- 1Department of Pharmacology and Cancer Biology, Duke University School of Medicine, Durham, NC, United States
- 2Department of Zoology, University of Lagos, Lagos, Nigeria
Benzene, toluene, ethylbenzene and xylene, also known as BTEX, are released into environmental media by petroleum product exploratory and exploitative activities and are harmful to humans and animals. Testing the effects of these chemicals on a significantly large scale requires an inexpensive, rapidly developing model organism such as Drosophila melanogaster. In this study, the toxicological profile of benzene, toluene, ethylbenzene, p-xylene, m-xylene, and o-xylene in D. melanogaster was evaluated. Adult animals were monitored for acute toxicity effects. Similarly, first instar larvae reared separately on the same compounds were monitored for the ability to develop into adult flies (eclosion). Further, the impact of fixed concentrations of benzene and xylene on apoptosis and mitosis were investigated in adult progenitor tissues found in third instar larvae. Toluene is the most toxic to adult flies with an LC50 of 0.166 mM, while a significant and dose-dependent decrease in fly eclosion was observed with benzene, p-xylene, and o-xylene. An increase in apoptosis and mitosis was also observed in animals exposed to benzene and p-xylene. Through Genome Wide Association Screening (GWAS), 38 regions of the D. melanogaster genome were identified as critical for responses to p-xylene. This study reveals the strength of D. Melanogaster genetics as an accessible approach to study BTEX compounds.
Introduction
Volatile organic aromatic compounds (mainly BTEX) have experienced a surge in their environmental presence as a result of increased industrial combustions, vehicular movement, and petroleum operations (Ojiodu, 2013; Cheng et al., 2018). Several years of oil exploration by Multinational Corporations as well as the spillage and gas flaring associated with such activities has led to a degraded environment (Ebegbulem et al., 2013). Release of petroleum hydrocarbons into the environment, whether by accident or due to anthropogenic activities, impacts water and soil and may contribute to regional or atmospheric pollution (Ite et al., 2013). Aside from oil spills and accidents, petroleum pollutant sources could be due to lack of maintenance culture, deliberate acts of violence, bunkering evaporative emission in gas stations, and release of volatile components of gasoline through automobile exhausts (McInnes, 1996; Nwilo and Badejo, 2005). Benzene, toluene, ethylbenzene, and xylene (BTEX) and other aromatic hydrocarbons are predominant components of emissions from gasoline and diesel-powered engines (Friedrich and Obermeier, 1999). Leading producers of petroleum products are now characterized by large scale oil utilization and industrial processes, leading to high pollution and release of volatile organic compounds (Olumayede and Okuo, 2012). Benzene and its alkylated derivatives are well-known for their mutagenicity and carcinogenicity in living organisms and are threat to public health. The United States Environmental Protection Agency (EPA) has classified benzene as a group A carcinogen (Snyder and Hedli, 1996; Thammakhet et al., 2004). Xylene in particular has been recommended to be listed in the National Priorities List (NPL) by the EPA (HHS, 2007).
Occupational exposures to BTEX occur at work places via inhalation or adsorption through the skin. Exposure levels to these compounds at the industrial level have been established through many monitoring and risk assessment studies (Moolla et al., 2015; Ruchirawat et al., 2010; Ciarrocca et al., 2012). Workers and drivers at refueling stations are exposed to high concentrations of BTEX in vehicles and in gas station environments (Esteve-Turrillas et al., 2007; Hazrati et al., 2016; Mosaddegh Mehrjerdi et al., 2014). Histopathological technicians are a high-risk group because they constantly come in contact with solvents already contaminated with xylene (Kandyala et al., 2010).
Benzene, toluene, ethylbenzene, and xylene compounds are not exclusively important in industrial areas. These compounds also pose a threat to household environments as samples of BTEX have been discovered in residential areas and other locations distant from gas stations and other major sources (Correa et al., 2012; Liu et al., 2013). Deliberate inhalation of paint or glue by solvent abusers may lead to a high level of exposure to toluene and other chemicals (Dorsey, 2000). Toluene exposure values at newsstands and at home during the use of magazines adds to the total human health impact originally produced during production and retail stages (Walser et al., 2013). Exposure to these toxicants presents health effects at the organismal level that are due to direct or indirect impact on the DNA at the subcellular level (Mandani et al., 2013; Shaikh et al., 2018; Warden et al., 2018). Experiments conducted with earthworms and Drosophila melanogaster indicate a relationship between BTEX exposure and DNA damage/fragmentation (Liu et al., 2010; Singh et al., 2011). Researchers have also linked neurotoxicity and loss of reflexes in factory workers to exposure to certain concentrations of benzene (Mandiracioglu et al., 2011). Pumo et al. (2006) reported behavioral, motor, and cognitive changes in the neonates of pregnant animals acutely exposed to benzene. In rats, toluene, ethylbenzene and the three isomers of xylene caused reduced body weights at birth and developmental delay (Thiel and Chahoud, 1997; Saillenfait et al., 2003). There is also an association between benzene exposure and the presence of micronuclei as well as other forms of DNA damage (Kim et al., 2010; Bucker et al., 2012; Salem et al., 2018). Toluene exposure is linked to induction of repression of genes in pathways associated with synaptic plasticity and mitochondria function (Hester et al., 2011). Altered expression of genes associated with stress has been reported with benzene, toluene and xylene exposure in D. melanogaster (Singh et al., 2009, 2010). Benzene exposure in adult flies also resulted in upregulation of transporter proteins involved in the sequestration of the conjugation product of the phase II detoxification reaction (Sharma et al., 2018).
One of the major challenges to advancement in the field of toxicology is to be able to correlate variation at the molecular level with phenotypic variation in quantitative traits such as susceptibility to disease and resistance to toxins (Mackay et al., 2012; Mackay and Huang, 2018). Genome Wide Association Studies (GWAS) can serve as an effective means to pull putative information from Single Nucleotide Polymorphisms (SNPs) to further learn about certain genes and the influence they have on phenotypic expression (Weeger, 2018).
This study utilizes the exposure of a model organism, D. melanogaster, to assess effects on both animal development and acute toxicity (in adults) of BTEX environmental pollutants. D. melanogaster, also known as the fruit or vinegar fly, is a reliable model organism due to its rapid life cycle and wide array of genetic reagents that are available (Gilbert, 2008; Pandey and Nichols, 2011; Ruden et al., 2012). Adult D. melanogaster contain numerous organs that are functionally equivalent to mammalian organs, including a heart, a respiratory organ system (trachea), and a gut (Rothenfluh and Heberlein, 2002). This study also takes advantage of the Drosophila Genetic Resource Panel (DGRP), consisting of 200 wild-derived inbred fly strains. This collection is a powerful community resource for interrogating heritable, natural variation and linking complex traits to underlying genotypes (Huang et al., 2014). The panel presents an excellent genetic model system for quantitative genetic analyses of complex traits, and has resulted in the identification of genetic networks that underlie several stress responses, such as starvation resistance, chill coma recovery, startle behavior, oxidative stress sensitivity, radiation resistance, and exposure to alcohol (Morgan and Mackay, 2006; Jordan et al., 2012; Vaisnav et al., 2014; Weber et al., 2012). Our goal here was to determine the acute, genotoxic and developmental effect of BTEX compounds on D. melanogaster, and to identify genetic regions that may impact BTEX susceptibility.
Materials and Methods
Test Chemicals
The test chemicals benzene, toluene, ethylbenzene and xylene (p-xylene, m-xylene, and o-xylene) were supplied by VWR (us.vwr.com). Different concentrations of test chemicals were prepared using serial dilution in dimethyl sulfoxide (DMSO). Absolute ethanol ≥99.5% and glacial acetic acid (Millipore Sigma) were used in the preparation of grape juice agar.
Drosophila melanogaster Culturing
The D. melanogaster strains used in most experiments were white1118 (w1118) with the exception of the GWAS study, which used the DGRP strains. The flies were reared in fly vials (diameter 25 mm × 90 mm) at a constant temperature of 25 ± 1°C in darkness except when they were transferred to a fresh medium. The flies were raised under a standard D. melanogaster media using molasses with other recipes as prepared by Archon Scientific; Durham, NC, United States1.
Adult Acute Exposure Studies
w1118 adult flies, aged 0–3 days old, were maintained in standard D. melanogaster food. Ten flies were placed in each replicate vial containing D. melanogaster molasses-based food (n = 10 per trial, 2 trials total). The flies were treated at different concentrations obtained from range finding experiments of benzene (0, 0.11, 0.23, 0.34, 0.45, 0.56, and 1.13 mM), toluene (0, 0.11, 0.16, 0.24, 0.33, and 0.65 mM), p-xylene, m-xylene (0, 0.11, 0.16, 0.27, 0.32, and 0.65 mM), and 0.3% DMSO (Nazir et al., 2003). The BTEX compounds were mixed into the food which was melted using a microwave. The flies were exposed to the compounds for 4 days and the mortality of flies was recorded on each day during that time period. The flies were fed in new vials containing fresh concentrations of the test compounds daily. The experiment was conducted at 25°C, eliminating heat stress as a potential confounder.
Larval Development Studies
The developmental toxicity experiment was conducted using a grape juice gel agar recipe developed by Salvaterra et al. (2006) and was modified in this study using standardized proportions for the laboratory. A liter of grape juice agar was prepared using 250 ml 100% frozen grape juice, 18 g agar, 12 ml acetic acid, 750 ml distilled water, and 12 ml 95% ethanol.
A population of flies containing 20 females and 10 males collected under light CO2 anesthesia were placed in standard egg collection cages covered with grape juice agar petri-dishes (Durham et al., 2014). The grape juice gel agar was supplemented with a 0.1 ml of yeast paste which increases egg laying by the flies. The flies were left in the cages overnight to ensure enough eggs have been laid on the surface of the embryo gel agar. The adult flies are subsequently removed from their cages. The embryo gel plates containing the laid eggs were left for 24 h after which the first instar larvae (L1) hatched. Upon emergence of the first instar larva, a soft pointed brush was used to pick up 25 L1 larvae, which were transferred to grape juice agar petri-dishes containing varying concentrations of the test chemicals. The L1 larvae were left to develop into adults over a period of 2 weeks.
The doses of different concentrations of BTEX that were used are as follows: benzene- 0.226 mM, 0.451 mM, 0.903 mM, 1.806 Mm, and 2.226 mM; toluene- 0.189 mM, 0.337 mM, 0.754 mM, 1.509 mM, 1.886 mM; ethylbenzene- 0.163 mM, 0.326 mM, 0.651 mM,1.303 mM, 1.6 mM; xylene- 0.08 mM, 0.160 mM, 0.320 mM, 0.640 mM, 1.290 mM, and 1.600 mM. These compounds were quickly added to 5 ml of grape juice agar and immediately covered. This is particularly important because the actual concentration of BTEX is expected to decrease as a function of time due to their volatile nature. Grape juice agar with and without DMSO added were used as control for this experiment. The experimental design made use of DMSO as the solvent.
Newly eclosed flies were counted on the 14th day through the 16th day at 25°C, after hatching, at which point it is presumed that all flies would have eclosed into adults under control conditions (Montgomery et al., 2014). Survival of flies in each vial group was recorded against concentration. Survival was defined as the ability of flies to eclose as adults before 16 days post-exposure to BTEX.
Initially for the DGRP lines, seven lines were randomly selected (RAL-832, RAL-38, RAL-41, RAL-882, RAL-509, RAL-859, and RAL-181) to test the suitability of the BTEX-assay for the entire panel of 200 lines. The methodology for harvesting L1 larvae in flies raised in cages as described above was adopted for all of the DGRP lines. From each of these lines a total of 75 L1 larvae were selected and raised in embryo gel plates containing a fixed concentration of p-xylene (1.068 mM) which is the LC50 value and were left to develop into adults over a period of 2 weeks. Only p-xylene was selected for the GWAS study because it resulted in the most reliable dose-response relationship in exposed flies. Due to the large number of animals utilized during the experiment, the assay was designed in batches and in triplicates of 25 L1 larvae per vial. To rule out batch effects in the panel, one strain (RAL-38) was repeated in all the batches.
Immunohistochemistry
Effect of Benzene and p-Xylene Exposure on Cell Viability and Mitosis in Imaginal Disk Cells
We examined apoptosis and mitosis during wing imaginal disk development using Dcp-1 and Phospho-Histone H3 antibody staining, respectively. The cellular effect of benzene a known mutagen and p-xylene which produced the most amenable dose- response data were evaluated. Flies were fed with 1.068 mM each of p-xylene and benzene, for 3 days followed by the dissection of 3rd instar larva to harvest the wing disks. In controls, the larvae were fed with 0.1% DMSO (Nazir et al., 2003) in distilled water. Immunohistochemistry of third instar larval imaginal disks was done according to the methods described by Roberts (1998) and Sarkissian et al. (2014). Mouse anti-Death caspase-1- (Dcp-1) and Rabbit anti- Phospho-Histone H3 (PH3) were the primary antibodies (1 μL of primary antibody in 500 μL of block buffer). Fixation and staining were as in Stormo and Fox (2016). Images were obtained using a ZEISS ApoTome.2 fluorescent microscope by ZEISS Research Microscopy Solutions and imaging data was collected using the associated Zen 2 Pro software.
Statistical Analysis
Statistical analyses for the results were obtained using SPSS 20.0 software. To be able to determine the statistical significance of the results, the data was analyzed by One-way ANOVA and the Tukey’s Honest Significant Difference (HSD) was used in the Post hoc. The differences between groups were considered significant at p > 0.05. The resulting data from the analysis were expressed as mean ± standard error of the larval emergence. Lethal concentration of the test chemicals were arrived at using Regression Probit Transformed Responses.
Genome Wide Association Study (GWAS)
To identify candidate SNPs that contribute to variation in animal phenotypes, we submitted the least squares line means of the trait (eclosion of flies 16 days post exposure to 1.068 mM of p-xylene) to the DGRP analysis pipeline2, GWA was completed on 144 of the 200 lines assayed, based on the sequence data available within the pipeline at the time of analysis. The DGRP Freeze 2 Release 5.46 GWA analysis uses simple linear model ANOVAs on approximately 2.49 million SNPs using the model Y = μ + M + g + ε, where Y is the line means adjusted for Wolbachia pipientis infection and five major inversion polymorphisms (In(2L)t, In(2R)NS, In(3R)Y, In(3R)P, and In(3R)Mo) in the DGRP, μ is the overall population mean, M is the effect of DNA (the effect of the SNP) variant being tested, and g is a polygenic component with covariance between lines determined by their genomic relationship and ε is the error variance (Huang et al., 2014).
In addition, we performed GWA analysis for survival with p-xylene, calculated as the difference of line means between flies that were reared on control medium and those that were reared on p-xylene supplemented medium, using the same pipeline. We report the top associations with P < 10–5, based on quantile-quantile plots, which showed deviations of observed p-values from expected values at this threshold. The polymorphism identified at the threshold of P < 10–5 could possibly contain false positives, but has come to be recognized as a standard in DGRP studies. Pairwise linkage disequilibrium was assessed between polymorphic variants using the r2 parameterization (Huang et al., 2014) to help evaluate to what extent clustered SNPs segregate independently. We categorized the lines that had fewer eclosion on p-xylene medium “poor performer” and the rest into “good performer.”
Furthermore, the DGRP output provided information on site class for each SNP: SNPs more than 5,000 base pairs from any known gene were identified as such; SNPs in coding regions were identified as synonymous, non-synonymous, downstream, UTR-3-prime or intron SNP variants, as appropriate.
When a phenotype file is submitted on the DGRP2 pipeline, the phenotype is adjusted for the effects of W. pipientis infection and five major inversions (In(2L)t, In(2R)NS, In(3R)K, In(3R)P, and In(3R)Mo) (Mackay et al., 2012). Because this infection is known to affect various fitness traits in D. melanogaster (Fry et al., 2004), we tested the effect of the infection on p-xylene (1.068 mM) exposure. For W. pipientis infection adjustment we fit a linear model with the infection status and major inversion genotypes as covariates and the raw phenotypes as the response variable.
Results
Acute Toxicity of Adult Flies to BTEX
We first determined the LC50 (the concentration which resulted in 50% mortality) in adult D. melanogaster following 96 h of BTEX exposure (96 h LC50). We also extrapolated the LC5 and LC95 (the concentrations which resulted in 5% and 95% mortality, respectively). We determined this value by generating dose response curves for concentrations of each of the test chemicals (Supplementary Figure 1). The LC50 of benzene, p-xylene, Toluene, and m-xylene was 0.807 mM, 0.168 mM, 0.166 mM, and 0.288 mM, respectively. Thus, it can be inferred that toluene is the most toxic at 4.86, 1.73, and 1.01 times more toxic than benzene, m-xylene and p-xylene in w1118 flies, respectively (Table 1). Extrapolation of LC5, LC50, and LC95 value for ethylbenzene and o-xylene was not possible following 96 h exposure due to extreme mortality at all doses tested. These results highlight the impact of BTEX compounds on adult D. melanogaster survival.
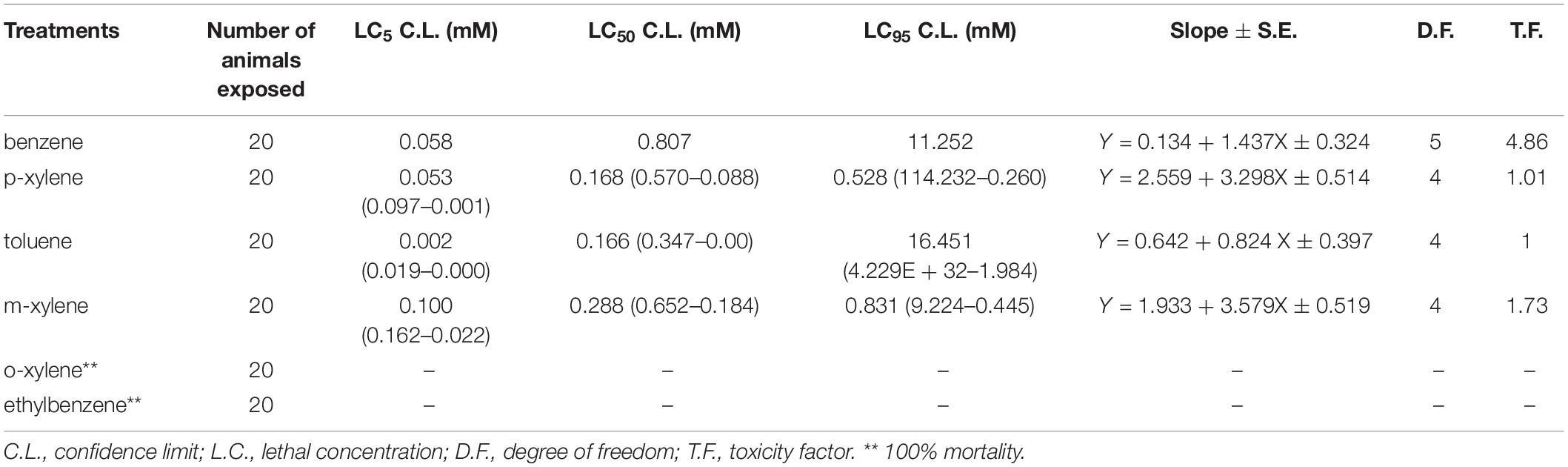
Table 1. Toxicity profile of benzene, toluene, ethylbenzene, and xylene in adult w1118 mutant Drosophila melanogaster in 4 days renewal exposure.
Developmental Progression Analysis in Animals Exposed to BTEX
We next examined the impact of BTEX compounds on developmental progression. We did so by examining the ability of newly hatched larvae to progress into adulthood in the presence of BTEX compounds. Animals fed DMSO + m-xylene, benzene, toluene, or ethylbenzene all exhibited some additional lethality relative to DMSO alone, though not in a dose-dependent manner (Table 2). However, we did observe consistent dose-dependent reductions (p < 0.05) in survival of animals fed p-xylene and o-xylene (Table 2). Overall, our results highlight an impact of BTEX compounds on animal development, with two xylene family compounds showing the most consistently detrimental effects.
P-Xylene Exposure Increase Both Apoptosis and Mitosis in Imaginal Disks
To examine the impact of BTEX compounds on animal development at a cellular resolution, we examined rates of cell death (apoptosis) and cell division. We first examined apoptosis using Dcp-1 antibody staining. After feeding with 1.068 mM of p-xylene for 3 days, the number of dying cells on the wing disk epithelia was significantly increased by a magnitude of 3-fold when compared to controls. Dcp-1 activity was increased in benzene-fed animals, but it was not significant (Figures 1A, 2). In controls, the larvae were fed with 0.1% DMSO. Thus, an effect of the acute ingestion of p-xylene is a large increase in cell death in the cells of the D. melanogaster wing disk epithelium. In contrast, apoptosis in the benzene-treated wing disks was only increased up to about 2 times of that in controls. These data indicate that p-xylene markedly increases the number of apoptotic cells in the wing disk of D. melanogaster and reflects the general toxicity of this compound during larval development.
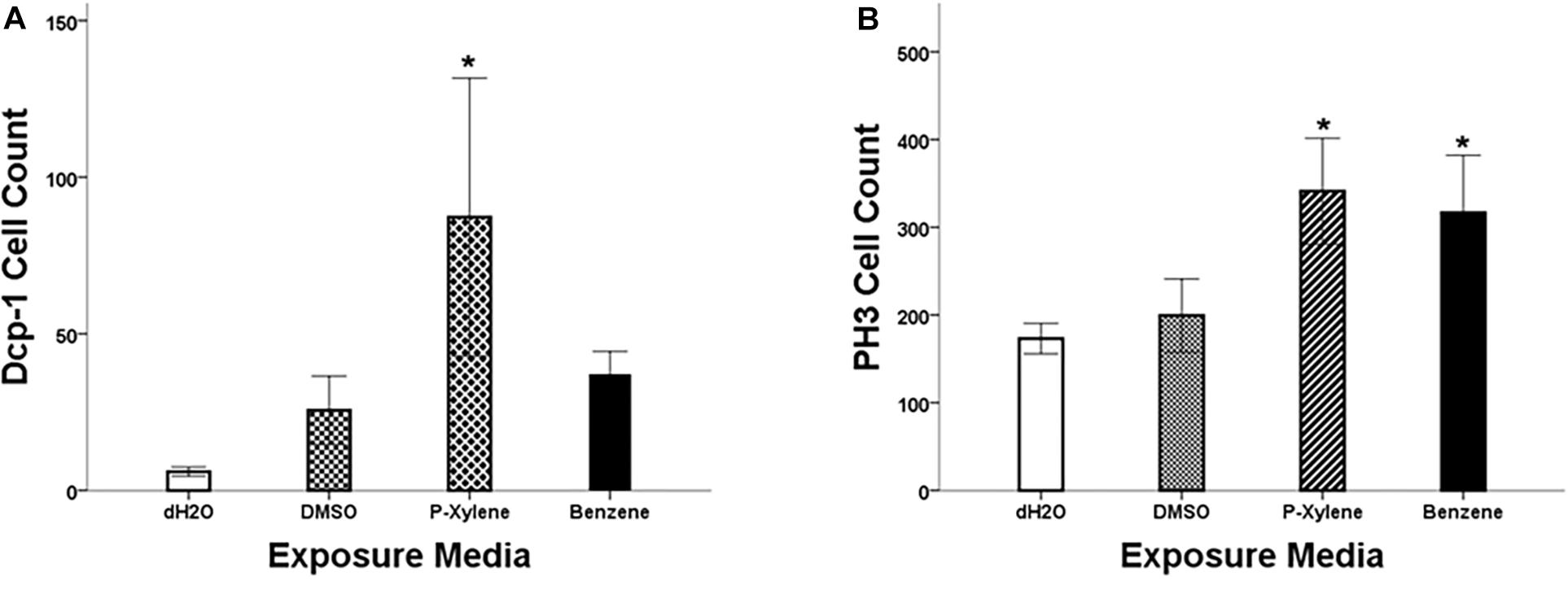
Figure 1. Quantitation of Dcp-1 and PH3 staining in wing imaginal disks following p-xylene and benzene feeding. (A) Summary of data showing activity of cleaved Dcp-1 in wing disks of w1118 3rd instar larva. Data are represented by mean antibody-positive cell count ± SE (N = 8). (B): Summary of data showing activity of PH3 in wing disks of w1118 3rd instar larva. Data are represented by mean cell count ± SE (N = 6). Statistical significance, treated versus control group: * (p < 0.05).
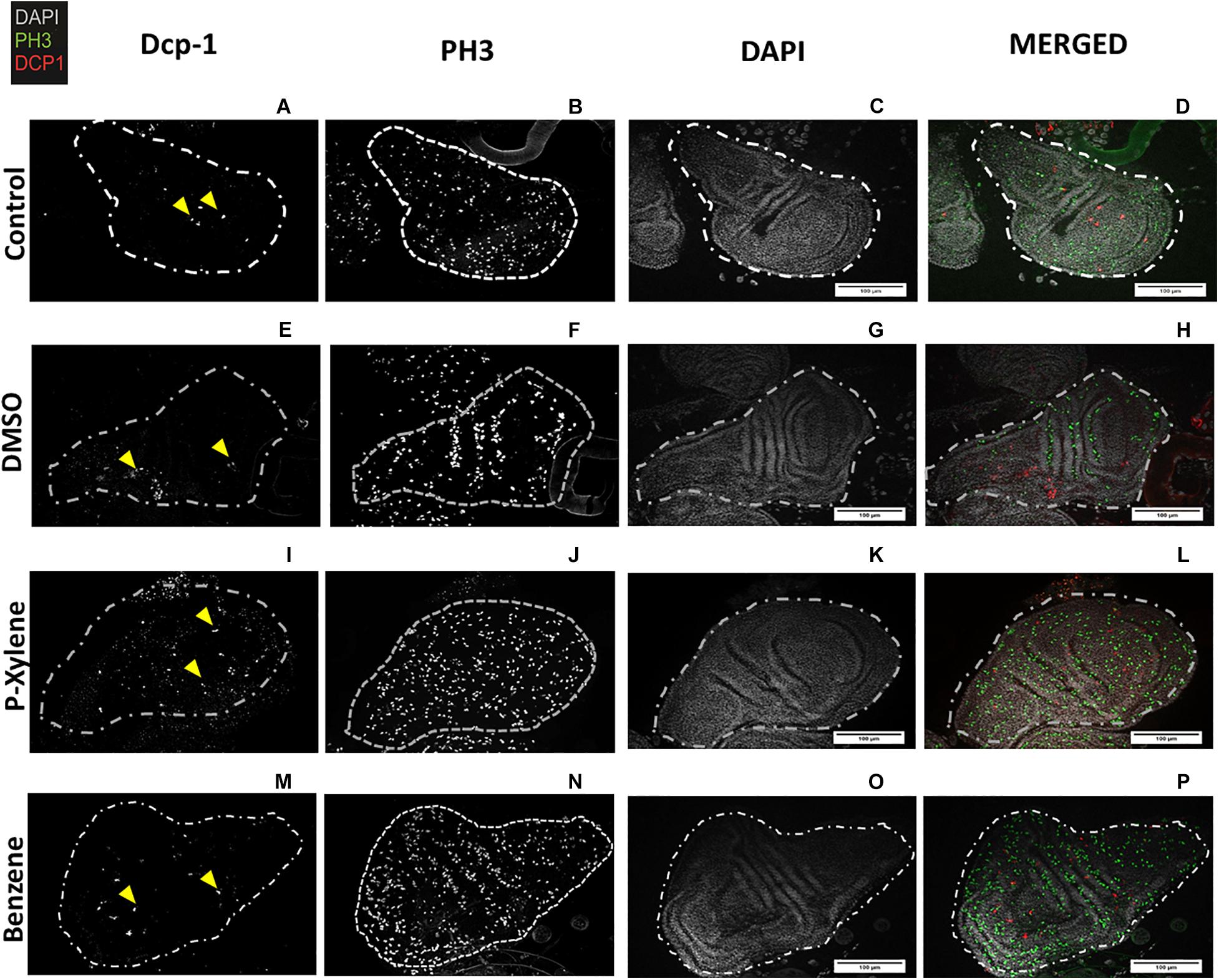
Figure 2. Visualization of Dcp-1 and PH3 staining in wing imaginal disks following p-xylene and benzene feeding. Wing disks of 3rd instar larvae immunostained for cleaved Dcp-1 (red), PH3 (green), and DAPI to visualize DNA (white). (A–D). Representative images of cell division and apoptosis in wing disk of control flies. First instar larvae were treated with 4 μL of deionized water for 72 h as described in “Materials and Methods” section. (E–H) Representative images of cell death and apoptosis in DMSO treatment. First instar larvae were treated with 0.1% of DMSO for 72 h as described in “Materials and Methods” section. (I–L) Representative images of cell division and apoptosis in p-xylene. First instar larvae were treated with 1.068 mM of p-xylene for 72 h as described in “Materials and Methods” section. (M–P) First instar larvae were treated with 1.068 mM of benzene for 72 h as described in “Materials and Methods” section. Dashed lines indicate shape of Drosophila melanogaster wing disk. Scale bar = 100 μm.
To determine if apoptosis caused by benzene and p-xylene treatment can result in signaling of the surrounding cells of dying epithelial cells to initiate tissue regeneration processes (Fox et al., 2020), wing imaginal disks were stained with an antibody against phospho-histone H3 (PH3), a specific marker of mitotic cells. In wing disks that were treated with 1.068 mM of p-xylene, there was a 2-fold increase in the number of the PH3-positive cells (Figures 1B, 2) which was significantly higher than those in control and DMSO. Similarly, animals that were fed with 1.068 mM of benzene for 3 days exhibited a significant 2-fold increase in the number of the PH3-positive cells in comparison with those in control and DMSO (Figures 1, 2). Together, these results suggest that exposure to p-xylene, and potentially benzene, kills D. melanogaster wing disk epithelial cells and stimulates compensatory cell division as part of an injury response.
GWAS Screen for BTEX Susceptibility
Given our findings that D. melanogaster is a convenient model system to study the impact of BTEX compounds, we next leveraged the ability of this system to reveal genetic regulation of BTEX responses. We used the DGRP collection, a sequenced and inbred library of lines used in GWAS studies (Mackay et al., 2012; Mackay and Huang, 2018). We determined the LC50 and also extrapolated the LC5 and LC95 using dose response curves generated from concentrations of the test compound. Randomly selected DGRP flies exposed to Benzene, m-xylene, ethylbenzene and toluene did not respond in a dose dependent manner and therefore the extrapolation of their LC50 is not predictive of the effect of the toxins against the flies. However, for p-xylene, we saw a reproducible dose dependent effect, and chose an LC50 of 1.068 mM (Table 3) p-xylene to further use in GWAS analysis, using the eclosion assay described in sections “Larval Development Studies” and “Developmental Progression Analysis in Animals Exposed to BTEX.”
To examine the suitability of the BTEX-assay for the DGRP lines and in order to arrive at a fixed dose for GWAS exposure, a panel of seven DGRP lines were selected (RAL-832, RAL-38, RAL-41, RAL-882, RAL-509, RAL-859, and RAL-181) at random. First instar larva were selected from these DGRP lines and allowed to feed on p-xylene treated plates for 24 h. Eclosion was recorded in each of the different concentrations. Only p-xylene was toxic in a dose dependent manner and showed variation in toxicity with the animals. To characterize natural variation in p-xylene response in 200 DGRP lines, a fixed dose of 1.068 mM p-xylene was selected. The animals were screened for survival post-p-xylene exposure, and we found extensive phenotypic and genetic variation in p-xylene exposure response across the tested DGRP panel (Figure 3). It was found that the lines fell into two distinct groups: one group of 56 lines with no survivors at all in the control (i.e., RAL line + DMSO) and p-xylene (i.e., RAL line + p-xylene) exposure, another group of 144 lines with survivors. The 144 lines were further grouped into 10 lines that showed resistance to p-xylene exposure, while the remaining 134 ranged from mildly susceptible to highly susceptible to p-xylene treatment and thus are sensitive. While lines such as RAL-189, RAL-857, RAL- 28, RAL-531, and RAL-195 did exceptionally well on a fixed concentration of p-xylene, RAL-790, RAL-443, RAL-142, RAL-774, and RAL-340 rarely eclosed 2 weeks post exposure in the presence of a fixed concentration of the test compound. We define performance as the mean difference of eclosion between control and of p-xylene treated media. Results for most lines were reproducible, with the exception of five highly variable lines (RAL-357, RAL-812, RAL-85, RAL-843, and RAL-362). Our results show that, for p-xylene, we identified differences in susceptibility within the DGRP collection. Importantly, it was observed that RAL-38 did not move from susceptible to resistant throughout the period of the experiment, suggesting there were minimal batch effects.
Next, we performed a case-control GWA analysis of the phenotype using SNPs from the DGRP freeze 1 sequencing data in order to identify genes that contain alleles conferring differences that correlate with p-xylene exposure susceptibility (Mackay et al., 2012). A total of 1,886,036 SNPs met the quality control thresholds and they were tested for association with p-xylene susceptibility using a linear mixed model (Supplementary Tables 1A,B). Using the Mackay lab GWAS protocol (Mackay et al., 2012), we identified SNPs that were significantly correlated with increased or decreased p-xylene susceptibility. A total of 38 SNPs were associated with both increased and decreased p-xylene susceptibility at p < 10–5, among which 34 were at p > 10–6, three (3) at p < 10–5, and one (1) at p > 10–7 (Supplementary Table 2 and Figure 4). The majority of the 38 SNPs were common variants, having a minor allele frequency of ≥5%. According to genomic site class, of the 38 SNPs, 10 were intronic, 4 fell in coding regions (one non-synonymous, four synonymous), three (3) occurred in the 3′ UTR, and nineteen (19) were intergenic or downstream of a gene. The heatmap of the pairwise Linkage Disequilibrium (LD) measurement generated by the call for the 38 SNPs reveals that the rate of LD decay is substantially lower on the X chromosome when compared to the autosomes (Figure 5).
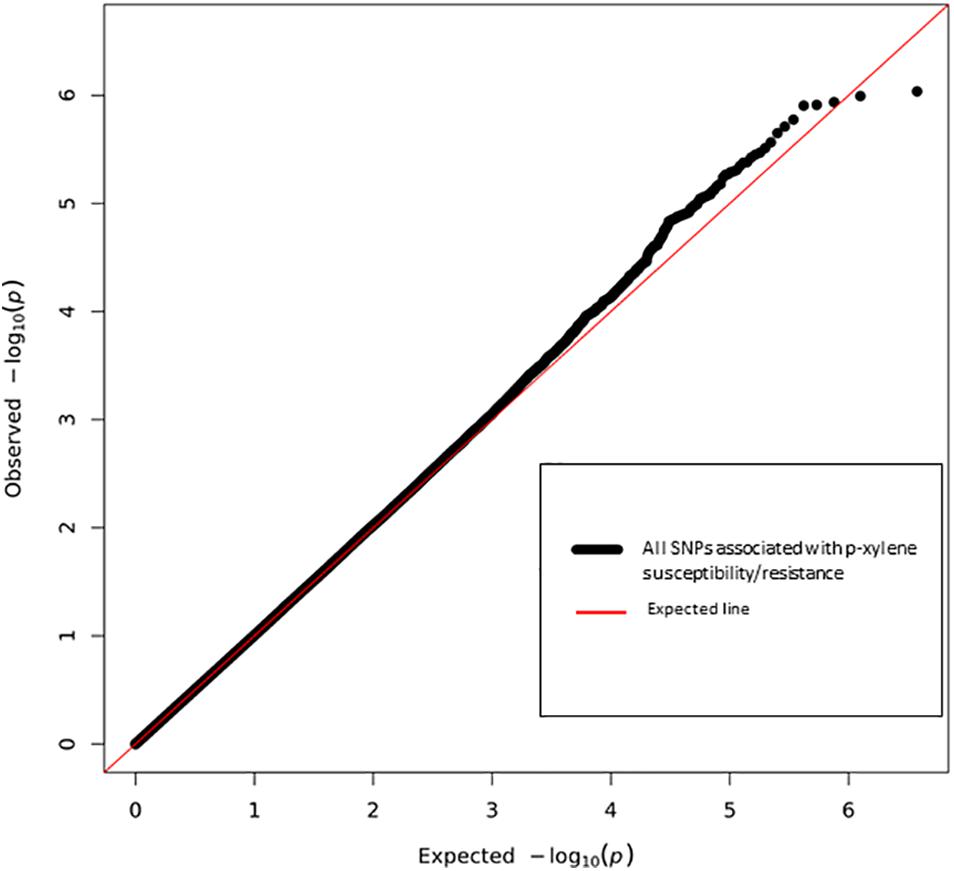
Figure 4. Quantile-Quantile plot of association analyses of p-xylene resistance and susceptibility among 144 DGRP lines. The red line indicates the expected and the black line the observed p values. Six top performing lines are highlighted.
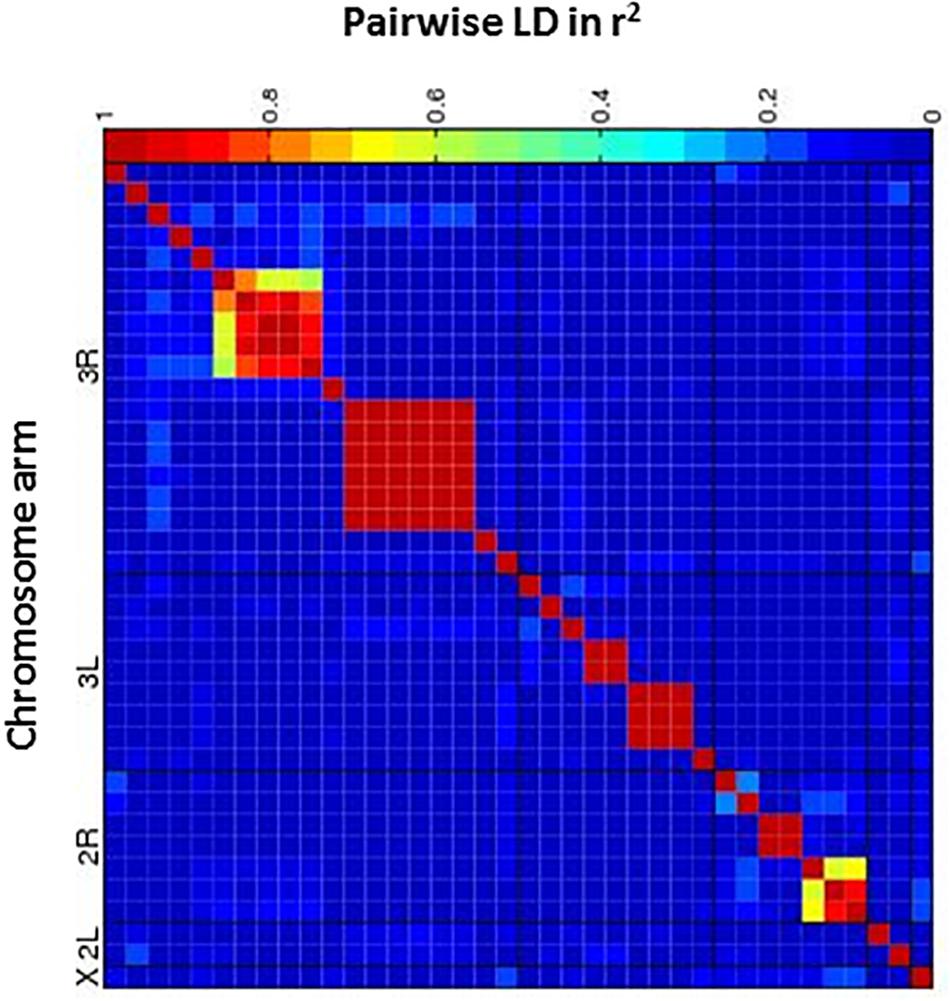
Figure 5. Heatmap of linkage disequilibrium (LD) values (R2) between candidate SNPs. The heat map depicts the degree of LD, r2, between variants. The five major chromosome arms are defined by the black lines. Red corresponds to complete LD and blue to absence of LD. A nominal P ≤ 10– 5 is indicated with a red line for each trait.
Excluding the intergenic regions, the remaining SNPs implicated 16 genes, 14 of which have human homologs. In particular, these data highlight the importance of chaff (cha) with respect to p-xylene susceptibility: a total of 5 polymorphisms (5 SNPs) were found in the first intron of this gene, and they are associated with natural variation in p-xylene in this analysis. chaff is a known maternal effect lethal and female sterile gene (Lindsley and Zimm, 1992). Besides the chaff gene with 5 hits, three other genes had multiple hits: toutatis (tou), which is primarily involved in transcription factor binding activities and regulation of chromatin structure (Fauvargue et al., 2001), had three SNPs located on the gene; defective proboscis extension response (dpr6) gene had two SNPs and it is known to form complexes that specify synaptic connections between neurons and target cells (Carrillo et al., 2015); phosphoglucomutase 2b (CG10202) had two SNPs and it is a protein coding gene that is predicted to be involved in carbohydrate metabolic process. Overall, we mapped SNPs in sixteen (16) genes in the GWA analyses for exposure to p-xylene using a significant criterion of p < 10–5. The genes are mdh2, pnt, tweek, CG13532, CG33970, CG10202, Irk1, DmsR-1, Meltrin, CG32112, Nckx30C, tou, dpr6, Dys, CR43451, and cha. All but two of the genes namely CR43451 and CG13532 have human homologs (Supplementary Table 3). We note that the cha and Dys genes are involved in biosynthesis of the neurotransmitter acetylcholine and the modulation of chemical synaptic transmission, respectively. While many of the candidate genes have primary functions and activities to which they are associated, for CG13532, CG32112, and CR43451, the molecular functions and the biological processes in which they are involved are not known, suggesting our analysis may reveal novel gene functions. All genes found in our screen are novel candidates in p-xylene susceptibility and resistance (Supplementary Table 3).
Discussion
In this study, our results show that, despite the volatility of most BTEX compounds, both larval and adult stage D. melanogaster can be used to study effects on developmental and acute toxicity. Our results reveal compound and dose-dependent effects on both adult animal survival and progression through larval and pupal development. Further, we find effects at the cellular level on both apoptosis and mitosis, and reveal genome regions that regulate BTEX susceptibility.
Toluene and p-xylene were extremely toxic to adult D. melanogaster in the adult toxicity assay. The data revealed an order of toxicity of toluene ≥ p-xylene > m-xylene > benzene. It must be noted that in all exposed groups, the toxicity of the BTEX compounds followed a dose-dependent response, except in o-xylene and ethylbenzene, where there was no clear response pattern for mortality. It is possible that toluene and p-xylene might be metabolized more slowly and thus may require a more prolonged exposure as compared to a rapidly metabolizing benzene and m-xylene (Avramov et al., 2013). On the other hand, it is also possible that the duration of exposure to the test chemicals could have favored the toxicity of toluene and p-xylene (Niaz et al., 2015). Toluene also promotes differential gene expression in D. melanogaster as described by Moskalev et al. (2014).
The acute effect of BTEX compounds in different organisms is well documented in the literature (Pumo et al., 2006; Gao et al., 2014; Camara-Lemarroy et al., 2015). In African earthworms (Eudrilus eugeniae), 96 h LC50 values for exposure to xylene, toluene, ethylbenzene and benzene were 0.011 mmol/kg, 0.014 mmol/kg, 0.013 mmol/kg, and 0.024 mmol/kg, respectively (Doherty et al., 2019). In Asian earthworms (Perionyx excavatus), toluene was acutely toxic with LC50 value of 0.5 μmol/cm2 after a 48 h exposure (An and Lee, 2008).
Results from our exposure of first instar larvae to benzene, ethylbenzene, o-, m-, p- xylene and toluene reveals that lower concentrations of the test substance has a milder effect on animal development when compared with the higher concentrations that resulted in developmental delays beyond 16 days post exposure. This effect was more significant in o-, m-, and p- xylene. It is possible that these toxicants act in a different way, other than direct effect on survival, to cause developmental delay in the exposed animals. Although the mechanism of the developmental toxicity of technical xylene components is unknown, it is possible that modulation of their metabolism may affect their toxicities. The response observed may be a result of environmental and genetic factors rather than from the toxicant themselves. Congruent to this study, a previous inhalation study in rats found that the three isomers of xylene produced developmental toxicity at concentrations between 500 and 2000 ppm (Ungvary et al., 1980; Saillenfait et al., 2003). In D. melanogaster in particular, exposure to benzene, xylene and toluene induced a delay in the number of flies that emerged into adult (Singh et al., 2009). In Xenopus laevis, treatment of tadpoles with p-xylene resulted in a significantly higher mortality, malformed tadpoles and developmental delay, in embryonic toxicity studies (Gao et al., 2016). In humans, there is also a relationship between high concentration of BTEX and neural tube defects in pregnant women (Lupo et al., 2011).
In addition to the developmental defects we observed at an organismal level, we also observed BTEX associated death (apoptosis) at the cellular level. Apoptosis is dependent on activation of caspases and is triggered during embryogenesis and normal tissue homeostasis in response to certain physiological changes. These changes are initiated by the activation of specific pathways, followed by changes in morphology such as nuclear and cytoplasmic condensation, cell shrinkage, increase or decrease in cellular ion concentration, DNA fragmentation and the release of a cellular component known as apoptotic bodies (Ai et al., 2011; Singh et al., 2011; Favaloro et al., 2012). Our study shows that the number of apoptotic cells was significantly increased in wing disks exposed to p-xylene compared to control media. Benzene, also induced an increase in apoptosis, but our results suggest it does not produce a significant effect on the number of dying cells, though we did observe an increased rate of mitosis in these animals. It is well documented that Reactive Oxygen Species (ROS) are generated during the metabolism of benzene and xylene (Singh et al., 2009) and availability of excessive free radicals is a culprit in cellular damage (Sarma et al., 2011). It has recently been found that xylene toxicity in human lymphocytes is stimulated through the generation of ROS (Salimi et al., 2017). These highly reactive radicals that are generated by benzene and p-xylene may have triggered a series of protein-protein interaction that then leads to an increase in membrane permeability of the cells. The ROS generated-effects may have moved from one cell to another through apoptotic signaling and thereby propagating there production in surrounding cells (Santabarbara-Ruiz et al., 2015). The results obtained could also be as a result of the induction of caspase-dependent cell death pathways mediated by mitochondria (Singh et al., 2011).
We observed increased cell division in developing tissues exposed to some BTEX compounds. Cell proliferation is a known mechanism by which a number of tissues in D. melanogaster respond to death of cells (Sun and Irvine, 2011; Yang et al., 2014). Administration of benzene in cultured porcine ovarian granulosa cells stimulates cell proliferation (Tarko et al., 2019). Similar to this study, benzene was confirmed to have the ability to induce chromosomal loss in wing primordial cells of D. melanogaster (Soós and Szabad, 2014). The increase in cell proliferation could be attributed to the activation of a cell death induced pathway that is required for regenerative growth in the wing disks of D. melanogaster in response to the increase in the number of dying cells (Bergantiños et al., 2010).
Our findings also represent a large-scale effort to identify genome wide associations that impact p-xylene exposure. One of the advantages of the DGRP collection is that it relies on freely occurring genetic variation. All strains in the DGRP panel were caught in the wild and inbred over twenty generations until isogenic (Mackay et al., 2012). This provides us with an opportunity to assess the magnitude of genotype by natural variation and their genetic basis, since complex behavior varies from individual to individual. Multiple studies have already utilized GWAS to generate novel interactors in complex traits such as aggression, stress response, and longevity, studies that were made possible by the intensive sequencing of all lines included in the DGRP (Shorter et al., 2015; Durham et al., 2014; Weber et al., 2012). It was then natural to leverage this tool to attempt a similar process in the field of toxicology. This type of study has been demonstrated with some range of toxicants including methylmercury, (Montgomery et al., 2014), radiation exposure (Vaisnav et al., 2014) and lead toxicity (Zhou et al., 2016).
This study identified a list of candidate genes that might play a role in modifying the phenotypic effect of p-xylene exposure. With the exception of two genes (CR43451 and CG13532), all the top candidate genes have human orthologs (14/16) (Supplementary Table 3). This high level of conservation suggests that the findings in this study could be highly informative as human candidates in translational studies. Our p-xylene GWA analysis reveals candidate genes that were enriched for functions in nervous system development, neuromuscular synaptic transmission, membrane signaling factors, carbohydrate metabolism, imaginal wing disk development, transcription factor binding sites (TFBS) and apoptotic processes (Supplementary Table 3). The representation of these candidate genes shows that 3 of the top 16 candidates have human homologs that are linked to increased risk of certain human diseases such as Alzheimer’s disease, asthma, and chronic obstructive pulmonary disease (Meyer et al., 2010; Dey and Ray, 2018). There may be common pathways or mechanisms shared between these human diseases and exposure to p-xylene. There may well be an interaction between apoptotic genes and those responsible for development of the imaginal wing disks, these could explain the increase apoptosis and mitosis observed with p-xylene exposure in D. melanogaster.
Among the genes represented by the significant SNP hits, four contain multiple SNPs (cha, tou, dpr6, and CG10202). This is in concordance with Moskalev et al. (2014) whose study demonstrated that cha was downregulated in toluene treated flies. Future studies will be required to ascertain if truly the biological selectivity of these genes is representative enough or they are simply in linkage disequilibrium with causative variants found in other loci.
Two SNPs (3L_10034780_SNP and 3L_10034781_SNP) both downstream, mapped to the gene dpr6, are significantly associated with p-xylene resistance (p = 1.94 × 10–6 and 2.24 × 10–6). This result is particularly very interesting because a different GWAS study had mapped a SNP to the gene Dpr6 that was associated with variation in Providencia rettgeri load in flies reared on different glucose diet (Unckless et al., 2015). Dpr6 belongs to a family of genes thought to be involved in synapse organization and that localizes to the neuron projection membrane, including gustatory perception of food, and contains an immunoglobulin domain that may be involved in cell-cell recognition. The gene is expressed in the medulla and ventral nerve cord (Nakamura et al., 2002; Carrillo et al., 2015). This genetic correlation could mean that resistance to p-xylene is linked in some way to the metabolic uptake and conversion of glucose, but a full characterization of this mechanism will require future study.
The cha gene is responsible for the synthesis of the neurotransmitter acetylcholine (ACh) and this is typical of the cholinergic neurons present in the peripheral and central nervous system. In a reversible reaction cha catalyzes synthesis of ACh from acetyl-CoA and choline, ACh then stimulates muscle contraction in the central nervous system and learning in the central nervous system (Cai et al., 2004). Due to the high number of SNPs that were mapped to this gene relative to all other top annotations, p-xylene could have a major modulatory effect on the regulation of the cha gene and by extension a defect in synaptic transmission. This was demonstrated in zebrafish where a missense mutation in the homolog of the cha gene chata shows a strong reduction in embryo motility (Joshi et al., 2018).
Although it has been reported that xylene can induce DNA damage and candidate gene studies have linked xylene with chronic myeloid leukemia and cancer (Lim et al., 2016), none of our top 16 candidate genes from the 38 significant hits are associated with DNA damage. This could be due to the fact that the mode of toxic action of p-xylene in in vitro and in whole animal conditions is quite different from what is observed at the genome-wide level studies.
In summary, our study reveals 38 SNPs associated with p-xylene resistance and susceptibility in D. melanogaster developmental and toxicity experiments involving benzene, toluene, ethylbenzene, p-xylene, m-xylene, and o-xylene. The study also suggests that benzene and p-xylene are capable of eliciting apoptotic and cell proliferative responses in imaginal wing disk of D. melanogaster. The GWA analyses in this study has demonstrated the strength of the DGRP in revealing a highly polygenic genetic architecture that underlies variation in susceptibility to p-xylene toxicity, which may give rise to subtle variations in neuromuscular synaptic transmission during early development. The study further reveals genes 15 genes, some of which are associated with p-xylene exposure and whose human homologs have been linked with increased risk of certain human diseases. Future functional studies involving p-xylene exposure should consider looking at the absence or presence of these genetic variants using existing mutant or RNAi strains. Such studies could lead to future work involving critical gene expression or proteomic responses to BTEX compounds. Additionally, our findings here can serve as a guide for future population-based studies in humans.
Data Availability Statement
The datasets presented in this study can be found in online repositories. The names of the repository/repositories and accession number(s) can be found in the article/ Supplementary Material.
Author Contributions
DF, AO, and TA contributed to the conception and design of the study. TA performed the experiments, data analysis, and wrote the first draft of the manuscript. All authors contributed to manuscript revision, read, and approved the submitted version.
Funding
This work was supported by the Petroleum Technology Development Fund (PTDF) Scholarship grant, Abuja, Nigeria, and funds from Duke University to DF.
Conflict of Interest
The authors declare that the research was conducted in the absence of any commercial or financial relationships that could be construed as a potential conflict of interest.
Acknowledgments
We would like to thank Delisa Clay and Scott Allen for their input and helpful advice. We would also like to acknowledge the key role played by the Society of Toxicology in connecting the lead author with Fox Lab at Duke University, Durham.
Supplementary Material
The Supplementary Material for this article can be found online at: https://www.frontiersin.org/articles/10.3389/fgene.2020.594179/full#supplementary-material
Footnotes
References
Ai, X., Butts, B., Vora, K., Li, W., Tache-Talmadge, C., Fridman, A., et al. (2011). Generation and characterization of antibodies specific for caspase-cleaved neo-epitopes: a novel approach. Cell Death Dis. 2:e205. doi: 10.1038/cddis.2011.91
An, Y. J., and Lee, W. M. (2008). Comparative and combined toxicities of toluene and methyl tert-butyl ether to an Asian earthworm Perionyx excavatus. Chemosphere 71, 407–411. doi: 10.1016/j.chemosphere.2007.11.010
Avramov, M., Schmidt, S. I., and Griebler, C. (2013). A new bioassay for the ecotoxicological testing of VOCs on groundwater invertebrates and the effects of toluene on Niphargus inopinatus. Aquat Toxicol. 130, 1–8. doi: 10.1016/j.aquatox.2012.12.023
Bergantiños, C., Corominas, M., and Serras, F. (2010). Cell death-induced regeneration in wing imaginal discs requires JNK signalling. Development 137, 1169–1179. doi: 10.1242/dev.045559
Bucker, A., Carvalho, M., Conceição, M., and Alves-Gomes, J. (2012). Micronucleus test and comet assay in erythrocytes of the Amazonian electric fish Apteronotus bonapartii exposed to benzene. Ecotoxicol. Environ. Contam. 7, 65–73. doi: 10.5132/jbse.2012.01.010
Cai, Y., Cronin, C. N., Engel, A. G., Ohno, K., Hersh, L. B., and Rodgers, D. W. (2004). Choline acetyltransferase structure reveals distribution of mutations that cause motor disorders. EMBO J. 23, 2047–2058. doi: 10.1038/sj.emboj.7600221
Camara-Lemarroy, C. R., Rodríguez-Gutiérrez, R., Monreal-Robles, R., and González-González, J. G. (2015). Acute toluene intoxication–clinical presentation, management and prognosis: a prospective observational study. BMC Emerg Med. 15:19. doi: 10.1186/s12873-015-0039-0
Carrillo, R. A., Özkan, E., Menon, K. P., Nagarkar-Jaiswal, S., Lee, P. T., Jeon, M., et al. (2015). Control of synaptic connectivity by a network of Drosophila IgSF cell surface proteins. Cell 163, 1770–1782. doi: 10.1016/j.cell.2015.11.022
Cheng, J., Zhang, Y., Wang, T., Xu, H., Norris, P., and Pan, W. P. (2018). Emission of volatile organic compounds (VOCs) during coal combustion at different heating rates. Fuel 225, 554–562. doi: 10.1016/j.fuel.2018.03.185
Ciarrocca, M., Tomei, G., Fiaschetti, M., Caciari, T., Cetica, C., Andreozzi, G., et al. (2012). Assessment of occupational exposure to benzene, toluene and xylenes in urban and rural female workers. Chemosphere 87, 813–819. doi: 10.1016/j.chemosphere.2012.01.008
Correa, S. M., Arbilla, G., Marques, M. R., and Oliveira, K. M. (2012). The impact of BTEX emissions from gas stations into the atmosphere. Atmospheric Pollut Res. 3, 163–169. doi: 10.5094/APR.2012.016
Dey, S., and Ray, K. (2018). Cholinergic activity is essential for maintaining the anterograde transport of Choline Acetyltransferase in Drosophila. Sci. Rep. 8, 1–11. doi: 10.1038/s41598-018-26176-z
Doherty, F. V., Aneyo, I., and Otitoloju, A. A. (2019). Histopathological and biochemical alterations in Eudrilus eugeniae (Kinberg 1867) as biomarkers of exposure to monocyclic aromatic hydrocarbons in oil impacted site. J. Basic Appl. Zool. 80:63. doi: 10.1186/s41936-019-0130-2
Dorsey, A. (2000). Toxicological Profile for Toluene. Washington, DC: U.S. Department of Health and Human Services.
Durham, M. F., Magwire, M. M., Stone, E. A., and Leips, J. (2014). Genome-wide analysis in Drosophila reveals age-specific effects of SNPs on fitness traits. Nat. Commun. 5:4338. doi: 10.1038/ncomms5338
Ebegbulem, J. C., Ekpe, D., and Adejumo, T. O. (2013). Oil exploration and poverty in the Niger delta region of Nigeria: a critical analysis. Int. J. Bus. Soc. Sci. 4, 279–287.
Esteve-Turrillas, F. A., Pastor, A., and De La Guardia, M. (2007). Assessing air quality inside vehicles and at filling stations by monitoring benzene, toluene, ethylbenzene and xylenes with the use of semipermeable devices. Anal. Chim. Acta 593, 108–116. doi: 10.1016/j.aca.2007.04.055
Fauvargue, M. O., Laurenti, P., Boivin, S., Griffin-Shea, R., Bourbon, H. M., and Dura, J. M. (2001). Dominant modifiers of the polyhomeotic extra-sex-combs phenotype induced by marked P element insertional mutagenesis in Drosophila. Genet Res. 78, 137–148. doi: 10.1017/S0016672301005274
Favaloro, B., Allocati, N., Graziano, V., Di Ilio, C., and De Laurenzi, V. (2012). Role of apoptosis in disease. Aging (Albany N Y) 4:330. doi: 10.18632/aging.100459
Fox, D. T., Cohen, E., and Smith-Bolton, R. (2020). Model systems for regeneration: Drosophila. Development 147, 173781. doi: 10.1242/dev.173781
Friedrich, R., and Obermeier, A. (1999). “Anthropogenic emissions of volatile organic compounds,” in Reactive Hydrocarbons in the Atmosphere, ed. N. Hewitt (San Diego, CA: Academic Press), 1–39. doi: 10.1016/B978-012346240-4/50002-3
Fry, A. J., Palmer, M. R., and Rand, D. M. (2004). Variable fitness effects of Wolbachia infection in Drosophila melanogaster. Heredity 93:379. doi: 10.1038/sj.hdy.6800514
Gao, J., Ruan, H., Qi, X., Guo, X., Zheng, J., Liu, C., et al. (2016). Increased apoptosis and abnormal visual behavior by histone modifications with exposure to para-xylene in developing Xenopus. Neuroscience 331, 177–185. doi: 10.1016/j.neuroscience.2016.06.027
Gao, Y., Zhang, Y., Kamijima, M., Sakai, K., Khalequzzaman, M., Nakajima, T., et al. (2014). Quantitative assessments of indoor air pollution and the risk of childhood acute leukemia in Shanghai. Environ. Pollut. 187, 81–89. doi: 10.1016/j.envpol.2013.12.029
Gilbert, L. I. (2008). Drosophila is an inclusive model for human diseases, growth and development. Mol. Cell. Endocrinol. 293, 25–31. doi: 10.1016/j.mce.2008.02.009
Hazrati, S., Rostami, R., Fazlzadeh, M., and Pourfarzi, F. (2016). Benzene, toluene, ethylbenzene and xylene concentrations in atmospheric ambient air of gasoline and CNG refueling stations. Air Quality Atmosp Health 9, 403–409. doi: 10.1007/s11869-015-0349-0
Hester, S. D., Johnstone, A. F., Boyes, W. K., Bushnell, P. J., and Shafer, T. J. (2011). Acute toluene exposure alters expression of genes in the central nervous system associated with synaptic structure and function. Neurotoxicol. Teratol. 33, 521–529. doi: 10.1016/j.ntt.2011.07.008
HHS (2007). Public Health Service, Agency for Toxic Substances and Disease Registry (ATSDR). Toxicological Profile for Xylene. Washington, DC: HHS (United States Department of Health and Human Services).
Huang, W., Massouras, A., Inoue, Y., Peiffer, J., Ràmia, M., Tarone, A. M., et al. (2014). Natural variation in genome architecture among 205 Drosophila melanogaster genetic reference panel lines. Genome Res. 24, 1193–1208. doi: 10.1101/gr.171546.113
Ite, A. E., Ibok, U. J., Ite, M. U., and Petters, S. W. (2013). Petroleum exploration and production: past and present environmental issues in the Nigeria’s Niger Delta. Am. J. Environ. Protect. 1, 78–90. doi: 10.12691/env-1-4-2
Jordan, K. W., Craver, K. L., Magwire, M. M., Cubilla, C. E., Mackay, T. F., and Anholt, R. R. (2012). Genome-wide association for sensitivity to chronic oxidative stress in Drosophila melanogaster. PLoS One 7:e38722. doi: 10.1371/journal.pone.0038722
Joshi, S., Virdi, S., Etard, C., Geisler, R., and Strähle, U. (2018). Mutation of a serine near the catalytic site of the choline acetyltransferase a gene almost completely abolishes motility of the zebrafish embryo. PLoS One 13:e0207747. doi: 10.1371/journal.pone.0207747
Kandyala, R., Raghavendra, S. P. C., and Rajasekharan, S. T. (2010). Xylene: an overview of its health hazards and preventive measures. J. Oral Maxillof Pathol. JOMFP 14:1. doi: 10.4103/0973-029X.64299
Kim, Y. J., Choi, J. Y., Cho, Y. H., Woo, H. D., and Chung, H. W. (2010). Micronucleus-centromere assay in workers occupationally exposed to low level of benzene. Hum. Exp Toxicol. 29, 343–350. doi: 10.1177/0960327110361500
Lim, J. H., Song, M. K., Cho, Y., Kim, W., Han, S. O., and Ryu, J. C. (2016). Expression of exosomal and cellular microRNAs: as biomarkers for toluene, ethylbenzene, xylene (TEX) exposure. Mol. Cell. Toxicol. 12, 359–369. doi: 10.1007/s13273-016-0040-z
Lindsley, D. L., and Zimm, G. G. (1992). The Genome of Drosophila Melanogaster. San Diego, CA: Academic Press, 1100.
Liu, Q., Liu, Y., and Zhang, M. (2013). Personal exposure and source characteristics of carbonyl compounds and BTEXs within homes in Beijing, China. Build. Environ. 61, 210–216. doi: 10.1016/j.buildenv.2012.12.014
Liu, Y., Zhou, Q., Xie, X., Lin, D., and Dong, L. (2010). Oxidative stress and DNA damage in the earthworm Eisenia fetida induced by toluene, ethylbenzene and xylene. Ecotoxicology 19, 1551–1559. doi: 10.1007/s10646-010-0540-x
Lupo, P. J., Symanski, E., Waller, D. K., Chan, W., Langlois, P. H., Canfield, M. A., et al. (2011). Maternal exposure to ambient levels of benzene and neural tube defects among offspring: Texas, 1999–2004. Environ. Health Perspect. 119, 397–402. doi: 10.1289/ehp.1002212
Mackay, T. F., and Huang, W. (2018). Charting the genotype–phenotype map: lessons from the Drosophila melanogaster genetic reference panel. Wiley Interdiscipl. Revi. 7:e289. doi: 10.1002/wdev.289
Mackay, T. F., Richards, S., Stone, E. A., Barbadilla, A., Ayroles, J. F., Zhu, D., et al. (2012). The Drosophila melanogaster genetic reference panel. Nature 482:173. doi: 10.1038/nature10811
Mandani, P., Desai, K., and Highland, H. (2013). Cytotoxic effects of benzene metabolites on human sperm function: an in vitro study. ISRN Toxicol. 2013:397524. doi: 10.1155/2013/397524
Mandiracioglu, A., Akgur, S., Kocabiyik, N., and Sener, U. (2011). Evaluation of neuropsychological symptoms and exposure to benzene, toluene and xylene among two different furniture worker groups in Izmir. Toxicol. Indust. Health 27, 802–809. doi: 10.1177/0748233711399309
McInnes, G. (1996). Joint EMEP/CORINAIR Atmospheric Emission Inventory Guidebook. Copenhagen: European Environmental Agency.
Meyer, H., Von Ohlen, T., Panz, M., and Paululat, A. (2010). The disintegrin and metalloprotease Meltrin from Drosophila forms oligomers via its protein binding domain and is regulated by the homeobox protein VND during embryonic development. Insect Biochem. Mol. Biol. 40, 814–823. doi: 10.1016/j.ibmb.2010.07.010
Montgomery, S. L., Vorojeikina, D., Huang, W., Mackay, T. F., Anholt, R. R., and Rand, M. D. (2014). Genome-wide association analysis of tolerance to methylmercury toxicity in Drosophila implicates myogenic and neuromuscular developmental pathways. PLoS One 9:e110375. doi: 10.1371/journal.pone.0110375
Moolla, R., Curtis, C., and Knight, J. (2015). Occupational exposure of diesel station workers to BTEX compounds at a bus depot. Int. J. Environ. Res. Public Health 12, 4101–4115. doi: 10.3390/ijerph120404101
Morgan, T. J., and Mackay, T. F. C. (2006). Quantitative trait loci for thermotolerance phenotypes in Drosophila melanogaster. Heredity 96:232. doi: 10.1038/sj.hdy.6800786
Mosaddegh Mehrjerdi, M. H., Tahmasebi, N., Barkhordari, FiroozAbadi, A., Fallahzadeh, H., Esmaielian, S., et al. (2014). The investigation of exposure to benzene, toluene, ethylbenzene and xylene (BTEX) with Solid Phase Microextraction Method in gas station in Yazd province. ISMJ 16, 419–427.
Moskalev, A., Shaposhnikov, M., Snezhkina, A., Kogan, V., Plyusnina, E., Peregudova, D., et al. (2014). Mining gene expression data for pollutants (dioxin, toluene, formaldehyde) and low dose of gamma-irradiation. PLoS One 9:e86051. doi: 10.1371/journal.pone.0086051
Nakamura, M., Baldwin, D., Hannaford, S., Palka, J., and Montell, C. (2002). Defective proboscis extension response (DPR), a member of the Ig superfamily required for the gustatory response to salt. J. Neurosci. 22, 3463–3472. doi: 10.1523/JNEUROSCI.22-09-03463.2002
Nazir, A., Mukhopadhyay, I., Saxena, D. K., and Chowdhuri, D. K. (2003). Evaluation of the no observed adverse effect level of solvent dimethyl sulfoxide in Drosophila melanogaster. Toxicol. Mechan. Methods 13, 147–152. doi: 10.1080/15376510309846
Niaz, K., Bahadar, H., Maqbool, F., and Abdollahi, M. (2015). A review of environmental and occupational exposure to xylene and its health concerns. EXCLI J. 14:1167. doi: 10.17179/excli2015-623
Nwilo, P. C., and Badejo, O. T. (2005). “Oil spill problems and management in the niger delta,” in Proceedings of the International Oil Spill Conference, Miami, FL, doi: 10.7901/2169-3358-2005-1-567
Ojiodu, C. C. (2013). Ambient volatile organic compounds (VOCs) pollution in isolo industrial area of lagos state, southwestern–nigeria. Ethiop. J. Environ. Stud. Manag. 6, 688–697. doi: 10.4314/ejesm.v6i6.12
Olumayede, E. G., and Okuo, J. M. (2012). Variation characteristics of volatile organic compounds in an urban atmosphere in nigeria. Polish J. Environ. Stud. 21, 177–186.
Pandey, U. B., and Nichols, C. D. (2011). Human disease models in Drosophila melanogaster and the role of the fly in therapeutic drug discovery. Pharmacol. Rev. 63, 411–436. doi: 10.1124/pr.110.003293
Pumo, R. L., Bellia, M., Nicosia, A., Micale, V., and Drago, F. (2006). Long-lasting neurotoxicity of prenatal benzene acute exposure in rats. Toxicology 223, 227–234. doi: 10.1016/j.tox.2006.04.001
D. B (Ed.). Roberts (1998). Drosophila: A Practical Approach, 2nd Edn. Oxford: Oxford University Press.
Rothenfluh, A., and Heberlein, U. (2002). Drugs, flies, and videotape: the effects of ethanol and cocaine on Drosophila locomotion. Curr. Opin. Neurobiol. 12, 639–645. doi: 10.1016/S0959-4388(02)00380-X
Ruchirawat, M., Navasumrit, P., and Settachan, D. (2010). Exposure to benzene in various susceptible populations: co-exposures to 1, 3-butadiene and PAHs and implications for carcinogenic risk. Chemico Biol. Interact. 184, 67–76. doi: 10.1016/j.cbi.2009.12.026
Ruden, D. M., Hirsch, H. V., Lnenicka, G., Possidente, D., Possidente, B., Garfinkel, M. D., et al. (2012). Drosophila melanogaster as a model for lead neurotoxicology and toxicogenomics research. Front. Genet. 3:68. doi: 10.3389/fgene.2012.00068
Saillenfait, A. M., Gallissot, F., Morel, G., and Bonnet, P. (2003). Developmental toxicities of ethylbenzene, ortho-, meta-, para-xylene and technical xylene in rats following inhalation exposure. Food Chem. Toxicol. 41, 415–429. doi: 10.1016/S0278-6915(02)00231-4
Salem, E., El-Garawani, I., Allam, H., El-Aal, B. A., and Hegazy, M. (2018). Genotoxic effects of occupational exposure to benzene in gasoline station workers. Indust. Health 56, 132–140. doi: 10.2486/indhealth.2017-0126
Salimi, A., Talatappe, B. S., and Pourahmad, J. (2017). Xylene induces oxidative stress and mitochondria damage in isolated human lymphocytes. Toxicol. Res. 33, 233–238. doi: 10.5487/TR.2017.33.3.233
Salvaterra, P. M., Hayashi, I., Perez-Magallanes, M., and Ikeda, K. (2006). Primary Culture of Drosophila Embryo Cells: Cell Biology. Philadelphia, PA: Academic Press, 151–155. doi: 10.1016/B978-012164730-8/50019-8
Santabarbara-Ruiz, P., Lopez-Santillan, M., Martinez-Rodriguez, I., Binagui-Casas, A., Perez, L., Milán, M., et al. (2015). ROS-induced JNK and p38 signaling is required for unpaired cytokine activation during Drosophila regeneration. PLoS Genet. 11:1005595. doi: 10.1371/journal.pgen.1005595
Sarkissian, T., Timmons, A., Arya, R., Abdelwahid, E., and White, K. (2014). Detecting apoptosis in Drosophila tissues and cells. Methods 68, 89–96. doi: 10.1016/j.ymeth.2014.02.033
Sarma, S. N., Kim, Y. J., Song, M., and Ryu, J. C. (2011). Induction of apoptosis in human leukemia cells through the production of reactive oxygen species and activation of HMOX1 and Noxa by benzene, toluene, and o-xylene. Toxicology 280, 109–117. doi: 10.1016/j.tox.2010.11.017
Shaikh, A., Barot, D., and Chandel, D. (2018). Genotoxic effects of exposure to gasoline fumes on petrol pump workers. Int. J. Occup. Environ. Med. (IJOEM) 9, 1159–1179. doi: 10.15171/ijoem.2018.1159
Sharma, D., Singh, M. P., Vimal, D., Kumar, S., Jha, R. R., and Chowdhuri, D. K. (2018). Benzene induced resistance in exposed Drosophila melanogaster: outcome of improved detoxification and gene modulation. Chemosphere 201, 144–158. doi: 10.1016/j.chemosphere.2018.02.135
Shorter, J., Couch, C., Huang, W., Carbone, M. A., Peiffer, J., Anholt, R. R., et al. (2015). Genetic architecture of natural variation in Drosophila melanogaster aggressive behavior. Proc. Natl. Acad. Sci. U.S.A. 112, E3555–E3563. doi: 10.1073/pnas.1510104112
Singh, M. P., Mishra, M., Sharma, A., Shukla, A. K., Mudiam, M. K. R., Patel, D. K., et al. (2011). Genotoxicity and apoptosis in Drosophila melanogaster exposed to benzene, toluene and xylene: attenuation by quercetin and curcumin. Toxicol. Appl. Pharmacol. 253, 14–30. doi: 10.1016/j.taap.2011.03.006
Singh, M. P., Ram, K. R., Mishra, M., Shrivastava, M., Saxena, D. K., and Chowdhuri, D. K. (2010). Effects of co-exposure of benzene, toluene and xylene to Drosophila melanogaster: alteration in hsp70, hsp60, hsp83, hsp26, ROS generation and oxidative stress markers. Chemosphere 79, 577–587. doi: 10.1016/j.chemosphere.2010.01.054
Singh, M. P., Reddy, M. K., Mathur, N., Saxena, D. K., and Chowdhuri, D. K. (2009). Induction of hsp70, hsp60, hsp83 and hsp26 and oxidative stress markers in benzene, toluene and xylene exposed Drosophila melanogaster: role of ROS generation. Toxicol. Appl. Pharmacol. 235, 226–243. doi: 10.1016/j.taap.2008.12.002
Snyder, R., and Hedli, C. C. (1996). An overview of benzene metabolism. Environ. Health Perspect. 104(Suppl. 6), 1165–1171. doi: 10.1289/ehp.961041165
Soós, I., and Szabad, J. (2014). Assaying benzene, a parquet varnish, and a synthetic thinner with respect to induction of in vivo chromosome loss in wing primordial cells of Drosophila. Mutat. Res. Genet. Toxicol. Environ. Mutag. 763, 18–22. doi: 10.1016/j.mrgentox.2013.11.007
Stormo, B. M., and Fox, D. T. (2016). Distinct responses to reduplicated chromosomes require distinct Mad2 responses. Elife 5:e15204. doi: 10.7554/eLife.15204
Sun, G., and Irvine, K. D. (2011). Regulation of Hippo signaling by Jun kinase signaling during compensatory cell proliferation and regeneration, and in neoplastic tumors. Dev. Biol. 350, 139–151. doi: 10.1016/j.ydbio.2010.11.036
Tarko, A., Štochmal’ová, A., Jedličková, K., Hrabovszká, S., Vachanová, A., Harrath, A. H., et al. (2019). Effects of benzene, quercetin, and their combination on porcine ovarian cell proliferation, apoptosis, and hormone release. Arch. Anim. Breed. 62:345. doi: 10.5194/aab-62-345-2019
Thammakhet, C., Villeneuve, T., Munisawang, V., Thavarungkul, P., and Kanatharana, P. (2004). Monitoring of BTX by passive sampling in Hat Yai. Songklan. J. Sci. Technol. 26(Suppl. 1), 151–160.
Thiel, R., and Chahoud, I. (1997). Postnatal development and behaviour of Wistar rats after prenatal toluene exposure. Arch. Toxicol. 71, 258–265. doi: 10.1007/s002040050385
Unckless, R. L., Rottschaefer, S. M., and Lazzaro, B. P. (2015). The complex contributions of genetics and nutrition to immunity in Drosophila melanogaster. PLoS Genet. 11:e1005030. doi: 10.1371/journal.pgen.1005030
Ungvary, G. Y., Tatrai, E., Hudak, A., Gy, B., and Lörincz, M. (1980). Studies on the embryotoxic effects of ortho-, meta-, and para-xylene. Toxicology 18, 61–74. doi: 10.1016/0300-483X(80)90038-4
Vaisnav, M., Xing, C., Ku, H. C., Hwang, D., Stojadinovic, S., Pertsemlidis, A., et al. (2014). Genome-wide association analysis of radiation resistance in Drosophila melanogaster. PLoS One 9:e104858. doi: 10.1371/journal.pone.0104858
Walser, T., Juraske, R., Demou, E., and Hellweg, S. (2013). Indoor exposure to toluene from printed matter matters: complementary views from life cycle assessment and risk assessment. Environ. Sci. Technol. 48, 689–697. doi: 10.1021/es403804z
Warden, H., Richardson, H., Richardson, L., Siemiatycki, J., and Ho, V. (2018). Associations between occupational exposure to benzene, toluene and xylene and risk of lung cancer in Montréal. Occup. Environ. Med. 75, 696–702. doi: 10.1136/oemed-2017-104987
Weber, A. L., Khan, G. F., Magwire, M. M., Tabor, C. L., Mackay, T. F., and Anholt, R. R. (2012). Genome-wide association analysis of oxidative stress resistance in Drosophila melanogaster. PLoS One 7:e34745. doi: 10.1371/journal.pone.0034745
Weeger, A. (2018). The power of GWAS: leveraging genome wide association studies to identify novel regulators of autophagy in Drosophila melanogaster. Gradu. Theses Dissert. 18:16486.
Yang, N., Ray, S. D., and Krafts, K. P. (2014). “Cell proliferation,” in Encyclopedia of Toxicology, 3 Edn, ed. P. Wexler (San Diego, CA: Elsevier), 761–765. doi: 10.1016/B978-0-12-386454-3.00274-8
Keywords: BTEX, Drosophila melanogaster, apoptosis, development, imaginal disk, GWAS, DGRP
Citation: Adebambo TH, Fox DT and Otitoloju AA (2020) Toxicological Study and Genetic Basis of BTEX Susceptibility in Drosophila melanogaster. Front. Genet. 11:594179. doi: 10.3389/fgene.2020.594179
Received: 12 August 2020; Accepted: 25 September 2020;
Published: 15 October 2020.
Edited by:
Joao Batista Teixeira da Rocha, Federal University of Santa Maria, BrazilReviewed by:
Elgion Lucio Silva Loreto, Federal University of Santa Maria, BrazilMahendra Singh, Lovely Professional University, India
Copyright © 2020 Adebambo, Fox and Otitoloju. This is an open-access article distributed under the terms of the Creative Commons Attribution License (CC BY). The use, distribution or reproduction in other forums is permitted, provided the original author(s) and the copyright owner(s) are credited and that the original publication in this journal is cited, in accordance with accepted academic practice. No use, distribution or reproduction is permitted which does not comply with these terms.
*Correspondence: Donald T. Fox, ZG9uLmZveEBkdWtlLmVkdQ==; Temitope H. Adebambo, dG9wamV0MjAwMkBnbWFpbC5jb20=