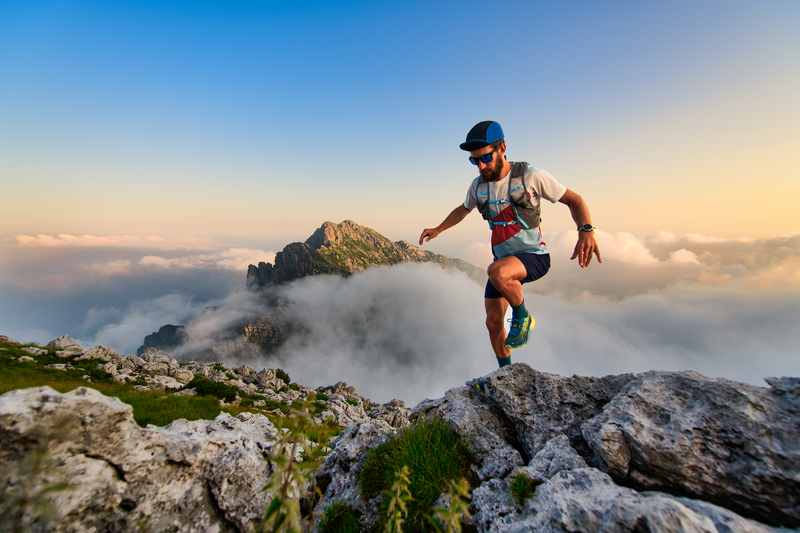
94% of researchers rate our articles as excellent or good
Learn more about the work of our research integrity team to safeguard the quality of each article we publish.
Find out more
ORIGINAL RESEARCH article
Front. Genet. , 11 September 2020
Sec. Systems Biology Archive
Volume 11 - 2020 | https://doi.org/10.3389/fgene.2020.00883
This article is part of the Research Topic Biological Control Systems and Disease Modelling View all 21 articles
Preterm birth (PTB) is the leading cause of morbidity and mortality in infants <1 year of age. Intrauterine inflammation is a hallmark of preterm and term parturition; however, this alone cannot fully explain the pathobiology of PTB. For example, the cervix undergoes a prolonged series of biochemical and biomechanical events, including extracellular matrix (ECM) remodeling and mechanochemical changes, culminating in ripening. Vaginal progesterone (P4) prophylaxis demonstrates great promise in preventing PTB in women with a short cervix (<25 mm). We used a primary culture model of human cervical stromal fibroblasts to investigate gene expression signatures in cells treated with interleukin-1β (IL-1β) in the presence or absence of P4 following 17β-estradiol (17β-E2) priming for 7–10 days. Microarrays were used to measure global gene expression in cells treated with cytokine or P4 alone or in combination, followed by validation of select transcripts by semiquantitative polymerase chain reactions (qRT-PCR). Primary/precursor (MIR) and mature microRNAs (miR) were quantified by microarray and NanoString® platforms, respectively, and validated by qRT-PCR. Differential gene expression was computed after data normalization followed by pathway analysis using Kyoto Encyclopedia Genes and Genomes (KEGG), Panther, Gene Ontology (GO), and Ingenuity Pathway Analysis (IPA) upstream regulator algorithm tools. Treatment of fibroblasts with IL-1β alone resulted in the differential expression of 1432 transcripts (protein coding and non-coding), while P4 alone led to the expression of only 43 transcripts compared to untreated controls. Cytokines, chemokines, and their cognate receptors and prostaglandin endoperoxide synthase-2 (PTGS-2) were among the most highly upregulated transcripts following either IL-1β or IL-1β + P4. Other prominent differentially expressed transcripts were those encoding ECM proteins, ECM-degrading enzymes, and enzymes involved in glycosaminoglycan (GAG) biosynthesis. We also detected differential expression of bradykinin receptor-1 and -2 transcripts, suggesting (prominent in tissue injury/remodeling) a role for the kallikrein–kinin system in cervical responses to cytokine and/or P4 challenge. Collectively, this global gene expression study provides a rich database to interrogate stromal fibroblasts in the setting of a proinflammatory and endocrine milieu that is relevant to cervical remodeling/ripening during preparation for parturition.
Cervical integrity is crucial for a successful human pregnancy. Throughout most of an uncomplicated gestation, the cervix provides a physical and immune barrier between the interior of the uterus and the vaginal microbiome (Word et al., 2007; Akgul et al., 2014; Vink and Feltovich, 2016). The cervix prepares for parturition by first slow and then rapid transition from an elongated, closed, and rigid structure to an orifice sufficiently soft and dilated to facilitate delivery of the fetus.
Human and animal studies have yielded a working model for the biomolecular underpinnings of cervical remodeling/ripening (Elovitz and Mrinalini, 2004; Word et al., 2007; Timmons et al., 2010; Yellon, 2017). Broadly speaking, normal remodeling is the product of changes in the organization and composition of the extracellular matrix (ECM) during pregnancy, including a decrease in cross-linked collagens I and III, changes in the composition of glycosaminoglycans (GAGs), especially elevated hyaluronan (HA) production, increased tissue hydration, and leukocyte infiltration (Sakamoto et al., 2005; Myers et al., 2009; Akgul et al., 2012; Dubicke et al., 2016).
Progesterone receptor (PR, NR3C3) signaling underpins many of the physiological processes that oppose untimely cervical dilation (Word et al., 2007). Two separate protein isoforms, PR-A (90 kDa) and PR-B (130 kDa), are encoded by a single gene differentially expressed via alternate promoter usage (Kastner et al., 1990; Stjernholm-Vladic et al., 2004). In rodents, pregnancy maintenance requires continued synthesis of progesterone (P4) by the corpus luteum. Systemic withdrawal of P4 during luteolysis in rodents evokes cervical ripening and labor at term, while premature cervical ripening is prompted by ovariectomy (Gonzalez et al., 2009). Additionally, pharmacologic antagonism of the PR by mifepristone (RU-486) promotes cervical ripening in animal models (Chwalisz et al., 1994). Conversely, administration of vaginal P4 reduces the incidence of preterm birth in women with a sonographically validated short cervix (Hassan et al., 2011).
There is strong evidence of the therapeutic utility of P4 for the prevention of untimely cervical ripening and preterm labor in at-risk women (Conde-Agudelo and Romero, 2016). However, unanswered questions persist regarding the mechanisms by which P4 influences the expression of inflammation-related genes, including cytokines and chemokines, extracellular matrix (ECM)-modifying enzymes, and bioactive lipid-generating enzymes (e.g., prostaglandin endoperoxidase synthase-2, PTGS-2; microsomal prostaglandin E synthase-1, mPGES; 5-lipoxygenase, 5-LOX) (Kniss, 1999; Sato et al., 2001; Ackerman et al., 2005) in gestational tissues, including the cervix. The overarching goal of the present study was to evaluate gene expression programs (including protein-coding and non-coding RNAs) executed in response to proinflammatory cytokine and/or PR:P4 stimulation in cervical fibroblasts. Our results revealed a multifaceted profile of gene expression in the cervical stroma, including (1) genes that are cytokine-responsive/P4-insensitive; (2) genes that are P4-sensitive/cytokine-independent; (3) genes that are cytokine-responsive and suppressed by P4; and (4) genes that are augmented by both cytokines and progesterone. These data provide a framework allowing us to construct gene networks involved in the manner by which PR signaling may prevent or delay inflammation-induced cervical ripening and consequent preterm labor. The ultimate goal of this work is that it allows us to identify and develop novel therapeutic targets for prevention and/or management of preterm labor, especially in at-risk women who manifest cervical insufficiency, a major cause of PTB.
With institutional review board approval (OSU Biomedical IRB Protocol Number 2013H0046), de-identified cervical tissues were obtained from premenopausal women undergoing hysterectomy for benign gynecological conditions. Primary human cervical stromal fibroblasts were isolated via outgrowth from explanted cervical stromal tissues as previously described (Ackerman et al., 2016b). For experiments, cells were grown to confluence in complete Dulbecco’s modified Eagle’s medium (DMEM, high-glucose, 4.5 g/l) supplemented with 10% fetal bovine serum (FBS), 50 μg/ml gentamicin sulfate, and 0.5 μg/ml amphotericin B (all from Invitrogen, Carlsbad, CA, United States). Next, the cells were rinsed with Dulbecco’s phosphate-buffered saline (DPBS) and then incubated in experimental medium containing phenol red-free DMEM/F12 (1:1) with 0.5% charcoal-stripped FBS (prepared in-house using charcoal-dextran extraction, clarification by centrifugation, and sterilization through a 0.2-μm filter) in the absence (0.001% ethanol vehicle control) or presence of 17β-estradiol (17β-E2, 10–8 M; Sigma-Aldrich, St. Louis, MO, United States) for 7–14 days to promote the expression of nuclear progesterone receptors (PRs) (Ackerman et al., 2016b). Finally, cells were incubated for 24 h in the absence or presence of P4 (10–7 M; Sigma-Aldrich), followed by challenge for 4 or 24 h with 0.2 ng/ml of human recombinant interleukin-1β (IL-1β; R&D Systems, Minneapolis, MN, United States) or an equivalent volume of vehicle (PBS with 0.1% bovine serum albumin, BSA). All experiments were performed between the 3rd and 7th passages after primary explant cultures were prepared. To validate the expression patterns of select transcripts following high-dimensional profiling studies, biological replicate experiments were performed using treatment conditions identical to those described above using a separate set of cell cultures. Cell cultures were routinely tested for mycoplasma species using an in-house PCR-based assay (MycoAlertTM, Lonza, Anaheim, CA, United States).
Total RNA was extracted from harvested cells using TRIzol (Invitrogen). Following the addition of chloroform and centrifugation, the aqueous phase was mixed with an equal volume of 100% ethanol, applied to a miRNeasy spin column (Qiagen, Valencia, CA, United States) and processed according to the manufacturer’s protocol. The extraction procedure included on-column DNase I digestion using the RNase-Free DNase Set (Qiagen) to remove contaminating genomic DNA. RNA was quantified by absorbance at 260 and 280 nm using a NanoDrop 2000 spectrophotometer (Thermo Fisher, Hudson, NH, United States).
Total RNA (250 ng per sample) was processed using the Ambion WT expression kit (Austin, TX, United States) and labeled with Affymetrix GeneChip (Santa Clara, CA, United States) whole-transcript sense target labeling assay, followed by hybridization to the Affymetrix Human Transcriptome 2.0 array according to the manufacturer’s protocols. Following hybridization and scanning, quality control and robust multichip averaging were performed on the feature intensity files using the Affymetrix Expression Console software version 1.4. Gene-level differential expression analysis was subsequently performed using the Affymetrix Transcriptome Analysis Console software version 3.0 using the paired-sample analytical pipeline (one-way repeated-measure ANOVA), and the Benjamini–Hochberg false discovery rate (FDR)-controlling procedure (Reiner et al., 2003). For the default differential gene expression analysis, a linear fold-change threshold of ± 2 and an FDR of 10% was applied.
Multiplexed mature miRNAs were profiled using the Human v3 miRNA Expression Assay (NanoString® Technologies, Seattle, WA, United States). Total RNA (100 ng) was used as input for the nCounter® miRNA sample preparation reactions according to the manufacturer’s instructions. Hybridization reactions were performed at 64°C for 18 h. Hybridized probes were analyzed using the nCounter digital analyzer. For each assay, a high-density scan (600 fields of view) was performed. The nSolver® Analysis Software version 3.0 was used for technical normalization. Probes with low levels of expression (defined as less than the mean 2 ± SD of counts assigned to negative control probes, which was 33.04 in our data set) were omitted from subsequent analyses. Differential expression analysis of the NanoString® data was performed using the edgeR (version 3.14.0) Bioconductor package (Robinson et al., 2010). The trimmed mean of M-value normalization was used together with the generalized linear model approach coupled with a paired-sample design matrix. Differential expression was determined using generalized linear model likelihood ratio tests. For FDR control, the Benjamini–Hochberg procedure was used (Reiner et al., 2003).
To validate the expression of select mRNAs, 1 μg of total RNA was reverse transcribed to complementary DNA (cDNA) using oligo(dT)12–18 primers with SuperScript® III Reverse Transcriptase (Life Technologies, Grand Island, NY, United States). Quantitative PCR was performed using an equal amount of cDNA per sample on a LightCycler 480 II System (Roche Applied Science, Indianapolis, IN, United States) using the following TaqMan® primer/probe sets (Applied Biosystems, Foster City, CA, United States): BDKRB1 (Hs00664201_s1), BDKRB2 (Hs00176121_m1), CXCL8 (Hs00174103_m1), FKBP5 (Hs01561006_m1), HAS2 (Hs00193435_m1), HSD11B1 (Hs00194153_m1), IL1B (Hs01555413_m1), IL6 (Hs00985639_m1), IRAK3 (Hs00936103_m1), MMP10 (Hs00233987_m1), PTGES (Hs00610420_m1), and PTGS2 (Hs00153133_m1). The expression of RPLP0 (4310879E) was used as a reference.
For primary/precursor miRNAs (pri-miRNAs), reverse transcription was performed using the high-capacity complementary deoxyribonucleic acid reverse transcription kit (Applied Biosystems), according to the manufacturer’s instructions. Each reaction comprised 10 μl of master mix (10 × reverse transcription buffer, deoxynucleotide triphosphates, reverse transcription random primers, MultiScribe® reverse transcription enzyme (Thermo Fisher Scientific), ribonuclease inhibitor, and nuclease-free water) and 1 μg of RNA (in 10 μl reaction volume). For qRT-PCR, TaqMan® gene expression master mix and TaqMan Pri-miRNA assays (Applied Biosystems) were used. The assays were Hs03303259_pri (MIR146A) and Hs03303349_pri (MIR155). For mature miRNAs, reverse transcription was performed with total RNA using the TaqMan microRNA reverse transcription kit (Applied Biosystems), per the manufacturer’s recommendations. Each reaction received 7 μl of TaqMan gene expression master mix, 5 μl of RNA (10 ng), and 3 μl of the reverse transcription primer appropriate for each target miRNA. TaqMan microRNA assays (Applied Biosystems) were used for qRT-PCR. The following assays were used: 000468 (hsa-miR-146a-5p) and 002623 (hsa-miR-155-5p). The relative abundance of each mRNA or miRNA was calculated by the comparative CT method (Schmittgen and Livak, 2008).
Pathway analysis for microarray data was performed using the Gene Set Enrichment Analysis (GSEA) Java desktop application (version 2.2.21) (Subramanian et al., 2005). For a given gene set, this algorithm calculates an enrichment score, which numerically reflects the degree to which a given dataset is overrepresented within a ranked list of gene expression data. The statistical significance of a given enrichment score is estimated using an empirical permutation test procedure, followed by correction for multiple-hypothesis testing. This analysis method tends to be more sensitive and robust than overrepresentation methods relying solely on differential expression with arbitrary cutoffs (Subramanian et al., 2005). Three databases of curated gene sets were obtained from an online repository2 (Merico et al., 2010) and queried: 1. Kyoto Encyclopedia of Genes and Genomes (KEGG)3; 2. Panther4; and 3. Gene Ontology (GO) molecular function5. All gene sets with 1–500 members were evaluated using 1000 gene-set permutations (as recommended when fewer than seven samples in any phenotype are available for analysis) by applying the default weighted enrichment statistic, the signal-to-noise ratio metric for ranking genes, and the default method for enrichment score normalization. Additionally, the Ingenuity Pathways Analysis (IPA) upstream regulator algorithm (Qiagen) was employed to infer upstream signaling events potentially contributing to observed gene expression signatures.
The promoter regions (i.e., FASTA sequences ± 1000 bp relative to each transcription start site) of differentially expressed genes in select conditions were programmatically retrieved from the University of California Santa Cruz hg38 human genome assembly via the TogoWS SOAP API website6 (Katayama et al., 2010). The “multiple DNA sequences” algorithm of the Transcription Factor Affinity Prediction (Thomas-Chollier et al., 2011) (TRAP) Web Tools suite7 was used to probe these regulatory regions for transcription factor binding affinities based on position-specific scoring matrices present in the non-redundant JASPAR core vertebrate database (Mathelier et al., 2014) using a background model of all human promoter regions.
Statistical analyses were performed using the Kruskal–Wallis statistical test with post hoc testing using Dunn’s multiple comparison test when appropriate. A p-value < 0.05 was considered significant. Microarray and qRT-PCR experiments were replicated three times in duplicate (i.e., n = 6 samples per treatment). The data were tested for Gaussian distribution, and, if normally distributed, they were expressed as mean ± SEM and evaluated by analysis of variance (ANOVA) followed by post hoc testing by the method of Tukey using GraphPad Prism 8.0 software (San Diego, CA, United States).
The experimental design for the studies described throughout this work is shown in Figure 1A. Importantly, cervical stromal fibroblasts were stimulated to elicit the expression of progesterone receptors (Figure 1B). After carrying out experiments, total RNA from each study group was extracted and purified, quantified, and subjected to microarray analysis of differential gene (coding and non-coding RNAs) expression. To confirm these results, we also conducted follow-up experiments using the identical design with biological replicates (i.e., a separate set of cell cultures not used for microarray analysis) to measure by qRT-PCR transcripts induced by either IL-1β, P4, or both agents incubated simultaneously. Extensive bioinformatics analysis was conducted using several publicly available genomic analytical tools (KEGG, Panther, and GO algorithms (Figure 2).
Figure 1. Experimental design and workflow. (A) Primary cultures of cervical fibroblasts were primed with 10–8 M 17β-estradiol (17β-E2) or 0.001% ethanol vehicle for 7–14 days in F12/DMEM++0.5% charcoal-stripped FBS. Cells were then treated with 10–7 M progesterone (P4) or vehicle for 24 h followed by a 4-h stimulation with 0.2 ng/ml interleukin-1β (IL-1upbeta) or vehicle (PBS/0.1% BSA). Total RNA was extracted to enrich for small RNAs and subjected to microarray analysis using the Affymetrix platform for long, coding transcripts and the NanoString® platform for small, non-coding RNAs. After normalization, the data were subjected to differential expression analysis was conducted using the Affymetrix Transcription Analysis Console. Downstream relationships were evaluated using Gene Set Enrichment Analysis (GSEA), Transcription Factor Binding Motif (TFBM) analysis, and Ingenuity Pathway Analysis. The relative expression data are the mean and FDR p-value from three separate sets of biological replicates. (B) depicts the expression of PR-A and PR-B using immunofluorescence (left panel) or immunoblotting (right panel) following priming with 17β-E2.
Figure 2. Microarray and bioinformatics analysis of differentially expressed genes in cervical stromal fibroblasts stimulated with IL-1β (0.2 ng/ml) or P4 (10–7 M) for hrs. Cells were primed with 17β-E2 (10–8 M) to induce progesterone receptors -A and -B and then challenged for 4 hrs with IL-1β (A–D) or P4 for 4 h (after a 24-h preincubation with the steroid) (E–H), total RNA isolated and quantified and differential gene expression by microarray analysis. The normalized data are expressed as mean signal intensity (log2) and are from three separate experiments carried out in duplicate (A,E). Bioinformatics analysis of differentially expressed transcripts using KEGG (B,F), Panther (C,G) and GO (D,H) algorithms.
Cervical fibroblasts cultured as described (Ackerman et al., 2016b) were pretreated for 7–14 days with 10–8 M 17β-E2 to induce nuclear PR expression. Relative to vehicle-treated controls, microarray profiling of cells stimulated for 4 h with IL-1β (0.2 ng/ml) revealed changes in gene expression, with significant (minimum ≥two-fold change in expression and FDR <0.1) upregulation of 913 and downregulation of 519 transcripts (Figure 2A and Supplementary Table S1). As we observed previously in other intrauterine cell types, e.g., amnion mesenchymal fibroblasts (Li et al., 2011) and uterine decidual stromal cells (Ibrahim et al., 2016), highly IL-1β-induced transcripts included those encoding a wide array of cytokines and chemokines, enzymes involved in prostaglandin synthesis, bradykinin receptors-1 and -2, and matrix metalloproteinases, among others. Other prominent differentially expressed mRNAs included acute phase reactants, members of the complement family including tissue factor pathway inhibitor 2, and other proteases and their inhibitors (Supplementary Table S1). Intracellular signal transduction pathways differentially expressed following IL-1β treatment included the interferon (IFN), signal transducer and activator of transcription 5 (STAT5), and mitogen-activated protein kinase (MAP kinase)/phosphatase pathways (Supplementary Table S1).
Highly enriched pathways, as determined by the GSEA algorithms (KEGG, Panther, and GO, respectively), included those related to inflammatory signaling, apoptosis, blood coagulation, cell adhesion, and arachidonic acid metabolism (Figures 2B–D and Supplementary Table S2). Based on this gene expression signature, the IPA upstream regulator algorithm predicted activation of 65 and inhibition of 23 transcriptional regulators. As expected, following proinflammatory cytokine challenge, activation was inferred for nuclear factor-κB (NF-κB), components of the JAK-STAT signaling pathway, interferon regulatory factors (IRFs), activator protein-1 (AP-1) subunits, and Forkhead box (FOX) transcription factors, among many others (Supplementary Table S3). Confirming these predictions, a complementary promoter scanning analysis (TRAP analysis) of the differentially regulated genes revealed overrepresentation of canonical binding motifs corresponding to several of these transcription factors, including NF-κB (i.e., the NF-kappaB, NFKB1, REL, and RELA model matrices), FOX (i.e., the Foxq1, FOXF2, FOXO3, FOXD1, Foxa2, and FOXI1 matrices), IRF (i.e., the IRF1 and IRF2 matrices), STAT (i.e., the STAT1 and STAT3 matrices), and AP1 (Supplementary Table S4).
Although not prominently represented in the pathway analyses, given the importance of the ECM in cervical remodeling, we surveyed IL-1β-responsive transcripts for changes in genes responsible for ECM integrity, including proteoglycans, fibrous proteins, and genes associated with ECM biosynthesis and degradation. IL-1β induced the expression of three proteoglycan-related proteins (hyaluronan receptor/CD44, syndecan-4/SDC4, and serglycin/SRGN) and attenuated the expression of an additional two (decorin/DCN and structural maintenance of chromosome 3/chondroitin sulfate proteoglycan-6/SMC3) proteins. IL-1β also induced the expression of elastin (ELN), a basement membrane-associated procollagen (collagen type IV, α1 chain/COL4A1), and several genes involved in glycosaminoglycan (GAG) biosynthesis (β-1,4-galactosyltransferase-1/B4GALT1, carbohydrate sulfotransferase-11/CHST11, exostosin glycosyltransferase-/EXT1, fucosyltransferase-8/FUT8, hyaluronan synthase-2/HAS2, heparan sulfate-glucosamine 3-sulfotransferase-3B1/HS3ST3B1, and/ST3 β-galactosidase α-2,3-sialytransferase 1/3GAL1), while decreasing the expression of collagen type III, α1 chain/COL3A1, a major fibrillar procollagen in the cervical ECM Supplementary Table S1.
In contrast to the surfeit of differentially expressed genes observed following IL-1β stimulation, the transcriptional response to progesterone (10–7 M, a dose similar to the maternal circulating levels late in human gestation) (Word et al., 2007) was modest. Compared to cells receiving vehicle alone, cervical fibroblasts incubated with P4 exhibited expression changes in only 52 genes, 34 of which were upregulated and 18 downregulated (Figure 2E and Supplementary Table S5).
Top-ranking pathways (analyzed by KEGG, Panther, and GO algorithms) associated with this gene expression signature included those related to the amino acid metabolism, xenobiotics, and fatty acid biosynthesis and catabolism, in addition to cortisol biosynthesis, insulin-like growth factor (IGF) signaling, and sodium reabsorption and the renin–angiotensin system (RAS) (Figures 2F–H and Supplementary Table S6). Based on this expression pattern, the IPA upstream regulator algorithm correctly predicted activation of the PR and inferred inhibition for a small number of inflammatory and growth regulators (Supplementary Table S7). Promoter scanning analysis revealed overrepresentation of the NR3C1 model matrix corresponding to the consensus binding motif of the glucocorticoid receptor (GRE: 5’RGRACANNNTGTYC3’, where R = purine, Y = pyrimidine, N = any nucleic acid) (Supplementary Table S8). This was somewhat expected, inasmuch as the response elements for the glucocorticoid and progesterone receptors are quite similar (Lieberman et al., 1993; Nelson et al., 1999), and a separate PR response element model was not included in the non-redundant JASPAR vertebrate matrix database used for this query.
Unexpectedly, however, these promoter regions were also enriched for NF-κB-binding motifs. Given that we expected NF-κB-responsive genes to be induced following cytokine challenge, we then compared the extent of overlap between the genes differentially expressed following IL-1β and P4 co-stimulation. We found that a considerable proportion (31%) of the genes influenced by P4 were also IL-1β responsive; specifically, nine genes were upregulated in both conditions. In addition, eight genes were downregulated following either treatment. Interestingly, most genes induced by either treatment were found to have roles in mitigating inflammation based on literature review (Table 1).
Relative to control cells, the global cellular response to IL-1β + P4, like that following IL-1β exposure alone, was considerable: 889 genes exhibited induced expression, while 504 showed diminished expression (Figures 3A–C and Supplementary Table S9). The Jaccard similarity indices between these treatment groups were 0.79 and 0.62 for upregulated and downregulated genes, respectively. A minority (24 transcripts) of the genes differentially expressed by IL-1β + P4 were also regulated by P4 alone. Among the 17 genes responsive to both IL-1β and P4 mentioned previously were ABI family member 3 binding protein/ABI3BP, collagen type VIII α1 chain/COL8A1, estrogen receptor 1/ESR1, osteomodulin/OMD, plexin domain containing 2/PLXDC2, prolactin receptor/PRLR, and secreted protein acidic and cysteine-rich-like 1/SPARCL1.
Figure 3. Differential gene expression in cervical stromal fibroblasts stimulated with either IL-1β (0.2 ng/ml), P4 (10–7 M) or both for 4 h followed by microarray analysis and data normalization. (A) shows the scatter plot and Venn diagrams comparing upregulated (B) and downregulated transcripts (C) in response to IL-1β +P4 or vehicle. (D) Scatter plot and (E) heat map of differentially expressed genes in response to IL-1β or IL-1β + P4. (F) Scatter plot and (G) heat map of differentially expressed genes in response to P4 or IL-1β +P4.
To assess the degree to which co-incubation with P4 modified global IL-1β-inducible gene expression, we next compared the IL-1β and IL-1β + P4 treatment groups. Of the 39 genes differentiating these two groups, 16 were downregulated by combined IL-1β + P4 treatment relative to IL-1β alone (Figure 3B and Supplementary Table S10). Of these, 11 genes were responsive when cells were incubated with IL-1β alone, including two proinflammatory interleukins (IL1B, IL6), a matrix metalloproteinase (MMP10), and the inhibin beta A subunit (INHBA) (Figure 3E). Finally, to determine how cytokine stimulation affected P4-induced gene expression, we compared the P4 and IL-1β + P4 treatment groups (Figure 3F and Supplementary Table S11). Among the 446 genes downregulated by IL-1β under these conditions were 16 genes differentially regulated by P4 alone. Among the 13 progestin-responsive genes exhibiting diminished expression when incubated in the presence of IL-1β were those encoding the transcription factor myocardin (MYOCD), alcohol dehydrogenase 1B beta (ADH1B), the WNT signaling pathway inhibitor Dickkopf Inhibitor 1 (DKK1), and the ECM scaffold glycoprotein fibrillin 2 (FBN2) (Figure 3G).
To validate the initial profiling results, we performed qRT-PCR for select transcripts in experiments using separate biological replicates. These transcripts were categorized into three general expression patterns: (1) additive/synergistic expression in response to IL-1β + P4 compared to either treatment alone (e.g., IRAK3, HSD11B1, and FKBP5) (Figure 4A); (2) induction by IL-1β with no significant response to P4 in the absence or presence of IL-1β (e.g., BDKRB1, BDKRB2, PTGES, and CXCL2) (Figure 4B); and (3) IL-1β-inducible expression attenuated when co-incubated with P4 (e.g., HAS2, IL1B, IL6, MMP10, and PTGS2) (Figure 4C).
Figure 4. Quantitative RT-PCR of select differentially expressed transcripts in cervical stromal fibroblasts stimulated with vehicle, IL-1β, P4 or IL-1β+P4 for 4 h. (A) Additive or synergistic relationship between P4 and IL-1 (B) No effect of P4 (C) Inhibitory effect of P4. After treatments, total RNA was isolated and quantified and primer sets were used to analyze individual transcripts in three separate experiments carried out in duplicate. Data are expressed as mean ± SEM of relative signal intensity (target/human acidic ribosomal protein).
The microarray chip analysis included both coding and non-coding RNA transcripts. We detected several miRNAs (host genes, pri-/pre-miRNAs, and mature miRNAs) and long, intergenic non-coding (LINC) RNAs in our microarray studies that were selectively upregulated by IL-1β stimulation. In addition, 12 non-coding RNAs, including five LINC RNAs, were downregulated when cells were challenged with the cytokine (Supplementary Table S1). In subsequent experiments, using biological replicates (samples separate from those used in the microarray studies), we used NanoString and qRT-PCR to further assess non-coding RNA expression. Given the modest differential expression of LINC RNAs in our experiments, we did not pursue these transcripts in follow-up analyses.
We surveyed the expression of 800 mature miRNAs simultaneously using the non-amplification-based NanoString platform. Overall, the expression of individual miRNAs spanned five orders of magnitude, with 346 transcripts having average normalized counts above the threshold for detection (Figure 5A). The most highly expressed mature miRNAs under basal (vehicle treatment only) conditions were let-7a-5p, let-7b-5p, miR-125b-5p, miR-145-5p, and miR-4516. When subjected to differential gene expression analysis, nine mature miRNAs were differentially expressed under any treatment condition (Figure 5B and Supplementary Table S12), with expression changes on the order of two-fold or smaller. This was in striking contrast to the rather large fold changes in expression estimated at the level of miRNA host genes (i.e., immature pri-/pre-miRNA transcripts). For example, our microarray data indicated that the host gene for miR-155 changed by 20-fold in response to IL-1β (Supplementary Table S1), yet the corresponding mature miR-155-5p exhibited no discernible change in expression in the NanoString dataset (not shown). Using qRT-PCR, we found that the pri-/pre-miRNAs MIR146A and MIR155 exhibited large changes in expression (166- and 32-fold, respectively) in response to IL-1β, yet the corresponding mature species changed only modestly (miR-146a-5p and miR-155-5p) (Figure 5).
Figure 5. Differential expression of microRNAs in cervical stromal fibroblasts stimulated with IL-1β, P4 or co-incubated with IL-1β + P4. (A) Basal (normalized counts) levels of the most prominently expressed mature miRNAs above the detection limit in cervical stromal cells using the unamplified NanoString® target counting platform. (B) Heat map of differentially expressed mature miRNAs following treatment with vehicle, IL-1β, P4 or co-incubated with IL-1β + P4. Data are from three separate experiments carried out in duplicate.
In our studies of miRNA expression in response to IL-1β and/or P4 exposure, we used the microarray platform to measure pri-/pre-miRNA transcripts while NanoString profiling was used to evaluate mature, fully processed miRNA species. We were surprised to learn that, while many pri-/pre-non-coding RNA host genes (including long non-coding and coding transcripts) were differentially expressed in cytokine-stimulated cells, far fewer full-processed miRNAs were observed (Supplementary Table S1 and Figure 5). This led us to postulate that this was due, at least in part, to the fact that while microarray analysis requires amplification of target transcripts, NanoString does not utilize a pre-amplification step prior to measurements.
To test this possibility, we compared directly two highly expressed miRNA transcripts (miR-146a and miR-155) using qRT-PCR of both immature and mature miRNAs. Figure 6A demonstrated that IL-1β stimulated robust upregulation of primary/precursors, MIR146a and MIR155, while co-incubation of IL-1β-treated cells with P4 attenuated MIR146a and MIR155 expression. In contrast, when we used the same analytical platform (qRT-PCR) to evaluate mature miRNAs, we found that, while IL-1β induced miR-146a-5p and miR-155-5p, P4 had no inhibitory effect on these two non-coding transcripts (Figure 6B).
Figure 6. Quantitative RT-PCR measurement of select primary/precursor and mature miRNA transcripts in cervical stromal fibroblasts from vehicle-, IL-1β-, P4, or IL-1β + P4-treated cells. Three separate experiments (carried out in duplicate) using biological replicates were incubated with the test agents listed above for 4 h, and then total RNA was isolated, quantified and subjected to RT-PCR using primers specific for either the primary/precursor or mature miRNA transcripts. (A) Primary/precursor miRNA transcripts. (B) Fully processed, mature miRNAs. Data are expressed as mean ± SEM of relative intensity (target/human acidic ribosomal protein) and were analyzed by one-way ANOVA followed by Tukey’s test for differences (p < 0.05, considered significant).
The current work investigated for the first time the modulation of cytokine-induced gene expression by P4 using a simple, clinically relevant in vitro model of human cervical stromal fibroblasts. We chose to study the stroma based on the fact that the cervical stroma is the primary tissue that undergoes biochemical and biophysical remodeling allowing the fetus to descend the birth canal at the time of delivery (Malmstrom et al., 2007; Dubicke et al., 2016; Yellon, 2017; Shukla et al., 2018). Using a microarray platform and qRT-PCR-based validation, we demonstrated that IL-1β stimulation of stromal cells elicited changes in a panoply of genes encoding proinflammatory mediators, including cytokines and chemokines, arachidonic acid synthesizing enzymes, ECM-synthesizing enzymes, MMPs, and other proteases, and intracellular signaling proteins and transcription factors.
We also examined the regulation of genes in response to incubation with physiological levels of progesterone that are present at the end of pregnancy. In contrast to previous reports by DeMayo and colleagues (Jeong et al., 2005; Rubel et al., 2012), we detected relatively few genes that were directly controlled by P4. With the exception of a few genes which were upregulated >four-fold (i.e., the matricellular protein SPARC-like 1/hevin, 18.66-fold; osteomodulin, 7.3-fold; inositol monophosphatase 2, 6.39-fold; alcohol dehydrogenase 1B, 5.36-fold; FK506-binding protein 5, 4.13-fold), most progestin-regulated genes were only modestly upregulated or downregulated in stromal cells.
In contrast to gene expression in the context of progestin alone, co-incubation of cervical stromal cells with IL-1β and P4 elicited three distinct patterns of regulation. One cohort of transcripts was profoundly upregulated by treatment with IL-1β but was unaffected by P4 treatment alone (Figure 4B). A second set of transcripts exhibited IL-1β-stimulated upregulation that was almost completely suppressed by co-incubation with P4 (Figure 4C). Notably, this set of genes was represented by proinflammatory cytokines (IL-1β and IL-6), the enzyme responsible for hyaluronan synthesis (i.e., HAS-2) (Garantziotis and Savani, 2019) and the rate-limiting enzyme in proinflammatory prostaglandin biosynthesis (i.e., PTGS-2) (Kniss, 1999). This can be interpreted as the subset of genes for which progestins exert anti-inflammatory actions.
Interestingly, there was a third set of IL-1β upregulated transcripts that was further elevated either additively or synergistically when cervical stromal cells were co-incubated with cytokine and progestin, including the steroid hormone receptor chaperone FK506-binding protein 5 (FKBP5) (Storer et al., 2011) and 11β-HSD1, the enzyme that converts biologically inert cortisone into bioactive cortisol (Tomlinson et al., 2004; Chapman K. et al., 2013; Figure 4A). Thus, the previously undescribed finding of three different patterns of gene expression exposed to cytokine and progestin in combination suggests that P4 exerts complex regulatory control in the cervical stromal compartment and that, strictly speaking, it is not simply an anti-inflammatory steroid hormone. The suppression of proinflammatory genes induced by IL-1β by physiological concentrations of P4 was predicted from previous studies in decidual stromal cells (Cakmak et al., 2005) and myometrial cells (Mendelson, 2009; Shynlova et al., 2013; Georgiou et al., 2016; Amini et al., 2019).
Analysis of the global expression of non-coding RNAs in cervical stromal cells treated with cytokine in the presence or absence of progestin revealed that miRNAs represent a relative minority of regulatory inputs to gene expression. Upregulated non-coding transcripts measured by microarray included both miRNAs encoded within host genes and LINC RNAs. The most highly upregulated miRNA transcript in IL-1β-treated cells was miR-155. MicroRNA-155 (hsa-mir155, coded within B-cell integration cluster (BIC) of non-coding transcripts located on chromosome 21, TargetScan, Release 7.1, June 2106; miRbase, Release 22.1, October 2018) (Madden et al., 2010; Kozomara and Griffiths-Jones, 2014) has been reported by many investigators to be induced in the setting of inflammation (O’Connell et al., 2007; Tili et al., 2007; Ceppi et al., 2009; Quinn and O’Neill, 2011; Xu et al., 2013) as a means to control tissue damage. We have recently demonstrated in decidual stromal cells that IL-1β causes the rapid and sustained upregulation of miR-155 (Ibrahim et al., 2016), and this was further confirmed using tissues isolated from patients who had a preterm delivery (Ackerman et al., 2016a). Thus, cytokine induction of miR-155 can be interpreted as a potential feedback loop to prevent tissue injury in the face of unrestrained acute inflammation (Baltimore et al., 2008; Contreras and Rao, 2012). One proposed mechanism by which miR-155 thwarts unwanted inflammation is by inhibition of the canonical inflammatory transcription factor NF-κB (Ma et al., 2011; Boldin and Baltimore, 2012).
Interestingly, in the NanoString platform, which does not amplify transcripts prior to their measurement, we were unable to detect significant differential expression of miR-155 following IL-1β stimulation, suggesting that, although induced by cytokine treatment the absolute abundance of this miRNA is quite low. When we analyzed miRNA expression using amplification-based qRT-PCR, we noted that, while the primary/precursor transcripts for miR-146a and miR-155 were substantially upregulated by IL-1β incubation, there was no significant induction of the corresponding mature miRNAs. These data further indicate that the regulation of miRNAs and their modulation of cytokine-induced gene expression can be quite modest (O’Neill et al., 2011; Ibrahim et al., 2016).
One of the most significantly downregulated miRNAs in IL-1β-treated cervical stromal cells was miR-143. This regulatory RNA transcript has been shown to target the COX-2 mRNA, suggesting that expression of this RNA in the basal state may prevent COX-2-mediated inflammatory prostaglandin biosynthesis (Kim et al., 2011; Pham et al., 2013).
Among the transcripts that were upregulated by cytokine but unaffected by progestin were the bradykinin receptors (BDKRB1 and BDKRB2). Bradykinin is a nonapeptide (H2N–Arg–Pro–Pro–Gly–Phe–Ser–Pro–Phe–Arg–COOH) member of the kallikrein–kinin system (Burch et al., 1989) that is generated following the activation of plasma or tissue kallikrein and subsequent cleavage of kininogen into several smaller products (Moreau et al., 2005; Bryant and Shariat-Madar, 2009; Bjorkqvist et al., 2013). Bradykinin is implicated in several pathophysiological events, including coagulation (Del Rosso et al., 2011; Wu, 2015; Weidmann et al., 2017) and thrombosis, fibrinolysis (Del Rosso et al., 2008), and acute inflammation (Kaplan and Joseph, 2014; Wu, 2015; Schmaier, 2016). Among the physiological functions of bradykinin is vascular permeability (Bjorkqvist et al., 2013; Couture et al., 2014) and smooth-muscle contractility (Ricciardolo et al., 2018). It is possible that bradykinin, acting via BDKR-B1 and/or -B2, mediates increased tissue hydration in the cervix during ripening by altering endothelial cell junctions (Couture et al., 2014). HAS-2, the enzyme that catalyzes the synthesis of hyaluronan (hydrophilic GAG) that accumulates in the stroma during ripening, is upregulated by IL-1β, and the induction is attenuated by P4. Thus, HAS-2- and BDKR-mediated functions of bradykinin may act in concert to orchestrate the softening that occurs during cervical ripening in preparation for parturition. To our knowledge, the current work is the first report of cytokine-induced bradykinin receptor expression in the cervical stroma. While P4 had no effect on IL-1β induction of BDKR-B1 or -B2, HAS-2 transcripts were nearly abolished when IL-1β-treated cells were co-incubated with P4. This observation provides a means by which progestins may prevent untimely hyaluronan synthesis and enhanced tissue hydration in the cervical stroma.
The regulation of ECM composition is a major determinant of the biomechanical features of cervical ripening in preparation for the onset of labor (Myers et al., 2008, 2009; House et al., 2009). During the initial phases of cervical remodeling in early pregnancy, collagens type I and III are highly organized and cross-linked via lysyl oxidase and lysyl hydroxylase (Hassan et al., 2009; Akins et al., 2011). This provides a rigid and closed cervix that resists the gravitational and contractile forces that would otherwise contribute to opening of the cervical os and premature delivery of the fetus (Danforth, 1983; Hao et al., 2018). Later in pregnancy, cross-linked collagens are replaced by randomly oriented fibers, increased hyaluronan accumulation, and tissue hydration (Garantziotis and Savani, 2019). Our in vitro studies demonstrated that treatment of cells with IL-1β downregulated collagen I and III and lysyl oxidase mRNA expression (Supplementary Table S1). Moreover, cytokine exposure of cervical stromal cells led to upregulated HAS-2 expression that was antagonized by simultaneous incubation with P4. These results are consistent with previous findings by Elovitz’s group that progestational agents act to maintain cervical integrity in a murine model of parturition (Xu et al., 2008).
We also showed that IL-1β caused the upregulation of several MMPs (i.e., MMP1/interstitial collagenase, 3/stromelysin 1, MMP10/stromelysin 2, and MMP12/macrophage elastase) by ≥five-fold. These data are consistent with previous reports using human cervical fibroblasts (Yoshida et al., 2002). Combined incubation of stromal cells with IL-1β and P4 resulted in ∼ 60% reduction in MMP10 mRNA expression (see Figure 4 and Supplementary Tables S1, S9). In contrast, co-incubation of cells with cytokine and progestin led to less pronounced diminution in MMP1 (∼15%) and MMP3 (∼42%) and no effect on MMP12 expression. These data suggest that P4 has minimal effects on the key proteinases involved in tissue remodeling in the cervix.
We also detected the upregulation of several GAG synthases (i.e., B4GALT1, CHST11, EXT1, 3GAL1, FUT8, HS3STB1, and ST3) by IL-1β which was largely unaffected by P4. Importantly, however, IL-1β-induced HAS-2 mRNA expression was strongly inhibited by P4 co-incubation (see Figure 4), consistent with a previous report by Uchiyama et al. (2005). Taken together, these data indicate that, while inflammatory cytokines have wide-ranging effects on molecules involved in ECM biosynthesis and turnover, progestin treatment has a modest effect on these functions.
The expression of 11β-HSD1, the enzyme that converts biologically inert cortisone into bioactive cortisol in several tissues, was induced several folds in stromal cells incubated with IL-1β. In addition, P4 treatment alone led to a 3-5-fold increase in 11β-HSD1 mRNA abundance, while 11β-HSD2, the enzyme that inactivates cortisol to cortisone, was unaffected by either cytokine or progestin exposure (data not shown) (Seckl, 2004). Surprisingly, when we combined exposure to IL-1β and P4 in cervical stromal cells, we detected a very robust synergistic upregulation of the 11β-HSD1 gene and no change in 11β-HSD2 gene expression. Previous studies have reported that proinflammatory cytokines can induce 11β-HSD1 leading to local synthesis of cortisol (Chapman K. et al., 2013). Chapman and Seckl have suggested that local expression of 11β-HSD1 and conversion of cortisone to cortisol provides a means to dampen acute inflammation that could lead to chronic tissue injury if left unchecked (Seckl, 2004; Chapman et al., 2006; Chapman K.E. et al., 2013). Ahasan et al. (2012) reported that IL-1β caused upregulation of 11β-HSD1 as an anti-inflammatory response in mesenchymal stromal cells. Similarly, Hardy et al. (2008, 2016) demonstrated upregulation of 11β-HSD1 mRNA expression in acute inflammation in skeletal muscle and in the context of inflamed synovial tissue with patients with rheumatoid arthritis (Hardy et al., 2008).
The current work has demonstrated that incubation of cervical stromal fibroblasts with a proinflammatory cytokine leads to a robust global gene expression profile that includes predicted inflammatory and anti-inflammatory transcripts (e.g., cytokines, chemokines, enzymes involved in bioactive lipid synthesis, signaling proteins, and transcription factors). In addition, we also demonstrated several other groups of genes expressed in response to IL-1β, including ECM proteins and ECM-modifying enzymes (e.g., matricellular proteins, MMPs, other proteases, and glycosyltransferases driving the biosynthesis of GAGs). Finally, several non-coding RNAs were identified, including miRNAs and LINCRNAs.
A major objective of the study was to examine the role played by progesterone in governing cytokine-driven gene expression. In this regard, we were somewhat surprised to observe that a relatively modest cohort of stromal cell transcripts was regulated either positively or negatively by progestin. P4 was found to inhibit only a subset of stromal cell genes following IL-1β treatment, suggesting that this hormone exerts only partial anti-inflammatory activity, at least in the cervical stroma. For example, while P4 completely abolished cytokine-mediated IL-6 mRNA expression, it was ineffective at inhibiting CXCL8/IL-8 gene expression.
Finally, this work reported for the first time that IL-1β and P4 conspired to upregulate a few genes, e.g., FKBP5, a steroid receptor chaperone and 11β-HSD1, the enzyme that converts inert cortisone into bioactive cortisol. In addition, we discovered for the first time that IL-1β upregulated the expression of bradykinin receptors B1 and B2 that mediate the actions of kallikrein products in vascular permeability and other events in the inflammatory cascade. These latter two observations open a new avenue for future investigations into the interaction of cytokines and progestins in governing the events leading to cervical ripening in preparation for parturition.
The microarray data is available at GEO (GSE154571).
The studies involving human participants were reviewed and approved by The Ohio State University Biomedical Institutional Review Board. The patients/participants provided their written informed consent to participate in this study.
DK conceived of the study, designed the experiments, analyzed the data, wrote the manuscript, and prepared the figures and tables. TS executed the experiments, assisted in data collection, and proofread the manuscript. All authors contributed to the article and approved the submitted version.
This work was supported by the March of Dimes Prematurity Research Collaborative of Ohio and The Ohio State University Perinatal Research and Development Fund.
The authors declare that the research was conducted in the absence of any commercial or financial relationships that could be construed as a potential conflict of interest.
We gratefully acknowledge the excellent technical assistance of members of The Ohio State University Comprehensive Cancer Center (OSUCCC) Genomics Shared Resource who contributed to the microarray and mature high-dimensional miRNA profiling studies described herein. Specifically, we thank Sarah Warner for performing the Affymetrix microarray studies and Dr. Paolo Fadda for performing the NanoString miRNA profiling assays. We also thank the Comprehensive Cancer Center Human Tissue Cooperative Network for their assistance in procuring cervical tissue specimens. Following the embargo during the publication process, the gene expression database will be deposited in GenBank at the National Center for Biotechnology Information.
The Supplementary Material for this article can be found online at: https://www.frontiersin.org/articles/10.3389/fgene.2020.00883/full#supplementary-material
Ackerman, W. E. IV, Buhimschi, I. A., Eidem, H. R., Rinker, D. C., Rokas, A., Rood, K., et al. (2016a). Comprehensive RNA profiling of villous trophoblast and decidua basalis in pregnancies complicated by preterm birth following intra-amniotic infection. Placenta 44, 23–33. doi: 10.1016/j.placenta.2016.05.010
Ackerman, W. E. IV, Summerfield, T. L., Mesiano, S., Schatz, F., Lockwood, C. J., and Kniss, D. A. (2016b). Agonist-dependent downregulation of progesterone receptors in human cervical stromal fibroblasts. Reprod. Sci. 23, 112–123. doi: 10.1177/1933719115597787
Ackerman, W. E. IV, Robinson, J. M., and Kniss, D. A. (2005). Despite transcriptional and functional coordination, cyclooxygenase-2 and microsomal prostaglandin E synthase-1 largely reside in distinct lipid microdomains in WISH epithelial cells. J. Histochem. Cytochem. 53, 1391–1401. doi: 10.1369/jhc.5a6710.2005
Ahasan, M. M., Hardy, R., Jones, C., Kaur, K., Nanus, D., Juarez, M., et al. (2012). Inflammatory regulation of glucocorticoid metabolism in mesenchymal stromal cells. Arthritis Rheum. 64, 2404–2413.
Akgul, Y., Holt, R., Mummert, M., Word, A., and Mahendroo, M. (2012). Dynamic changes in cervical glycosaminoglycan composition during normal pregnancy and preterm birth. Endocrinology 153, 3493–3503. doi: 10.1210/en.2011-1950
Akgul, Y., Word, R. A., Ensign, L. M., Yamaguchi, Y., Lydon, J., Hanes, J., et al. (2014). Hyaluronan in cervical epithelia protects against infection-mediated preterm birth. J. Clin. Invest. 124, 5481–5489. doi: 10.1172/jci78765
Akins, M. L., Luby-Phelps, K., Bank, R. A., and Mahendroo, M. (2011). Cervical softening during pregnancy: regulated changes in collagen cross-linking and composition of matricellular proteins in the mouse. Biol. Reprod. 84, 1053–1062. doi: 10.1095/biolreprod.110.089599
Amini, P., Wilson, R., Wang, J., Tan, H., Yi, L., Koeblitz, W. K., et al. (2019). Progesterone and cAMP synergize to inhibit responsiveness of myometrial cells to pro-inflammatory/pro-labor stimuli. Mol. Cell. Endocrinol. 479, 1–11. doi: 10.1016/j.mce.2018.08.005
Baltimore, D., Boldin, M. P., O’Connell, R. M., Rao, D. S., and Taganov, K. D. (2008). MicroRNAs: new regulators of immune cell development and function. Nat. Immunol. 9, 839–845. doi: 10.1038/ni.f.209
Begum, N. A., Kobayashi, M., Moriwaki, Y., Matsumoto, M., Toyoshima, K., and Seya, T. (2002). Mycobacterium bovis BCG cell wall and lipopolysaccharide induce a novel gene, BIGM103, encoding a 7-TM protein: identification of a new protein family having Zn-transporter and Zn-metalloprotease signatures. Genomics 80, 630–645. doi: 10.1006/geno.2002.7000
Bjorkqvist, J., Jamsa, A., and Renne, T. (2013). Plasma kallikrein: the bradykinin-producing enzyme. Thromb. Haemost. 110, 399–407. doi: 10.1160/th13-03-0258
Boldin, M. P., and Baltimore, D. (2012). MicroRNAs, new effectors and regulators of NF-kappaB. Immunol. Rev. 246, 205–220. doi: 10.1111/j.1600-065x.2011.01089.x
Bryant, J. W., and Shariat-Madar, Z. (2009). Human plasma kallikrein-kinin system: physiological and biochemical parameters. Cardiovasc. Hematol. Agents Med. Chem. 7, 234–250. doi: 10.2174/187152509789105444
Burch, R. M., Connor, J. R., and Tiffany, C. W. (1989). The kallikrein-kininogen-kinin system in chronic inflammation. Agents Actions 27, 258–260. doi: 10.1007/bf01972790
Cakmak, H., Schatz, F., Huang, S. T., Buchwalder, L., Rahman, M., Arici, A., et al. (2005). Progestin suppresses thrombin- and interleukin -induced interleukin-11 production in term decidual cells: implications for preterm delivery. J. Clin. Endocrinol. Metab. 90, 5279–5286. doi: 10.1210/jc.2005-0210
Ceppi, M., Pereira, P. M., Dunand-Sauthier, I., Barras, E., and Reith, W. (2009). MicroRNA-155 modulates the interleukin-1 signaling pathway in activated human monocyte-derived dendritic cells. Proc. Natl. Acad. Sci. U.S.A. 106, 2735–2740. doi: 10.1073/pnas.0811073106
Chapman, K., Holmes, M., and Seckl, J. (2013). 11β−hydroxysteroid dehydrogenases: intracellular gate-keepers of tissue glucocorticoid action. Physiol. Rev. 93, 1139–1206. doi: 10.1152/physrev.00020.2012
Chapman, K. E., Coutinho, A., Gray, M., Gilmour, J. S., Savill, J. S., and Seckl, J. R. (2006). Local amplification of glucocorticoids by 11 hydroxysteroid dehydrogenase type 1 and its role in the inflammatory response. Ann. N. Y. Acad. Sci. 1088, 265–273. doi: 10.1196/annals.1366.030
Chapman, K. E., Coutinho, A. E., Zhang, Z., Kipari, T., Savill, J. S., and Seckl, J. R. (2013). Changing glucocorticoid action: 11β−hydroxysteroid dehydrogenase type 1 in acute and chronic inflammation. J. Steroid Biochem. Mol. Biol. 137, 82–92. doi: 10.1016/j.jsbmb.2013.02.002
Chen, G. I., Tisayakorn, S., Jorgensen, C., D’Ambrosio, L. M., Goudreault, M., and Gingras, A. C. (2008). PP4R4/KIAA1622 forms a novel stable cytosolic complex with phosphoprotein phosphatase 4. J. Biol. Chem. 283, 29273–29284. doi: 10.1074/jbc.m803443200
Christenson, L. K., Gunewardena, S., Hong, X., Spitschak, M., Baufeld, A., and Vanselow, J. (2013). Research resource: preovulatory LH surge effects on follicular theca and granulosa transcriptomes. Mol. Endocrinol. 27, 1153–1171. doi: 10.1210/me.2013-1093
Chwalisz, K., Benson, M., Scholz, P., Daum, J., Beier, H. M., and Hegele-Hartung, C. (1994). Cervical ripening with the cytokines interleukin 8, interleukin 1 beta and tumour necrosis factor alpha in guinea-pigs. Hum. Reprod. 9, 2173–2181. doi: 10.1093/oxfordjournals.humrep.a138413
Conde-Agudelo, A., and Romero, R. (2016). Vaginal progesterone to prevent preterm birth in pregnant women with a sonographic short cervix: clinical and public health implications. Am. J. Obstet. Gynecol. 214, 235–242. doi: 10.1016/j.ajog.2015.09.102
Contreras, J., and Rao, D. S. (2012). MicroRNAs in inflammation and immune responses. Leukemia 26, 404–413. doi: 10.1038/leu.2011.356
Couture, R., Blaes, N., and Girolami, J. P. (2014). Kinin receptors in vascular biology and pathology. Curr. Vasc. Pharmacol. 12, 223–248. doi: 10.2174/1570161112666140226121627
Danforth, D. N. (1983). The morphology of the human cervix. Clin. Obstet. Gynecol. 26, 7–13. doi: 10.1097/00003081-198303000-00005
Del Rosso, M., Fibbi, G., Pucci, M., Margheri, F., and Serrati, S. (2008). The plasminogen activation system in inflammation. Front. Biosci. 13, 4667–4686. doi: 10.2741/3032
Del Rosso, M., Margheri, F., Serrati, S., Chilla, A., Laurenzana, A., and Fibbi, G. (2011). The urokinase receptor system, a key regulator at the intersection between inflammation, immunity, and coagulation. Curr. Pharm. Des. 17, 1924–1943. doi: 10.2174/138161211796718189
Duan, J. J., Cai, J., Guo, Y. F., Bian, X. W., and Yu, S. C. (2016). ALDH1A3, a metabolic target for cancer diagnosis and therapy. Int. J. Cancer 139, 965–975. doi: 10.1002/ijc.30091
Dubicke, A., Ekman-Ordeberg, G., Mazurek, P., Miller, L., and Yellon, S. M. (2016). Density of stromal cells and macrophages associated with collagen remodeling in the human cervix in preterm and term birth. Reprod. Sci. 23, 595–603. doi: 10.1177/1933719115616497
Elovitz, M. A., and Mrinalini, C. (2004). Animal models of preterm birth. Trends Endocrinol Metab. 15, 479–487. doi: 10.1016/j.tem.2004.10.009
Garantziotis, S., and Savani, R. C. (2019). Hyaluronan biology: a complex balancing act of structure, function, location and context. Matrix Biol. 7, 1–10. doi: 10.1016/j.matbio.2019.02.002
Georgiou, E. X., Lei, K., Lai, P. F., Yulia, A., Herbert, B. R., Castellanos, M., et al. (2016). The study of progesterone action in human myometrial explants. Mol. Hum. Reprod. 22, 877–889. doi: 10.1093/molehr/gaw037
Gonzalez, J. M., Xu, H., Chai, J., Ofori, E., and Elovitz, M. A. (2009). Preterm and term cervical ripening in CD1 Mice (Mus musculus): similar or divergent molecular mechanisms? Biol. Reprod. 81, 1226–1232. doi: 10.1095/biolreprod.108.075309
Hao, J., Yao, W., Harris, W. R., Vink, J. Y., Myers, K., and Donnelly, E. (2018). Characterization of collagen microstructural organization of human cervical tissue. Reproduction 156, 71–79. doi: 10.1530/rep-17-0763
Hardy, R., Rabbitt, E. H., Filer, A., Emery, P., Hewison, M., Stewart, P. M., et al. (2008). Local and systemic glucocorticoid metabolism in inflammatory arthritis. Ann. Rheum. Dis. 67, 1204–1210. doi: 10.1136/ard.2008.090662
Hardy, R. S., Doig, C. L., Hussain, Z., O’Leary, M., Morgan, S. A., Pearson, M. J., et al. (2016). 11β−Hydroxysteroid dehydrogenase type 1 within muscle protects against the adverse effects of local inflammation. J. Pathol. 240, 472–483. doi: 10.1002/path.4806
Hassan, S. S., Romero, R., Tarca, A. L., Nhan-Chang, C. L., Vaisbuch, E., Erez, O., et al. (2009). The transcriptome of cervical ripening in human pregnancy before the onset of labor at term: identification of novel molecular functions involved in this process. J. Matern. Fetal Neonatal Med. 22, 1183–1193. doi: 10.3109/14767050903353216
Hassan, S. S., Romero, R., Vidyadhari, D., Fusey, S., Baxter, J. K., Khandelwal, M., et al. (2011). Vaginal progesterone reduces the rate of preterm birth in women with a sonographic short cervix: a multicenter, randomized, double-blind, placebo-controlled trial. Ultrasound Obstet. Gynecol. 38, 18–31. doi: 10.1002/uog.9017
Himes, B. E., Jiang, X., Wagner, P., Hu, R., Wang, Q., Klanderman, B., et al. (2014). RNA-Seq transcriptome profiling identifies CRISPLD2 as a glucocorticoid responsive gene that modulates cytokine function in airway smooth muscle cells. PLoS One 9:e99625. doi: 10.1371/journal.pone.0099625
House, M., Kaplan, D. L., and Socrate, S. (2009). Relationships between mechanical properties and extracellular matrix constituents of the cervical stroma during pregnancy. Semin. Perinatol. 33, 300–307. doi: 10.1053/j.semperi.2009.06.002
Huang, H., Jin, T., He, J., Ding, Q., Xu, D., Wang, L., et al. (2012). Progesterone and adipoQ receptor 11 links ras signaling to cardiac development in zebrafish. Arterioscler. Thromb. Vasc. Biol. 32, 2158–2170. doi: 10.1161/atvbaha.112.252775
Hubler, T. R., Denny, W. B., Valentine, D. L., Cheung-Flynn, J., Smith, D. F., and Scammell, J. G. (2003). The FK506-binding immunophilin FKBP51 is transcriptionally regulated by progestin and attenuates progestin responsiveness. Endocrinology 144, 2380–2387. doi: 10.1210/en.2003-0092
Hubler, T. R., and Scammell, J. G. (2004). Intronic hormone response elements mediate regulation of FKBP5 by progestins and glucocorticoids. Cell Stress Chaper. 9, 243–252.
Ibrahim, S. A., Ackerman, W. E. IV, Summerfield, T. L., Lockwood, C. J., Schatz, F., and Kniss, D. A. (2016). Inflammatory gene networks in term human decidual cells define a potential signature for cytokine-mediated parturition. Am. J. Obstet. Gynecol. 214, 284.e1–284.e47. doi: 10.1016/j.ajog.2015.08.075
Jeong, J. W., Lee, K. Y., Kwak, I., White, L. D., Hilsenbeck, S. G., Lydon, J. P., et al. (2005). Identification of murine uterine genes regulated in a ligand-dependent manner by the progesterone receptor. Endocrinology 146, 3490–3505. doi: 10.1210/en.2005-0016
Kaplan, A. P., and Joseph, K. (2014). Pathogenic mechanisms of bradykinin mediated diseases: dysregulation of an innate inflammatory pathway. Adv. Immunol. 121, 41–89. doi: 10.1016/b978-0-12-800100-4.00002-7
Kastner, P., Krust, A., Turcotte, B., Stropp, U., Tora, L., Gronemeyer, H., et al. (1990). Two distinct estrogen-regulated promoters generate transcripts encoding the two functionally different human progesterone receptor forms A and B. EMBO J. 9, 1603–1614. doi: 10.1002/j.1460-2075.1990.tb08280.x
Katayama, T., Nakao, M., and Takagi, T. (2010). TogoWS: integrated SOAP and REST APIs for interoperable bioinformatics Web services. Nucleic Acids Res. 38, W706–W711.
Kim, S. Y., Romero, R., Tarca, A. L., Bhatti, G., Lee, J., Chaiworapongsa, T., et al. (2011). miR-143 regulation of prostaglandin-endoperoxidase synthase 2 in the amnion: implications for human parturition at term. PLoS One 6:e24131. doi: 10.1371/journal.pone.0024131
Kniss, D. A. (1999). Cyclooxygenases in reproductive medicine and biology. J. Soc. Gynecol. Invest. 6, 285–292. doi: 10.1016/s1071-5576(99)00034-9
Kobayashi, K., Hernandez, L. D., Galan, J. E., Janeway, C. A. Jr., Medzhitov, R., and Flavell, R. A. (2002). IRAK-M is a negative regulator of Toll-like receptor signaling. Cell 110, 191–202. doi: 10.1016/s0092-8674(02)00827-9
Kozomara, A., and Griffiths-Jones, S. (2014). miRBase: annotating high confidence microRNAs using deep sequencing data. Nucleic Acids Res. 42, D68–D73.
Kuroda, K., Venkatakrishnan, R., Salker, M. S., Lucas, E. S., Shaheen, F., Kuroda, M., et al. (2013). Induction of 11beta-HSD 1 and activation of distinct mineralocorticoid receptor- and glucocorticoid receptor-dependent gene networks in decidualizing human endometrial stromal cells. Mol. Endocrinol. 27, 192–202. doi: 10.1210/me.2012-1247
Li, R., Ackerman, W. E. IV, Summerfield, T. L., Yu, L., Gulati, P., Zhang, J., et al. (2011). Inflammatory gene regulatory networks in amnion cells following cytokine stimulation: translational systems approach to modeling human parturition. PLoS One 6:e20560. doi: 10.1371/journal.pone.0020560
Li, W., and He, F. (2014). Monocyte to macrophage differentiation-associated (MMD) targeted by miR-140-5p regulates tumor growth in non-small cell lung cancer. Biochem. Biophys. Res. Commun. 450, 844–850. doi: 10.1016/j.bbrc.2014.06.075
Lieberman, B. A., Bona, B. J., Edwards, D. P., and Nordeen, S. K. (1993). The constitution of a progesterone response element. Mol. Endocrinol. 7, 515–527. doi: 10.1210/me.7.4.515
Liu, M. J., Bao, S., Galvez-Peralta, M., Pyle, C. J., Rudawsky, A. C., Pavlovicz, R. E., et al. (2013). ZIP8 regulates host defense through zinc-mediated inhibition of NF-kappaB. Cell Rep. 3, 386–400. doi: 10.1016/j.celrep.2013.01.009
Liu, Q., Zheng, J., Yin, D. D., Xiang, J., He, F., Wang, Y.-C., et al. (2012). Monocyte to macrophage differentiation-associated (MMD) positively regulates ERK and Akt activation and TNF-alpha and NO production in macrophages. Mol. Biol. Rep. 39, 5643–5650. doi: 10.1007/s11033-011-1370-5
Ma, X., Becker Buscaglia, L. E., Barker, J. R., and Li, Y. (2011). MicroRNAs in NF-kappaB signaling. J. Mol. Cell Biol. 3, 159–166.
Madden, S. F., Carpenter, S. B., Jeffery, I. B., Bjorkbacka, H., Fitzgerald, K. A., O’Neill, L. A., et al. (2010). Detecting microRNA activity from gene expression data. BMC Bioinformatics 11:257. doi: 10.1186/1471-2105-11-257
Malmstrom, E., Sennstrom, M., Holmberg, A., Frielingsdorf, H., Eklund, E., Malmstrom, L., et al. (2007). The importance of fibroblasts in remodelling of the human uterine cervix during pregnancy and parturition. Mol. Hum. Reprod. 13, 333–341. doi: 10.1093/molehr/gal117
Mathelier, A., Zhao, X., Zhang, A. W., Parcy, F., Worsley-Hunt, R., Arenillas, D. J., et al. (2014). JASPAR 2014: an extensively expanded and updated open-access database of transcription factor binding profiles. Nucleic Acids Res. 42, D142–D147.
Mendelson, C. R. (2009). Minireview: fetal-maternal hormonal signaling in pregnancy and labor. Mol. Endocrinol. 23, 947–954. doi: 10.1210/me.2009-0016
Merico, D., Isserlin, R., Stueker, O., Emili, A., and Bader, G. D. (2010). Enrichment map: a network-based method for gene-set enrichment visualization and interpretation. PLoS One 5:e13984. doi: 10.1371/journal.pone.0013984
Moreau, M. E., Garbacki, N., Molinaro, G., Brown, N. J., Marceau, F., and Adam, A. (2005). The kallikrein-kinin system: current and future pharmacological targets. J. Pharmacol. Sci. 99, 6–38. doi: 10.1254/jphs.srj05001x
Myers, K., Socrate, S., Tzeranis, D., and House, M. (2009). Changes in the biochemical constituents and morphologic appearance of the human cervical stroma during pregnancy. Eur. J. Obstet. Gynecol. Reprod. Biol. 144, (Suppl. 1), S82–S89.
Myers, K. M., Paskaleva, A. P., House, M., and Socrate, S. (2008). Mechanical and biochemical properties of human cervical tissue. Acta Biomater. 4, 104–116. doi: 10.1016/j.actbio.2007.04.009
Nelson, C. C., Hendy, S. C., Shukin, R. J., Cheng, H., Bruchovsky, N., Koop, B. F., et al. (1999). Determinants of DNA sequence specificity of the androgen, progesterone, and glucocorticoid receptors: evidence for differential steroid receptor response elements. Mol. Endocrinol. 13, 2090–2107. doi: 10.1210/mend.13.12.0396
O’Connell, R. M., Taganov, K. D., Boldin, M. P., Cheng, G., and Baltimore, D. (2007). MicroRNA-155 is induced during the macrophage inflammatory response. Proc. Natl. Acad. Sci. U.S.A. 104, 1604–1609. doi: 10.1073/pnas.0610731104
O’Neill, L. A., Sheedy, F. J., and McCoy, C. E. (2011). MicroRNAs: the fine-tuners of Toll-like receptor signalling. Nat. Rev. Immunol. 11, 163–175. doi: 10.1038/nri2957
Oppermann, U. C., Persson, B., and Jornvall, H. (1997). Function, gene organization and protein structures of 11beta-hydroxysteroid dehydrogenase isoforms. Eur. J. Biochem. 249, 355–360. doi: 10.1111/j.1432-1033.1997.t01-1-00355.x
Panchatcharam, M., Salous, A. K., and Brandon, J. (2014). Mice with targeted inactivation of ppap2b in endothelial and hematopoietic cells display enhanced vascular inflammation and permeability. Arterioscler. Thromb. Vasc. Biol. 34, 837–845. doi: 10.1161/atvbaha.113.302335
Pham, H., Rodriguez, C. E., Donald, G. W., Hertzer, K. M., Jung, X. S., Chang, H. H., et al. (2013). miR-143 decreases COX-2 mRNA stability and expression in pancreatic cancer cells. Biochem. Biophys. Res. Commun. 439, 6–11. doi: 10.1016/j.bbrc.2013.08.042
Quinn, S. R., and O’Neill, L. A. (2011). A trio of microRNAs that control Toll-like receptor signalling. Int. Immunol. 23, 421–425. doi: 10.1093/intimm/dxr034
Rehli, M., Krause, S. W., Schwarzfischer, L., Kreutz, M., and Andreesen, R. (1995). Molecular cloning of a novel macrophage maturation-associated transcript encoding a protein with several potential transmembrane domains. Biochem. Biophys. Res. Commun. 217, 661–667. doi: 10.1006/bbrc.1995.2825
Reiner, A., Yekutieli, D., and Benjamini, Y. (2003). Identifying differentially expressed genes using false discovery rate controlling procedures. Bioinformatics 19, 368–375. doi: 10.1093/bioinformatics/btf877
Ricciardolo, F. L. M., Folkerts, G., Folino, A., and Mognetti, B. (2018). Bradykinin in asthma: modulation of airway inflammation and remodelling. Eur. J. Pharmacol. 827, 181–188. doi: 10.1016/j.ejphar.2018.03.017
Robinson, M. D., McCarthy, D. J., and Smyth, G. K. (2010). edgeR: a Bioconductor package for differential expression analysis of digital gene expression data. Bioinformatics 26, 139–140. doi: 10.1093/bioinformatics/btp616
Rubel, C. A., Lanz, R. B., Kommagani, R., Franco, H. L., Lydon, J. P., and DeMayo, F. J. (2012). Research resource: genome-wide profiling of progesterone receptor binding in the mouse uterus. Mol. Endocrinol. 26, 1428–1442. doi: 10.1210/me.2011-1355
Sakamoto, Y., Moran, P., Bulmer, J. N., Searle, R. F., and Robson, S. C. (2005). Macrophages and not granulocytes are involved in cervical ripening. J. Reprod. Immunol. 66, 161–173. doi: 10.1016/j.jri.2005.04.005
Sato, T., Michizu, H., Hashizume, K., and Ito, A. (2001). Hormonal regulation of PGE2 and COX-2 production in rabbit uterine cervical fibroblasts. J. Appl. Physiol. 90, 1227–1231. doi: 10.1152/jappl.2001.90.4.1227
Schmaier, A. H. (2016). The contact activation and kallikrein/kinin systems: pathophysiologic and physiologic activities. J. Thromb. Haemost. 14, 28–39. doi: 10.1111/jth.13194
Schmittgen, T. D., and Livak, K. J. (2008). Analyzing real-time PCR data by the comparative C(T) method. Nat. Protoc. 3, 1101–1108. doi: 10.1038/nprot.2008.73
Seckl, J. R. (2004). 11β−hydroxysteroid dehydrogenases: changing glucocorticoid action. Curr. Opin. Pharmacol. 4, 597–602. doi: 10.1016/j.coph.2004.09.001
Seki, M., Kohno, S., Newstead, M. W., Zeng, X., Bhan, U., Lukacs, N. W., et al. (2010). Critical role of IL-1 receptor-associated kinase-M in regulating chemokine-dependent deleterious inflammation in murine influenza pneumonia. J. Immunol. 184, 1410–1418. doi: 10.4049/jimmunol.0901709
Shukla, V., Barnhouse, V., Ackerman, W. E. IV, Summerfield, T. L., Powell, H. M., Leight, J. L., et al. (2018). Cellular mechanics of primary human cervical fibroblasts: influence of progesterone and a pro-inflammatory cytokine. Ann. Biomed. Eng. 46, 197–207. doi: 10.1007/s10439-017-1935-0
Shynlova, O., Lee, Y. H., Srikhajon, K., and Lye, S. J. (2013). Physiologic uterine inflammation and labor onset: integration of endocrine and mechanical signals. Reprod. Sci. 20, 154–167. doi: 10.1177/1933719112446084
Stjernholm-Vladic, Y., Wang, H., Stygar, D., Ekman, G., and Sahlin, L. (2004). Differential regulation of the progesterone receptor A and B in the human uterine cervix at parturition. Gynecol. Endocrinol. 18, 41–46. doi: 10.1080/09513590310001651777
Storer, C. L., Dickey, C. A., Galigniana, M. D., Rein, T., and Cox, M. B. (2011). FKBP51 and FKBP52 in signaling and disease. Trends Endocrinol. Metab. 22, 481–490. doi: 10.1016/j.tem.2011.08.001
Subramanian, A., Tamayo, P., Mootha, V. K., Mukherjee, S., Ebert, B. L., Gillette, M. A., et al. (2005). Gene set enrichment analysis: a knowledge-based approach for interpreting genome-wide expression profiles. Proc. Natl. Acad. Sci. U.S.A. 102, 15545–15550. doi: 10.1073/pnas.0506580102
Thomas-Chollier, M., Hufton, A., Heinig, M., O’Keeffe, S., Masri, N. E., Roider, H. G., et al. (2011). Transcription factor binding predictions using TRAP for the analysis of ChIP-seq data and regulatory SNPs. Nat. Protoc. 6, 1860–1869. doi: 10.1038/nprot.2011.409
Tili, E., Michaille, J. J., Cimino, A., Costinean, S., Dumitru, C. D., Adair, B., et al. (2007). Modulation of miR-155 and miR-125b levels following lipopolysaccharide/TNF-alpha stimulation and their possible roles in regulating the response to endotoxin shock. J. Immunol. 179, 5082–5089. doi: 10.4049/jimmunol.179.8.5082
Timmons, B., Akins, M., and Mahendroo, M. (2010). Cervical remodeling during pregnancy and parturition. Trends Endocrinol. Metab. 21, 353–361. doi: 10.1016/j.tem.2010.01.011
Tomlinson, J. W., Walker, E. A., Bujalska, I. J., Draper, N., Lavery, G. G., Cooper, M. S., et al. (2004). 11β−hydroxysteroid dehydrogenase type 1: a tissue-specific regulator of glucocorticoid response. Endocr. Rev. 25, 831–866. doi: 10.1210/er.2003-0031
Trasino, S. E., Harrison, E. H., and Wang, T. T. (2007). Androgen regulation of aldehyde dehydrogenase 1A3 (ALDH1A3) in the androgen-responsive human prostate cancer cell line LNCaP. Exp. Biol. Med. 232, 762–771.
Uchiyama, T., Sakuta, T., and Kanayama, T. (2005). Regulation of hyaluronan synthases in mouse uterine cervix. Biochem. Biophys. Res. Commun. 327, 927–932. doi: 10.1016/j.bbrc.2004.12.092
Vink, J., and Feltovich, H. (2016). Cervical etiology of spontaneous preterm birth. Semin. Fetal Neonatal Med. 21, 106–112. doi: 10.1016/j.siny.2015.12.009
Wang, Z. Q., Xing, W. M., Fan, H. H., Wang, K.-S., Zhang, H.-K., Wang, Q.-W., et al. (2009). The novel lipopolysaccharide-binding protein CRISPLD2 is a critical serum protein to regulate endotoxin function. J. Immunol. 183, 6646–6656. doi: 10.4049/jimmunol.0802348
Weidmann, H., Heikaus, L., Long, A. T., Naudin, C., Schluter, H., and Renne, T. (2017). The plasma contact system, a protease cascade at the nexus of inflammation, coagulation and immunity. Biochim. Biophys. Acta Mol. Cell Res. 1864, 2118–2127. doi: 10.1016/j.bbamcr.2017.07.009
Word, R. A., Li, X. H., Hnat, M., and Carrick, K. (2007). Dynamics of cervical remodeling during pregnancy and parturition: mechanisms and current concepts. Semin. Reprod. Med. 25, 69–79.
Wu, C., Huang, R. T., Kuo, C. H., Kumar, S., Kim, C. W., Lin, Y.-C., et al. (2015). Mechanosensitive PPAP2B regulates endothelial responses to atherorelevant hemodynamic forces. Circ. Res. 117, e41–e53.
Xu, C., Ren, G., Cao, G., Chen, Q., Shou, P., Zheng, C., et al. (2013). miR-155 regulates immune modulatory properties of mesenchymal stem cells by targeting TAK1-binding protein 2. J. Biol. Chem. 288, 11074–11079. doi: 10.1074/jbc.m112.414862
Xu, H., Gonzalez, J. M., Ofori, E., and Elovitz, M. A. (2008). Preventing cervical ripening: the primary mechanism by which progestational agents prevent preterm birth? Am. J. Obstet. Gynecol. 198, 314–318.
Yellon, S. M. (2017). Contributions to the dynamics of cervix remodeling prior to term and preterm birth. Biol. Reprod. 96, 13–23. doi: 10.1095/biolreprod.116.142844
Yong, P. Y., Harlow, C., Thong, K. J., and Hillier, S. G. (2002). Regulation of 11beta-hydroxysteroid dehydrogenase type 1 gene expression in human ovarian surface epithelial cells by interleukin-1. Hum. Reprod. 17, 2300–2306. doi: 10.1093/humrep/17.9.2300
Yoo, J. Y., Shin, H., Kim, T. H., Choi, W.-S., Ferguson, S. D., Fazleabas, A. T., et al. (2014). CRISPLD2 is a target of progesterone receptor and its expression is decreased in women with endometriosis. PLoS One 9:e100481. doi: 10.1371/journal.pone.100481
Yoshida, M., Sagawa, N., Itoh, H., Yura, S., Takemura, M., Wada, Y., et al. (2002). Prostaglandin F, cytokines and cyclic mechanical stretch augment matrix metalloproteinase-1 secretion from cultured human uterine cervical fibroblast cells. Mol. Hum. Reprod. 8, 681–687. doi: 10.1093/molehr/8.7.681
Keywords: cervix, inflammation, microRNA, preterm birth, transcriptomics
Citation: Kniss DA and Summerfield TL (2020) Progesterone Receptor Signaling Selectively Modulates Cytokine-Induced Global Gene Expression in Human Cervical Stromal Cells. Front. Genet. 11:883. doi: 10.3389/fgene.2020.00883
Received: 24 December 2019; Accepted: 17 July 2020;
Published: 11 September 2020.
Edited by:
Babatunde Ogunnaike, University of Delaware, United StatesReviewed by:
Georges Nemer, American University of Beirut, LebanonCopyright © 2020 Kniss and Summerfield. This is an open-access article distributed under the terms of the Creative Commons Attribution License (CC BY). The use, distribution or reproduction in other forums is permitted, provided the original author(s) and the copyright owner(s) are credited and that the original publication in this journal is cited, in accordance with accepted academic practice. No use, distribution or reproduction is permitted which does not comply with these terms.
*Correspondence: Douglas A. Kniss, a25pc3MuMUBvc3UuZWR1
Disclaimer: All claims expressed in this article are solely those of the authors and do not necessarily represent those of their affiliated organizations, or those of the publisher, the editors and the reviewers. Any product that may be evaluated in this article or claim that may be made by its manufacturer is not guaranteed or endorsed by the publisher.
Research integrity at Frontiers
Learn more about the work of our research integrity team to safeguard the quality of each article we publish.