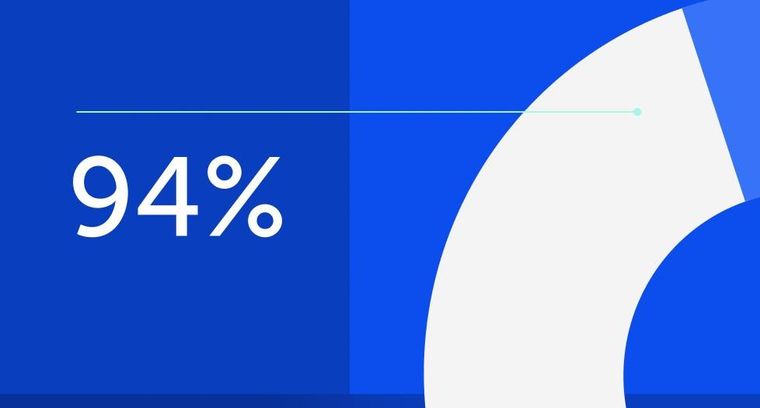
94% of researchers rate our articles as excellent or good
Learn more about the work of our research integrity team to safeguard the quality of each article we publish.
Find out more
ORIGINAL RESEARCH article
Front. Immunol., 30 July 2018
Sec. Cytokines and Soluble Mediators in Immunity
Volume 9 - 2018 | https://doi.org/10.3389/fimmu.2018.01604
Blood levels of the acute phase reactant C-reactive protein (CRP) are frequently measured as a clinical marker for inflammation, but the biological functions of CRP are still controversial. CRP is a phosphocholine (PC)-binding pentraxin, mainly produced in the liver in response to elevated levels of interleukin-1β (IL-1β) and of the IL-1β-dependent cytokine IL-6. While both cytokines play important roles in host defense, excessive systemic IL-1β levels can cause life-threatening diseases such as trauma-associated systemic inflammation. We hypothesized that CRP acts as a negative feedback regulator of monocytic IL-1β maturation and secretion. Here, we demonstrate that CRP, in association with PC, efficiently reduces ATP-induced inflammasome activation and IL-1β release from human peripheral blood mononuclear leukocytes and monocytic U937 cells. Effective concentrations are in the range of marginally pathologic CRP levels (IC50 = 4.9 µg/ml). CRP elicits metabotropic functions at nicotinic acetylcholine (ACh) receptors (nAChRs) containing subunits α7, α9, and α10 and suppresses the function of ATP-sensitive P2X7 receptors in monocytic cells. Of note, CRP does not induce ion currents at conventional nAChRs, suggesting that CRP is a potent nicotinic agonist controlling innate immunity without entailing the risk of adverse effects in the nervous system. In a prospective study on multiple trauma patients, IL-1β plasma concentrations negatively correlated with preceding CRP levels, whereas inflammasome-independent cytokines IL-6, IL-18, and TNF-α positively correlated. In conclusion, PC-laden CRP is an unconventional nicotinic agonist that potently inhibits ATP-induced inflammasome activation and might protect against trauma-associated sterile inflammation.
Interleukin-1β (IL-1β) is a pro-inflammatory cytokine of innate immunity that plays a seminal role in host defense (1). Secretion of monocytic IL-1β into the circulation in response to severe multiple trauma, however, may be more harmful than beneficial, as IL-1β is swept away from the site of inflammation, and high blood plasma levels can cause systemic inflammatory response syndrome (SIRS) and multi organ dysfunction syndrome (MODS) (2–5). Despite decades of intensive research, treatment of SIRS and MODS is mainly supportive, and mortality remains unacceptably high (5, 6).
IL-1β secretion normally requires two consecutive danger signals (5, 7–9). Ligands of toll-like receptors are typical first signals inducing pro-IL-1β synthesis, and extracellular ATP is a typical second signal that activates the ATP-sensitive P2X7 receptor (P2X7R), induces NLRP3 (NACHT, LRR, and PYD domains-containing protein 3) inflammasome assembly, caspase-1 activation, pro-IL-1β cleavage, and secretion of mature IL-1β (7–11). The secretion of IL-18 and high mobility group box 1 protein (HMGB1) also depends on inflammasome activation (7–13). Apart from the ATP-induced pathway typical for trauma-associated sterile inflammation, several alternative mechanisms of IL-1β maturation are activated during infection (1).
C-reactive protein (CRP) is a pentraxin (14, 15), mainly produced in the liver in response to elevated systemic levels of IL-1β and IL-6 (14, 15). Blood concentrations of this acute phase protein are a frequently used sensitive clinical marker for inflammation. In addition, slightly raised CRP levels correlate with cardiovascular disease and some disorders of the central nervous system (16–18). The biological functions of CRP are, however, highly disputed. CRP seems to play a vital role in humans, as its gene was conserved during evolution and there are no reports on CRP-deficient individuals (14). It has been proposed to be involved in the clearance of pathogens or apoptotic cells (14, 15), to induce pro-inflammatory cytokines (19, 20), and to play a pathogenic role in cardiovascular diseases (21). At least some of the pro-inflammatory effects are mediated by activated CRP that exposes binding sites for complement and immunoglobulins and is mainly found within inflamed tissues (22, 23). In contrast to the more pro-inflammatory functions, CRP induces high levels of the anti-inflammatory IL-1 receptor antagonist (24), and transgenic animals overexpressing human CRP are protected from inflammatory diseases including sepsis (25), alveolitis (26), arthritis (27), and atherosclerosis (28).
In the presence of Ca2+, CRP associates with phosphocholine (PC) and a range of more complex molecules containing a PC group at a 1:1 M ratio per CRP monomer (14, 15, 29). Our group recently demonstrated that phosphatidylcholines and their metabolites including free PC are efficient inhibitors of ATP-induced release of IL-1β from human monocytes by a mechanism that involves non-canonical metabotropic functions of nicotinic acetylcholine (ACh) receptors (nAChRs) (30–33).
Here, we demonstrate that purified human endogenous CRP (eCRP) efficiently inhibits the ATP-induced release of IL-1β from monocytic cells. We provide evidence that CRP presents PC to nAChRs and thus potentiates the effect of free PC. PC-laden CRP is hence a novel agonist of unconventional nAChRs that controls inflammasome activation by inhibiting the function of P2X7R.
U937 cells (DSMZ, Braunschweig, Germany) were maintained in RPMI 1640 (Gibco by Life Technologies, Darmstadt, Germany) supplemented with 10% fetal calf serum (FCS, Biochrome, Berlin, Germany) and 2 mM l-glutamine (Gibco by Life Technologies) under 5% CO2 atmosphere at 37°C. Cells (1 × 106 cells/ml) were seeded in 24-well plates, primed for 5 h with 1 µg/ml lipopolysaccharide (LPS) from Escherichia coli (L2654, Sigma-Aldrich, Deisenhofen, Germany) (30). BzATP [2′(3′)-O-(4-benzoylbenzoyl)adenosine 5′-triphosphate triethylammonium salt; 100 µM, Sigma-Aldrich] or nigericin (50 µM, Sigma-Aldrich) were added for another 30 min in the presence or absence of different concentrations of eCRP from human pleural fluid (Millipore, AG732), recombinant CRP (rCRP) produced in E. coli (Millipore, 236608), serum amyloid P (SAP; Millipore, 565190), or PC chloride calcium salt tetrahydrate (Sigma-Aldrich). Nicotinic antagonists mecamylamine hydrochloride (Sigma-Aldrich), strychnine hydrochloride (Sigma-Aldrich), α-bungarotoxin (Tocris Bioscience, Bristol, UK), ArIB [V11L, V16D] (500 nM) (34, 35) and RgIA4 (200 nM) (31, 36) were also applied together with BzATP. Supernatants were stored at 20°C until cytokine and lactate dehydrogenase (LDH) measurement.
Peripheral blood mononuclear cells were obtained from healthy (self-reported) male non-smoking adult volunteers. The local ethics committee at the University of Giessen approved all studies on primary human cells (approval No. 81/13). Blood was drawn into sterile syringes containing 17.5 IU heparin (Ratiopharm, Ulm, Germany) per ml blood and PBMC were separated on Leucosep gradients (Greiner Bio-One, Frickenhausen, Germany). LPS (5 ng/ml) was added to blood samples before gradient centrifugation (30). PBMC were cultured in 24-well plates at a density of 5 × 105 cells/0.5 ml in RPMI 1640, 10% FCS, 2 mM l-glutamine for 3 h. Non-adherent cells were removed, and cell culture medium was replaced by medium devoid of FCS. Stimulation with BzATP in the presence or absence of eCRP was done as described for U937 cells.
Non-Radioactive Cytotoxicity Assay (Promega, Madison, WI, USA) was used to measure LDH concentrations in cell free supernatants as indicated by the supplier. LDH values are given as percentage of the total LDH content of lysed control cells. Cell viability was unimpaired in all experimental settings.
Blood concentrations of IL-1β, IL-18, and tumor necrosis factor-α (TNF-α) were measured by the Human Quantikine® Immunoassays (R&D Systems, Minneapolis, MN, USA). IL-6 was measured on the Siemens 150.
Immulite 2000 XPI system using the Siemens IL-6 reagent (Siemens, Erlangen, Germany). HMGB1 was measured by an ELISA obtained from IBL International (Hamburg, Germany). To detect low cytokine levels in cell culture supernatants, for IL-1β the Human IL-1 beta/IL-1F2 DuoSet ELISA (R&D Systems) was used, whereas IL6 and TNF-α were measured by the Human Quantikine® Immunoassays (R&D Systems, Minneapolis, MN, USA).
Endogenous CRP was dissolved at a concentration of 5 µg/ml in PBS devoid of Ca2+ and Mg2+ (Gibco) containing 1.1 mM ethylenediaminetetraacetic acid (EDTA; Sigma-Aldrich), incubated at 37°C for 15 min followed by ultrafiltration using Amicon® Ultra centrifugal filters. The high molecular weight fraction was diluted in PBS/EDTA, ultrafiltrated, and transferred to PBS, 5 mM Ca2+, without EDTA by two additional ultrafiltration steps. In control, the same procedure was performed in the absence of EDTA. CRP purified by ultrafiltration and rCRP were incubated at a 1:1 and 1:3 M ratio per monomer, respectively, with PC at 37°C for 30 min and tested in IL-1β release assays at a concentration of 5 µg/ml CRP and 1 µM PC.
The expression of nAChR subunits α7 (CHRNA7), α9 (CHRNA9), and α10 (CHRNA10) in U937 cells was silenced by transfection of small interfering RNA (siRNA; 30 pmol per 1 × 106 cells, ON-TARGETplus human CHRNA7, CHRNA9, or CHRNA10 siRNA SMARTpool, Thermo Fisher Scientific, Schwerte, Germany) using the Amaxa® Cell line Nucleofector® Kit C (Lonza Cologne AG, Cologne, Germany) and the Nucleofector® device II (Lonza Cologne AG). Negative control ON-TARGETplus non-targeting pool (Thermo Fisher Scientific) was included to control for non-specific effects of transfection. A reduction of the mRNA expression of subunits α9 and α10 to about 50% of control-transfected cells was recently shown by our group in the same experimental setting via real-time RT-PCR 6 h after transfection (30). The basal expression of α7 mRNA, however, was too low to be quantified. IL-1β release experiments were performed 2 days after transfection.
Lipopolysaccharide-primed PBMC were cultured in CELLview™ slides (Greiner Bio-One) at a density of 2 × 105 cells per well in 200 µl medium and stimulated with BzATP (100 µM) in the presence or absence of eCRP (5 µg/ml). Cells were fixed and permeabilized with ice-cold Cytofix/Cytoperm™ (BD Biosciences, Heidelberg, Germany) for 20 min, washed with Perm/Wash™ buffer (BD Biosciences), and air-dried before storage at 4°C. Slides were rehydrated with Perm/Wash™ buffer, endogenous peroxidase activity was inhibited by treatment with 1% H2O2 in Perm/Wash™ buffer, followed by 1% bovine serum albumin in Perm/Wash™ buffer for 30 min at ambient temperature. Polyclonal rabbit antibodies to human ASC (1:50, SC-22514-R, Santa Cruz Biotechnology, Dallas, TX, USA) or monoclonal mouse antibodies to human CD14 (1:100, HCD14, BioLegend via Biozol, Eching, Germany) were diluted in Perm/Wash™ buffer containing 1% bovine serum albumin and 5% human heat-inactivated serum. Bound antibodies were detected with horseradish peroxidase-conjugated goat anti-rabbit Ig (1:50) and rabbit anti-mouse Ig (1:70) antibodies (both from Dako Cytomation, Glostrup, Denmark) and 0.5 mg/ml 3,3′-diaminobenzidine (Sigma-Aldrich), 1% H2O2, 0.3 M Tris-buffered saline, pH 7.6, for 10 min at room temperature. Slides were slightly counterstained with hemalumn and cover-slipped in Glycergel mounting medium (Dako Cytomation). Slides were evaluated blinded for the experimental groups at a 200-fold magnification using an Olympus BX51 microscope and the analySIS software (Olympus, Hamburg, Germany). At least 150 cells in 12 fields of vision were counted per experiment. Total numbers of cells and specks were converted into mean numbers of specks per 100 cells. In negative controls that resulted in no staining, primary antibodies were omitted. The specificity of the antibodies to ASC was verified by Western blotting of protein extracts from U937 cells that revealed a single band with the expected molecular mass (not shown).
SDS polyacrylamide gel electrophoresis was performed under reducing conditions according to Laemmli (37). PBMC were lysed, the protein concentration of the lysate was determined (Micro BCA protein assay kit, Pierce Biotechnology, Rockford, IL, USA) and adjusted to 15 μg/10 μl. Cell culture supernatants were harvested at the end of the experiments, concentrated by a factor of 10 using Amicon® Ultra centrifugal filters (Ultracel™ 10K, Merck Millipore, Darmstadt, Germany) and mixed with 2× sample buffer. Samples (10 µl each) were loaded onto 15% SDS-polyacrylamide gels, transferred to polyvinylidene difluoride membranes (Millipore, Billerica, MA, USA) and stained with Brilliant Blue G (Sigma-Aldrich). Prestained molecular weight standards (Precision Plus Protein Standards, dual color, Bio Rad, Hercules, CA, USA) were separated in each gel. Mouse monoclonal antibodies to IL-1β that detect both pro-IL-1β and mature IL-1β (1:10,000, 3ZD, kindly supplied by the National Cancer Institute, Frederick, MD, USA), polyclonal rabbit anti-caspase-1 antibodies (1:1,000, #2225, Cell Signaling Technology, Danvers, MA, USA) and mouse monoclonal antibodies to β-actin (1:500,000, A2228, Sigma-Aldrich) were applied and detected with horseradish peroxidase-conjugated rabbit anti-mouse Ig (1:5,000) and goat anti-rabbit Ig (1:5,000) antibodies (both from Dako Cytomation). SuperSignal West Dura Extended Duration Substrate (Thermo Scientific, Rockford, IL, USA) was used to detect IL-1β and Lumi-Light substrate (Roche, Mannheim, Germany) to detect β-actin. Documentation and densitometry of the blots were performed using a digital gel documentation system (Biozym, Hessisch Oldendorf, Germany).
U937 cells were incubated in poly-l-lysine-coated culture dishes (Nunc, Roskilde, Denmark) in bath solution [5.4 mM KCl, 120 mM NaCl, 2 mM CaCl2, 1 mM MgCl2, 10 mM HEPES (4-(2-hydroxyethyl)-piperazine-1-ethanesulfonic acid), 25 mM glucose, pH 7.4] for 5 h with LPS (1 µg/ml) at 37°C. Thereafter, whole-cell recordings were performed at ambient temperature on an inverted microscope (Axiovert, Zeiss, Göttingen, Germany). Patch pipettes were pulled from borosilicate glass capillaries (outer diameter 1.6 mm, Hilgenberg, Malsfeld, Germany) to a resistance of 2–4 MΩ using an automated puller (Zeitz, Augsburg, Germany). Pipettes were filled with pipette solution (120 mM KCl, 1 mM CaCl2, 2 mM MgCl2, 10 mM HEPES, 11 mM ethylene glycol tetraacetic acid, 20 mM glucose, pH 7.3). The membrane potential of LPS-primed U937 cells was voltage-clamped to −60 mV and transmembrane currents in response to BzATP (100 µM) were amplified with an EPC 9 amplifier (HEKA, Lambrecht, Germany) and acquired via an ITC-16 interface with the Pulse software (HEKA). A pressure-driven microperfusion system was used to apply BzATP, eCRP (5 µg/ml) and RgIA4 (200 nM).
To measure intracellular [Ca2+]i, U937 cells were incubated in poly-l-lysine-coated glass bottom culture dishes (CELLview™, Greiner Bio-One) for 5 h with LPS (1 µg/ml) at 37°C in the same bath solution as described for the whole-cell patch-clamp recordings. Thereafter, cells were loaded with 3.3 µM Fura-2/AM (Thermo Fisher Scientific) for 25 min at 37°C. Fura-2/AM was excited at 340 and 380 nm wavelengths and the fluorescence emission 510 nm was measured. Four independent batches of U937 cells were used in this experiment and a total number of 243 cells were tracked individually, and the fluorescence intensity ratio of 340:380 nm was recorded. Experiments were run at room temperature. After a calibration time of 100 s, cells were exposed to eCRP (5 µg/ml) for 300 s. At the end of the experiments, a positive control for the Ca2+ imaging setup was included. Forskolin (40 µM; Biozol), an activator of adenylyl cyclase that elevates cyclic adenosine monophosphate (cAMP) levels, was applied to induce a cAMP-triggered rise in [Ca2+]i (38).
Defolliculated Xenopus laevis oocytes were obtained from Ecocyte Bioscience (Castrop-Rauxel, Germany). Oocytes from at least two different Xenopus laevis individuals were used in all experimental groups. Oocytes were stored in Ringer’s solution (ORi) containing (in mM) 90 NaCl, 1 KCl, 2 CaCl2, 5 HEPES, 2.5 pyruvate, 20 mg/ml penicillin, and 25 mg/ml streptomycin (pH 7.4) at 16°C. All chemicals used for ORi preparation were purchased from Fluka (Deisenhofen, Germany), except for HEPES, penicillin, and streptomycin (Sigma-Aldrich). Plasmid DNAs encoding the human CHRNA9 and CHRNA10 as well as the 43 kDa receptor-associated protein of the synapse (RAPSN) were obtained from Eurofins Genomics (Ebersberg, Germany) and capped cRNA was synthesized as described before (31). Human CHRNA7 encoding cRNA was kindly provided by G. Schmalzing (Department of Molecular Pharmacology, RWTH Aachen University, Aachen, Germany) and synthesized as described before (33). cRNA was dissolved in nuclease-free water and injected into oocytes in a volume of 50.6 nl using a microinjector (Nanoject, Drummond Scientific, Broomall, PA, USA). Oocytes were injected with cRNA encoding CHRNA7, CHRNA9, and CHRNA10 nAChR subunits (16, 19, and 19 ng/oocyte, respectively) along with 5 ng cRNA encoding RAPSN, and oocytes were incubated at 16°C for 3–5 days. In control experiments, 50.6 nl of nuclease-free water was injected.
In TEVC measurements, oocytes were perfused (gravity driven) with ORi without pyruvate and antibiotics (pH 7.4). Intracellular borosilicate microelectrodes were filled with 1 M KCl solution and the membrane voltage was clamped to –60 mV using a TEVC amplifier (Warner Instruments, Hamden, CT, USA). Low-pass filtered transmembrane currents (1,000 Hz, Frequency Devices 902, Haverhill, MA, USA) were recorded using a strip chart recorder (Kipp & Zonen, Delft, The Netherlands). For experiments examining the inhibition and recovery from inhibition of choline-gated currents in presence and absence of eCRP (5 µg/ml), oocytes were injected with a 1:1 ratio of cRNA encoding human CHRNA9 and CHRNA10 in a 1:1 ratio, incubated at 17°C for 2–3 days and measured as described before (31).
A single center prospective observational study (trial registration: DRKS00010991) was approved by the ethics committee of the medical faculty Giessen, Germany (No. 164/14) and performed in accordance with the Helsinki Declaration. Written informed consent was given by each patient or patient’s legal representative. Patients were recruited at the surgical intensive care unit (ICU) of the University Hospital of Giessen, Germany from January 2015 until February 2016. Only patients with severe trauma as defined by an injury severity score (ISS) above 16 (39) were included. Patients younger than 18 years or with a history of HIV or hepatitis B/C infection were excluded. Detailed patient characteristics are listed in Table 1.
The first venous blood sample (day 0) was drawn within 15 h after admission to the hospital followed by daily blood collection in the morning. The levels of IL-1β, IL-18, TNF-α, IL-6, and HMGB1 were determined in the plasma of heparinized blood. CRP levels were analyzed turbidometrically by Siemens ADVIA XPT system (Siemens) using the wrCRP reagent (Siemens).
SPPS® (Version 23, IBM®, Armonk, NC, USA) and GraphPad Prism® (Version 6, GaphPad Software, La Jolla, CA, USA) were used for statistical and linear regression analyses. The IC50 value of eCRP in human U937 cells was determined in GraphPad Prism® (Version 6, GaphPad Software) by fitting log-transformed concentration values and the original effect data. Multiple groups were first analyzed by non-parametric Kruskal–Wallis test. In case of p ≤ 0.05, non-parametric Mann–Whitney rank sum test was performed to compare between individual groups and again, a p ≤ 0.05 was considered as statistically significant. Paired data were analyzed by Wilcoxon sign rank test.
To test if CRP inhibits ATP-induced IL-1β release, we primed human monocytic U937 cells for 5 h with LPS (1 µg/ml). Thereafter, 100 µM BzATP, a P2X7R agonist (40), was applied for 30 min in presence and absence of eCRP and IL-1β concentrations were measured in cell culture supernatants. We found that in LPS-primed U937 cells the BzATP-induced release of IL-1β was dose-dependently and efficiently inhibited by eCRP (Figure 1A). The half maximal inhibitory concentration (IC50) was 4.9 µg/ml corresponding to an about 40 nM concentration of pentameric CRP. Of note, plasma levels between 3 and 10 µg/ml are clinically regarded as a minor pathological CRP elevation (41). In contrast to eCRP, the acute phase protein (5 µg/ml), another pentraxin with high structural similarity to CRP (15), did not inhibit ATP-induced IL-1β release (Figure 1B).
Figure 1. Purified human endogenous C-reactive protein (eCRP) inhibits BzATP-induced release of interleukin-1β (IL-1β) from U937 cells. Lipopolysaccharide (LPS)-primed (1 µg/ml, 5 h) U937 cells were stimulated with BzATP (2′(3′)-O-(4-benzoylbenzoyl)adenosine 5′-triphosphate triethylammonium salt; 100 µM) and IL-1β was measured 30 min later in cell culture supernatants. (A) eCRP dose-dependently inhibited the BzATP-induced IL-1β release, nicotine (Nic; 100 µM) served as a positive control. (B,C) Serum amyloid P (5 µg/ml), human recombinant CRP (rCRP) (5 µg/ml), or low concentrations of free phosphocholine (PC) (1 µM) did not impair IL-1β release, but a combination of rCRP and PC (1 µM) did. (D) The inhibitory effect of eCRP (CRP I; 5 µg/ml) was preserved after ultrafiltration (cutoff 10 kDa; CRP II), but abolished by ultrafiltration in the presence of ethylenediaminetetraacetic acid (1.1 mM; CRP III). PC (1 µM) reconstituted the activity of CRP III, whereas 1 µM PC alone was ineffective. (E) CRP was retained in CRP III and absent from the low molecular weight fraction (LMW). SDS-PAGE followed by staining with Brilliant Blue. (F) The effect of eCRP was reversed by nicotinic acetylcholine receptor (nAChR) antagonists mecamylamine (Mec; 100 µM), α-bungarotoxin (α-Bun; 1 µM), strychnine (Stry; 10 µM), ArIB (500 nM), and RgIA4 (200 nM). (G) In experiments using small interfering RNA (siRNA), silencing of the nAChR subunits α7, α9, and α10, but not control siRNA (con) attenuated the inhibition by eCRP. Data are presented as individual data points, bar represents median, whiskers encompass the 25th to 75th percentile. *p ≤ 0.05, different from LPS-primed cells stimulated with BzATP alone. #p ≤ 0.05, different LPS-primed cells were stimulated with BzATP and eCRP. Kruskal–Wallis followed by Mann–Whitney rank sum test.
C-reactive protein, but not SAP, Ca2+-dependently associates with PC in a 1:1 M ratio per subunit (15, 29). We previously showed that PC efficiently inhibits BzATP-induced IL-1β secretion from human monocytes (30, 31). Thus, we hypothesized that the inhibitory effect of eCRP on the release of IL-1β depends on its association with molecules containing a PC group. Accordingly, human rCRP (5 µg/ml) produced in E. coli that is expected to be devoid of PC, did not inhibit the BzATP-triggered release of IL-1β from LPS-primed U937 cells (Figure 1C), whereas a mixture of 5 µg/ml rCRP, 1 µM PC, and 5 mM Ca2+ was fully effective (Figure 1C). The same concentration of free PC was ineffective (Figure 1C), as expected (30). In a similar approach, eCRP was treated with EDTA (1.1 mM) to detach ligands from the PC-binding sites and was separated from small molecules by ultrafiltration (Figures 1D,E). This resulted in an inactive CRP preparation, the activity of which was restored by adding PC (1 µM) in the presence of Ca2+ (Figure 1D). Hence, the inhibition of ATP-induced IL-1β release by eCRP depends on its association with PC. Moreover, as the IC50 eCRP is around 40 nM and that of free PC around 10 µM (30, 31), CRP potentiates the inhibitory effect of PC by at least two orders of magnitude.
Next, we tested if eCRP signals via nAChRs in human monocytic U937 cells. Indeed, the eCRP-dependent inhibition of ATP-induced IL-1β release was completely reversed by the non-selective nAChR antagonist mecamylamine (100 µM). Similar results were obtained by using α-bungarotoxin (1 µM) or strychnine (10 µM), both preferential antagonists of the α7 and α9 nAChRs (42–44). The α-conotoxins ArIB [V11L, V16D] (500 nM) (34, 35) and RgIA4 (200 nM) (31, 36) that are specific for nAChRs composed of subunits α7 and α9α10, respectively, also completely blocked the inhibitory effect of eCRP (Figure 1F). These results indicate that eCRP signals via nAChRs containing subunits α7, α9, and α10. To corroborate the involvement of these nAChR subunits, U937 cells were transfected with siRNA targeting CHRNA7, CHRNA9, and CHRNA10. Indeed, single-gene silencing of each nAChR subunit significantly blunted the inhibitory effect of eCRP (Figure 1G).
The canonical ionotropic function of nAChR can be monitored by using Xenopus laevis oocytes as heterologous expression systems (45). To investigate if eCRP elicits ionfluxes human nAChR subunits α7, α9, and α10 were heterologously expressed in oocytes to perform TEVC measurements. We showed that eCRP (5 µg/ml) did not induce ion currents, whereas the classical nAChR agonist choline did (Figures 2A–C). Nevertheless, eCRP interacted with canonical nAChRs, as it reduced choline-triggered currents in oocytes expressing α9α10 nAChRs (Figures 2D,E). Thus, eCRP does not trigger canonical ion channel functions of nAChRs but acts as a silent agonist or partial antagonist that modulates the responses to classical nicotinic agonists.
Figure 2. Purified human endogenous C-reactive protein (eCRP) does not induce ion channel functions at canonical nAChRs. Two-electrode voltage-clamp (TEVC) measurements were performed on Xenopus laevis oocytes that heterologously expressed human nAChR subunits α7, α9, and α10. (A,C) The application of the known nAChR agonist choline (Cho1, 2 min, 1 mM, black bars) induced a stimulation of the transmembrane ion current (IM) that could be repeated by a second Cho application (Cho2; n = 7). (B,C) By contrast, eCRP (5 µg/ml) did not provoke current responses, whereas a subsequent Cho application did (n = 6). (C) All changes of IM (ΔIM) induced by cholinergic stimulation are shown as individual data points, bars represent median, whiskers encompass the 25th to 75th percentile. Wilcoxon signed-rank test. (D) Representative current traces of Cho-gated currents in oocytes expressing α9α10 nAChRs illustrating the inhibitory effect of eCRP (5 µg/ml). The current traces represent 30 s recordings each and are shown concatenated omitting the 30 s gap between each individual trace. The oocytes were continuously perfused with saline solution and stimulated with Cho (1 s pulses, 1 mM) once per min until steady-state baseline responses were observed (indicated by the arrow). Subsequently, the Cho-gated currents were monitored in the presence of eCRP for changes in amplitude for 12 min. Thereafter, eCRP was washed out and the Cho-gated currents were monitored for recovery. (E) Graphical representation and analysis of the experimental results shown in (D). Mean and SEM from five oocytes.
Next, we tested if eCRP induces ion fluxes in LPS-primed U937 cells. Application of eCRP (5 µg/ml) did not induce ion currents in whole-cell patch-clamp experiments (Figures 3A,B) and intracellular Ca2+ levels remained unchanged (Figure 3C). By contrast, BzATP (100 µM) induced a robust and repeatable current response in LPS-primed U937 cells (Figures 3A,B), as reported previously (30, 31). Remarkably, in the presence of eCRP, the BzATP-induced current responses were completely abrogated (Figures 3A,B). The inhibitory effect of eCRP was sensitive to the α-conotoxin RgIA4 (Figures 3A,B), confirming the involvement of nAChR subunits α9 and/or α10.
Figure 3. Purified human endogenous C-reactive protein (eCRP) suppresses BzATP-induced whole-cell currents via nAChRs. (A) BzATP-induced ion currents were detected by whole-cell patch-clamp measurements in lipopolysaccharide (LPS)-primed (1 µg/ml, 5 h) U937 cells. Repetitive current changes were provoked by two (1, 2) consecutive BzATP (100 µM) applications (upper panel). Application of eCRP (5 µg/ml) alone did not provoke ion currents but fully inhibited the response to BzATP (middle panel). The inhibitory effect of eCRP was antagonized by addition of the α9α10 nAChR-specific α-conotoxin RgIA4 (200 nM; lower panel). (B) Graphical presentation of the two consecutive BzATP-induced ion current changes (1, 2, ΔIBzATP). (C) [Ca2+]i of LPS-primed U937 cells were recorded as Fura-2/AM (Fura-2) fluorescence intensity ratio of 340:380 nm excitation (mean ± SEM). Application of eCRP (5 µg/ml; indicated by arrow) did not cause significant alterations in [Ca2+]i (values before eCRP compared to values obtained 300 s after eCRP application: p = 0.726). At the end of the experiments, a positive control for cell viability and the Ca2+ imaging setup was included: forskolin (Fsk, 40 µM, indicated by arrow) was applied to induce a cyclic adenosine monophosphate-triggered rise in [Ca2+]i. (D) The ATP-independent release of interleukin-1β (IL-1β) from LPS-primed monocytic U937 cells induced by nigericin (Nig; 50 µM) is neither inhibited by nicotine (Nic; 100 µM) nor by various concentrations of eCRP. Data are presented as individual data points, bar represents median, whiskers encompass the 25th to 75th percentile (B,D). Wilcoxon signed-rank test (B,C) or Kruskal–Wallis followed by Mann–Whitney rank sum test (D).
NLRP3 inflammasomes, in addition to extracellular ATP, assemble in response to other pro-inflammatory stimuli including pore-forming toxins (1). Here, the pore-forming bacterial toxin nigericin was used to investigate if eCRP also affects ATP-independent IL-1β release, which was clearly not the case (Figure 3D). Hence, stimulation of nAChRs with eCRP efficiently inhibits BzATP-induced ion currents in monocytic U937 cells but does not provoke canonical ion channel functions of nAChRs.
We performed experiments on the adherent fraction of freshly isolated PBMC from healthy human donors that were primed with a short pulse of LPS (5 ng/ml) during cell isolation. The spontaneous secretion of IL-1β by these cells was low, whereas a considerable amount of IL-1β was released in response to BzATP (100 µM). Indeed, eCRP (5 µg/ml) significantly attenuated the BzATP-induced release of IL-1β from these cells (Figure 4A), whereas the inflammasome-independent cytokines IL-6 and TNF-α (46) were neither induced by BzATP nor regulated by eCRP (Figures 4B,C). The concentrations of IL-6 and TNF-α released within 30 min after BzATP application are low and all apparent changes in response to BzATP or eCRP are not significant and probably random. We reported before, that almost no IL-18 is secreted by these cells in response to BzATP (30).
Figure 4. Purified human endogenous C-reactive protein (eCRP) inhibits BzATP-induced release of interleukin-1β (IL-1β) from human peripheral blood mononuclear cells (PBMC). PBMC freshly isolated from healthy volunteers were pulsed with lipopolysaccharide (5 ng/ml) during the process of PBMC isolation and cultured for 3 h. The cells were stimulated with 100 µM BzATP in the presence or absence of eCRP (5 µg/ml) for 30 min. (A) IL-1β, (B) IL-6, and (C) TNF-α were measured by ELISA in cell culture supernatants (n = 5). Data points obtained from individual blood donors are coded by different colors and connected by lines. Wilcoxon signed-rank test.
Inflammasome activation can lead to the formation of large aggregates, so-called specks or pyroptosomes, that can be detected with antibodies directed to ASC (apoptosis-associated speck-like protein containing a caspase activation and recruitment domain) (1). To investigate if CRP inhibits inflammasome and caspase-1 activation in primary monocytic cells LPS-pulsed PBMC were challenged with BzATP (100 µM) in the presence and absence of eCRP (5 µg/ml). A significant increase in ASC speck formation was detected in LPS-primed PBMC in response to BzATP, which was largely suppressed by concomitant application of eCRP (Figure 5A). Pro-IL-1β and pro-caspase-1, but not their mature forms, were detected by Western blotting of cell lysates of LPS-primed PBMC stimulated with BzATP in the absence or presence of eCRP (Figure 5B). In concentrated supernatants, however, mature caspase-1 and IL-1β were detectable upon BzATP-treatment, and eCRP significantly reduced their amount (Figures 5C,D). We conclude that CRP suppresses inflammasome assembly, pyroptosome formation, activation of capase-1 and maturation of IL-1β.
Figure 5. Purified human endogenous C-reactive protein (eCRP) inhibits BzATP-induced inflammasome activation, caspase-1 activation, and maturation of interleukin-1β (IL-1β) in human peripheral blood mononuclear cells (PBMC). (A) ASC specks (arrows) were detected by immunocytochemistry using antibodies directed to ASC (brown staining). Cell nuclei were lightly counterstained with hemalum. Specks were induced by treatment with BzATP and occurred less frequently when eCRP was added concomitantly (n = 7). (B–D) Western blot experiments were performed using antibodies that detect both, the pro-forms and the mature forms of caspase-1 and IL-1β, respectively (n = 5). Cell lysates and cell culture supernatants were investigated from lipopolysaccharide (LPS)-primed PBMC (1), LPS-primed PBMC stimulated with BzATP (2), and LPS-primed PBMC stimulated with BzATP in the presence of eCRP (3). (B) Pro-caspase-1 and pro-IL-1β were detected in cell lysates at about equal amounts irrespective of stimulation with BzATP, whereas no mature forms were present, although the antibodies used detect both the pro-forms and the native forms. Detection of β-actin was included as a loading control. (C) Only mature caspase-1 and (D) IL-1β were present in concentrated cell culture supernatants. Stimulation with BzATP induced the release of both proteins and the release was reduced by eCRP. Immunoreactivity was measured by densitometry [optical density (OD)]. Data points obtained from individual blood donors are coded by different colors and connected by lines. Wilcoxon signed-rank test.
Our in vitro experiments led to the provocative hypothesis that elevated CRP levels protect against trauma-induced release of IL-1β into the circulation in vivo. We performed a prospective study on multiple trauma patients admitted to our hospital. Patient characteristics are summarized in Table 1. Plasma levels of IL-1β, IL-18, IL-6, TNF-α, and HMGB1 were measured in blood drawn at daily intervals until day 4 after admission. During the first 2 days, IL-1β levels negatively correlated with CRP values of the preceding day (Figures 6A–D), which is in line with our hypothesis. On day 4 after trauma, plasma levels of IL-6 (Figure S1 in Supplementary Material), IL-18 (Figure S2 in Supplementary Material), and TNF-α (Figure S3 in Supplementary Material) positively correlated with CRP values on day 3, whereas no correlation was seen for HMGB1 (Figure S4 in Supplementary Material). On days 0–2 after trauma, IL-1β levels did not correlate with disease severity acute physiology and chronic health evaluation score (APACHE II) and sequential organ failure assessment score (SOFA). In patients who remained on the ICU on days 3–4 after trauma a negative correlation of IL-1β levels with the SOFA score was seen (Table S1 in Supplementary Material). This subpopulation of patients typically suffers from a severe disease course. CRP levels did not correlate with APACHE II and SOFA score (Table S2 in Supplementary Material). Albeit statistically significant (p ≤ 0.05), the correlation coefficients (r) and, accordingly, the coefficients of variation (CV) (Figure 6; Figures S1–S4 and Tables S1 and S2 in Supplementary Material), do not prove causality.
Figure 6. High levels of C-reactive protein (CRP) negatively correlate with low interleukin-1β (IL-1β) levels in trauma patients. A prospective clinical study was performed on patients suffering from multiple traumata. Plasma levels of IL-1β were measured at daily intervals from the day of admission (day 0) until day 4 after trauma and a correlation analysis was performed with CRP values obtained one day earlier. (A) IL-1β at day 1 versus CRP at day 0 (n = 36), (B) IL-1β at day 2 versus CRP at day 1 (n = 31), (C) IL-1β at day 3 versus CRP at day 2 (n = 23), and (D) IL-1β at day 4 versus CRP at day 3 (n = 21). Linear regression analysis, r = correlation coefficient, CV = coefficient of variation.
C-reactive protein is among the most frequently used clinical marker of inflammation, but its biological function is still a matter of debate. Here, we demonstrate that eCRP dose-dependently and efficiently inhibits the ATP-induced release of IL-1β from monocytic U937 cells at an IC50 of 4.9 µg/ml, corresponding to a marginally elevated blood CRP level (41). The activity of eCRP depends on the Ca2+-dependent interaction with a small molecule, presumably PC (14, 15), and on nAChR subunits α7, α9, and α10. Stimulation of monocytic nAChRs in turn fully inhibits ATP-induced ion currents (Figure 7). Furthermore, eCRP is also active in primary human adherent PBMC, where it inhibits inflammasome and caspase-1 activation. First clinical evidence from multiple trauma patients is in line with the results obtained in vitro but does not prove causality. As IL-1β and IL-6 are the main inducers of hepatic CRP synthesis during systemic inflammation and IL-1β is an important stimulus for IL-6 expression, we suggest that CRP is a negative feedback regulator of the ATP-dependent production of mature IL-1β by human monocytes (Figure 7).
Figure 7. Suggested mechanism of the mutual control of C-reactive protein (CRP) and interleukin-1β (IL-1β) during trauma-associated sterile inflammation. Injury causes the release of cytoplasmic danger-associated molecular patterns (DAMP) and ATP, and frequently microbiota, a source of pathogen-associated molecular patterns (PAMP), get access to the damaged tissue. DAMP and PAMP can bind to pattern recognition receptors such as toll-like receptors (TLR) at monocytic cells and induce the synthesis of pro-IL-1β, the inactive cytoplasmic precursor of IL-1β. Extracellular ATP activates the P2X7R and leads to inflammasome assembly and caspase-1 activation. Activated caspase-1 cleaves pro-IL-1β and enables the release of mature bioactive IL-1β that in turn, induces IL-6. IL-1β and IL-6 activate the hepatic synthesis of CRP and blood levels of CRP quickly rise to 1,000-fold. We demonstrated that purified human endogenous CRP inhibits the response of P2X7R to ATP via nAChR subunits α7, α9, and α10. The function of CRP critically depends on its association with small molecules such as phosphocholine (red dots) that seem to mediate the interaction with nicotinic receptors. We suggest that the CRP-mediated control of ATP-induced IL-1β release is a negative feedback loop that controls excessive systemic inflammation in response to injury. Although the nAChR is illustrated as a pentamer, its molecular structure remains to be elucidated.
CRP is commonly regarded as an opsonizing agent that binds to PC present on the surfaces of some pro- and eukaryotic pathogens as well as on dying cells (14, 15). Phagocytosis of these opsonized particles is mediated via complement fixation and binding of CRP to different Fc-receptors expressed by phagocytic cells (14, 15). The suggested role of CRP in host defense against infections is, however, in sharp contrast to a large body of literature showing that PC-containing cell surface molecules of bacteria as well as PC-modified proteins secreted by helminths exert anti-inflammatory functions leading to immune evasion and chronic colonization of the host (47, 48). We demonstrated before that PC-modified bovine serum albumin and PC-modified lipooligosaccharides from Haemophilus influenzae control the release of IL-1β via mechanisms resembling those of CRP (30). This suggests that pathogens with PC-modified surfaces hijacked the here described mechanism, which underscores the biological and medical relevance of CRP.
We identify nAChRs composed of subunits α7, α9, and α10 as receptors for eCRP. Our data corroborate the almost 30 years old finding, that CRP binds Ca2+-dependently to human monocytes with an EC50 of about 2.3 mg/l (49). Presumably, these authors measured the PC-dependent binding of CRP to monocytic nAChRs. We showed that PC-free CRP is inactive, and its activity can be reconstituted by adding low concentrations of PC that are in the range of those present in the serum of healthy persons (50). Thus, the interaction of eCRP with the ligand-binding site of nAChRs is probably mediated by CRP-bound PC. It has been shown before that endogenous human CRP can be laden with PC and other molecules with a PC group (14, 15, 29). In the same line, we demonstrated recently that high concentrations of free PC also inhibit the ATP-mediated release of IL-1β via nAChR (30–33), suggesting that CRP potentiates the effect of free PC. This is of importance in vivo, since typical human blood plasma concentrations of free PC are about 2 µM (50), while the IC50 of free PC is in the range of 10 µM, and 100 µM are needed for a full inhibition of the ATP-induced release of monocytic IL-1β (30).
Ligand-binding sites of conventional pentameric nAChRs that function as ligand-gated ion channels are formed by two neighboring nAChR subunits each and close upon binding of their cognate agonists (51). Interestingly, binding of a single ligand to only one of the five sites is sufficient for maximal ionotropic response of α7 nAChRs and additional binding sites enhance agonist sensitivity (52). The nAChR binding sites are dimensioned for classical nicotinic agonists but are certainly too small to enclose a macromolecule such as eCRP, suggesting that the structures of monocytic nAChRs are different. The activation of nAChR by a large molecule like eCRP is surprising but not unprecedented, as we demonstrated before that PC covalently bound to bovine serum albumin, PC-modified lipooligosaccharides and dipalmitoyl phosphatidylcholine function as potent agonists at unconventional monocytic nAChRs (30, 32). The IC50 values of PC-modified bovine serum albumin and lipooligosaccharides are also in the nanomolar range and hence, considerably lower than free PC, choline, or acetylcholine (30). If the structure of monocytic nAChRs differs from classical pentamers, it might be speculated that one eCRP pentamer interacts with several monocytic nAChRs. Once CRP binds to a nAChR, other receptors might be quickly recruited and activated. This hypothesis might explain the observed low IC50 values and the steep dose-response curve. This hypothesis may also apply to PC-modified albumin and to PC-lipooligosaccharides, because the PC-albumin investigated contained nine PC groups per BSA (30) and lipooligosaccharides tend to form micelles due to their amphiphilic structure. In contrast to the above-mentioned molecules with covalent PC modifications, it is also possible that eCRP delivers free PC to nAChRs because the affinities of PC to both molecules are in the same range (53). However, the considerably lower IC50 value of CRP compared to free PC (30) speaks against his theory. It may, however, explain, why eCRP acts as a silent agonist at canonical nAChRs heterologously expressed by Xenopus oocytes. Nevertheless, the structure of such unconventional nAChRs that exert metabotropic functions remains to be elucidated and it is even unclear if the leukocytic nAChR subunits form pentameric receptors at all.
Increasing evidence suggests that leukocytes in general respond to nicotinic stimuli via nAChRs with metabotropic responses, and no ligand-gated ion channel functions have been reported so far (44, 45, 54). A prominent example is the cholinergic regulation of the transcription and translation of pro-inflammatory cytokines by macrophages that is mediated via nAChR subunit α7 (54, 55). Here, we demonstrate that the P2X7R function of monocytic cells and the ATP-dependent release of IL-1β are fully inhibited by eCRP via stimulation of nAChRs containing subunits α7, α9, and α10. Nicotine, choline, and free PC exert similar effects albeit at much higher molar concentrations (30, 31, 33). The molecular signaling mechanism down-stream of monocytic nAChRs is currently under investigation.
Of note, eCRP neither induces ionotropic functions at monocytic nAChRs containing subunits α7, α9, and α10, nor at conventional nAChRs that were heterologously expressed by Xenopus oocytes. Similar results were shown before for free PC (31). Seemingly, eCRP is a novel agonist of nAChRs that exclusively induces metabotropic receptor functions and does not activate canonical ionotropic nAChR functions of excitable cells. Furthermore, we show that eCRP down-modulates the choline-induced ionotropic activity of heterologously expressed α9α10 nAChRs and provide evidence that CRP might be a silent agonist of nAChRs. As CRP and the nAChR subunits α7, α9, and α10 were highly conserved during evolution (14, 15, 51, 56), and mononuclear phagocytes are already present in primitive multicellular organisms (57), we speculate that the control of the P2X7R by nAChRs might be, in an evolutionary sense, older than neurotransmission.
We showed for the first time that in patients suffering from multiple traumata, IL-1β blood levels negatively correlated with preceding CRP levels. These results are in line with the hypothesis that the CRP-mediated control of IL-1β release is active in vivo, albeit they do not prove causality. According to Hill’s criteria for causation (58), “a small association does not mean that there is not a causal effect, though the larger the association, the more likely that it is causal.” Hill lists further criteria for causation including temporality, biological gradients, plausibility, and experimental evidence. Although, our data by and large meet with these criteria, more experimental and larger clinical multi-center studies are warranted, before we can dare to claim causality. The absence of a negative correlation of CRP levels with IL-18 and HMGB1 may be due to the ubiquitous expression of these inflammasome-dependent mediators, in contrast to IL-1β which is mainly produced by monocytes/macrophages (59).
Our data suggest that elevated CRP levels attenuate inflammatory diseases that are caused by ATP-induced inflammasome activation. It remains to be investigated if the here described mechanism also contributes to the protection against experimental inflammation in animals overexpressing human CRP (25–28). Interestingly, CRP was recently shown to impair dendritic cell development, maturation and function (60). This anti-inflammatory mechanism involves the inhibitory Fcγ receptor IIB (60). The potential clinical implications of these and our findings deserve further investigation, including a careful consideration of the known pro-inflammatory functions of CRP (14, 15, 22).
Several therapeutics targeting IL-1 or its receptor, among them the IL-1 receptor antagonist anakinra, the decoy receptor rilonacept and the neutralizing monoclonal anti-IL-1β antibody canakinumab, were tested in large trials but never reached the clinical arena for the treatment of SIRS (4). A major disadvantage of these approaches might be the general inhibition of IL-1β that should result in an impaired host defense against infections. By contrast, we showed that eCRP specifically inhibits the P2X7R-mediated response to extracellular ATP that is a danger signal mainly associated with mechanical cell damage (61). Viral, bacterial, and fungal pathogens activate numerous additional ATP-independent pathways of inflammasome activation and IL-1β maturation (1). Hence, we speculate that CRP predominantly inhibits trauma-associated release of IL-1β without preventing the IL-1β response to infection.
In conclusion, CRP efficiently inhibits ATP-dependent inflammasome activation and IL-1β release from human monocytic blood cells in vitro. This effect of CRP seems to depend on bound PC and activation of non-canonical nAChRs that efficiently inhibit the ion channel functions of monocytic ATP receptors. In the same line, we provide the first clinical evidence that elevated CRP levels might reduce systemic IL-1β release in patients suffering from multiple traumata.
The local ethics committee at the University of Giessen approved all studies on primary human cells (approval No. 81/13). The study protocol for clinical sample collection (trial registration: DRKS00010991) was approved by the ethics committee of the medical faculty Giessen, Germany (No. 164/14) and performed in accordance with the Helsinki Declaration. All patients completed written informed consent prior to study entry.
KR, SS, ATZ, MK, JD, SH, SW, and AJH performed experiments and interpreted results; IA, MP, SR, AH, and CK recruited healthy donors and patients and interpreted results; IRK performed statistical analyses and interpreted results; WK, MS, K-DS, WP, JMM, and CK were involved in study design, interpretation of the results, and in writing; in addition, JMM provided seminal reagents; VG designed the study, interpreted data, and wrote the paper.
Certain conotoxins, including RgIA4 have been patented by the University of Utah; JMM is an inventor on these patents. The other authors declare that the research was conducted in the absence of any commercial or financial relationships that could be construed as a potential conflict of interest.
The authors wish to thank healthy donors and patients for donation of blood as well as Gabriele Fuchs-Moll, Sabine Stumpf, Anja Schnecko, and Daniela Schreiber (University Giessen, Germany) for excellent technical support. We are grateful to Christian Freyer, Nils Schmerer, Tara Procida, and Julia Schäffer for experimental support, to Mike Althaus and Ivan Manzini (University Giessen) for providing essential experimental equipment, to Borros M. Arneth (University Giessen) for clinical routine measurements, to Günther Schmalzing and Ralf Hausmann (University Aachen, Germany) for providing cRNA encoding for human CHRNA7, and to Günther Lochnit (University Giessen) for helpful suggestions.
VG was funded by the German Research Foundation (GR 1094/7-1). JMM received funding from the National Institutes of Health (GM48677 and GM103801).
The Supplementary Material for this article can be found online at https://www.frontiersin.org/articles/10.3389/fimmu.2018.01604/full#supplementary-material.
1. Broz P, Dixit VM. Inflammasomes: mechanism of assembly, regulation and signalling. Nat Rev Immunol (2016) 16(7):407–20. doi:10.1038/nri.2016.58
2. Bone RC, Balk RA, Cerra FB, Dellinger RP, Fein AM, Knaus WA, et al. Definitions for sepsis and organ failure and guidelines for the use of innovative therapies in sepsis. The ACCP/SCCM Consensus Conference Committee. American College of Chest Physicians/Society of Critical Care Medicine. Chest (1992) 101(6):1644–55. doi:10.1378/chest.101.6.1644
3. Xiao W, Mindrinos MN, Seok J, Cuschieri J, Cuenca AG, Gao H, et al. A genomic storm in critically injured humans. J Exp Med (2011) 208(13):2581–90. doi:10.1084/jem.20111354
4. Dinarello CA, Simon A, van der Meer JW. Treating inflammation by blocking interleukin-1 in a broad spectrum of diseases. Nat Rev Drug Discov (2012) 11(8):633–52. doi:10.1038/nrd3800
5. Manson J, Thiemermann C, Brohi K. Trauma alarmins as activators of damage-induced inflammation. Br J Surg (2012) 99(Suppl 1):12–20. doi:10.1002/bjs.7717
6. Westphal M, Kampmeier T. The race against the "septic shark". Crit Care (2015) 19(Suppl 3):S11. doi:10.1186/cc14729
7. Gross O, Thomas CJ, Guarda G, Tschopp J. The inflammasome: an integrated view. Immunol Rev (2011) 243(1):136–51. doi:10.1111/j.1600-065X.2011.01046.x
8. Rathinam VA, Vanaja SK, Fitzgerald KA. Regulation of inflammasome signaling. Nat Immunol (2012) 13(4):333–42. doi:10.1038/ni.2237
9. Rathinam VA, Fitzgerald KA. Inflammasome complexes: emerging mechanisms and effector functions. Cell (2016) 165(4):792–800. doi:10.1016/j.cell.2016.03.046
10. Grahames CB, Michel AD, Chessell IP, Humphrey PP. Pharmacological characterization of ATP- and LPS-induced IL-1beta release in human monocytes. Br J Pharmacol (1999) 127(8):1915–21. doi:10.1038/sj.bjp.0702732
11. Cekic C, Linden J. Purinergic regulation of the immune system. Nat Rev Immunol (2016) 16(3):177–92. doi:10.1038/nri.2016.4
12. Novick D, Kim S, Kaplanski G, Dinarello CA. Interleukin-18, more than a Th1 cytokine. Semin Immunol (2013) 25(6):439–48. doi:10.1016/j.smim.2013.10.014
13. Yang H, Antoine DJ, Andersson U, Tracey KJ. The many faces of HMGB1: molecular structure-functional activity in inflammation, apoptosis, and chemotaxis. J Leukoc Biol (2013) 93(6):865–73. doi:10.1189/jlb.1212662
14. Pepys MB, Hirschfield GM. C-reactive protein: a critical update. J Clin Invest (2003) 111(12):1805–12. doi:10.1172/JCI200318921
15. Mantovani A, Garlanda C, Doni A, Bottazzi B. Pentraxins in innate immunity: from C-reactive protein to the long pentraxin PTX3. J Clin Immunol (2008) 28(1):1–13. doi:10.1007/s10875-007-9126-7
16. Badimon L, Peña E, Arderiu G, Padró T, Slevin M, Vilahur G, et al. C-reactive protein in atherothrombosis and angiogenesis. Front Immunol (2018) 9:430. doi:10.3389/fimmu.2018.00430
17. Del Giudice M, Gangestad SW. Rethinking IL-6 and CRP: why they are more than inflammatory biomarkers, and why it matters. Brain Behav Immun (2018) 70:61–75. doi:10.1016/j.bbi.2018.02.013
18. O’Toole MS, Bovbjerg DH, Renna ME, Lekander M, Mennin DS, Zachariae R. Effects of psychological interventions on systemic levels of inflammatory biomarkers in humans: a systematic review and meta-analysis. Brain Behav Immun (2018). doi:10.1016/j.bbi.2018.04.005
19. Ballou SP, Lozanski G. Induction of inflammatory cytokine release from cultured human monocytes by C-reactive protein. Cytokine (1992) 4(5):361–8. doi:10.1016/1043-4666(92)90079-7
20. Galve-de Rochemonteix B, Wiktorowicz K, Kushner I, Dayer JM. C-reactive protein increases production of IL-1 alpha, IL-1 beta, and TNF-alpha, and expression of mRNA by human alveolar macrophages. J Leukoc Biol (1993) 53(4):439–45. doi:10.1002/jlb.53.4.439
21. Ridker PM. From C-reactive protein to interleukin-6 to interleukin-1: moving upstream to identify novel targets for atheroprotection. Circ Res (2016) 118(1):145–56. doi:10.1161/CIRCRESAHA.115.306656
22. Braig D, Nero TL, Koch H-G, Kaiser B, Wang X, Thiele JR, et al. Transitional changes in the CRP structure lead to the exposure of proinflammatory binding sites. Nat Commun (2017) 8:14188. doi:10.1038/ncomms14188
23. Thiele JR, Zeller J, Kiefer J, Braig D, Kreuzaler S, Lenz Y, et al. A conformational change in C-reactive protein enhances leukocyte recruitment and reactive oxygen species generation in ischemia/reperfusion injury. Front Immunol (2018) 9:95. doi:10.3389/fimmu.2018.00675
24. Tilg H, Vannier E, Vachino G, Dinarello CA, Mier JW. Antiinflammatory properties of hepatic acute phase proteins: preferential induction of interleukin 1 (IL-1) receptor antagonist over IL-1 beta synthesis by human peripheral blood mononuclear cells. J Exp Med (1993) 178(5):1629–36. doi:10.1084/jem.178.5.1629
25. Mold C, Rodriguez W, Rodic-Polic B, Du Clos TW. C-reactive protein mediates protection from lipopolysaccharide through interactions with Fc gamma R. J Immunol (2002) 169(12):7019–25. doi:10.4049/jimmunol.169.12.7019
26. Heuertz RM, Xia D, Samols D, Webster RO. Inhibition of C5a des Arg-induced neutrophil alveolitis in transgenic mice expressing C-reactive protein. Am J Physiol (1994) 266(6 Pt 1):L649–54.
27. Jiang S, Xia D, Samols D. Expression of rabbit C-reactive protein in transgenic mice inhibits development of antigen-induced arthritis. Scand J Rheumatol (2006) 35(5):351–5. doi:10.1080/03009740600757963
28. Torzewski J, Fan J, Schunkert H, Szalai A, Torzewski M. C-reactive protein and arteriosclerosis. Mediators Inflamm (2014) 2014:646817. doi:10.1155/2014/683598
29. Thompson D, Pepys MB, Wood SP. The physiological structure of human C-reactive protein and its complex with phosphocholine. Structure (1999) 7(2):169–77. doi:10.1016/S0969-2126(99)80023-9
30. Hecker A, Küllmar M, Wilker S, Richter K, Zakrzewicz A, Atanasova S, et al. Phosphocholine-modified macromolecules and canonical nicotinic agonists inhibit ATP-induced IL-1β release. J Immunol (2015) 195(5):2325–34. doi:10.4049/jimmunol.1400974
31. Richter K, Mathes V, Fronius M, Althaus M, Hecker A, Krasteva-Christ G, et al. Phosphocholine – an agonist of metabotropic but not of ionotropic functions of α9-containing nicotinic acetylcholine receptors. Sci Rep (2016) 6:28660. doi:10.1038/srep28660
32. Backhaus S, Zakrzewicz A, Richter K, Damm J, Wilker S, Fuchs-Moll G, et al. Surfactant inhibits ATP-induced release of interleukin-1β via nicotinic acetylcholine receptors. J Lipid Res (2017) 58(6):1055–66. doi:10.1194/jlr.M071506
33. Zakrzewicz A, Richter K, Agné A, Wilker S, Siebers K, Fink B, et al. Canonical and novel non-canonical cholinergic agonists inhibit ATP-induced release of monocytic interleukin-1β via different combinations of nicotinic acetylcholine receptor subunits α7, α9 and α10. Front Cell Neurosci (2017) 11:491. doi:10.3389/fncel.2017.00189
34. Whiteaker P, Christensen S, Yoshikami D, Dowell C, Watkins M, Gulyas J, et al. Discovery, synthesis, and structure activity of a highly selective alpha7 nicotinic acetylcholine receptor antagonist. Biochemistry (2007) 46(22):6628–38. doi:10.1021/bi7004202
35. Innocent N, Livingstone PD, Hone A, Kimura A, Young T, Whiteaker P, et al. Alpha-conotoxin Arenatus IBV11L,V16D corrected is a potent and selective antagonist at rat and human native alpha7 nicotinic acetylcholine receptors. J Pharmacol Exp Ther (2008) 327(2):529–37. doi:10.1124/jpet.108.142943
36. Romero HK, Christensen SB, Di Cesare Mannelli L, Gajewiak J, Ramachandra R, Elmslie KS, et al. Inhibition of α9α10 nicotinic acetylcholine receptors prevents chemotherapy-induced neuropathic pain. Proc Natl Acad Sci U S A (2017) 114(10):E1825–32. doi:10.1073/pnas.1621433114
37. Laemmli UK. Cleavage of structural proteins during the assembly of the head of bacteriophage T4. Nature (1970) 227(5259):680–5. doi:10.1038/227680a0
38. Taylor CW. Regulation of IP3 receptors by cyclic AMP. Cell Calcium (2017) 63:48–52. doi:10.1016/j.ceca.2016.10.005
39. Baker SP, O’Neill B, Haddon W, Long WB. The injury severity score: a method for describing patients with multiple injuries and evaluating emergency care. J Trauma (1974) 14(3):187–96. doi:10.1097/00005373-197403000-00001
40. North RA. Molecular physiology of P2X receptors. Physiol Rev (2002) 82(4):1013–67. doi:10.1152/physrev.00015.2002
41. Kushner I, Antonelli MJ. What should we regard as an "elevated" C-reactive protein level? Ann Intern Med (2015) 163(4):326. doi:10.7326/L15-5126
42. Papke RL, Bencherif M, Lippiello P. An evaluation of neuronal nicotinic acetylcholine receptor activation by quaternary nitrogen compounds indicates that choline is selective for the alpha 7 subtype. Neurosci Lett (1996) 213(3):201–4. doi:10.1016/0304-3940(96)12889-5
43. Baker ER, Zwart R, Sher E, Millar NS. Pharmacological properties of alpha 9 alpha 10 nicotinic acetylcholine receptors revealed by heterologous expression of subunit chimeras. Mol Pharmacol (2004) 65(2):453–60. doi:10.1124/mol.65.2.453
44. Peng H, Ferris RL, Matthews T, Hiel H, Lopez-Albaitero A, Lustig LR. Characterization of the human nicotinic acetylcholine receptor subunit alpha (alpha) 9 (CHRNA9) and alpha (alpha) 10 (CHRNA10) in lymphocytes. Life Sci (2004) 76(3):263–80. doi:10.1016/j.lfs.2004.05.031
45. Papke RL. Merging old and new perspectives on nicotinic acetylcholine receptors. Biochem Pharmacol (2014) 89(1):1–11. doi:10.1016/j.bcp.2014.01.029
46. Erttmann SF, Härtlova A, Sloniecka M, Raffi FA, Hosseinzadeh A, Edgren T, et al. Loss of the DNA damage repair kinase ATM impairs inflammasome-dependent anti-bacterial innate immunity. Immunity (2016) 45(1):106–18. doi:10.1016/j.immuni.2016.06.018
47. Grabitzki J, Lochnit G. Immunomodulation by phosphocholine – biosynthesis, structures and immunological implications of parasitic PC-epitopes. Mol Immunol (2009) 47(2–3):149–63. doi:10.1016/j.molimm.2009.09.035
48. Clark SE, Weiser JN. Microbial modulation of host immunity with the small molecule phosphorylcholine. Infect Immun (2013) 81(2):392–401. doi:10.1128/IAI.01168-12
49. Ballou SP, Buniel J, Macintyre SS. Specific binding of human C-reactive protein to human monocytes in vitro. J Immunol (1989) 142(8):2708–13.
50. Ilcol YO, Ozbek R, Hamurtekin E, Ulus IH. Choline status in newborns, infants, children, breast-feeding women, breast-fed infants and human breast milk. J Nutr Biochem (2005) 16(8):489–99. doi:10.1016/j.jnutbio.2005.01.011
51. Boffi JC, Marcovich I, Gill-Thind JK, Corradi J, Collins T, Lipovsek MM, et al. Differential contribution of subunit interfaces to α9α10 nicotinic acetylcholine receptor function. Mol Pharmacol (2017) 91(3):250–62. doi:10.1124/mol.116.107482
52. Andersen N, Corradi J, Sine SM, Bouzat C. Stoichiometry for activation of neuronal α7 nicotinic receptors. Proc Natl Acad Sci U S A (2013) 110(51):20819–24. doi:10.1073/pnas.1315775110
53. Christopeit T, Gossas T, Danielson UH. Characterization of Ca2+ and phosphocholine interactions with C-reactive protein using a surface plasmon resonance biosensor. Anal Biochem (2009) 391(1):39–44. doi:10.1016/j.ab.2009.04.037
54. Olofsson PS, Rosas-Ballina M, Levine YA, Tracey KJ. Rethinking inflammation: neural circuits in the regulation of immunity. Immunol Rev (2012) 248(1):188–204. doi:10.1111/j.1600-065X.2012.01138.x
55. Kawashima K, Fujii T, Moriwaki Y, Misawa H, Horiguchi K. Reconciling neuronally and nonneuronally derived acetylcholine in the regulation of immune function. Ann N Y Acad Sci (2012) 1261:7–17. doi:10.1111/j.1749-6632.2012.06516.x
56. Lipovsek M, Im GJ, Franchini LF, Pisciottano F, Katz E, Fuchs PA, et al. Phylogenetic differences in calcium permeability of the auditory hair cell cholinergic nicotinic receptor. Proc Natl Acad Sci U S A (2012) 109(11):4308–13. doi:10.1073/pnas.1115488109
57. Buchmann K. Evolution of innate immunity: clues from invertebrates via fish to mammals. Front Immunol (2014) 5:459. doi:10.3389/fimmu.2014.00459
58. Hill AB. The environment and disease: association or causation? Proc R Soc Med (1965) 58:295–300.
59. Vladimer GI, Marty-Roix R, Ghosh S, Weng D, Lien E. Inflammasomes and host defenses against bacterial infections. Curr Opin Microbiol (2013) 16(1):23–31. doi:10.1016/j.mib.2012.11.008
60. Jimenez RV, Wright TT, Jones NR, Wu J, Gibson AW, Szalai AJ. C-reactive protein impairs dendritic cell development, maturation, and function: implications for peripheral tolerance. Front Immunol (2018) 9:372. doi:10.3389/fimmu.2018.00372
Keywords: C-reactive protein, interleukin-1β, NLRP3 inflammasome, monocytes, nicotinic acetylcholine receptors, sterile inflammation
Citation: Richter K, Sagawe S, Hecker A, Küllmar M, Askevold I, Damm J, Heldmann S, Pöhlmann M, Ruhrmann S, Sander M, Schlüter K-D, Wilker S, König IR, Kummer W, Padberg W, Hone AJ, McIntosh JM, Zakrzewicz AT, Koch C and Grau V (2018) C-Reactive Protein Stimulates Nicotinic Acetylcholine Receptors to Control ATP-Mediated Monocytic Inflammasome Activation. Front. Immunol. 9:1604. doi: 10.3389/fimmu.2018.01604
Received: 09 March 2018; Accepted: 27 June 2018;
Published: 30 July 2018
Edited by:
Ji Ming Wang, National Cancer Institute at Frederick, United StatesCopyright: © 2018 Richter, Sagawe, Hecker, Küllmar, Askevold, Damm, Heldmann, Pöhlmann, Ruhrmann, Sander, Schlüter, Wilker, König, Kummer, Padberg, Hone, McIntosh, Zakrzewicz, Koch and Grau. This is an open-access article distributed under the terms of the Creative Commons Attribution License (CC BY). The use, distribution or reproduction in other forums is permitted, provided the original author(s) and the copyright owner(s) are credited and that the original publication in this journal is cited, in accordance with accepted academic practice. No use, distribution or reproduction is permitted which does not comply with these terms.
*Correspondence: Katrin Richter, a2F0cmluLnJpY2h0ZXJAY2hpcnUubWVkLnVuaS1naWVzc2VuLmRl
†These authors have contributed equally to this work.
Disclaimer: All claims expressed in this article are solely those of the authors and do not necessarily represent those of their affiliated organizations, or those of the publisher, the editors and the reviewers. Any product that may be evaluated in this article or claim that may be made by its manufacturer is not guaranteed or endorsed by the publisher.
Research integrity at Frontiers
Learn more about the work of our research integrity team to safeguard the quality of each article we publish.