- Institute of Experimental Immunology, EUROIMMUN AG, Lübeck, Germany
Background: A plurality of neurological syndromes is associated with autoantibodies against neural antigens relevant for diagnosis and therapy. Identification of these antigens is crucial to understand the pathogenesis and to develop specific immunoassays. Using an indirect immunofluorescence assay (IFA)-based approach and applying different immunoprecipitation (IP), chromatographic and mass spectrometric protocols was possible to isolate and identify a spectrum of autoantigens from brain tissue.
Methods: Sera and CSF of 320 patients suspected of suffering from an autoimmune neurological syndrome were comprehensively investigated for the presence of anti-neural IgG autoantibodies by IFA using mosaics of biochips with brain tissue cryosections and established cell-based recombinant antigen substrates as well as immunoblots. Samples containing unknown brain tissue-specific autoantibodies were subjected to IP with cryosections of cerebellum and hippocampus (rat, pig, and monkey) immobilized to glass slides or with lysates produced from homogenized tissue, followed by sodium dodecyl sulfate-polyacrylamide gel electrophoresis, tryptic digestion, and matrix-assisted laser desorption/ionization–time of flight mass spectrometry analysis. Identifications were confirmed by IFA with recombinant HEK293 cells and by neutralizing the patients’ autoantibodies with the respective recombinantly expressed antigens in the tissue-based immunofluorescence test.
Results: Most samples used in this study produced speckled, granular, or homogenous stainings of the hippocampal and cerebellar molecular and/or granular layers. Others exclusively stained the Purkinje cells. Up to now, more than 20 different autoantigens could be identified by this approach, among them ATP1A3, CPT1C, Flotillin1/2, ITPR1, NBCe1, NCDN, RGS8, ROCK2, and Syntaxin-1B as novel autoantigens.
Discussion: The presented antigen identification strategy offers an opportunity for identifying up to now unknown neural autoantigens. Recombinant cell substrates containing the newly identified antigens can be used in serology and the clinical relevance of the autoantibodies can be rapidly evaluated in cohort studies.
Introduction
In autoimmune diseases, an abnormal response of the immune system attacks the body’s own cells causing malfunction or injury. The immune response is often associated with the appearance of autoreactive antibodies (=autoantibodies) that bind specifically to the body’s own structures (=autoantigens). In neurological autoimmune diseases the nervous system is affected as a result. The spectrum of diagnoses of neurological autoimmune disorders has expanded rapidly in the recent years due to the discovery of new anti-neural antibodies.
Most of the initially described anti-neural autoantibodies are directed against intracellular proteins like Hu, Yo, Ri, Ta, GAD, and amphiphysin (1). They are generally considered to be epiphenomena of a T-cell-driven reaction against tumor cells expressing neuronal antigens. Because of their limited access to their target antigens, they probably bear no pathogenic potential in vivo though anti-Hu has recently been shown to activate neurons (2). They are crucial biomarkers for the diagnosis of paraneoplastic autoimmune disorders and often lead to very early diagnosis of the corresponding cancers.
In the past years, a significant number of pathogenic autoantibodies against neural surface-associated proteins have been described, including AQP4, NMDAR, LGI1, CASPR2, AMPAR1, and AMPAR2, GABA-A and -B receptors, glycin receptor, DPPX, mGluR5, IgLON5, and neurexin-3-alpha (1, 3–7). These autoantibodies are frequently non-paraneoplastic, generally occur in association with inflammatory damage to the brain and can trigger seizures, impairment of visual acuity, psychosis-like symptoms, and/or movement disorders.
Most notably, anti-neural autoantibodies are not only important diagnostic markers. Knowing the identity of the corresponding autoantigens also helps to determine the treatment strategy. Patients with antibodies targeting intracellular proteins generally respond poorly to immunosuppressive treatments but need fast onset of oncotherapy in most cases. In contrast, antibodies targeting cell surface proteins can have direct pathogenic effects, and patients’ symptoms often improve after immunotherapy.
The most frequently used method for neural cell surface antigen identification has been immunoprecipitation after antibody binding to live primary hippocampal neurons (5–11). The neuronal cells used for these experiments are isolated from rat embryos and need to be cultivated for at least 14 days to allow differentiation before patient antibodies are applied to the culture. Preparation and cultivation of the cells are elaborate, thus hampering their use for screening of autoantibodies in clinical diagnostics. Moreover, the method is limited as it can only be used if the antigenic target is presented at the surface of the cultivated neurons.
For identification of intracellular antigens, immunoscreening of cDNA expression libraries (12, 13) or libraries of purified recombinant proteins (14) has been used successfully. Other strategies rely on the separation of brain tissue extracts in one- or two-dimensional gel electrophoresis followed by transfer of the separated proteins onto membranes and incubation with patient antibodies (15, 16). In these experiments, the frequency of irrelevant positive results is high because revelation of hidden epitopes may lead to unspecific binding of antibodies to unfolded and/or electrophoretically concentrated proteins. At the same time, these methods often denature the three-dimensional structure of protein antigens. Since autoantibodies sometimes do not bind to structurally non-authentic antigens in these setups, their usefulness is further limited.
Here, we report on an antigen identification strategy that is derived from indirect immunofluorescence assay (IFA) using brain tissue cryosections which is still one of the most versatile screening procedures for anti-neural autoantibodies, especially against up to now unspecified target antigens. Cryosectioning generally conserves the microenvironment of tissues such that protein structures are stabilized and epitopes presented nearly authentical.
Materials and Methods
In the first step, the immunocomplexes formed by the patient’s autoantibodies and the animal tissue cryosections were analyzed in an immunocomplex extraction assay. A combination of histo- or tissue-immunoprecipitation (HIP/TIP), chromatography, and mass spectrometry was used for antigen identification. Correct antigen identification was verified by IFA using the respective antigen recombinantly expressed in HEK293 cells and by neutralizing the autoantibodies’ tissue reaction with the recombinant antigen.
Reagents
Reagents were obtained from Merck, Darmstadt, Germany, or Sigma-Aldrich, Heidelberg, Germany if not specified otherwise.
Human Samples
An ethics approval was not required as per institutional and national guidelines. The serum samples were collected by the clinical immunological laboratory Stöcker, Lübeck (Germany) for the purpose of autoantibody testing and were provided to the authors in an anonymized form. Hence, the authors did not have access to identifiable information. After use for clinical diagnostics the samples were coded and stored at −20°C until first research use after which they were stored at +4°C for further experiments to avoid repeated freeze/thaw cycles. Stored sera samples were used as sources of IgG antibodies.
Control collectives included healthy blood donors and patients with defined autoantibody-associated neurological syndromes.
Indirect Immunofluorescence Assay
Indirect immunofluorescence assay was conducted using slides with a diagnostic biochip screening array of brain tissue cryosections (hippocampus of rat, cerebellum of rat and monkey) combined with recombinant HEK293 cells, each biochip separately expressing the following 30 brain antigens: Hu, Yo, Ri, CV2, SOX1, PNMA1, PNMA2, ARHGAP26, Homer3, CARP VIII, ZIC4, DNER/Tr, GAD65, GAD67, amphiphysin, recoverin, GABAB receptor, glycine receptor, DPPX, glutamate receptors (types NMDA, AMPA, mGluR1, mGluR5), LGI1, CASPR2, AQP4 (M1 and M23), MOG, MP-0, and MAG. Each biochip mosaic was incubated with 70 µL of PBS-diluted sample at room temperature for 30 min, washed with PBS-Tween for 5 min. In the second step, either Alexa488-labeled goat anti-human IgG (Jackson Research, Suffolk, United Kingdom), or fluorescein isothiocyanate-labeled goat anti-human IgG (Euroimmun) were applied and incubated at room temperature for 30 min. Slides were washed again with PBS-Tween for 5 min. Slides were embedded in PBS-buffered, DABCO containing glycerol, and examined by fluorescence microscopy. Positive and negative controls were included. Samples were classified as positive or negative based on fluorescence reactivity of the transfected cells in direct comparison with mock-transfected cells and control samples. Cell nuclei were visualized by DNA staining with TO-PRO3 iodide (dilution 1:2,000) (ThermoFisher Scientific, Schwerte, Germany). In neutralization experiments, recombinant antigens were mixed with diluted serum samples 1 h prior to the IFA as described in Stöcker et al. (17). Results were evaluated independently by two observers using a laser scanning microscope (LSM700, Zeiss, Jena, Germany).
Immunocomplex Extraction Assay
The assay was performed using slides with a biochip array of brain tissue cryosections (rat hippocampus, rat cerebellum, and monkey cerebellum). Each biochip mosaic was incubated with PBS-diluted sample at room temperature for 30 min, washed with PBS-Tween, and immersed in PBS-Tween for 5 min. In the second step, the slides were incubated either in extraction buffer [100 mmol/L Tris–HCl pH 7.4, 150 mmol/L sodium chloride, 2.5 mmol/L ethylenediamine tetraacetic acid (EDTA), 0.5% (w/v) deoxycholate, and 1% (w/v) Triton X-100 containing protease inhibitors] or in detergent-free control buffer for 30 min at room temperature while gently shaking. After washing with PBS-Tween, the incubation with secondary antibody, washing, and preparation of slides fluorescence microscopy was performed as described for IFA. Extraction of the immunocomplexes was estimated by direct comparison of the signal intensity of the biochip mosaics incubated with extraction buffer or with the control buffer.
Histo-Immunoprecipitation
Cerebellum from rat or pig was dissected and shock-frozen in −160°C isopentane. The tissue was cryosectioned (4 µm) with a SM2000R microtome (Leica Microsystems, Nussloch, Germany), placed on the entire surface of glass slides, and dried. Whole slides were then incubated with patient’s serum (diluted 1:100) at 4°C for 3 h followed by three washing steps with PBS containing 0.2% (w/v) Tween 20. Immunocomplexes were extracted from the sections by incubation in extraction buffer [100 mmol/L Tris–HCl pH 7.4, 150 mmol/L sodium chloride, 2.5 mmol/L EDTA, 0.5% (w/v) deoxycholate, and 1% (w/v) Triton X-100 containing protease inhibitors] at room temperature for 30 min. Detached material was homogenized and centrifuged at 16,000 × g at 4°C for 15 min. The clear supernatants were then incubated with Protein G Dynabeads (ThermoFisher Scientific, Dreieich, Germany) at 4°C overnight to capture immunocomplexes. The beads were washed three times with PBS and eluted with NuPage LDS sample buffer (ThermoFisher Scientific, Schwerte, Germany) containing 25 mmol/L dithiothreitol at 70°C for 10 min. Carbamidomethylation with 59 mM iodoacetamide (Bio-Rad, Hamburg, Germany) was performed prior to sodium dodecyl sulfate (SDS)-polyacrylamide gel electrophoresis (PAGE) (NuPAGE, ThermoFisher Scientific, Schwerte, Germany). Separated proteins were visualized with Coomassie Brilliant Blue (G-250) (Merck) and identified by mass spectrometric (MS) analysis (see below for details).
Tissue-Immunoprecipitation
Hippocampus or cerebellum from rat was dissected and shock-frozen in liquid nitrogen. The tissues were homogenized in extraction buffer [100 mmol/L Tris–HCl pH 7.4, 150 mmol/L sodium chloride, 2.5 mmol/L EDTA, 0.5% (w/v) sodium deoxycholate, and 1% (w/v) Triton X-100] containing protease inhibitors (Complete mini, Roche Diagnostics, Penzberg, Germany) with a Miccra D-8 (Roth, Karlsruhe, Germany) and a hand homogenizer (Sartorius, Göttingen, Germany) at 4°C. The tissue lysates was centrifuged at 21,000 × g at 4°C for 15 min and clear supernatants were incubated with patient’s serum (diluted 1:33) at 4°C for 3 h. Pulldown of immunocomplexes and analysis of eluate fractions was performed as described for HIP.
Mass Spectrometry
Mass spectrometry sample preparation was performed similar to Koy et al. (18). Unless otherwise indicated, hardware, software, MALDI targets, peptide standards, and matrix reagents were obtained from Bruker Daltonics, Bremen, Germany.
Briefly, visible protein bands were excised from Coomassie Brilliant Blue G-250 stained gels. After destaining and tryptic digestion peptides were extracted and spotted with α-cyano-4-hydroxycinnamic acid onto a MTP AnchorChip™ 384 TF target.
Matrix-assisted laser desorption/ionization–time of flight mass spectrometry/TOF measurements were performed with an Autoflex III smartbeam TOF/TOF200 System using flexControl 3.0, 3.3, or 3.4 software. MS spectra for peptide mass fingerprinting (PMF) were recorded in positive ion reflector mode with 4,000–10,000 shots and in a mass range from 600 to 4,000 Da. Spectra were calibrated externally with the commercially available Peptide Calibration Standard II, processed with flexAnalysis 3.0, 3.3, or 3.4 and peak lists were analyzed with BioTools 3.2.
The Mascot search engine Mascot Server 2.3 (Matrix Science, London, UK) was used for protein identification by searching against the NCBI or SwissProt database limited to Mammalia. Search parameters were as follows: mass tolerance was set to 80 ppm, one missed cleavage site was accepted, and carbamidomethylation of cysteine residues as well as oxidation of methionine residues were set as fixed and variable modifications, respectively. To evaluate the protein hits, a significance threshold of p < 0.05 was chosen.
For further confirmation of the PMF hits two to five peptides of each identified protein were selected for MS/MS measurements using the WARP feedback mechanism of BioTools. Parent and fragment masses were recorded with 400 and 1,000 shots, respectively. Spectra were processed and analyzed as described above with a fragment mass tolerance of 0.7 Da.
Recombinant Expression of Antigens in HEK293 Cells
For cloning details see Table S1 in Supplementary Material. The antigens were expressed in the human cell line HEK293 after ExGen500-mediated transfection (ThermoFisher Scientific) according to the manufacturer’s instructions.
For the preparation of IFA substrates, HEK293 were grown on sterile cover glasses, transfected, and allowed to express the recombinant antigens for 48 h. Cover glasses were washed with PBS, fixed with acetone for 10 min at room temperature, air-dried, cut into 2 mm × 2 mm-sized fragments (biochips) and used as substrates in IFA as described. Alternatively, cells were transfected in standard T-flasks and the cells harvested after 48 h. The cell suspension was centrifuged at 1,500 × g, 4°C for 20 min and the resulting sediment extracted with 20 mmol/L Tris–HCl pH 7.4, 50 mmol/L potassium chloride, 5 mmol/L EDTA. The extracts were stored in aliquots at −80°C until further use.
Results
Results of Serum Prescreening by IFA
The samples used in this study had originally been sent to the Clinical Immunological Laboratory for determination of anti-neural autoantibodies between 01/2011 and 07/2016. The 320 sera included in this study showed different IgG staining patterns on brain tissue cryosections (hippocampus of rat, cerebellum of rat and monkey) with a minimum endpoint titer of 1:100 (Figure S1 in Supplementary Material). Most samples (n = 261) produced speckled, granular, or homogenous staining of the hippocampal and cerebellar molecular and granular layers. Others (n = 59) stained cerebellar Purkinje cells. None of these sera revealed reactivity with the monospecific biochips of the diagnostic biochip screening array.
Immunocomplex Extractability
The extractability of immunocomplexes from cryosections bound to the glass slide surface was determined for each serum sample prior to immunoprecipitation. For sera which showed medium to high extractability (n = 182), HIP was applied, while for the other sera TIP was used (n = 138) (Figure 1).
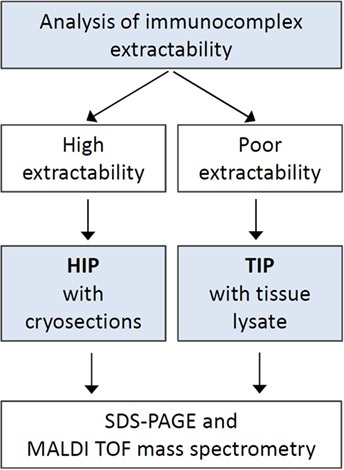
Figure 1. Procedure diagram. Schematic representation of the steps of antigen identification. Abbreviations: HIP, histo-immunoprecipitation; TIP, tissue-immunoprecipitation.
Some sera did not show any differences in the signal intensities after using extraction and control buffer, while other sera showed slight or even strong reduction of signal intensity after incubation with extraction buffer (Figure 2). A strong decrease of signal intensity was interpreted as high extractability of the respective immunocomplexes.
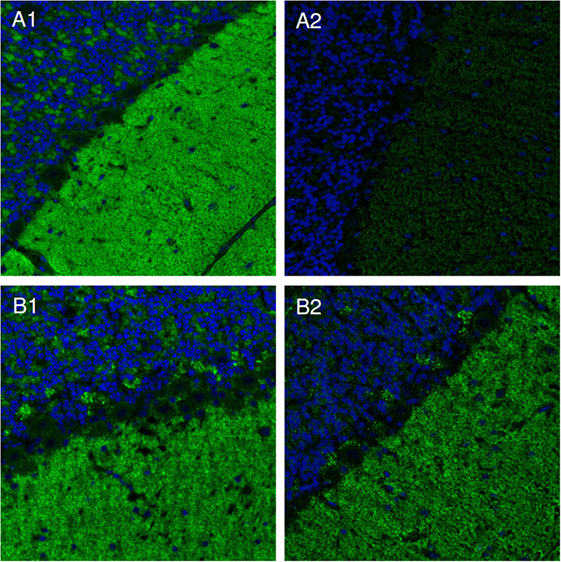
Figure 2. Immunocomplex extraction. Slides with cerebellum cryosections were incubated with patients’ sera and subsequently with either extraction [100 mmol/L Tris–HCl pH 7.4, 150 mmol/L sodium chloride, 2.5 mmol/L ethylenediamine tetraacetic acid, 0.5% (w/v) deoxycholate, 1% (w/v) Triton X-100] (A2,B2), or detergent-free control buffer (A1,B1). Bound IgG was visualized by Alexa488-labeled goat anti-human IgG. Nuclei were counterstained by incubation with TO-PRO-3 iodide (blue). Examples of soluble (A) and insoluble (B) immunocomplexes are shown.
Identified Autoantigens
Coomassie stained SDS-PAGE of eluate fractions obtained from HIP preparations generally showed lower numbers of protein bands and less IgG compared to TIP eluate fractions (Figure 3B), because washing the cryosections attached to glass coverslides removed more effectively unbound antibodies (Figure 3A). Specific antigen bands and unspecific protein bands were discriminated by comparison with eluate fractions of control sera. Using matrix-assisted laser desorption/ionization–time of flight mass spectrometry (MALDI–TOF MS), several antigens could be identified, among them: AP3B2, ATP1A3, CLIP1, CNTN1/CASPR1, CPT1C, ERC1, Flotillin1/2, GLURD2, GRIPAP1, Hexokinase-1, Homer 3, ITPR1, KCNA2, NBCe1, Neurochondrin, RGS8, ROCK2, RyR2, and STX1b (Table 1). The immunoprecipitation method which was performed for the identification of each antigen is indicated in Table 2.
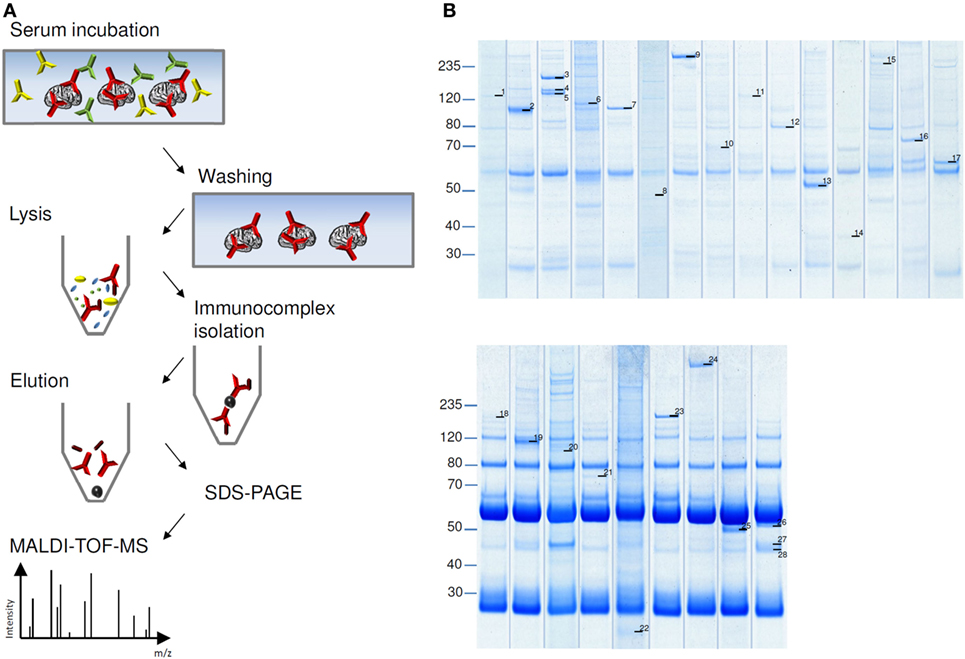
Figure 3. Immunoprecipitation of autoantigens. (A) Schema of histo-immunoprecipitation (HIP) with cerebellum cryosections immobilized on glass slides. Slides were incubated with patient’s serum followed by washing and removal of unbound antibodies. Cells were lysed and soluble immunocomplexes were isolated by protein-G-coated magnetic beads. Sodium dodecyl sulfate (SDS)-eluted proteins were separated by SDS-polyacrylamide gel electrophoresis and visualized by staining with colloidal coomassie blue. Antigen bands were prepared and analyzed with matrix-assisted laser desorption/ionization–time of flight mass spectrometry (MALDI–TOF MS). (B) Examples of HIP (upper panel) and tissue-immunoprecipitation (lower panel). Labeled protein bands were analyzed in MALDI–TOF MS. Results are listed in Table 1.
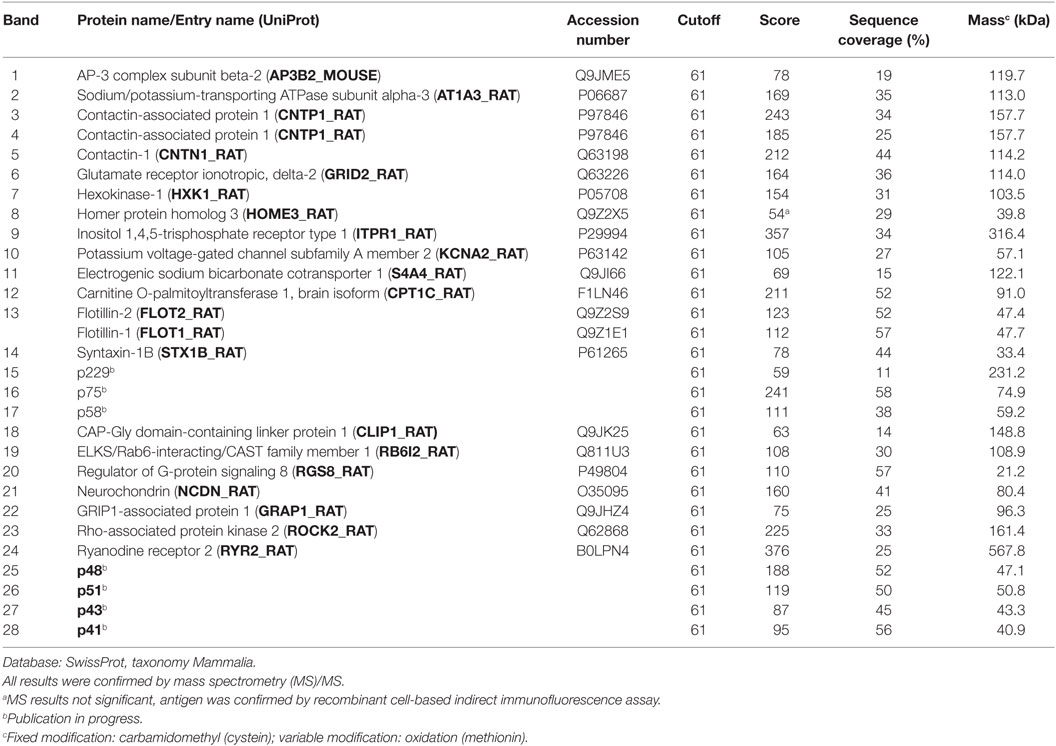
Table 1. Proteins identified from immunoprecipitates using matrix-assisted laser desorption/ionization–time of flight mass spectrometry by peptide mass fingerprinting.
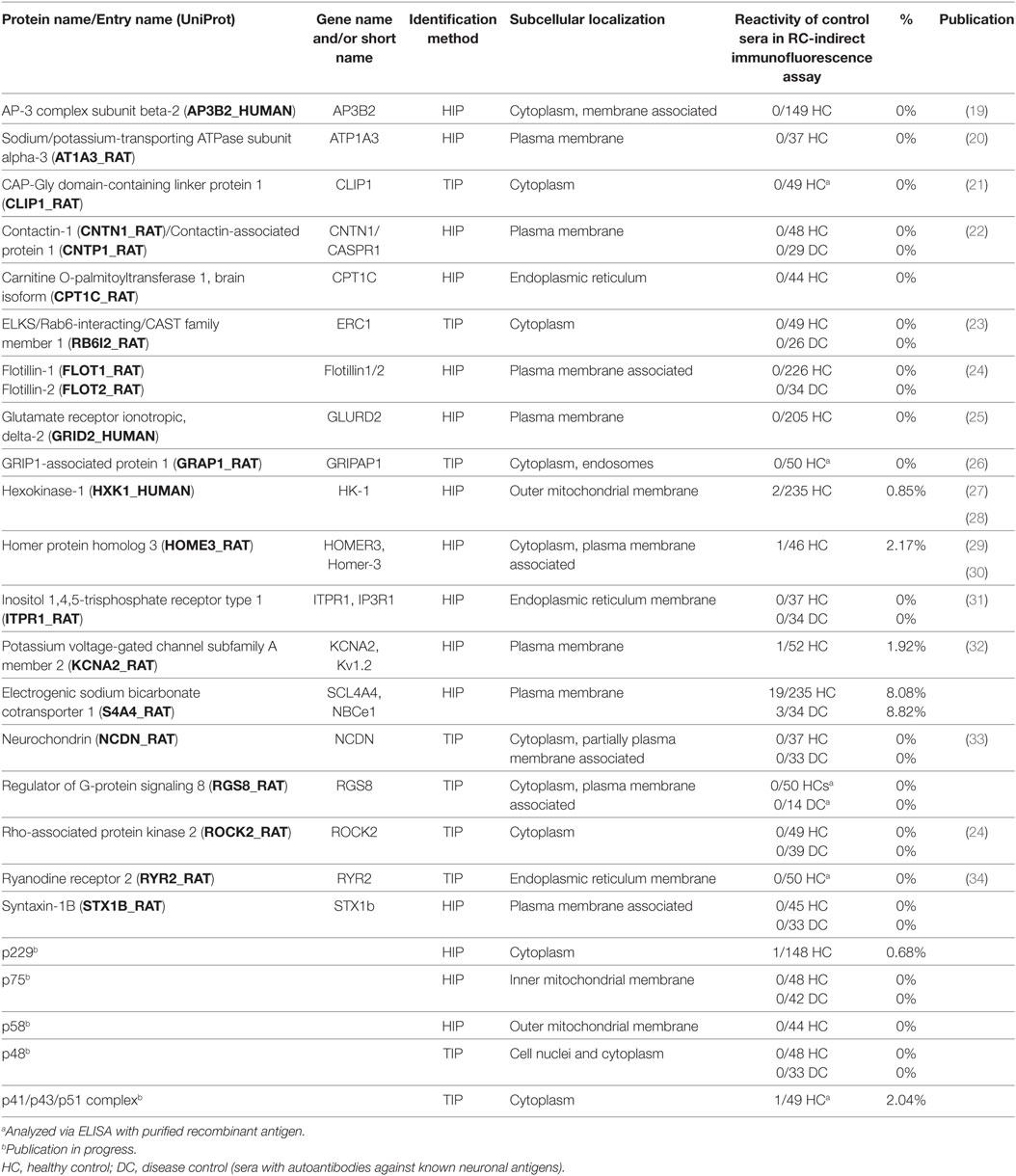
Table 2. Antigens identified using histo-immunoprecipitation (HIP) or tissue-immunoprecipitation (TIP).
Verification
As a proof for correct antigen identification, the patients’ samples were then tested by IFA using transfected HEK293 cells which expressed the new target antigens (Figure 4A). Characteristic staining patterns were obtained on all cellular substrates containing the respective recombinant target antigens, while there were no corresponding stainings of mock-transfected cells or cells expressing the other target antigens.
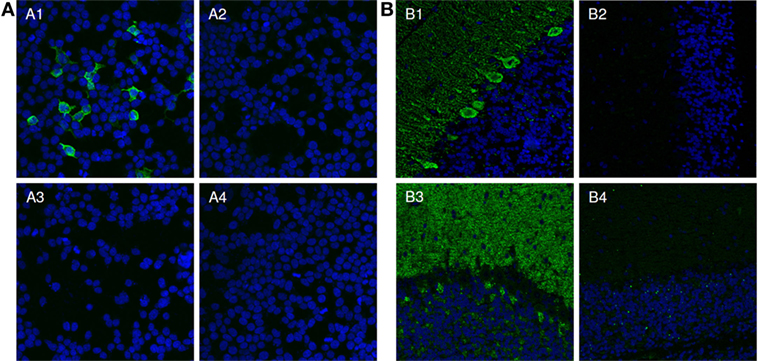
Figure 4. Verification of identified antigens by indirect immunofluorescence. (A) Indirect immunofluorescence analysis of transfected HEK293 cells. Acetone-fixed recombinant HEK293 cells expressing the protein of interest (1, 3) or a mock-transfected control (2, 4) were incubated with patient serum (1, 2) or with control serum (3, 4) (both 1:1,000). Cell nuclei were counterstained with TO-PRO-3 iodide (blue). Only HEK293-expressing the protein of interest reacted with the patient sera (green). (B) Two examples for the neutralization of immunofluorescence reaction on neuronal tissues. Serum 1 and 2 (green) were pre-incubated with extracts of HEK293 cells transfected with empty control vector (1, 3) or the plasmid harboring the cDNA of interest and analyzed in an indirect immunofluorescence assay with rat cerebellar cryosections (2, 4). The extract containing the protein of interest greatly reduced or abolished the immune reaction of the serum on rat cerebellum (2, 4). The control extracts had no effect (1, 3). Nuclei were counterstained by incubation with TO-PRO-3 iodide (blue).
The reaction of the patients’ autoantibodies on brain tissue sections could be abolished or significantly reduced in all cases by pre-incubation with HEK293 lysate containing the respective recombinant antigen (Figure 4B). Antibody binding was unaffected when a comparable fraction from mock-transfected HEK293 cells was used.
Sera from patients with various anti-neural autoantibodies as disease controls (anti-NMDAR, anti-Hu, anti-Yo, anti-Ri, anti-AQP4, anti-LGI1, and anti-CASPR2), and sera from healthy blood donors were analyzed by IFA or ELISA with the recombinant antigens in parallel to the samples of the index patients. 18 of the 24 recombinant substrates showed no positive reactions with control sera. With five recombinant antigens only around 1–2% of the healthy blood donor sera reacted in a 1:10 dilution (Table 2).
Discussion
Here, we describe a potent strategy to discover new neural autoantigens. Starting point is the definition of a characteristic IFA staining pattern on neural tissue. In sera of patients with putative neurological autoimmune diseases reacting with cryosections of cerebellum or hippocampus by indirect immunofluorescence but not with established brain autoantigens, we were able to identify more than 20 different target antigens. We verified all antigen identifications by (1) specific immunostaining of HEK293 cells expressing the respective recombinant antigens by the patient’s IgG and (2) specific competitive inhibition of the patient’s IgG antibody by pre-incubation with the antigen extracted from recombinant HEK293 cells.
In the first step, we analyzed the immunocomplex extractability, which determined the immunoprecipitation approach. Analyzing immunocomplex extractability rather than antigen extractability is beneficial, as autoantibody binding in first step of our HIP protocol might influence antigen solubility. The extraction buffer contained 0.5% (w/v) deoxycholate and 1% (w/v) Triton X-100 as detergents. Other solubilization agents like 0.1% SDS, as well as increased salt-concentration or pH variations could be used to adjust optimal extraction conditions for individual immunocomplexes.
In the second step, HIP was performed for extractable immunocomplexes. The antibodies bind to their targets, which are presented in their natural environment and conformation on cryosections of rat or primate cerebellum immobilized on glass slides. If HIP was not performed because of poor immunocomplex extractability, we were successful in nine cases using a classical immunoprecipitation approach by incubating tissue lysates with patients’ sera (TIP) (Table 2). In TIP, the antigens were solubilized prior to antibody binding, avoiding antigen insolubility by antibody cross linkage.
A number of techniques for the identification of autoantigens have been in use, since the discovery of autoantibodies. One approach that has been used for the discovery of neuronal surface-autoantigens in the past years relies on cultivated intact primary hippocampal neurons of rat embryos as antigen source followed by immunoprecipitation and mass spectrometry like in our protocol. Though it is focused on antibody-accessible surface proteins, and thus promises the discovery of immunosuppressable disease phenotypes it also has limitations. In particular, isolation and cultivation of these cells requires sophisticated technical skills and is highly labor-intensive. Moreover, intracellular antigens cannot be discovered by living cell-based immunoprecipitation. HIP provides better temporal flexibility as substrates can be prepared in bulk amounts and stored in aliquots for later use. In addition, cell surface and intracellular antigens are presented in their natural conformation and are accessible to antibody binding.
Compared to TIP, HIP immunoprecipitates showed fewer background bands and only weak IgG bands due to removal of unbound antibodies. This simplifies the selection of bands to be analyzed in MALDI–TOF MS and reduces false identifications. HIP also has the potential to be used for target antigen identification in patients with other organ-specific or not organ-specific autoimmune diseases.
Using HIP, five cell surface proteins could be identified (Table 2). Among them GLURD2, KCNA2, and CNTN1/CASPR1 have previously been reported as neuronal autoantigens (22, 32, 35). Out of the 19 identified intracellular antigens 7 are known antigenic targets in autoimmune diseases (19, 21, 23, 26–29, 34). Moreover, we were able to identify 14 novel autoantigens. Not all of the antigens we identified are exclusively expressed in neural tissues. For some antigens like ATP1A3, ITPR1, or ROCK2 an expression in tumor samples of the respective index patient was observed, pointing to a paraneoplastic autoimmune background (20, 31, 36). Antigens like Flotillin1/2, GRIPAP1, or CLIP1 are also expressed in non-neural tissues without a tumor association (21, 24, 26). However, other autoantibodies, like AQP4 or DPPX which are established markers for neurological autoimmune diseases (37, 38) show also expression in non-neuronal tissues.
Following the successful identification of target autoantigens based on index samples, cohort studies including patients with similar neurological symptoms and disease as well as healthy controls must validate whether the discovered autoantibodies appear rarely or are common markers for specific phenotypes like autoantibody-mediated brain disorders or paraneoplastic neurological syndromes. For autoantibodies against ATP1A3, ITPR1, NCDN, and ROCK2 an association to autoimmune cerebellar syndromes has already been demonstrated by the authors (20, 31, 33, 36). Moreover, anti-Flotillin1/2 autoantibodies were found to be present in multiple sclerosis patients (24). Thus, the antigen identification strategy that we present offers a potent instrument for identifying unknown autoantigens and contributes to better diagnosis of autoimmune diseases.
Author Contributions
MS, SK, SH, and RM were involved in immunoprecipitation, interpretation of data, antibody testing, and microscopy. MS and RM were involved in writing of the manuscript. NB and YD performed mass spectrometric analysis. NR, CR, SB, and CP performed molecular biology work. BT and WS were involved in supervising of antibody testing and microscopy. WS and LK were involved in the conception and organization of the research project and in writing of the manuscript.
Conflict of Interest Statement
MS, RM, SK, SH, YD, NB, NR, CR, SB, CP, BT, and LK are employees of the Euroimmun AG, a company that develops, produces, and manufactures immunoassays for the detection of disease-associated antibodies. WS is member of the Board of the Euroimmun AG.
Acknowledgments
We thank Susann Satow, Laura Olejko, Jonas Joneleit, and Beatrice Witt for their excellent technical assistance.
Supplementary Material
The Supplementary Material for this article can be found online at https://www.frontiersin.org/articles/10.3389/fimmu.2018.01447/full#supplementary-material.
Abbreviations
EDTA, ethylenediamine tetraacetic acid; FITC, fluorescein isothiocyanate; HIP, histo-immunoprecipitation; IFA, indirect immunofluorescence assay; MALDI–TOF MS, matrix-assisted laser desorption/ionization–time of flight mass spectrometry; PAGE, polyacrylamide gel electrophoresis; PMF, peptide mass fingerprint; RC-IFA, recombinant cell-based indirect immunofluorescence assay; SDS, sodium dodecyl sulfate; TIP, tissue-immunoprecipitation.
References
1. Probst C, Saschenbrecker S, Stoecker W, Komorowski L. Anti-neuronal autoantibodies: current diagnostic challenges. Mult Scler Relat Disord (2014) 3(3):303–20. doi:10.1016/j.msard.2013.12.001
2. Li Q, Michel K, Annahazi A, Demir IE, Ceyhan GO, Zeller F, et al. Anti-Hu antibodies activate enteric and sensory neurons. Sci Rep (2016) 6:38216. doi:10.1038/srep38216
3. Lennon VA, Kryzer TJ, Pittock SJ, Verkman AS, Hinson SR. IgG marker of optic-spinal multiple sclerosis binds to the aquaporin-4 water channel. J Exp Med (2005) 202(4):473–7. doi:10.1084/jem.20050304
4. Dalmau J, Gleichman AJ, Hughes EG, Rossi JE, Peng X, Lai M, et al. Anti-NMDA-receptor encephalitis: case series and analysis of the effects of antibodies. Lancet Neurol (2008) 7(12):1091–8. doi:10.1016/S1474-4422(08)70224-2
5. Boronat A, Gelfand JM, Gresa-Arribas N, Jeong HY, Walsh M, Roberts K, et al. Encephalitis and antibodies to dipeptidyl-peptidase-like protein-6, a subunit of Kv4.2 potassium channels. Ann Neurol (2013) 73(1):120–8. doi:10.1002/ana.23756
6. Petit-Pedrol M, Armangue T, Peng X, Bataller L, Cellucci T, Davis R, et al. Encephalitis with refractory seizures, status epilepticus, and antibodies to the GABAA receptor: a case series, characterisation of the antigen, and analysis of the effects of antibodies. Lancet Neurol (2014) 13(3):276–86. doi:10.1016/S1474-4422(13)70299-0
7. Sabater L, Gaig C, Gelpi E, Bataller L, Lewerenz J, Torres-Vega E, et al. A novel non-rapid-eye movement and rapid-eye-movement parasomnia with sleep breathing disorder associated with antibodies to IgLON5: a case series, characterisation of the antigen, and post-mortem study. Lancet Neurol (2014) 13(6):575–86. doi:10.1016/S1474-4422(14)70051-1
8. Lai M, Hughes EG, Peng X, Zhou L, Gleichman AJ, Shu H, et al. AMPA receptor antibodies in limbic encephalitis alter synaptic receptor location. Ann Neurol (2009) 65(4):424–34. doi:10.1002/ana.21589
9. Lai M, Huijbers MG, Lancaster E, Graus F, Bataller L, Balice-Gordon R, et al. Investigation of LGI1 as the antigen in limbic encephalitis previously attributed to potassium channels: a case series. Lancet Neurol (2010) 9(8):776–85. doi:10.1016/S1474-4422(10)70137-X
10. Lancaster E, Lai M, Peng X, Hughes E, Constantinescu R, Raizer J, et al. Antibodies to the GABA(B) receptor in limbic encephalitis with seizures: case series and characterisation of the antigen. Lancet Neurol (2010) 9(1):67–76. doi:10.1016/S1474-4422(09)70324-2
11. Gresa-Arribas N, Planaguma J, Petit-Pedrol M, Kawachi I, Katada S, Glaser CA, et al. Human neurexin-3alpha antibodies associate with encephalitis and alter synapse development. Neurology (2016) 86(24):2235–42. doi:10.1212/WNL.0000000000002775
12. Gure AO, Stockert E, Scanlan MJ, Keresztes RS, Jager D, Altorki NK, et al. Serological identification of embryonic neural proteins as highly immunogenic tumor antigens in small cell lung cancer. Proc Natl Acad Sci U S A (2000) 97(8):4198–203. doi:10.1073/pnas.97.8.4198
13. Bataller L, Sabater L, Saiz A, Serra C, Claramonte B, Graus F. Carbonic anhydrase-related protein VIII: autoantigen in paraneoplastic cerebellar degeneration. Ann Neurol (2004) 56(4):575–9. doi:10.1002/ana.20238
14. Jarius S, Wandinger KP, Horn S, Heuer H, Wildemann B. A new Purkinje cell antibody (anti-Ca) associated with subacute cerebellar ataxia: immunological characterization. J Neuroinflammation (2010) 7:21. doi:10.1186/1742-2094-7-21
15. Dalmau J, Furneaux HM, Gralla RJ, Kris MG, Posner JB. Detection of the anti-Hu antibody in the serum of patients with small cell lung cancer – a quantitative western blot analysis. Ann Neurol (1990) 27(5):544–52. doi:10.1002/ana.410270515
16. Honnorat J, Antoine JC, Derrington E, Aguera M, Belin MF. Antibodies to a subpopulation of glial cells and a 66 kDa developmental protein in patients with paraneoplastic neurological syndromes. J Neurol Neurosurg Psychiatry (1996) 61(3):270–8. doi:10.1136/jnnp.61.3.270
17. Stöcker W, Otte M, Ulrich S, Normann D, Finkbeiner H, Stocker K, et al. Autoimmunity to pancreatic juice in Crohn’s disease. Results of an autoantibody screening in patients with chronic inflammatory bowel disease. Scand J Gastroenterol Suppl (1987) 139:41–52. doi:10.3109/00365528709089774
18. Koy C, Mikkat S, Raptakis E, Sutton C, Resch M, Tanaka K, et al. Matrix-assisted laser desorption/ionization-quadrupole ion trap-time of flight mass spectrometry sequencing resolves structures of unidentified peptides obtained by in-gel tryptic digestion of haptoglobin derivatives from human plasma proteomes. Proteomics (2003) 3(6):851–8. doi:10.1002/pmic.200300381
19. Darnell RB, Furneaux HM, Posner JB. Antiserum from a patient with cerebellar degeneration identifies a novel protein in Purkinje cells, cortical neurons, and neuroectodermal tumors. J Neurosci (1991) 11(5):1224–30. doi:10.1523/JNEUROSCI.11-05-01224.1991
20. Scharf M, Miske R, Heidenreich F, Giess R, Landwehr P, Blocker IM, et al. Neuronal Na+/K+ ATPase is an autoantibody target in paraneoplastic neurologic syndrome. Neurology (2015) 84(16):1673–9. doi:10.1212/WNL.0000000000001493
21. Griffith KJ, Ryan JP, Senecal JL, Fritzler MJ. The cytoplasmic linker protein CLIP-170 is a human autoantigen. Clin Exp Immunol (2002) 127(3):533–8. doi:10.1046/j.1365-2249.2002.01756.x
22. Querol L, Nogales-Gadea G, Rojas-Garcia R, Martinez-Hernandez E, Diaz-Manera J, Suarez-Calvet X, et al. Antibodies to contactin-1 in chronic inflammatory demyelinating polyneuropathy. Ann Neurol (2013) 73(3):370–80. doi:10.1002/ana.23794
23. Huijbers MG, Lipka AF, Potman M, Hensbergen PJ, Titulaer MJ, Niks EH, et al. Antibodies to active zone protein ERC1 in Lambert-Eaton myasthenic syndrome. Hum Immunol (2013) 74(7):849–51. doi:10.1016/j.humimm.2013.03.004
24. Hahn S, Trendelenburg G, Scharf M, Denno Y, Brakopp S, Teegen B, et al. Identification of the flotillin-1/2 heterocomplex as a target of autoantibodies in bona fide multiple sclerosis. J Neuroinflammation (2017) 14(1):123. doi:10.1186/s12974-017-0900-z
25. Miske R, Hahn S, Rosenkranz T, Müller M, Dettmann IM, Mindorf S, et al. Autoantibodies against glutamate receptor δ2 after allogenic stem cell transplantation. Neurol Neuroimmunol Neuroinflamm (2016) 3(4):e255. doi:10.1212/NXI.0000000000000255
26. Stinton LM, Selak S, Fritzler MJ. Identification of GRASP-1 as a novel 97 kDa autoantigen localized to endosomes. Clin Immunol (2005) 116(2):108–17. doi:10.1016/j.clim.2005.03.021
27. Gonzalez-Gronow M, Cuchacovich M, Francos R, Cuchacovich S, Fernandez Mdel P, Blanco A, et al. Antibodies against the voltage-dependent anion channel (VDAC) and its protective ligand hexokinase-I in children with autism. J Neuroimmunol (2010) 227(1–2):153–61. doi:10.1016/j.jneuroim.2010.06.001
28. Norman GL, Yang CY, Ostendorff HP, Shums Z, Lim MJ, Wang J, et al. Anti-kelch-like 12 and anti-hexokinase 1: novel autoantibodies in primary biliary cirrhosis. Liver Int (2015) 35(2):642–51. doi:10.1111/liv.12690
29. Zuliani L, Sabater L, Saiz A, Baiges JJ, Giometto B, Graus F. Homer 3 autoimmunity in subacute idiopathic cerebellar ataxia. Neurology (2007) 68(3):239–40. doi:10.1212/01.wnl.0000251308.79366.f9
30. Höftberger R, Sabater L, Ortega A, Dalmau J, Graus F. Patient with homer-3 antibodies and cerebellitis. JAMA Neurol (2013) 70(4):506–9. doi:10.1001/jamaneurol.2013.1955
31. Jarius S, Scharf M, Begemann N, Stocker W, Probst C, Serysheva II, et al. Antibodies to the inositol 1,4,5-trisphosphate receptor type 1 (ITPR1) in cerebellar ataxia. J Neuroinflammation (2014) 11:206. doi:10.1186/s12974-014-0206-3
32. Hart IK, Waters C, Vincent A, Newland C, Beeson D, Pongs O, et al. Autoantibodies detected to expressed K+ channels are implicated in neuromyotonia. Ann Neurol (1997) 41(2):238–46. doi:10.1002/ana.410410215
33. Miske R, Gross CC, Scharf M, Golombeck KS, Hartwig M, Bhatia U, et al. Neurochondrin is a neuronal target antigen in autoimmune cerebellar degeneration. Neurol Neuroimmunol Neuroinflamm (2017) 4(1):e307. doi:10.1212/NXI.0000000000000307
34. Mygland A, Tysnes OB, Matre R, Volpe P, Aarli JA, Gilhus NE. Ryanodine receptor autoantibodies in myasthenia gravis patients with a thymoma. Ann Neurol (1992) 32(4):589–91. doi:10.1002/ana.410320419
35. Shiihara T, Kato M, Konno A, Takahashi Y, Hayasaka K. Acute cerebellar ataxia and consecutive cerebellitis produced by glutamate receptor delta2 autoantibody. Brain Dev (2007) 29(4):254–6. doi:10.1016/j.braindev.2006.09.004
36. Popkirov S, Ayzenberg I, Hahn S, Bauer J, Denno Y, Rieckhoff N, et al. Rho-associated protein kinase 2 (ROCK2): a new target of autoimmunity in paraneoplastic encephalitis. Acta Neuropathol Commun (2017) 5(1):40. doi:10.1186/s40478-017-0447-3
37. Radicke S, Cotella D, Graf EM, Ravens U, Wettwer E. Expression and function of dipeptidyl-aminopeptidase-like protein 6 as a putative beta-subunit of human cardiac transient outward current encoded by Kv4.3. J Physiol (2005) 565(Pt 3):751–6. doi:10.1113/jphysiol.2005.087312
Keywords: neural autoantibodies, immunoprecipitation, antigen identification, autoantigens, indirect immunofluorescence
Citation: Scharf M, Miske R, Kade S, Hahn S, Denno Y, Begemann N, Rochow N, Radzimski C, Brakopp S, Probst C, Teegen B, Stöcker W and Komorowski L (2018) A Spectrum of Neural Autoantigens, Newly Identified by Histo-Immunoprecipitation, Mass Spectrometry, and Recombinant Cell-Based Indirect Immunofluorescence. Front. Immunol. 9:1447. doi: 10.3389/fimmu.2018.01447
Received: 02 November 2017; Accepted: 11 June 2018;
Published: 09 July 2018
Edited by:
Herman Waldmann, University of Oxford, United KingdomReviewed by:
David Cameron Wraith, University of Birmingham, United KingdomLucienne Chatenoud, Université Paris Descartes, France
Copyright: © 2018 Scharf, Miske, Kade, Hahn, Denno, Begemann, Rochow, Radzimski, Brakopp, Probst, Teegen, Stöcker and Komorowski. This is an open-access article distributed under the terms of the Creative Commons Attribution License (CC BY). The use, distribution or reproduction in other forums is permitted, provided the original author(s) and the copyright owner are credited and that the original publication in this journal is cited, in accordance with accepted academic practice. No use, distribution or reproduction is permitted which does not comply with these terms.
*Correspondence: Madeleine Scharf, bS5zY2hhcmYmI3gwMDA0MDtldXJvaW1tdW4uZGU=;
Ramona Miske, ci5taXNrZSYjeDAwMDQwO2V1cm9pbW11bi5kZQ==
†These authors have contributed equally to this work.