed in sepsis—in other words: why do some patients exhibitit a prevailing hyperinflammatory response while others display immunoparalysis—would greatly aid biomarker discovery and development. To the best of our knowledge, this is currently unknown and a multifactorial etiology is likely, including host-related factors such as age, gender, comorbidities, (epi)genetic predisposition, microbiome composition, expression levels of pattern-recognition receptors (PRRs), and release of DAMPs as well as pathogen-related factors such as the type of pathogen, its virulence and load, and quorum sensing.
Whether it concerns hyperinflammation or immunoparalysis, it appears implausible that a single marker can act as a reliable tool to guide immunomodulating therapy since biomarkers are often related to one or a limited number of pathophysiological mechanisms/pathways, while it has become clear that multiple pathways are activated or inhibited at the same time in sepsis. Therefore, it appears likely that a panel of markers reflects the immune status of the sepsis patient more accurately.
At this moment, plasma markers or expression of molecules in/on circulating immune cells are used to identify the immune status of sepsis patients. However, due to compartmentalization of the immune response and temporal differences in the immune response between compartments, the phenotype of blood leukocytes may not always be reflective of the current immune status. There are several observations in support of this notion. For instance, leukopenia is associated with a more pronounced cytokine response in animal models of sepsis (63), indicating limited importance of blood immune cells in producing inflammatory mediators that are important for host defense. Furthermore, the compartmentalized nature of the immune response is supported by several results obtained by our group in the human endotoxemia model. In this model endotoxin [also known as lipopolysaccharide (LPS)] is administered to healthy volunteers. Numerous studies have established that the immune response to LPS captures many hallmarks of the immune response observed in sepsis, including a phase of immune suppression. The latter phenomenon is known as “endotoxin tolerance,” and characterized by a blunted inflammatory response upon subsequent LPS challenges in vivo and ex vivo (64). In keeping with the overlap between LPS-elicited effects and clinical sepsis, endotoxin tolerance bears many similarities to sepsis-induced immunoparalysis, including decreased cytokine production by circulating leukocytes and attenuated mHLA-DR expression (25, 27, 43). Therefore, endotoxin tolerance has been used in translational research to model and investigate treatments for sepsis-induced immunoparalysis, both in vitro (65) and in vivo in humans (43). Strikingly, however, animal studies have shown that despite a diminished immune response indicating endotoxin tolerance, pathogen clearance and survival upon a live bacterial challenge were improved in mice pre-treated with LPS or other TLR ligands (66–69). It is unknown whether these counterintuitive findings of an enhanced defense against pathogens concurrent with endotoxin tolerance are also present in humans, which should be an important focus for future studies. Nevertheless, using the human endotoxemia model we have demonstrated profound differences in ex vivo and in vivo endotoxin tolerance kinetics. Both mHLA-DR expression (43) and ex-vivo cytokine production by stimulated leukocytes (70–72) are suppressed rapidly in the first hours after endotoxin administration. The same studies show that these markers normalize quickly afterwards and are restored within 24 h after endotoxin administration, indicating that functionality of circulating leukocytes can quickly recover in the absence of ongoing inflammation. In contrast, the in vivo response 1 to 2 weeks after the first endotoxin administration is still severely blunted with an attenuation of pro-inflammatory cytokine levels of approximately 60% compared to the first endotoxin challenge (43, 70). From these observations it could be concluded that parameters measured in the blood compartment may not reflect the responsiveness of the immune system as a whole, and that immune cells in other compartments than the blood may likely better reflect the in vivo immune status at a given moment in time. It is currently unknown whether this discrepancy between the immune status of cells within the blood compartment and the responsiveness of the in vivo immune system is of clinical importance in sepsis patients, but tissue resident macrophages and not circulating immune cells appear to be predominantly responsible for the innate immune response in sepsis. The relevance of organ-specific immunology and possible consequences of a hyperinflammatory or immune-suppressed state in several tissues (e.g., lungs, brain, adipose tissue, and bone marrow), are graphically presented in Figure 1 and outlined below.
The lungs likely represent a highly relevant compartment in the context of sepsis-induced hyperinflammation and immunoparalysis. An exaggerated pro-inflammatory response in the lungs may result in the life-threatening condition of ARDS, requiring invasive mechanical ventilation and complex respiratory therapy (73). In contrary, an immunosuppressed response as observed in immunoparalysis increases the susceptibility to secondary pulmonary infections. Along these lines, hospital-acquired pneumonia represents the secondary infection with the highest incidence observed in patients that recovered from their initial sepsis (42, 74, 75). Alveolar macrophages (AMs), as the first line of defense in the lungs, play a key role in host defense. Whereas previous work has demonstrated that AMs display a primed rather than tolerant phenotype shortly (1.5–6 h) after LPS administration in healthy volunteers (76), small studies in septic patients point toward a tolerant phenotype of AMs (77, 78). This may indicate that AMs switch from an initially primed phenotype to a tolerant immunosuppressed phenotype at later stages of disease progression, but these dynamics have yet to be unraveled.
The brain was long regarded as an immune privileged organ. The last decades of research have shown an important immunological role of the brain in “non-immunological” diseases like dementia, or psychiatric diseases. The so-called microglial cells represent the resident macrophage population in the brain, and account for 5–20% of the total glial cell population of the brain. Research in healthy humans (79), animal data (80) and post-mortem brain tissue of patients suffering from severe systemic inflammation (81, 82) show that systemic inflammation is a strong trigger that activates the resident microglia (macrophages) of the brain. During systemic inflammation, systemic inflammatory cytokines can enter brain tissue due to a disrupted blood-brain barrier, but also through several parts of the brain that lack a blood-brain barrier and directly activate microglial cells. As a result, systemic inflammation may result in an exaggerated neuroinflammatory cascade, which disrupts normal homeostasis and cell function and may lead to neuronal cell loss and cognitive deterioration (83, 84). Neuroinflammation is thought to contribute to both acute sepsis-associated encephalopathy, as well as long-term cognitive impairment following critical illness. Previously, research into immune responses of the brain during systemic inflammation was impossible in living patients as the brain is a body compartment not accessible for immunological research purposes without using the invasive procedure of brain biopsies. Recently, several innovative nuclear imaging tracers have been developed that can quantitatively measure microglial activation in vivo, by targeting the mitochondrial 18 kDa translocator protein (TSPO). Systemic inflammation evoked during experimental human endotoxemia was demonstrated to induce a 30–60% increase in microglial activation in healthy volunteers 3 h after administration of endotoxin (79). However, patients suffering from sepsis often develop more prolonged periods of systemic inflammation and a subsequent immunosuppressive state. The longitudinal effects of endotoxemia and systemic inflammation on tissue resident macrophage activation in the brain are unclear. A recent study in mice showed that repeated subjection to systemic inflammation on consecutive days induced a brain-specific training effect initially, followed by reduced immunological response (immune tolerance) of the brain after successive stimuli (85). In addition, a recent human study in prostate surgery patients found decreased microglial activity, measured by reduced microglial nuclear ligand binding, 3–4 days postoperatively compared to baseline (86). These results are the first indications that innate immunity responses in the brain show signs of immunoparalysis, coinciding with ex vivo and in vivo peripheral immunoparalysis.
Recent studies have demonstrated that microbial components can directly interact with hematopoietic stem and progenitor cells (HSPCs) in the bone marrow via Toll-like receptors (TLRs) expressed on HSPCs (87, 88). Microbial sensing by HSPCs during infection may therefore influence hematopoietic cell division and differentiation (88), and may ultimately impact the efficacy of host defenses during infection. Moreover, septic patients also exhibit reduced expression of HLA-DR in the myeloid lineage of the bone marrow (89). To date, the effects of systemic inflammation on HSPCs and their role in development and maintenance of hyperinflammatory responses or immunoparalysis in humans are unknown and represents an exciting field for further study.
Adipose tissue is increasingly recognized as an immunological organ, containing substantial amounts of adipose tissue macrophages (ATMs) (90). Advances on the interplay between metabolic and immunological research suggest an important role of the immune system in metabolic conditions such as obesity and diabetes mellitus (91). Lipids are important signaling moieties for both immune responses and metabolic regulation. Lipid infusion in vivo activates TLR4 signaling in adipocytes and macrophages and enhances inflammatory gene expression in adipose tissue (92) which adds to systemic insulin resistance. During obesity, many immune cells infiltrate in adipose tissue and promote a low-grade chronic inflammation (91). Conversely is the role of adipose tissue during systemic inflammation (e.g., sepsis) less well studied and human studies concerning the dynamics of immune suppression/tolerance in ATMs are completely lacking.
Future studies to characterize the immune response in other body compartments are ongoing and necessary to further characterize the in vivo immune status. These studies will improve the insight in the mechanistics of the immunological response during sepsis. It needs to be acknowledged that guiding immunotherapy in clinical practice based on other compartments than blood will however not be readily applicable, since tissue-resident immunological markers are not easily harvested or measured.
As mentioned earlier, previous therapeutic strategies for sepsis patients have virtually exclusively focused on blocking inflammation early in the course of sepsis. It has become clear that a considerable proportion of sepsis patients do not die from an overwhelming immune response and that suppressing the immune system is not an effective strategy when applied to all sepsis patients. Nevertheless, it is conceivable that a subgroup of hyperinflamed patients may have benefited from immunosuppressive therapy if they had been treated according to their immune status. For example, the phase 3 trial evaluating the immune suppressant IL-1 receptor antagonist (anakinra), performed more than 20 years ago, revealed no effect on mortality in severe sepsis patients (21). However, a post-hoc analysis of this study published many years later (93) demonstrated a significantly lower mortality in a subgroup of patients with MAS, which represented approximately 6% of the enrolled sepsis patients. Although no definitive conclusions can be drawn from this post-hoc analysis, it does suggest that a specific subgroup of patients in a hyperinflammatory state could benefit from immune suppressive therapy. Another argument that therapy aimed at inhibition of the immune response should not be discarded as of yet comes from a meta-analysis of 17 randomized controlled trials (almost 9,000 patients) evaluating the effects of anti-tumor necrosis factor alpha (TNF-α) therapy on mortality in severe sepsis. Despite negative results in each individual study, the pooled odds ratio showed a significantly reduced 28-day all cause mortality (94).
In the last decade, several immunostimulatory treatments have shown promise in preclinical, as well as in case series and/or small clinical studies (58, 95–104). Granulocyte-macrophage colony stimulating factor (GM-CSF) and interferon-gamma (IFNγ) are the most extensively investigated immunostimulatory agents in sepsis. These compounds are potent stimulators of myeloid cell function and they potentiate antigen presentation capabilities through increasing mHLA-DR expression on and pro-inflammatory cytokine production by monocytes (105, 106). A biomarker-guided (inclusion criterion mHLA-DR>8000 monoclonal antibodies per cell) randomized controlled trial comparing GM-CSF to placebo showed that GM-CSF was safe and effective in restoring monocytic immunocompetence. Exploratory endpoints suggested that treated patients had shorter duration of mechanical ventilation and a more swift decrease of disease severity scores (95). Although a meta-analysis of 4 RCT's did not reveal a beneficial effect of GM-CSF on 28-day mortality, patient numbers were probably too small for evaluation of this endpoint (107). Treatment with IFNγ resulted in increased mHLA-DR expression and restored TNFα production in a human endotoxemia study, while further attenuating production of the key anti-inflammatory cytokine IL-10 (43). Furthermore, IFNγ showed promising results in several small case series, for instance in patients suffering from opportunistic infections not responding to regular treatment (97). Targeting lymphocyte loss with apoptosis inhibitors has shown potential in animal studies (108–110) but could theoretically result in uncontrolled cell growth and organ injury as consequence of neutrophil accumulation in tissues. Recently, the IRIS-7 randomized controlled phase 2 trial was published, in which 27 patients with septic shock were treated with recombinant human IL-7 or placebo (111). In this trial, severe lymphopenia was used to identify immunosuppressed patients. The anti-apoptotic and lymphocyte function-enhancing cytokine IL-7 was well tolerated and reversed sepsis-induced lymphopenia in these patients. Naturally, statistical power to demonstrate clinically relevant treatment effects was inadequate. Another attractive immunostimulatory target is blockade of programmed death-1 (PD-1) or its ligand PD-L1. The PD-1 system is upregulated in sepsis patients (37) and inhibition of the interaction between PD-1 and its ligands promotes immune responses and antigen-specific T-cell responses. In recent years, positive responses to anti-PD-L1 therapy was demonstrated in the field of oncology and given the many similarities between the immunosuppressive mechanisms in cancer and sepsis, this could be promising for the potential of PD-L1 antagonism in sepsis-induced immunoparalysis. Unfortunately, a large multicenter trial was recently aborted by the sponsor due to other priorities (“CA209-9FH, a randomized, double-blind, placebo-controlled, parallel-group study to evaluate the efficacy and safety of nivolumab in adults with sepsis”). Table 1 summarizes the mechanism of action and the (clinical) evidence thus far of the immunomodulatory compounds described in this paragraph.
Fortunately, a number of clinical trials investigating these and other immunostimulatory treatments are currently underway or planned, illustrating the current interest and relevance of this type of treatment for sepsis3. However, these either do not use markers to enrich their patient population, or use biomarkers of which the accuracy and robustness has not been sufficiently demonstrated.
This review highlights the current challenges we face toward precision immunotherapy for patients suffering from sepsis. The urgent need for a patient-tailored approach in sepsis treatment is clear, as 40 years of undirected sepsis trials have not resulted in a single adjunctive therapy in current clinical use. Clearly, we need tools to determine whether hyperinflammation or immune suppression is the overriding immune dysfunction in a specific patient. The paradigm shift in the understanding of the pathophysiology of sepsis has resulted in increased interest for promising immunostimulatory therapies, but it is key to identify and select the appropriate patient population who may most likely benefit from these compounds. So far, current circulating biomarkers measured in blood have not been found sufficiently robust for use in clinical practice. From a pathophysiological perspective studies into other body compartments are warranted to increase our understanding of the in vivo immune response in patients with sepsis, but these insights will not be easily translated in feasible methodology for clinical practice. As such, finding a reliable biomarker to classify and monitor the overall immune response in patients with sepsis and to guide and personalize immunotherapy remains a holy grail.
MK and AP drafted, and WA and PP revised the manuscript. All the authors read and approved the final version of the manuscript.
WA is supported by a research grant from the Netherlands Organization for Health Research and Development (ZonMw Clinical Fellowship Grant 90715610). This funding agency had no role in the concept, design, and writing of this review, nor the collection or analysis of the literature presented.
The authors declare that the research was conducted in the absence of any commercial or financial relationships that could be construed as a potential conflict of interest.
1. ^Agency for Healthcare Research and Quality Healthcare Cost and Utilization Project Statistical Brief No 160. Available online at: https://www.hcup-us.ahrq.gov/reports/statbriefs/sb160.pdf. 2013; August.
2. ^Available online at: https://www.global-sepsis-alliance.org/news/2017/5/26/wha-adopts-resolution-on-sepsis. WHA resolution sepsis.
3. ^Clinicaltrials.gov registration no's: NCT02797431, NCT02576457, NCT02361528, NCT02867267.
1. Singer M, Deutschman CS, Seymour CW, Shankar-Hari M, Annane D, Bauer M, et al. The third international consensus definitions for sepsis and septic shock (Sepsis-3). JAMA (2016) 315:801–10. doi: 10.1001/jama.2016.0287
2. Fleischmann C, Scherag A, Adhikari NK, Hartog CS, Tsaganos T, Schlattmann P, et al. Assessment of global incidence and mortality of hospital-treated sepsis. current estimates and limitations. Am J Resp Crit Care Med. (2016) 193:259–72. doi: 10.1164/rccm.201504-0781OC
3. Reinhart K, Daniels R, Kissoon N, Machado FR, Schachter RD, Finfer S. Recognizing Sepsis as a global health priority-a WHO resolution. N Engl J Med. (2017) 377:414–7. doi: 10.1056/NEJMp1707170
4. Gaieski DF, Edwards JM, Kallan MJ, Carr BG. Benchmarking the incidence and mortality of severe sepsis in the United States. Crit Care Med. (2013) 41:1167–74. doi: 10.1097/CCM.0b013e31827c09f8
5. Angus DC, Linde-Zwirble WT, Lidicker J, Clermont G, Carcillo J, Pinsky MR. Epidemiology of severe sepsis in the United States: analysis of incidence, outcome, and associated costs of care. Crit Care Med. (2001) 29:1303–10. doi: 10.1097/00003246-200107000-00002
6. Marshall JC. Why have clinical trials in sepsis failed? Trends Mole Med. (2014) 20:195–203. doi: 10.1016/j.molmed.2014.01.007
7. Delano MJ, Ward PA. Sepsis-induced immune dysfunction: can immune therapies reduce mortality? J Clin Invest. (2016) 126:23–31. doi: 10.1172/JCI82224
8. Mebazaa A, Laterre PF, Russell JA, Bergmann A, Gattinoni L, Gayat E, et al. Designing phase 3 sepsis trials: application of learned experiences from critical care trials in acute heart failure. J Inten Care (2016) 4:24. doi: 10.1186/s40560-016-0151-6
9. Angus DC, van der Poll T. Severe sepsis and septic shock. N Engl J Med. (2013) 369:840–51. doi: 10.1056/NEJMra1208623
10. Venet F, Lukaszewicz AC, Payen D, Hotchkiss R, Monneret G. Monitoring the immune response in sepsis: a rational approach to administration of immunoadjuvant therapies. Curr Opin Immunol. (2013) 25:477–83. doi: 10.1016/j.coi.2013.05.006
11. Ranieri VM, Rubenfeld GD, Thompson BT, Ferguson ND, Caldwell E, Fan E, et al. Acute respiratory distress syndrome: the Berlin definition. JAMA (2012) 307:2526–33. doi: 10.1001/jama.2012.5669
12. Levi M, van der Poll T. Inflammation and coagulation. Crit Care Med. (2010) 38(2 Suppl):S26–34. doi: 10.1097/CCM.0b013e3181c98d21
13. Peters van Ton AM, Pickkers P, Abdo W. Systemic Inflammation and cerebral dysfunction. In: Vincent JL, editor. Annual Update in Intensive Care and Emergency Medicine. Brussels: Springer (2018). p. 487–501.
14. Veterans Administration Systemic Sepsis Cooperative Study Group. Effect of high-dose glucocorticoid therapy on mortality in patients with clinical signs of systemic sepsis. N Engl J Med. (1987) 317:659–65. doi: 10.1056/NEJM198709103171102
15. Lefering R, Neugebauer EA. Steroid controversy in sepsis and septic shock: a meta-analysis. Crit Care Med. (1995) 23:1294–303.
16. Sprung CL, Caralis PV, Marcial EH, Pierce M, Gelbard MA, Long WM, et al. The effects of high-dose corticosteroids in patients with septic shock. a prospective, controlled study. N Engl J. Med. (1984) 311:1137–43. doi: 10.1056/NEJM198411013111801
17. Bone RC, Fisher CJ Jr, Clemmer TP, Slotman GJ, Metz CA, Balk RA. A controlled clinical trial of high-dose methylprednisolone in the treatment of severe sepsis and septic shock. N Engl J Med. (1987) 317:653–8.
18. Sessler CN. Steroids for septic shock: back from the dead? (Con). Chest (2003) 123 (5 Suppl.):482S−9S. doi: 10.1378/chest.123.5_suppl.482s
19. Cronin L, Cook DJ, Carlet J, Heyland DK, King D, Lansang MA, et al. Corticosteroid treatment for sepsis: a critical appraisal and meta-analysis of the literature. Crit Care Med. (1995) 23:1430–9.
20. Fisher CJ Jr, Agosti JM, Opal SM, Lowry SF, Balk RA, Sadoff JC, et al. Treatment of septic shock with the tumor necrosis factor receptor:Fc fusion protein. the Soluble TNF receptor sepsis study group. N Engl J Med. (1996) 334:1697–702. doi: 10.1056/NEJM199606273342603
21. Opal SM, Fisher CJ Jr, Dhainaut JF, Vincent JL, Brase R, Lowry SF, et al. Confirmatory interleukin-1 receptor antagonist trial in severe sepsis: a phase III, randomized, double-blind, placebo-controlled, multicenter trial. the Interleukin-1 receptor antagonist sepsis investigator group. Crit Care Med. (1997) 25:1115–24.
22. McCloskey RV, Straube RC, Sanders C, Smith SM, Smith CR. Treatment of septic shock with human monoclonal antibody HA-1A. a randomized, double-blind, placebo-controlled trial. CHESS trial study group. Ann Inter Med. (1994) 121:1–5.
23. Angus DC, Birmingham MC, Balk RA, Scannon PJ, Collins D, Kruse JA, et al. E5 murine monoclonal antiendotoxin antibody in gram-negative sepsis: a randomized controlled trial. E5 study investigators. JAMA (2000) 283:1723–30. doi: 10.1001/jama.283.13.1723
24. Opal SM, Laterre PF, Francois B, LaRosa SP, Angus DC, Mira JP, et al. Effect of eritoran, an antagonist of MD2-TLR4, on mortality in patients with severe sepsis: the ACCESS randomized trial. JAMA (2013) 309:1154–62. doi: 10.1001/jama.2013.2194
25. Leentjens J, Kox M, van der Hoeven JG, Netea MG, Pickkers P. Immunotherapy for the adjunctive treatment of sepsis: from immunosuppression to immunostimulation. time for a paradigm change? Am J Respir Crit Care Med. (2013) 187:1287–93. doi: 10.1164/rccm.201301-0036CP
26. Hotchkiss RS, Monneret G, Payen D. Sepsis-induced immunosuppression: from cellular dysfunctions to immunotherapy. Nat Rev Immunol. (2013) 13:862–74. doi: 10.1038/nri3552
27. Hamers L, Kox M, Pickkers P. Sepsis-induced immunoparalysis: mechanisms, markers, and treatment options. Minerva anestesiol. (2015) 81:426–39.
28. Hotchkiss RS, Opal S. Immunotherapy for sepsis–a new approach against an ancient foe. N Engl J Med. (2010) 363:87–9. doi: 10.1056/NEJMcibr1004371
29. Patil NK, Bohannon JK, Sherwood ER. Immunotherapy: A promising approach to reverse sepsis-induced immunosuppression. Pharmacol Res. (2016) 111:688–702. doi: 10.1016/j.phrs.2016.07.019
30. Voll RE, Herrmann M, Roth EA, Stach C, Kalden JR, Girkontaite I. Immunosuppressive effects of apoptotic cells. Nature (1997) 390:350–1. doi: 10.1038/37022
31. Green DR, Beere HM. Apoptosis. Gone but not forgotten. Nature (2000) 405:28–9. doi: 10.1038/35011175
32. Heidecke CD, Hensler T, Weighardt H, Zantl N, Wagner H, Siewert JR, et al. Selective defects of T lymphocyte function in patients with lethal intraabdominal infection. Am J Surg. (1999) 178:288–92. doi: 10.1016/S0002-9610(99)00183-X
33. Wesche DE, Lomas-Neira JL, Perl M, Chung CS, Ayala A. Leukocyte apoptosis and its significance in sepsis and shock. J Leukocyte Biol. (2005) 78:325–37. doi: 10.1189/jlb.0105017
34. Drewry AM, Samra N, Skrupky LP, Fuller BM, Compton SM, Hotchkiss RS. Persistent lymphopenia after diagnosis of sepsis predicts mortality. Shock (2014) 42:383–91. doi: 10.1097/SHK.0000000000000234
35. Otto GP, Sossdorf M, Claus RA, Rodel J, Menge K, Reinhart K, et al. The late phase of sepsis is characterized by an increased microbiological burden and death rate. Crit Care (2011) 15:R183. doi: 10.1186/cc10332
36. Limaye AP, Kirby KA, Rubenfeld GD, Leisenring WM, Bulger EM, Neff MJ, et al. Cytomegalovirus reactivation in critically ill immunocompetent patients. JAMA (2008) 300:413–22. doi: 10.1001/jama.300.4.413
37. Boomer JS, To K, Chang KC, Takasu O, Osborne DF, Walton AH, et al. Immunosuppression in patients who die of sepsis and multiple organ failure. JAMA (2011) 306:2594–605. doi: 10.1001/jama.2011.1829
38. Muenzer JT, Davis CG, Chang K, Schmidt RE, Dunne WM, Coopersmith CM, et al. Characterization and modulation of the immunosuppressive phase of sepsis. Infect Immun. (2010) 78:1582–92. doi: 10.1128/IAI.01213-09
39. Torgersen C, Moser P, Luckner G, Mayr V, Jochberger S, Hasibeder WR, et al. Macroscopic postmortem findings in 235 surgical intensive care patients with sepsis. Anest Analg. (2009) 108:1841–7. doi: 10.1213/ane.0b013e318195e11d
40. Walton AH, Muenzer JT, Rasche D, Boomer JS, Sato B, Brownstein BH, et al. Reactivation of multiple viruses in patients with sepsis. PLoS ONE (2014) 9:e98819. doi: 10.1371/journal.pone.0098819
41. Cohen J, Opal S, Calandra T. Sepsis studies need new direction. Lancet Infect Dis. (2012) 12:503–5. doi: 10.1016/S1473-3099(12)70136-6
42. van Vught LA, Klein Klouwenberg PM, Spitoni C, Scicluna BP, Wiewel MA, Horn J, et al. Incidence, risk factors, and attributable mortality of secondary infections in the intensive care unit after admission for sepsis. JAMA (2016) 315:1469–79. doi: 10.1001/jama.2016.2691
43. Leentjens J, Kox M, Koch RM, Preijers F, Joosten LA, van der Hoeven JG, et al. Reversal of immunoparalysis in humans in vivo: a double-blind, placebo-controlled, randomized pilot study. Am J Resp Crit Care Med. (2012) 186:838–45. doi: 10.1164/rccm.201204-0645OC
44. Faix JD. Biomarkers of sepsis. Crit Rev Clin Lab Sci. (2013) 50:23–36. doi: 10.3109/10408363.2013.764490
45. Batfalsky A, Lohr A, Heussen N, Neunhoeffer F, Orlikowsky TW. Diagnostic value of an interleukin-6 bedside test in term and preterm neonates at the time of clinical suspicion of early- and late-onset bacterial infection. Neonatology (2012) 102:37–44. doi: 10.1159/000336632
46. Patel RT, Deen KI, Youngs D, Warwick J, Keighley MR. Interleukin 6 is a prognostic indicator of outcome in severe intra-abdominal sepsis. Br J Surg. (1994) 81:1306–8. doi: 10.1002/bjs.1800810914
47. Pettila V, Hynninen M, Takkunen O, Kuusela P, Valtonen M. Predictive value of procalcitonin and interleukin 6 in critically ill patients with suspected sepsis. Inten Care Med. (2002) 28:1220–5. doi: 10.1007/s00134-002-1416-1
48. Stryjewski GR, Nylen ES, Bell MJ, Snider RH, Becker KL, Wu A, et al. Interleukin-6, interleukin-8, and a rapid and sensitive assay for calcitonin precursors for the determination of bacterial sepsis in febrile neutropenic children. Pediatr Crit Care Med. (2005) 6:129–35. doi: 10.1097/01.PCC.0000149317.15274.48
49. Bozza FA, Salluh JI, Japiassu AM, Soares M, Assis EF, Gomes RN, et al. Cytokine profiles as markers of disease severity in sepsis: a multiplex analysis. Crit Care (2007) 11:R49. doi: 10.1186/cc5783
50. Hofer N, Zacharias E, Muller W, Resch B. An update on the use of C-reactive protein in early-onset neonatal sepsis: current insights and new tasks. Neonatology (2012) 102:25–36. doi: 10.1159/000336629
51. Assicot M, Gendrel D, Carsin H, Raymond J, Guilbaud J, Bohuon C. High serum procalcitonin concentrations in patients with sepsis and infection. Lancet (1993) 341:515–8. doi: 10.1016/0140-6736(93)90277-N
52. Bopp C, Hofer S, Weitz J, Bierhaus A, Nawroth PP, Martin E, et al. sRAGE is elevated in septic patients and associated with patients outcome. J Surg Res. (2008) 147:79–83. doi: 10.1016/j.jss.2007.07.014
53. Schulert GS, Grom AA. Pathogenesis of macrophage activation syndrome and potential for cytokine- directed therapies. Ann Rev Med. (2015) 66:145–59. doi: 10.1146/annurev-med-061813-012806
54. Carson WF, Cavassani KA, Dou Y, Kunkel SL. Epigenetic regulation of immune cell functions during post-septic immunosuppression. Epigenetics (2011) 6:273–83. doi: 10.4161/epi.6.3.14017
55. Lukaszewicz AC, Grienay M, Resche-Rigon M, Pirracchio R, Faivre V, Boval B, et al. Monocytic HLA-DR expression in intensive care patients: interest for prognosis and secondary infection prediction. Crit Care Med. (2009) 37:2746–52. doi: 10.1097/CCM.0b013e3181ab858a
56. Landelle C, Lepape A, Voirin N, Tognet E, Venet F, Bohe J, et al. Low monocyte human leukocyte antigen-DR is independently associated with nosocomial infections after septic shock. Inten Care Med. (2010) 36:1859–66. doi: 10.1007/s00134-010-1962-x
57. Heagy W, Hansen C, Nieman K, Cohen M, Richardson C, Rodriguez JL, et al. Impaired ex vivo lipopolysaccharide-stimulated whole blood tumor necrosis factor production may identify “septic” intensive care unit patients. Shock (2000) 14:271–6; discussion 276–7. doi: 10.1097/00024382-200014030-00005
58. Zhang Y, Li J, Lou J, Zhou Y, Bo L, Zhu J, et al. Upregulation of programmed death-1 on T cells and programmed death ligand-1 on monocytes in septic shock patients. Crit Care. (2011) 15:R70. doi: 10.1186/cc10059
59. Inoue S, Bo L, Bian J, Unsinger J, Chang K, Hotchkiss RS. Dose-dependent effect of anti-CTLA-4 on survival in sepsis. Shock (2011) 36:38–44. doi: 10.1097/SHK.0b013e3182168cce
60. Sherwood ER, Hotchkiss RS. BTLA as a biomarker and mediator of sepsis-induced immunosuppression. Crit Care (2013) 17:1022. doi: 10.1186/cc13143
61. Kessel A, Bamberger E, Masalha M, Toubi E. The role of T regulatory cells in human sepsis. J Autoimmun. (2009) 32:211–5. doi: 10.1016/j.jaut.2009.02.014
62. Xiong Y, Medvedev AE. Induction of endotoxin tolerance in vivo inhibits activation of IRAK4 and increases negative regulators IRAK-M, SHIP-1, and A20. J Leukoc Biol. (2011) 90:1141–8. doi: 10.1189/jlb.0611273
63. Netea MG, Kullberg BJ, Blok WL, Netea RT, van der Meer JW. The role of hyperuricemia in the increased cytokine production after lipopolysaccharide challenge in neutropenic mice. Blood (1997) 89:577–82.
64. Biswas SK, Lopez-Collazo E. Endotoxin tolerance: new mechanisms, molecules and clinical significance. Trends Immunol. (2009) 30:475–87. doi: 10.1016/j.it.2009.07.009
65. Cheng SC, Scicluna BP, Arts RJ, Gresnigt MS, Lachmandas E, Giamarellos-Bourboulis EJ, et al. Broad defects in the energy metabolism of leukocytes underlie immunoparalysis in sepsis. Nat Immunol. (2016) 17:406–13. doi: 10.1038/ni.3398
66. Varma TK, Durham M, Murphey ED, Cui W, Huang Z, Lin CY, et al. Endotoxin priming improves clearance of Pseudomonas aeruginosa in wild-type and interleukin-10 knockout mice. Infect Immun. (2005) 73:7340–7. doi: 10.1128/IAI.73.11.7340-7347.2005
67. Murphey ED, Fang G, Varma TK, Sherwood ER. Improved bacterial clearance and decreased mortality can be induced by LPS tolerance and is not dependent upon IFN-gamma. Shock (2007) 27:289–95. doi: 10.1097/01.shk.0000245024.93740.28
68. Murphey ED, Fang G, Sherwood ER. Endotoxin pretreatment improves bacterial clearance and decreases mortality in mice challenged with Staphylococcus aureus. Shock (2008) 29:512–8. doi: 10.1097/shk.0b013e318150776f
69. Murphey ED, Sherwood ER. Pretreatment with the Gram-positive bacterial cell wall molecule peptidoglycan improves bacterial clearance and decreases inflammation and mortality in mice challenged with Pseudomonas aeruginosa. Microbes Infect. (2008) 10:1244–50. doi: 10.1016/j.micinf.2008.07.021
70. Kox M, de KS, Pompe JC, Ramakers BP, Netea MG, van der Hoeven JG, et al. Differential ex vivo and in vivo endotoxin tolerance kinetics following human endotoxemia. Crit Care Med. (2011) 39:1866–70. doi: 10.1097/CCM.0b013e3182190d5d
71. de Vos AF, Pater JM, van den Pangaart PS, de Kruif MD, van 't Veer C, van der Poll T. In vivo lipopolysaccharide exposure of human blood leukocytes induces cross-tolerance to multiple TLR ligands. J Immunol. (2009) 183:533–42. doi: 10.4049/jimmunol.0802189
72. van der Poll T, Coyle SM, Moldawer LL, Lowry SF. Changes in endotoxin-induced cytokine production by whole blood after in vivo exposure of normal humans to endotoxin. J Infect Dis. (1996) 174:1356–60. doi: 10.1093/infdis/174.6.1356
73. Bos LD, Martin-Loeches I, Schultz MJ. ARDS: challenges in patient care and frontiers in research. Eur Resp Rev. (2018) 27:170107. doi: 10.1183/16000617.0107-2017
74. Song Z, Zhang J, Zhang X, Li D, Wang H, Xu X, et al. Interleukin 4 deficiency reverses development of secondary Pseudomonas aeruginosa pneumonia during sepsis-associated immunosuppression. J Infect Dis. (2015) 211:1616–27. doi: 10.1093/infdis/jiu668
75. Hutchins NA, Unsinger J, Hotchkiss RS, Ayala A. The new normal: immunomodulatory agents against sepsis immune suppression. Trends Mol Med. (2014) 20:224–33. doi: 10.1016/j.molmed.2014.01.002
76. Smith PD, Suffredini AF, Allen JB, Wahl LM, Parrillo JE, Wahl SM. Endotoxin administration to humans primes alveolar macrophages for increased production of inflammatory mediators. J Clin Immunol. (1994) 14:141–8.
77. Simpson SQ, Modi HN, Balk RA, Bone RC, Casey LC. Reduced alveolar macrophage production of tumor necrosis factor during sepsis in mice and men. Crit Care Med. (1991) 19:1060–6.
78. Mokart D, Guery BP, Bouabdallah R, Martin C, Blache JL, Arnoulet C, et al. Deactivation of alveolar macrophages in septic neutropenic ARDS. Chest (2003) 124:644–52. doi: 10.1378/chest.124.2.644
79. Sandiego CM, Gallezot JD, Pittman B, Nabulsi N, Lim K, Lin SF, et al. Imaging robust microglial activation after lipopolysaccharide administration in humans with PET. Proc Nat Acad Sci USA. (2015) 112:12468–73. doi: 10.1073/pnas.1511003112
80. Hoogland IC, Houbolt C, van Westerloo DJ, van Gool WA, van de Beek D. Systemic inflammation and microglial activation: systematic review of animal experiments. J Neuroinflamm. (2015) 12:114. doi: 10.1186/s12974-015-0332-6
81. Lemstra AW, Groen in't Woud JC, Hoozemans JJ, van Haastert ES, Rozemuller AJ, Eikelenboom P, et al. Microglia activation in sepsis: a case-control study. J Neuroinflamm. (2007) 4:4. doi: 10.1186/1742-2094-4-4
82. Sharshar T, Annane D, de la Grandmaison GL, Brouland JP, Hopkinson NS, Francoise G. The neuropathology of septic shock. Brain Pathol. (2004) 14:21–33. doi: 10.1111/j.1750-3639.2004.tb00494.x
83. Block ML, Zecca L, Hong JS. Microglia-mediated neurotoxicity: uncovering the molecular mechanisms. Nat Rev Neurosci. (2007) 8:57–69. doi: 10.1038/nrn2038
84. Godbout JP, Chen J, Abraham J, Richwine AF, Berg BM, Kelley KW, et al. Exaggerated neuroinflammation and sickness behavior in aged mice following activation of the peripheral innate immune system. FASEB J. (2005) 19:1329–31. doi: 10.1096/fj.05-3776fje
85. Wendeln AC, Degenhardt K, Kaurani L, Gertig M, Ulas T, Jain G, et al. Innate immune memory in the brain shapes neurological disease hallmarks. Nature (2018) 556:332–8. doi: 10.1038/s41586-018-0023-4
86. Forsberg A, Cervenka S, Jonsson Fagerlund M, Rasmussen LS, Zetterberg H, Erlandsson Harris H, et al. The immune response of the human brain to abdominal surgery. Ann Neurol. (2017). 81:572–82. doi: 10.1002/ana.24909
87. Sioud M, Floisand Y, Forfang L, Lund-Johansen F. Signaling through toll-like receptor 7/8 induces the differentiation of human bone marrow CD34+ progenitor cells along the myeloid lineage. J Mol Biol. (2006) 364:945–54. doi: 10.1016/j.jmb.2006.09.054
88. Nagai Y, Garrett KP, Ohta S, Bahrun U, Kouro T, Akira S, et al. Toll-like receptors on hematopoietic progenitor cells stimulate innate immune system replenishment. Immunity (2006) 24:801–12. doi: 10.1016/j.immuni.2006.04.008
89. Faivre V, Lukaszewicz AC, Payen D. Downregulation of blood monocyte HLA-DR in ICU patients is also present in bone marrow cells. PLoS ONE (2016) 11:e0164489. doi: 10.1371/journal.pone.0164489
90. Grant RW, Dixit VD. Adipose tissue as an immunological organ. Obesity (2015) 23:512–8. doi: 10.1002/oby.21003
91. Sun S, Ji Y, Kersten S, Qi L. Mechanisms of inflammatory responses in obese adipose tissue. Ann Rev Nutr. (2012) 32:261–86. doi: 10.1146/annurev-nutr-071811-150623
92. Shi H, Kokoeva MV, Inouye K, Tzameli I, Yin H, Flier JS. TLR4 links innate immunity and fatty acid-induced insulin resistance. J Clin Invest. (2006) 116:3015–25. doi: 10.1172/JCI28898
93. Shakoory B, Carcillo JA, Chatham WW, Amdur RL, Zhao H, Dinarello CA, et al. Interleukin-1 Receptor blockade is associated with reduced mortality in sepsis patients with features of macrophage activation syndrome: reanalysis of a prior phase III trial. Crit Care Med. (2016) 44:275–81. doi: 10.1097/CCM.0000000000001402
94. Lv S, Han M, Yi R, Kwon S, Dai C, Wang R. Anti-TNF-alpha therapy for patients with sepsis: a systematic meta-analysis. Inter J Clin Pract. (2014) 68:520–8. doi: 10.1111/ijcp.12382
95. Meisel C, Schefold JC, Pschowski R, Baumann T, Hetzger K, Gregor J, et al. Granulocyte-macrophage colony-stimulating factor to reverse sepsis-associated immunosuppression: a double-blind, randomized, placebo-controlled multicenter trial. Am J Respir Crit Care Med. (2009) 180:640–8. doi: 10.1164/rccm.200903-0363OC
96. Hall MW, Knatz NL, Vetterly C, Tomarello S, Wewers MD, Volk HD, et al. Immunoparalysis and nosocomial infection in children with multiple organ dysfunction syndrome. Inten Care Med. (2011) 37:525–32. doi: 10.1007/s00134-010-2088-x
97. Delsing CE, Gresnigt MS, Leentjens J, Preijers F, Frager FA, Kox M, et al. Interferon-gamma as adjunctive immunotherapy for invasive fungal infections: a case series. BMC Infect Dis. (2014) 14:166. doi: 10.1186/1471-2334-14-166
98. Nalos M, Santner-Nanan B, Parnell G, Tang B, McLean AS, Nanan R. Immune effects of interferon gamma in persistent staphylococcal sepsis. Am J Resp Crit Care Med. (2012) 185:110–2. doi: 10.1164/ajrccm.185.1.110
99. Unsinger J, McGlynn M, Kasten KR, Hoekzema AS, Watanabe E, Muenzer JT, et al. IL-7 promotes T cell viability, trafficking, and functionality and improves survival in sepsis. J Immunol. (2010) 184:3768–79. doi: 10.4049/jimmunol.0903151
100. Kasten KR, Prakash PS, Unsinger J, Goetzman HS, England LG, Cave CM, et al. Interleukin-7 (IL-7) treatment accelerates neutrophil recruitment through gamma delta T-cell IL-17 production in a murine model of sepsis. Infect Immun. (2010) 78:4714–22. doi: 10.1128/IAI.00456-10
101. Chang KC, Burnham CA, Compton SM, Rasche DP, Mazuski R, Smcdonough J, et al. Blockade ofthe negative co-stimulatory molecules PD-1 and CTLA-4 improves survival in primary and secondary fungal sepsis. Crit Care (2013) 17:R85. doi: 10.1186/cc12711
102. Zhang Y, Zhou Y, Lou J, Li J, Bo L, Zhu K, et al. PD-L1 blockade improves survival in experimental sepsis by inhibiting lymphocyte apoptosis and reversing monocyte dysfunction. Crit Care. (2010) 14:R220. doi: 10.1186/cc9354
103. Hotchkiss RS, Monneret G, Payen D. Immunosuppression in sepsis: a novel understanding of the disorder and a new therapeutic approach. Lancet Infect Dis. (2013) 13:260–8. doi: 10.1016/S1473-3099(13)70001-X
104. Chang K, Svabek C, Vazquez-Guillamet C, Sato B, Rasche D, Wilson S, et al. Targeting the programmed cell death 1: programmed cell death ligand 1 pathway reverses T cell exhaustion in patients with sepsis. Crit Care (2014) 18:R3. doi: 10.1186/cc13176
105. Flohe S, Lendemans S, Selbach C, Waydhas C, Ackermann M, Schade FU, et al. Effect of granulocyte-macrophage colony-stimulating factor on the immune response of circulating monocytes after severe trauma. Crit Care Med. (2003) 31:2462–9. doi: 10.1097/01.CCM.0000089640.17523.57
106. Kox WJ, Bone RC, Krausch D, Docke WD, Kox SN, Wauer H, et al. Interferon gamma-1b in the treatment of compensatory anti-inflammatory response syndrome. a new approach: proof of principle. Archi Inter Med. (1997) 157:389–93. doi: 10.1001/archinte.1997.00440250031004
107. Bo L, Wang F, Zhu J, Li J, Deng X. Granulocyte-colony stimulating factor (G-CSF) and granulocyte-macrophage colony stimulating factor (GM-CSF) for sepsis: A meta-analysis. Crit Care (2011) 15:R58. doi: 10.1186/cc10031
108. Hotchkiss RS, Chang KC, Swanson PE, Tinsley KW, Hui JJ, Klender P, et al. Caspase inhibitors improve survival in sepsis: a critical role of the lymphocyte. Nat Immunol. (2000) 1:496–501. doi: 10.1038/82741
109. Hotchkiss RS, Tinsley KW, Swanson PE, Chang KC, Cobb JP, Buchman TG, et al. Prevention of lymphocyte cell death in sepsis improves survival in mice. Proc Nat Acad Sci USA. (1999) 96:14541–6.
110. Weaver JG, Rouse MS, Steckelberg JM, Badley AD. Improved survival in experimental sepsis with an orally administered inhibitor of apoptosis. FASEB J. (2004) 18:1185–91. doi: 10.1096/fj.03-1230com
Keywords: sepsis, hyperinflammation, immunoparalysis, immunosuppressive therapy, immunostimulatory therapy, biomarkers, precision medicine
Citation: Peters van Ton AM, Kox M, Abdo WF and Pickkers P (2018) Precision Immunotherapy for Sepsis. Front. Immunol. 9:1926. doi: 10.3389/fimmu.2018.01926
Received: 05 July 2018; Accepted: 06 August 2018;
Published: 05 September 2018.
Edited by:
Thierry Roger, Centre Hospitalier Universitaire Vaudois (CHUV), SwitzerlandReviewed by:
Edward Sherwood, Vanderbilt University Medical Center, United StatesCopyright © 2018 Peters van Ton, Kox, Abdo and Pickkers. This is an open-access article distributed under the terms of the Creative Commons Attribution License (CC BY). The use, distribution or reproduction in other forums is permitted, provided the original author(s) and the copyright owner(s) are credited and that the original publication in this journal is cited, in accordance with accepted academic practice. No use, distribution or reproduction is permitted which does not comply with these terms.
*Correspondence: Peter Pickkers, cGV0ZXIucGlja2tlcnNAcmFkYm91ZHVtYy5ubA==
Disclaimer: All claims expressed in this article are solely those of the authors and do not necessarily represent those of their affiliated organizations, or those of the publisher, the editors and the reviewers. Any product that may be evaluated in this article or claim that may be made by its manufacturer is not guaranteed or endorsed by the publisher.
Research integrity at Frontiers
Learn more about the work of our research integrity team to safeguard the quality of each article we publish.