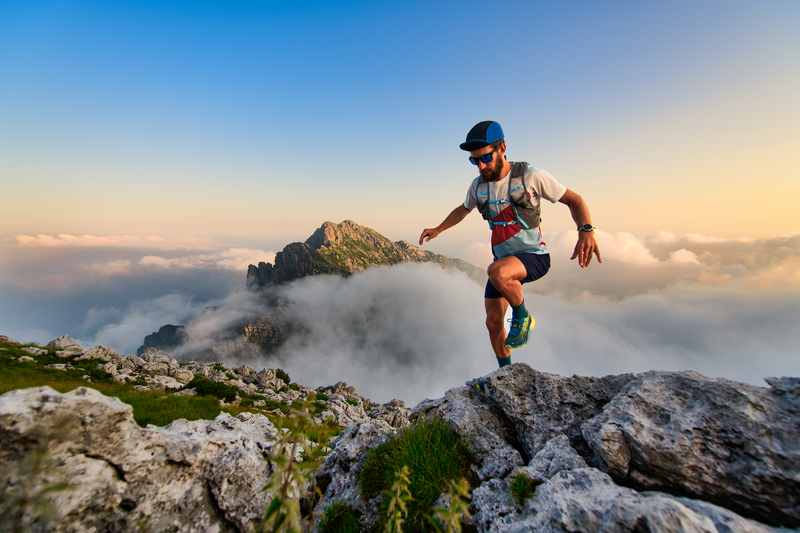
94% of researchers rate our articles as excellent or good
Learn more about the work of our research integrity team to safeguard the quality of each article we publish.
Find out more
ORIGINAL RESEARCH article
Front. Immunol. , 30 July 2018
Sec. Vaccines and Molecular Therapeutics
Volume 9 - 2018 | https://doi.org/10.3389/fimmu.2018.01705
Skin vaccination using biodegradable microneedle patch (MNP) technology in vaccine delivery is a promising strategy showing significant advantages over conventional flu shots. In this study, we developed an MNP encapsulating a 4M2e-tFliC fusion protein and two types of whole inactivated influenza virus vaccines (H1N1 and H3N2) as a universal vaccine candidate. We demonstrated that mice receiving this tri-component influenza vaccine via MNP acquired improved IgG1 antibody responses with more balanced IgG1/IgG2a antibody responses and enhanced cellular immune responses, including increased populations of IL-4 and IFN-γ producing cells and higher frequencies of antigen-specific plasma cells compared with intramuscular injection. In addition, stronger germinal center reactions, increased numbers of Langerin-positive migratory dendritic cells, and increased cytokine secretion were observed in the skin-draining lymph nodes after immunization with the tri-component influenza MNP vaccine. The MNP-immunized group also possessed enhanced protection against a heterologous reassortant A/Shanghai/2013 H7N9 (rSH) influenza virus infection. Furthermore, the sera collected from 4M2e-tFliC MNP-immunized mice were demonstrated to have antiviral efficacy against reassortant A/Vietnam/1203/2004 H5N1 (rVet) and A/Shanghai/2013 H7N9 (rSH) virus challenges. The immunological advantages of skin vaccination with this tri-component MNP vaccine could offer a promising approach to develop an easily applicable and broadly protective universal influenza vaccine.
Influenza virus infection results in high morbidity and mortality in most flu seasons (1–3). Although influenza affects all humans, some groups—including the elderly, infants, children under 5 years old, pregnant women, and people with chronic diseases—are much more vulnerable to influenza virus infection and have increased mortality rates (4–6). Vaccination has been proven to be an effective way to prevent influenza virus infection. However, the protective efficacy of the seasonal flu vaccines is greatly compromised by the high antigenic variation of flu. For instance, the influenza pandemic outbreak in 2009–2010 occurred because of the antigenic drift of an influenza strain rendering the seasonal vaccine ineffective (7). The 2017–2018 flu season demonstrated a widespread outbreak of flu in most of the US. The overall vaccine effectiveness in the 2017–2018 season was 36% overall and only 25% against the H3N2 virus (8). In addition, the highly pathogenic avian influenzas H5N1 and H7N9 have infected humans with high fatality rates in recent years, indicating the possibility of new influenza pandemics in the future.
The first human infection by H7N9 was reported in 2013 and has since caused five seasonal outbreaks and a total of 1,223 infections in humans with a ~40% mortality rate (9, 10). Furthermore, it is reported that the latest H7N9 isolates have diverged into two lineages, the Yangtze River Delta and Pearl River Delta, in which only the latter lineage seems to be sensitive to the existing H7N9 vaccines. This is an example where genetic drift has resulted in a mismatch between the strains in the wild and the potential vaccine strain and reduced our overall preparedness for a flu pandemic. New candidate vaccine strains will need to be included in the H7N9 vaccine formulations to ensure protection.
As the outcome of molecular evolution, H7N9 virus continuously adapts and grows in mammalian species (11–14). Some other mutations have occurred when facing therapeutic pressure (15, 16). The newly emerging H7N9 virus displays increased resistance to neuraminidase inhibitors and poor clinical treatment outcomes. Increased human infections by H7N9 influenza viruses and its rapid divergence have raised concerns and increased interest in the development of broadly protective and rapidly dispersible influenza vaccines.
The promise and effectiveness of skin vaccination is being recognized by researchers, enabled by the novel technology of dissolving microneedle patches (MNPs) (17–20). Vaccines can be delivered by MNP into the epidermis and dermis of the skin, which is a promising vaccination site harboring abundant lymphatic vessels and many different types of immune cells. These skin-migrated and -resident leukocytes are important inducers of the innate immune response and regulators of adaptive immunity (21, 22).
In a recent study, we designed a 4M2e-tFliC construct in which we replaced the hyperimmunogenic region of FliC with four M2e sequences from different influenza subtypes. We found that an M2e-tFliC encapsulated MNP-boosting skin immunization could rapidly broaden the immunity generated by a conventional influenza vaccine prime to confer cross-protection against heterologous virus challenge (23).
M2 is a conserved surface antigen and a promising target for the development of universal influenza vaccines. Results of ours and others have demonstrated that vaccines using recombinant tandem M2e sequences containing the human consensus sequence or diverse sequences from multiple species provided broader protection against influenza virus challenges (16–22). M2e vaccines were found to synergize the efficacy of other influenza vaccines. Therefore, encapsulating M2e vaccines and conventional influenza vaccines into a single MNP is a promising and convenient vaccine strategy to provide broad protection against influenza virus infection.
In this study, we investigated whether skin vaccination with MNPs encapsulating 4M2e-tFliC fusion protein and a divalent inactivated vaccine (DIV) (A/Aichi/2/68, H3N2, Aichi, and A/PR/8/34, H1N1, PR8) can induce increased innate and adaptive immune responses capable of cross-protection against a reassortant H7N9 virus infection.
All animal experiments were performed in accordance with the protocol (protocol number A16029) approved by Georgia State University’s Institutional Animal Care and Use Committee (IACUC). This study was in strict compliance with the Animal Welfare Act Regulations, the Public Health Service (PHS) Policy on Humane Care and Use of Laboratory Animals, and the Guide for the Care and Use of Laboratory Animals.
The 4M2e-tFliC fusion protein was purified and identified as previously described (23, 24). For animal studies, two groups of 6- to 8-week-old female BALB/c mice (Harlan Laboratories, Indianapolis, IN, USA) were intramuscularly or MNP skin immunized with tri-component vaccines including 3.2 µg of the 4M2e-tFliC fusion protein, 1.6 µg of HA equivalent Aichi, and 1.6 µg of HA equivalent PR8 whole inactivated influenza virus vaccines. One group of mice were given 1.6 µg of both HA equivalent Aichi and PR8 inactivated influenza vaccines (the DIV group). One group of naïve mice was used as a control. For MNP skin application, mice were shaved and treated with hair remover lotion 2 days prior to the immunization. MNPs were firmly held on the shaved dorsal area for 1 min and then left on the skin for 30 min. At week 4, mice were challenged with 2 × LD50 of a reassortant A/Vietnam/1203/2004 H5N1 or 2 × LD50 of a reassortant A/Shanghai/2013 H7N9 [a recombinant virus containing HA and NA from A/Shanghai (H7N9) or A/Vietnam (H5N1) with PR8 backbone, designated rSH and rVet, respectively] and their body weights were monitored daily for 14 days (25).
Mice were primed and boosted with 5 µg HA equivalent of PR8 and Aichi inactivated vaccines at week 0 and week 3, respectively. One group of mice was immunized with 4M2e-tFliC MNPs after the boosting immunization. Three weeks after 4M2e-tFliC MNPs immunization (week 9 after the primary vaccination), two groups of inactivated vaccine immunized mice were intraperitoneally (IP) injected with 200 µl of naïve serum or 4M2e-tFliC immune serum 2 h before 2 × LD50 of rVet and rSH virus challenges. One group of naïve mice were injected with 4M2e-tFliC serum as a control. 4M2e-tFliC immune serum was collected and pooled at 3 weeks after mice receiving the 4M2e-tFliC MNPs immunization.
Madin–Darby canine kidney (ATCC) cells were cultured as described previously (26). Mouse-adapted influenza A/PR/8/34 (H1N1, PR8), A/Aichi/2/68 (H3N2, Aichi), rSH, and rVet were grown and titrated in the lab (27). The 4M2e-tFliC fusion protein was designed with four different M2e sequences from four different viruses: A/California/07/2009 (H1N1, CA09), A/Aichi/2/68 (H3N2, Aichi), A/Avian/Washington/2014 (H5N1), and A/Avian/Shanghai/2013 (H7N9). Production and determination of the whole inactivated influenza virus vaccines (PR8 and Aichi) and the 4M2e-tFliC fusion protein was done as previously described (23).
Microneedle patches containing 100 solid, conical microneedles (250-µm diameter at the base and 650-µm long) were fabricated using a two-step molding process on polydimethylsiloxane (PDMS) molds. The first filling solution was a mixture containing 0.5 μg/μl A/Aichi, 0.5 μg/μl A/PR8, 1 µg/µl 4M2e-tFliC, 1% (w/w) sodium carboxymethyl cellulose (CMC-Na), and 10% (w/w) sucrose in 100 mM dibasic potassium phosphate buffer pH 7.4, which was prepared by mixing the different components in the desired ratios. This solution was cast on PDMS molds under vacuum to facilitate filling the MN cavities with the solution. After 30 min, excess solution was removed. The filled molds were then left under vacuum for another 20 min. The second filling solution, containing 18% (w/w) PVA and 18% (w/w) sucrose, was then cast on the filled PDMS molds. This solution was dried under vacuum for another 3 h and then further dried at 35°C overnight before demolding the patches. The patches were immediately stored with desiccant in individually sealed pouches before application. The stability and delivery efficiency of MNPs in vivo were determined as previously described (23).
Blood samples were collected at 3 weeks post-immunization. The levels of Aichi, PR8, and M2e-specific IgG and IgG isotype (IgG1 and IgG2a) antibody titers were measured by ELISA using 4 µg/ml of purified Aichi and PR8 viruses or synthetic M2e peptides as coating antigens, respectively. Mice bronchoalveolar lavage fluids (BALF) and lungs were collected 5 days post challenge, and the measurement of M2e-specific IgG titers in mouse BALF was done by ELISA. Hemagglutinin inhibition (HAI) titers were performed following the WHO manual (28).
Mice were sacrificed at week 4 post-immunization. Spleens and bone marrow were collected and processed into single-cell suspensions with complete RPMI media as previously described (29). Antigen-specific IL-4-, IL-2-, and IFN-γ-secreting cells and virus-specific antibody-secreting cells (ASCs) were determined by an ELISPOT assay as previously described (23).
Inguinal lymph nodes (ILNs) and spleens were collected 7 days post-immunization for the FACS evaluation of germinal center (GC) reactions, including CD4+ follicular T helper cells and B cells. 106/100 μl diluted single-cell suspensions were stained with CD4-PE-Cy7, CXCR5-FITC, B220-APC, and GL-7-FITC antibodies for 30 min on ice. Single-cell suspensions from ILNs were also used for the detection of migratory dendritic cells (DCs) with MHCII-PE, CD11c-APC, Langerin-PE, CD11b-Percp/Cy5.5, and CD103-FITC antibodies. The isolation of mouse skin lymphocytes was conducted following a previously described protocol (30). Antibodies were purchased from BD Biosciences and BioLegend. Cells were extensively washed and analyzed by a Fortessa Flow cytometer (BD Biosciences).
Lungs were collected 5 days post challenge. The lung homogenates were prepared for viral titer detection via plaque assay as described previously and calculated with the Reed–Muench method (26).
Single-cell suspensions of ILNs were cultured in vitro with PBS and 4 µg/ml of the 4M2e-tFliC fusion protein or PR8 inactivated virus as stimulators for 5 days at 37°C. The supernatants were collected 5 days later to determine the IL-2, IL-4, IL-6, IL-12/p40, and IL-17A levels by cytokine ELISA. Briefly, 96-well plates (MaxiSorp, Nunc) were coated with LEAF™ Purified anti-mouse IL-2, IL-4, IL-6, IL-12/p40, or IL-17A antibodies (BioLegend) at 4°C overnight. After blocking, 100 µl supernatant was added and incubated at 37°C for 2 h. Plates were then washed with PBST and incubated with Biotin anti-mouse IL-2, IL-4, IL-6, IL-12/p40, or IL-17A antibodies (BioLegend) at 37°C for 1 h. After washing with PBST, plates were incubated with streptavidin-HRP (BioLegend) for 1 h and treated with TMB substrate (Thermo Fisher Scientific) for reaction and subsequently 0.18 M H2SO4 for termination.
A Shapiro–Wilk Normality test was employed to check the data distribution. A two-tailed Student’s t-test was performed to compare the difference significance between two groups if data showing normal distribution. Otherwise, a Mann–Whitney U test was used. Statistical results were shown in each figure. A p value <0.05 was considered to be statistically significant. p < 0.05 (*), p < 0.01 (**), p < 0.001 (***), p > 0.05 (n.s).
The efficacy of skin vaccination via dissolving MNP was compared with traditional intramuscular (IM) route. The dissolving MNP encapsulated 3.2 µg of the 4M2e-tFliC fusion protein and 1.6 µg of each component of the DIV (PR8 and Aichi) (tri-component). One IM vaccine group received the same dose of the tri-component vaccine. Another IM vaccine group received only the DIV.
Mice immunized with MNPs or IM showed similar levels of Aichi- or PR8-specific IgG endpoint titers and HAI titers at week 3 post-immunization (p > 0.05) (Figures 1A,B). IgG1 and IgG2a could indicate the Th2 and Th1 type cell responses, and then we also detected the levels of these virus-specific IgG isotypes. We observed significantly pronounced IgG1 titers in MNP-immunized mice (PR8 and Aichi-specific IgG1/IgG2a ratio = 1.158 and 0.812) compared with the tri-component IM group (PR8 and Aichi-specific IgG1/IgG2a ratio = 0.198 and 0.103) or the DIV IM group (PR8 and Aichi-specific IgG1/IgG2a ratio = 0.063 and 0.063) (Figures 1C,D). The DIV and the tri-component IM immunizations induced a Th1-biased immune response. The supplementation of 4M2e-tFliC to the DIV increased the ratio of the IgG1/IgG2a through MNPs immunization. Meanwhile, immunization with the tri-component vaccine with MNPs elicited a more balanced IgG1/IgG2a ratio, which means MNPs immunization induced strong Th1 cell responses and potent Th2 cell responses as well.
Figure 1. Evaluation of humoral immune response. BALB/c mice were intramuscular (IM) immunized with Aichi and PR8-inactivated bi-component vaccine [divalent inactivated vaccine (DIV)], the combination of the 4M2e-tFliC fusion protein, Aichi and PR8-inactivated tri-component vaccine, or microneedle patch (MNP) skin vaccination with the tri-component vaccine. Serum samples (N = 8) were collected 3 weeks post immunization and analyzed for levels of Aichi-specific IgG (A) and IgG isotypes (D), PR8-specific IgG (A) and IgG isotypes (C) and M2e-specific IgG and IgG isotypes (E). (B) Hemagglutinin inhibition titers against Aichi and PR8. Data represent mean ± SEM. The statistical significance was analyzed by Mann–Whitney U test. *p < 0.05, **p < 0.01, ***p < 0.001, n.sp > 0.05.
We also determined the levels of M2e-specific IgG and IgG isotype-specific antibody titers. Compared with the DIV IM immunized group, the tri-component vaccine delivered via MNPs or IM promoted significantly higher M2e-specific IgG and IgG1 titers. Only low amounts of IgG2a were detected in all the groups (Figure 1E). Meanwhile, we observed no differences in M2e-specific IgG or IgG1 levels between the MNPs and IM groups that were immunized with the tri-component vaccine. These results were consistent with our previous data which demonstrated that immunization with 4M2e-tFliC fusion protein alone elicited an IgG1 predominant immune response (23). The observation that 4M2e-tFliC alone MNPs induced higher M2e-specific IgG1, and immunization with the tri-component MNPs induced a much higher level of virus-specific IgG1, indicated that the MNP-based vaccine tended to induce a more balanced IgG1 and IgG2a responses.
We measured IL-4 and IFN-γ secreting cells by cytokine ELISPOT 4 weeks after immunizations. After stimulation with Aichi or PR8 virus, we observed significantly elevated numbers of IL-4- and IFN-γ-secreting spleen cells in the MNP-immunized group versus either the DIV or tri-component IM immunized groups. Mice receiving the MNP immunization also showed much more IL-4- and IFN-γ-secreting cells in the spleen after stimulation with M2e peptides versus the IM groups (Figures 2A,B). The IM vaccination groups had similar numbers of IL-4- and IFN-γ-secreting spleen cells. Meanwhile, we detected equal amounts of IL-2-secreting cells within splenocytes from all immunized groups when using inactivated Aichi or PR8 viruses or M2e peptides as stimulators (Figure S1A in Supplementary Material). The increased levels of IL-4-secreting cells in the MNP-immunized group provide evidence that skin vaccination with MNP-encapsulated tri-component vaccine enhances Th2 immune responses.
Figure 2. Cellular immune response after the immunization. Splenocytes and bone marrow cells were isolated from mice (N = 4) at week 4 post vaccination. (A) IL-4-secreting cells in spleens and (B) IFN-γ-secreting cells in spleens. Cytokine-secreting cells in splenocytes were measured in the presence of 4 µg/ml purified inactivated Aichi virus, PR8 virus, or M2e peptides as stimulators by the ELISPOT method. (C,D) Numbers of Aichi- and PR8-specific IgG plasma cells in bone marrow and spleens, respectively. Data represent mean ± SEM. The statistical significance was analyzed by Student’s t-test. *p < 0.05, **p < 0.01, ***p < 0.001, n.sp > 0.05.
IL-4 not only plays important roles in Th2 cell differentiation but also facilitates the proliferation and differentiation of B cells into antibody-secreting plasma cells (31–34). Therefore, we evaluated the spleen ASCs after the primary immunization, as well as the bone marrow plasma cells which derived from circulating B plasma cells and behaved independently of IL-4 (35). As shown in Figure 2C, Aichi and PR8 virus-specific IgG plasma cells in bone marrow were elevated 4 weeks after tri-component MNP or IM immunization. However, the number of virus-specific IgG plasma cells in the DIV group was as low as in the naïve group. Similarly, we observed more virus-specific IgA plasma cells in the tissues of mice receiving the tri-component vaccine (Figure S1B in Supplementary Material). Unlike the responses in bone marrow, we observed no significant differences between any of the groups in the numbers of Aichi or PR8-specific IgG ASCs in splenocytes (p > 0.05) (Figure 2D). These results show 4M2e-tFliC plays a role in supporting the stimulation of virus-specific bone marrow plasma cells.
Germinal center responses stimulate the development of long-lived plasma cells which are critical for the promotion of long-term immune memory. We analyzed the activation of B cells and follicular B helper T cells (Tfh) from ILNs, 7 days post-immunization. We detected a threefold increase in the frequency of B220 and GL-7 double-positive B cells in ILNs from the MNP group when compared with either of the IM groups (Figure 3A). We also detected an elevated frequency of double-positive CD4 and CXCR5 cells in the MNP-immunized mice compared with tri-component IM group (Figure 3B). We also determined the numbers of B220+GL-7+ B cells and CD4+CXCR5+ T cells in splenocytes. We observed a higher frequency of B220+GL-7+ splenocytes in the tri-component IM immunized mice (Figure S2 in Supplementary Material).
Figure 3. Microneedle patch immunization induced germinal center (GC) reactions in the draining lymph node. The number of GC B cells (A) and Tfh cells (B) from inguinal lymph nodes was determined 7 days post immunization by FACS (N = 4). Data represent mean ± SEM. The statistical significance was analyzed by Student’s t-test. *p < 0.05, **p < 0.01, ***p < 0.001, n.sp > 0.05.
To exclude the possibility that the enhanced GC reactions were caused by the MNP device, two groups of mice were immunized with either PBS or 4M2e-tFliC MNPs to test the GC responses in ILNs 7 days post-immunization. As shown in Figures S3A,B in Supplementary Material, compared with the PBS MNP-immunized group, the 4M2e-tFliC MNP induced an approximately 3.5- and 4-fold increase in the numbers of B220+GL-7+ B cells and CD4+CXCR5+ T cells, respectively. These results show that MNP-based immunization is a potent strategy to induce GC reactions.
The secretion levels of IL-2, IL-4, IL-6, IL-12/p40, and IL-17A from the ILN lymphocytes are indicators of innate and cellular immune responses. We analyzed these cytokines for a better understanding of the immune responses to MNP immunization. At day 7 after the immunizations, we re-stimulated lymphocytes isolated from ILNs with 4M2e-tFliC fusion proteins or inactivated PR8 for 5 days to determine the cytokines secreted into the supernatant in vitro.
We observed twofold higher levels of IL-4 after 4M2e-tFliC or inactivated PR8 stimulation in the MNP-immunized group versus the IM immunized groups or naïve group (Figure 4A). We also observed higher levels of IL-2 in the MNP-immunized group after stimulation (Figure 4B). The most striking differences in the cytokines released after stimulation we observed were the significant levels of IL-12/p40 and IL-17A levels in the MNP-immunized group. In sharp contrast, the levels of IL-12/p40 and IL-17A cytokines in the IM groups or naïve group were very low (Figures 4C,D).
Figure 4. Induction of cytokine secretion in vitro. Single-cell suspensions from inguinal lymph nodes (N = 3) were stimulated with 4M2e-tFliC fusion protein or PR8-inactivated virus in vitro for 5 days. The cytokines in supernatants were determined by cytokine ELISA. (A) IL2; (B) IL-4; (C) IL-12/P40; (D) IL-17; (E) IL-6. Data represent mean ± SEM. The statistical significance was analyzed by Student’s t-test. *p < 0.05, **p < 0.01, ***p < 0.001, n.sp > 0.05.
IL-17A is involved in the mediation of proinflammatory responses by inducing production of many other cytokines, such as IL-6. As shown in Figure 4E, we detected higher levels of IL-6 in the MNP group following 4M2e-tFliC fusion protein stimulation, but we observed no difference following stimulation by inactivated PR8. Meanwhile, we observed increased secretion of IL-12/p40 and IL-17A from cell suspensions of ILNs at day 7, but not day 4, after 4M2e-tFliC MNP immunization (Figure S4 in Supplementary Material).
Overall, these data provide evidence indicating that by creating a mildly inflammatory environment in draining lymph nodes, MNP immunization induces more comprehensive immune responses than the traditional IM injection, such as elevated GC B cell and Tfh cell responses.
We have demonstrated that the tri-component MNP vaccination augmented immune responses versus the other vaccines tested. To compare the protective efficacy against avian influenza virus infection, we immunized groups of mice and challenged them with 2 × LD50 of the reassortant A/Shanghai/2013 (H7N9, rSH) influenza virus. As shown in Figures 5A,B, after rSH infection, all naïve mice reached their endpoints within 9 days. The DIV IM immunization provided 60% protection, and mice were severely sick. Compared with the DIV IM group, the tri-component MNP and IM vaccines provided 100% protection against H7N9 virus challenge with mice showing 10 and 15% body weight loss, respectively (Figures 5A,B).
Figure 5. Protection efficacy against a heterologous influenza virus challenge. BALB/c mice (N = 5) were intramuscular (IM) immunized with divalent inactivated vaccine (DIV), tri-component vaccine, or microneedle patch (MNP) immunized with the tri-component vaccine. Four weeks after the immunization, mice were challenged with 2 × LD50 of A/Shanghai/02/2013 (H7N9, rSH) reassortant virus. Body weight changes (A) and survival rates (B) were monitored daily for 14 days. Mice were sacrificed at day 5 post challenge for the collection of spleens, bronchoalveolar lavage fluids (BALF), and lungs. (C) Cytokine-secreting cells in splenocytes were measured in the presence of 4 µg/ml purified A/Shanghai/02/2013 (H7N9, rSH) virus by ELISPOT. (D) The levels of M2e-specific IgG in BALF. (E) Lung viral titers. Data represent mean ± SEM. The statistical significance was analyzed by Student’s t-test. *p < 0.05, **p < 0.01, ***p < 0.001, n.sp > 0.05.
We sacrificed mice at day 5 post rSH infection and measured the cellular immune responses in the spleen. Compared with the DIV IM group, there were greater numbers of IL-2-, IL-4-, and IFN-γ-secreting cells in the tri-component immunized groups following stimulation with inactivated rSH. We detected the largest numbers of IL-2- and IL-4-secreting cells in the MNP-immunized group (Figure 5C). No significant differences were observed among the immunization groups in the numbers of IL-6-secreting cells (Figure 5C).
In agreement with the observed cellular immune responses in the spleen, the tri-component immunizations enhanced M2e-specific IgG antibody titers in BALF 5 days after rSH infection. We detected over two times higher levels of M2e-specific IgG antibody titers in the BALF of mice from the MNP-immunized group versus the tri-component IM group (Figure 5D). Due to the improved cellular immune responses in the spleen and the humoral immune responses in the BALF, we measured significantly lower levels of lung viral titers from the tri-component immunized mice. We detected the lowest levels of viral titers in the MNP-immunized group (Figure 5E).
These results show that immunization with 4M2e-tFliC fusion protein improved immune responses and increased inhibition of virus infection. MNPs fabricated with 4M2e-tFliC and inactivated influenza vaccines amplified the already enhanced immune responses and provided extra protection against the heterologous avian influenza viral infection.
We performed passive administration of 4M2e-tFliC MNP immune serum to determine the protective role of M2e-specific antibodies. We collected 4M2e-tFliC MNP immune sera from vaccinated mice and pooled them together at 3 weeks after the immunization. The detailed immunization method was described in Section “Materials and Methods” and a diagram of the experimental strategy was included in Supplementary Material (Figure S6 in Supplementary Material).
We primed and boosted three groups of mice with inactivated PR8 and Aichi at week 0 and week 3, respectively. Three weeks after the boosting immunization, we immunized one group of mice with 4M2e-tFliC MNPs. At 3 weeks after the 4M2e-tFliC MNPs immunization (week 9 after the primary vaccination) and 2 h before A/Vietnam/1203/2004 (H5N1, rVet) or rSH reassortant virus challenges, we IP injected the other two groups of inactivated influenza immunized mice with naïve serum or 4M2e-tFliC immune serum. We injected one group of naïve mice with 4M2e-tFliC serum as a control.
As shown in Figures 6A,B, 4M2e-tFliC immune serum passive transfer decreased lung viral titers and provided 100% protection to naïve mice that were infected with 2 × LD50 of H5N1 or H7N9 influenza viruses. We also observed that mice receiving the 4M2e-tFliC immune serum showed significantly decreased H7N9 viral titers in lungs compared with similar mice, naïve, or immunized, which received the naïve serum (Figure 6D).
Figure 6. 4M2e-tFliC microneedle patch (MNP) immune sera play a protective role in heterologous virus infection. BALB/c mice (N = 5) were intramuscular (IM) immunized twice with the divalent inactivated vaccine (DIV). One group of immunized mice was boosted with 4M2e-tFliC MNPs for the third immunization (DIV + MNPs). Three weeks after the MNP vaccination, the other two groups of DIV-immunized mice were intraperitoneally (IP) injected with naïve serum (DIV + Naïve serum) or 4M2e-tFliC immune serum (DIV + 4M2e-tFliC serum), respectively. Naïve mice received 4M2e-tFliC immune serum as a control (Naïve + 4M2e-tFliC serum). (A,B) Body weight change after 2 × LD50 of A/Vietnam/1203/2004 (H5N1, rVet) and A/Shanghai/02/2013 (H7N9, rSH) reassortant virus challenges. (C,D) Lung viral titers at day 5 post H5N1 and H7N9 challenges. Data represent mean ± SEM. The statistical significance was analyzed by Student’s t-test. *p < 0.05, **p < 0.01, ***p < 0.001, n.sp > 0.05.
We detected much lower levels of virus in 4M2e-tFliC MNP-immunized mice versus all other groups (Figures 6C,D). This demonstrates that besides the inhibition function of the M2e-specific antibodies, the cellular immune responses induced by the MNP and the heterologous prime-boost were critical for the protective efficacy of this vaccination strategy.
To further understand the immunological value of MNP vaccination, we immunized mice with PBS or 4M2e-tFliC MNP. We aimed to determine the early phase cellular immune responses after 4M2e-tFliC MNP immunization. Seven days post-immunization, we collected skin tissue and ILNs to analyze the frequency of different cell populations. As shown in Figure 7A, 53.1% MHCII+ cells could be detected at the skin immunization site of the mice 7 days post-immunization compared with the 18.1% MHC II+ cells observed in the PBS MNP-immunized group.
Figure 7. Microneedle patch (MNP) skin vaccination promoted antigen-presenting cell (APC) recruitment and dendritic cells (DCs) migration. (A) MHC II-positive cells were detected from mouse skin lymphocytes at day 7 post PBS treatment or 4M2e-tFliC MNP skin vaccination. The frequencies (B) and the numbers (C) of migratory DCs and subsets of migratory DCs (D–F) in inguinal lymph nodes were determined at day 7 post vaccination by FACS (N = 4). Data represent mean ± SEM. The statistical significance was analyzed by Student’s t-test. *p < 0.05, **p < 0.01, ***p < 0.001, n.sp > 0.05.
Dendritic cells are the primary antigen-presenting cells (APCs) that initiate T cell responses. We observed a significantly increased population of migratory MHC II++ CD11c++ DCs in the skin-draining lymph nodes (ILN) after 4M2e-tFliC MNP immunization (Figures 7B,C). Langerin is an important marker of epidermal and dermal DCs. We could detect increased numbers of Langerin+ migratory DCs and a greater percentage of Langerin+CD11b+ cells in the skin-draining lymph nodes of the 4M2e-tFliC MNP-immunized mice (Figures 7D–F). Meanwhile, compared with soluble 4M2e-tFliC IM or PBS MNP immunization, 4M2e-tFliC MNP vaccination elicited an increased percentage of GC B cells and CD4+ Tfh cells in ILNs (Figures S5 and S3 in Supplementary Material).
MHC II is highly upregulated in the process of the APCs maturation. Therefore, these results show that 4M2e-tFliC MNP immunization recruited MHC II+ APCs to the immunization site and stimulated the migration of Langerin+ DCs to skin-draining lymph nodes and induced strong GC reactions.
This study shows that skin vaccination with a tri-component influenza vaccine using an MNP can generate robust humoral and cellular immune responses and provide better protection than the traditional IM injection. Besides the immunological advantages, the MNP vaccination platform possesses many logistical advantages over traditional vaccines, such as ease of use (e.g., mass vaccination and self-administration), avoidance of biohazardous sharps waste, and independence from the cold chain (17–20). These characteristics make MNPs an excellent candidate for a potential universal influenza vaccine.
Our work and other previous reports have shown that MNPs containing a monovalent influenza vaccine provided 100% protection against the homologous virus and limited protection against heterologous virus infection (29, 36, 37). A recent clinical trial showed MNP vaccination generated robust immune responses, had an excellent safety profile, and had strong acceptability by study participants (38). Also, MNPs containing M2e-based vaccines have been shown to provide better cross-protection against heterologous virus infections (26, 39). In this study, we combined an M2e-based vaccine with two subtypes of inactivated influenza vaccines and fabricated them into a single MNP with the aim of developing a feasible universal influenza vaccine. Compared with the traditional IM injection, the tri-component vaccine delivered by MNP induced increased immunological responses and better protective efficacy against heterologous avian influenza virus infection. The results demonstrate the advantages of MNP-based skin vaccination. In this study, PR8 and Aichi inactivated viruses represent different subtypes of influenza A virus, and these two subtypes are the major subtypes that spread during the flu seasons. PR8 and Aichi can be replaced by any predicted seasonal influenza strains. We hope this newly designed vaccine could be used as a model for the design of future universal influenza vaccine.
The skin has been shown to be an attractive target for vaccine administration due to its high concentration of APCs. These APCs can be divided into several populations according to the expression of Langerin and their location in the skin structure. These divisions include epidermal Langerhans cell (LCs, Langerin+CD11b+), Langerin+ dermal DCs (Langerin+XCR1+CD11blow, Langerin+XCR1+CD103+/−), Langerin− dermal DCs (Langerin−CD11b+/low), dermal macrophages, and monocyte-derived DC (21, 40, 41). Migration to the LNs and interaction with naïve T cells are basic features of the epidermal LCs and dermal DCs. MNPs contain 100 or more tiny microneedles that penetrate the epidermis and reach the dermis with only minor disturbance of the vasculature. This shallow dermal penetration allows for MNP-based vaccines to fully exploit the potential of dermal APCs for better antigen processing and presentation. Our observations—of significantly increased numbers of cells highly expressing MHC II in the inoculated skin and highly double-positive MHC II/CD11c cells in the skin-draining LNs—support the application of MNPs.
We detected a population of Langerin+CD11b+ migratory DCs and a higher number of activated CD4+ follicular helper T cell and GC B cells in the skin-draining LNs at day 7 post 4M2e-tFliC MNP skin vaccination. These data suggest that the increased humoral and cellular immune responses might be due to the maturation of Langerin+ CD11b+ skin DCs (LCs), the migration of LCs to the ILN, and LCs interaction with and activation of the CD4+ T cells in the draining lymph nodes.
However, more detailed information regarding which subsets of migratory DCs was induced by the MNP vaccine is needed. Uncovering the regulatory mechanisms of MNP vaccination on skin DC maturation and migration is important for targeting specialized subsets of skin DCs to elicit different T cell responses. For example, targeting antigens to XCR1+ conventional dendritic cells with anti-Clec9A antibodies in mice enabled strong CD8 and CD4 T cell responses (42, 43). In another example, intradermal immunization with lentivectors activated skin dermal dendritic cells to induce CD8+ T cell responses (44). Also, the protective effector memory T (TEM) cells generated by skin vaccination can enter the skin to induce local T cell responses for protection against skin infections (45). These observations support that well-designed MNP-based vaccines induce robust and broad T cell responses for protection against a range of different infections.
Skin DCs are a bridge between the innate and adaptive immunity. Activated CD4+ T cells can be stimulated by different cytokines secreted by skin DCs to differentiate into diverse subtypes, including Th1, Th2, Th17, and Treg. APC-derived IL-12 plays key roles in the development of Th1 cell responses with the production of IL-2 and IFN-γ, while Th2 cell differentiation heavily depends on T-cell-derived IL-4 (46, 47). The balanced high levels of humoral IgG1 and IgG2a antibody titers in serum of the MNP-immunized group correlated with high ratios of IL-4- versus IL-2- and IFN-γ-secreting cells in the draining LNs and the spleen. Meanwhile, the increased production of IL-12/p40 and IL-4 in vitro after stimulation with the 4M2e-tFliC fusion protein or inactivated PR8 suggest the formation of both Th1 and Th2 subtypes.
Previous research has shown that IL-1β, IL-6, IL-23, and TGF-β might be critical components for supporting Th17 differentiation (48). Th17 cells are characterized by the production of cytokines (IL-17A, IL-6, and IL-22), the ability to recruit neutrophils and macrophages, and the ability to regulate inflammation reactions (47, 49). The increased in vitro secretion levels of IL-6, IL12/p40, and IL-17A in lymphocytes from skin-draining LNs show that our tri-component MNP vaccine upregulated Th17 differentiation. In addition, LCs have been shown to be essential for the induction of antigen-specific Th17 responses (50, 51). Therefore, Th17 cell responses may participate in immune responses after 4M2e-tFliC MNP skin vaccination. Further studies are required to deduce the regulatory mechanisms involved.
Several approaches could be explored to improve the interactions between antigens encapsulated in MNPs and skin DCs to maximize the desired immune responses. For example, nanoparticles composed of protein or DNA could be an efficient way to optimize DC targeting by MNP vaccines (52–54). Nanoparticle-based vaccines with components directly targeting markers on DC surfaces, like the TLR and NOD ligands, can further enhance the immunogenicity of the antigens within the nanoparticles (55, 56). TLRs are expressed on many cells in the skin, including TLR5, which is known to recognize bacterial FliC and activate innate immunity (57). Thus, the FliC in the 4M2e-tFliC fusion protein delivered via MNP may target this specific subset of dermal DCs and contribute to the increased immune responses we observed.
The frequent human infection of the avian H7N9 strain could escalate into a pandemic when it experiences future genetic drift or shift. This possibility highlights the urgency to develop a more broadly effective and rapidly distributable influenza vaccine. The vaccination strategy in this study takes advantage of MNP skin vaccination, M2e immunity, the immunogenicity of inactivated influenza vaccines and FliC’s adjuvant effect. We demonstrated that mice receiving the combination of the 4M2e-tFliC fusion protein and the two types of inactivated influenza via MNP skin vaccination are an effective approach to generate extra protection against heterologous avian influenza virus infection.
All animal experiments were performed in accordance with the protocol (Protocol number A16029) approved by Georgia State University’s Institutional Animal Care and Use Committee (IACUC). This study was in strict compliance with the Animal Welfare Act Regulations, the Public Health Service (PHS) Policy on Humane Care and Use of Laboratory Animals, and the Guide for the Care and Use of Laboratory Animals.
WZ, MP, and B-ZW designed research. WZ, SL, and CW performed research. WZ, SL, GY, and B-ZW analyzed the data. WZ, MP, and B-ZW wrote the paper.
MP is an inventor of patents licensed to companies developing microneedle-based products, is a paid advisor to companies developing microneedle-based products, and is a founder/shareholder of companies developing microneedle-based products (Micron Biomedical). This potential conflict of interest has been disclosed and is managed by Georgia Tech and Emory University. All other authors have no potential conflict of interest.
We thank the staff from the GSU Department of Animal Resource, for their administrative support in animal studies. This work is supported by NIAID NIH R01AI101047 and R01AI116835 to B-ZW. The content is solely the responsibility of the authors and does not necessarily represent the official views of the NIH.
The Supplementary Material for this article can be found online at https://www.frontiersin.org/articles/10.3389/fimmu.2018.01705/full#supplementary-material.
1. World Health Organization (WHO). Influenza (Seasonal). Fact Sheet. (2018). Available from: http://www.who.int/mediacentre/factsheets/fs211/en/ (Accessed: July 19, 2018).
2. Luckhaupt SE, Sweeney MH, Funk R, Calvert GM, Nowell M, D’Mello T, et al. Influenza-associated hospitalizations by industry, 2009-10 influenza season, United States. Emerg Infect Dis (2012) 18(4):556–62. doi:10.3201/eid1804.110337
3. Thompson WW, Shay DK, Weintraub E, Brammer L, Cox N, Anderson LJ, et al. Mortality associated with influenza and respiratory syncytial virus in the United States. JAMA (2003) 289(2):179–86. doi:10.1001/jama.289.2.179
4. Baldo V, Baldovin T, Pellegrini M, Angiolelli G, Majori S, Floreani A, et al. Immunogenicity of three different influenza vaccines against homologous and heterologous strains in nursing home elderly residents. Clin Dev Immunol (2010) 2010:517198. doi:10.1155/2010/517198
5. Nolan T, McVernon J, Skeljo M, Richmond P, Wadia U, Lambert S, et al. Immunogenicity of a monovalent 2009 influenza A(H1N1) vaccine in infants and children: a randomized trial. JAMA (2010) 303(1):37–46. doi:10.1001/jama.2009.1911
6. Ding H, Santibanez TA, Jamieson DJ, Weinbaum CM, Euler GL, Grohskopf LA, et al. Influenza vaccination coverage among pregnant women—National 2009 H1N1 Flu Survey (NHFS). Am J Obstet Gynecol (2011) 204(6):S96–106. doi:10.1016/j.ajog.2011.03.003
7. Centers for Disease Control and Prevention (CDC). 2009-2010 Influenza (Flu) Season. (2011). Available from: https://www.cdc.gov/flu/pastseasons/0910season.htm (Accessed: July 19, 2018).
8. Centers for Disease Control and Prevention (CDC). Interim Estimates of 2017–18 Seasonal Influenza Vaccine Effectiveness—United States, February 2018. (2018). Available from: https://www.cdc.gov/mmwr/volumes/67/wr/mm6706a2.htm (Accessed: July 19, 2018).
10. World Health Organization (WHO). Human infection with avian influenza A(H7N9) virus – China. Disease Outbreak News. (2017). Available from: http://www.who.int/csr/don/28-june-2017-ah7n9-china/en/ (Accessed: July 19, 2018).
11. Xiong X, Martin SR, Haire LF, Wharton SA, Daniels RS, Bennett MS, et al. Receptor binding by an H7N9 influenza virus from humans. Nature (2013) 499(7459):496–9. doi:10.1038/nature12372
12. Tharakaraman K, Jayaraman A, Raman R, Viswanathan K, Stebbins NW, Johnson D, et al. Glycan receptor binding of the influenza A virus H7N9 hemagglutinin. Cell (2013) 153(7):1486–93. doi:10.1016/j.cell.2013.05.034
13. Manz B, de Graaf M, Mogling R, Richard M, Bestebroer TM, Rimmelzwaan GF, et al. Multiple natural substitutions in avian influenza A virus PB2 facilitate efficient replication in human cells. J Virol (2016) 90(13):5928–38. doi:10.1128/JVI.00130-16
14. Chen GW, Kuo SM, Yang SL, Gong YN, Hsiao MR, Liu YC, et al. Genomic signatures for avian H7N9 viruses adapting to humans. PLoS One (2016) 11(2):e0148432. doi:10.1371/journal.pone.0148432
15. Marjuki H, Mishin VP, Chesnokov AP, De La Cruz JA, Davis CT, Villanueva JM, et al. Neuraminidase mutations conferring resistance to oseltamivir in influenza A(H7N9) viruses. J Virol (2015) 89(10):5419–26. doi:10.1128/JVI.03513-14
16. Itoh Y, Shichinohe S, Nakayama M, Igarashi M, Ishii A, Ishigaki H, et al. Emergence of H7N9 influenza A virus resistant to neuraminidase inhibitors in nonhuman primates. Antimicrob Agents Chemother (2015) 59(8):4962–73. doi:10.1128/AAC.00793-15
17. Arya J, Prausnitz MR. Microneedle patches for vaccination in developing countries. J Control Release (2016) 240:135–41. doi:10.1016/j.jconrel.2015.11.019
18. Marshall S, Sahm LJ, Moore AC. The success of microneedle-mediated vaccine delivery into skin. Hum Vaccin Immunother (2016) 12(11):2975–83. doi:10.1080/21645515.2016.1171440
19. Leone M, Monkare J, Bouwstra JA, Kersten G. Dissolving microneedle patches for dermal vaccination. Pharm Res (2017) 34(11):2223–40. doi:10.1007/s11095-017-2223-2
20. Shin CI, Jeong SD, Rejinold NS, Kim YC. Microneedles for vaccine delivery: challenges and future perspectives. Ther Deliv (2017) 8(6):447–60. doi:10.4155/tde-2017-0032
21. Clausen BE, Stoitzner P. Functional specialization of skin dendritic cell subsets in regulating T cell responses. Front Immunol (2015) 6:534. doi:10.3389/fimmu.2015.00534
22. Heath WR, Carbone FR. The skin-resident and migratory immune system in steady state and memory: innate lymphocytes, dendritic cells and T cells. Nat Immunol (2013) 14(10):978–85. doi:10.1038/ni.2680
23. Zhu W, Pewin W, Wang C, Luo Y, Gonzalez GX, Mohan T, et al. A boosting skin vaccination with dissolving microneedle patch encapsulating M2e vaccine broadens the protective efficacy of conventional influenza vaccines. J Control Release (2017) 261:1–9. doi:10.1016/j.jconrel.2017.06.017
24. Skountzou I, Martin Mdel P, Wang B, Ye L, Koutsonanos D, Weldon W, et al. Salmonella flagellins are potent adjuvants for intranasally administered whole inactivated influenza vaccine. Vaccine (2010) 28(24):4103–12. doi:10.1016/j.vaccine.2009.07.058
25. Deng L, Mohan T, Chang TZ, Gonzalez GX, Wang Y, Kwon YM, et al. Double-layered protein nanoparticles induce broad protection against divergent influenza A viruses. Nat Commun (2018) 9(1):359. doi:10.1038/s41467-017-02725-4
26. Wang BZ, Gill HS, He C, Ou C, Wang L, Wang YC, et al. Microneedle delivery of an M2e-TLR5 ligand fusion protein to skin confers broadly cross-protective influenza immunity. J Control Release (2014) 178:1–7. doi:10.1016/j.jconrel.2014.01.002
27. Wang BZ, Quan FS, Kang SM, Bozja J, Skountzou I, Compans RW. Incorporation of membrane-anchored flagellin into influenza virus-like particles enhances the breadth of immune responses. J Virol (2008) 82(23):11813–23. doi:10.1128/JVI.01076-08
28. WHO Global Influenza Surveillance Network. Manual for the Laboratory Diagnosis and Virological Surveillance of Influenza. (2011). 153 p. Available from: http://www.who.int/influenza/gisrs_laboratory/manual_diagnosis_surveillance_influenza/en/ (Accessed: July 19, 2018).
29. Koutsonanos DG, Martin DP, Zarnitsyn VG, Jacob J, Prausnitz MR, Compans RW, et al. Serological memory and long-term protection to novel H1N1 influenza virus after skin vaccination. J Infect Dis (2011) 204(4):582–91. doi:10.1093/infdis/jir094
30. Benck CJ, Martinov T, Fife BT, Chatterjea D. Isolation of infiltrating leukocytes from mouse skin using enzymatic digest and gradient separation. J Vis Exp (2016) 107:e53638. doi:10.3791/53638
31. Chen L, Grabowski KA, Xin JP, Coleman J, Huang Z, Espiritu B, et al. IL-4 induces differentiation and expansion of Th2 cytokine-producing eosinophils. J Immunol (2004) 172(4):2059–66. doi:10.4049/jimmunol.172.4.2059
32. Pelly VS, Kannan Y, Coomes SM, Entwistle LJ, Ruckerl D, Seddon B, et al. IL-4-producing ILC2s are required for the differentiation of TH2 cells following Heligmosomoides polygyrus infection. Mucosal Immunol (2016) 9(6):1407–17. doi:10.1038/mi.2016.4
33. Saito T, Kitayama D, Sakamoto A, Tsuruoka N, Arima M, Hatano M, et al. Effective collaboration between IL-4 and IL-21 on B cell activation. Immunobiology (2008) 213(7):545–55. doi:10.1016/j.imbio.2008.01.006
34. Gaya M, Barral P, Burbage M, Aggarwal S, Montaner B, Warren Navia A, et al. Initiation of antiviral B cell immunity relies on innate signals from spatially positioned NKT cells. Cell (2018) 172(3):517–33.e20. doi:10.1016/j.cell.2017.11.036
35. O’Connor BP, Cascalho M, Noelle RJ. Short-lived and long-lived bone marrow plasma cells are derived from a novel precursor population. J Exp Med (2002) 195(6):737–45. doi:10.1084/jem.20011626
36. Koutsonanos DG, Esser ES, McMaster SR, Kalluri P, Lee JW, Prausnitz MR, et al. Enhanced immune responses by skin vaccination with influenza subunit vaccine in young hosts. Vaccine (2015) 33(37):4675–82. doi:10.1016/j.vaccine.2015.01.086
37. Wang J, Li B, Wu MX. Effective and lesion-free cutaneous influenza vaccination. Proc Natl Acad Sci U S A (2015) 112(16):5005–10. doi:10.1073/pnas.1500408112
38. Rouphael NG, Paine M, Mosley R, Henry S, McAllister DV, Kalluri H, et al. The safety, immunogenicity, and acceptability of inactivated influenza vaccine delivered by microneedle patch (TIV-MNP 2015): a randomised, partly blinded, placebo-controlled, phase 1 trial. Lancet (2017) 390(10095):649–58. doi:10.1016/S0140-6736(17)30575-5
39. Kim MC, Lee JW, Choi HJ, Lee YN, Hwang HS, Lee J, et al. Microneedle patch delivery to the skin of virus-like particles containing heterologous M2e extracellular domains of influenza virus induces broad heterosubtypic cross-protection. J Control Release (2015) 210:208–16. doi:10.1016/j.jconrel.2015.05.278
40. Malissen B, Tamoutounour S, Henri S. The origins and functions of dendritic cells and macrophages in the skin. Nat Rev Immunol (2014) 14(6):417–28. doi:10.1038/nri3683
41. Levin C, Perrin H, Combadiere B. Tailored immunity by skin antigen-presenting cells. Hum Vaccin Immunother (2015) 11(1):27–36. doi:10.4161/hv.34299
42. Sancho D, Mourao-Sa D, Joffre OP, Schulz O, Rogers NC, Pennington DJ, et al. Tumor therapy in mice via antigen targeting to a novel, DC-restricted C-type lectin. J Clin Invest (2008) 118(6):2098–110. doi:10.1172/JCI34584
43. Caminschi I, Proietto AI, Ahmet F, Kitsoulis S, Teh JS, Lo JCY, et al. The dendritic cell subtype-restricted C-type lectin Clec9A is a target for vaccine enhancement. Blood (2008) 112(8):3264–73. doi:10.1182/blood-2008-05-155176
44. Furmanov K, Elnekave M, Lehmann D, Clausen BE, Kotton DN, Hovav AH. The role of skin-derived dendritic cells in CD8+ T cell priming following immunization with lentivectors. J Immunol (2010) 184(9):4889–97. doi:10.4049/jimmunol.0903062
45. Clark RA. Resident memory T cells in human health and disease. Sci Transl Med (2015) 7(269):269rv1. doi:10.1126/scitranslmed.3010641
46. Yoshimoto T, Takeda K, Tanaka T, Ohkusu K, Kashiwamura S, Okamura H, et al. IL-12 up-regulates IL-18 receptor expression on T cells, Th1 cells, and B cells: synergism with IL-18 for IFN-gamma production. J Immunol (1998) 161(7):3400–7.
47. Gutcher I, Becher B. APC-derived cytokines and T cell polarization in autoimmune inflammation. J Clin Invest (2007) 117(5):1119–27. doi:10.1172/JCI31720
48. Terhune J, Berk E, Czerniecki BJ. Dendritic cell-induced Th1 and Th17 cell differentiation for cancer therapy. Vaccines (Basel) (2013) 1(4):527–49. doi:10.3390/vaccines1040527
49. Laan M, Cui ZH, Hoshino H, Lotvall J, Sjostrand M, Gruenert DC, et al. Neutrophil recruitment by human IL-17 via C-X-C chemokine release in the airways. J Immunol (1999) 162(4):2347–52.
50. Igyarto BZ, Haley K, Ortner D, Bobr A, Gerami-Nejad M, Edelson BT, et al. Skin-resident murine dendritic cell subsets promote distinct and opposing antigen-specific T helper cell responses. Immunity (2011) 35(2):260–72. doi:10.1016/j.immuni.2011.06.005
51. Mathers AR, Janelsins BM, Rubin JP, Tkacheva OA, Shufesky WJ, Watkins SC, et al. Differential capability of human cutaneous dendritic cell subsets to initiate Th17 responses. J Immunol (2009) 182(2):921–33. doi:10.4049/jimmunol.182.2.921
52. Lee BR, Ko HK, Ryu JH, Ahn KY, Lee YH, Oh SJ, et al. Engineered human ferritin nanoparticles for direct delivery of tumor antigens to lymph node and cancer immunotherapy. Sci Rep (2016) 6:35182. doi:10.1038/srep35182
53. Joshi VB, Geary SM, Salem AK. Biodegradable particles as vaccine delivery systems: size matters. AAPS J (2013) 15(1):85–94. doi:10.1208/s12248-012-9418-6
54. Mahe B, Vogt A, Liard C, Duffy D, Abadie V, Bonduelle O, et al. Nanoparticle-based targeting of vaccine compounds to skin antigen-presenting cells by hair follicles and their transport in mice. J Invest Dermatol (2009) 129(5):1156–64. doi:10.1038/jid.2008.356
55. Hamdy S, Molavi O, Ma Z, Haddadi A, Alshamsan A, Gobti Z, et al. Co-delivery of cancer-associated antigen and toll-like receptor 4 ligand in PLGA nanoparticles induces potent CD8+ T cell-mediated anti-tumor immunity. Vaccine (2008) 26(39):5046–57. doi:10.1016/j.vaccine.2008.07.035
56. Pavot V, Rochereau N, Primard C, Genin C, Perouzel E, Lioux T, et al. Encapsulation of Nod1 and Nod2 receptor ligands into poly(lactic acid) nanoparticles potentiates their immune properties. J Control Release (2013) 167(1):60–7. doi:10.1016/j.jconrel.2013.01.015
Keywords: microneedle patch, skin vaccination, influenza vaccine, H7N9 influenza virus, immune responses
Citation: Zhu W, Li S, Wang C, Yu G, Prausnitz MR and Wang B-Z (2018) Enhanced Immune Responses Conferring Cross-Protection by Skin Vaccination With a Tri-Component Influenza Vaccine Using a Microneedle Patch. Front. Immunol. 9:1705. doi: 10.3389/fimmu.2018.01705
Received: 12 April 2018; Accepted: 10 July 2018;
Published: 30 July 2018
Edited by:
Florian Krammer, Icahn School of Medicine at Mount Sinai, United StatesReviewed by:
Carole Henry, University of Chicago, United StatesCopyright: © 2018 Zhu, Li, Wang, Yu, Prausnitz and Wang. This is an open-access article distributed under the terms of the Creative Commons Attribution License (CC BY). The use, distribution or reproduction in other forums is permitted, provided the original author(s) and the copyright owner(s) are credited and that the original publication in this journal is cited, in accordance with accepted academic practice. No use, distribution or reproduction is permitted which does not comply with these terms.
*Correspondence: Bao-Zhong Wang, YndhbmcyM0Bnc3UuZWR1
Disclaimer: All claims expressed in this article are solely those of the authors and do not necessarily represent those of their affiliated organizations, or those of the publisher, the editors and the reviewers. Any product that may be evaluated in this article or claim that may be made by its manufacturer is not guaranteed or endorsed by the publisher.
Research integrity at Frontiers
Learn more about the work of our research integrity team to safeguard the quality of each article we publish.