- 1Institute of Ecology and Geobotany, Yunnan University, Kunming, China
- 2CAS Key Laboratory for Plant Diversity and Biogeography of East Asia, Kunming Institute of Botany, Chinese Academy of Sciences, Kunming, China
- 3Department of Botany and Plant Sciences, University of California, Riverside, Riverside, CA, United States
- 4CAS Key Laboratory of Plant Germplasm Enhancement and Specialty Agriculture, Wuhan Botanical Garden, Chinese Academy of Sciences, Wuhan, China
- 5Yunnan Key Laboratory for Integrative Conservation of Plant Species with Extremely Small Population, Kunming Institute of Botany, Chinese Academy of Sciences, Kunming, China
Most species of Santalales (the sandalwood order) are hemiparasites, including both facultative and obligate hemiparasites. Despite its rich diversity, only a small fraction of the species in the sandalwood order have sequenced plastomes. The evolution of parasitism–associated plastome reduction in Santalales remains under-studied. Here, we report the complete plastomes of three facultative hemiparasites (Pyrularia edulis, Cervantesiaceae; Osyris wightiana, and Santalum album, Santalaceae), and two obligate hemiparasites (Viscum liquidambaricolum and Viscum ovalifolium, Viscaceae). Coupled with publicly available data, we investigated the dynamics of plastome degradation in Santalales hemiparasites. Our results indicate that these hemiparasites can be characterized by various degrees of plastome downsizing, structural rearrangement, and gene loss. The loss or pseudogenization of ndh genes was commonly observed in Santalales hemiparasites, which may be correlated to the lifestyle shift from photoautotroph to hemiparasitism. However, the obligate hemiparasites did not exhibit a consistently higher level of gene loss or pseudogenization compared to facultative hemiparasites, which suggests that the degree of plastome reduction is not correlated with the trophic level facultative or obligate hemiparasitism. Instead, closely related taxa tend to possess highly similar plastome size, structure, and gene content. This implies the parasitism-associated plastome degradation in Santalales may evolve in a lineage-specific manner.
Introduction
Chloroplast, which possess an independent genome (the plastome), is the key organelle in plant cells for photosynthesis and carbon assimilation, as well as the biosynthetic pathways of starch, fatty acids, pigments, and amino acids (Palmer, 1985; Neuhaus and Emes, 2000; Daniell et al., 2016). Due to the essential roles of chloroplast in green plants, their plastomes are characterized by high conservatism in terms of genome size, structure, gene content, and organization (Daniell et al., 2016). A typical angiosperm plastome encodes 113 unique genes arranged in a quadripartite structure, consisting of a large single copy (LSC, 80–90 kbp) region and a small single copy (SSC, 16–27 kbp) region separated by a pair of inverted repeats (IRs, 20–30 kbp) regions (Wicke et al., 2011). Nevertheless, the lifestyle transition from autotrophy to parasitism leads to varying degrees of plastome modification, including decreasing size, physical or functional loss of genes, and structural rearrangement (reviewed by Neuhaus and Emes, 2000; Wicke and Naumann, 2018). As a result, the plastomes of parasitic plants are significantly divergent from their autotrophic relatives (Krause, 2008; Wicke et al., 2013, 2016; Petersen et al., 2015; Frailey et al., 2018; Schneider et al., 2018; Shin and Lee, 2018; Wicke and Naumann, 2018).
There are approximately 4,500 parasitic species documented in 20 families of flowering plants (Nickrent, 1997, 2002; Heide–Jørgensen, 2008), and parasitism likely has evolved at least 11 or 12 times in angiosperms (Barkman et al., 2007; Westwood et al., 2010). Hemiparasitic plants, different from holoparasitic plants (non-photosynthetic parasites) that obtain all nutrition and energy from the host plants, comprise more than 90% of plant parasites and are capable of photosynthesis (Nickrent, 1997; Heide–Jørgensen, 2008; Tešitel, 2016). They hence display a lower degree of plastome degradation than holoparasitic plants, and their plastomes are often within the size range of regular angiosperm plastomes (Wicke and Naumann, 2018).
Hemiparasitic plants can be further divided into facultative and obligate hemiparasites: an obligate cannot survive without exploiting a host, while a facultative does not rely on its host throughout its entire life cycle (Nickrent, 1997; Heide–Jørgensen, 2008). A previous study revealed that facultative hemiparasites may have relatively slight plastome degradation compared with obligate hemiparasites (Petersen et al., 2015). However, the currently available sequenced plastomes of hemiparasitic plants only represent a small fraction of the species diversity. Thus, it remains uncharacterized to what extent their plastomes are divergent, and whether facultative/obligate parasitism is correlated with different levels of plastome degradation.
Comparative and phylogenetic analysis of parasitic plastomes can provide insight into the evolutionary reduction concomitant with the lifestyle shift from autotrophy to parasitism (Wicke et al., 2016). Under a phylogenetic framework, reconstructing an evolutionary pathway of plastome degradation in relation to parasitism becomes feasible (Wicke and Naumann, 2018). The sandalwood order (Santalales) consists of 18 families, approximately 160 genera and 2,200 species (Nickrent et al., 2010; Su et al., 2015), which cover all lifestyles from autotrophy to hemiparasitism and holoparasitism (Nickrent, 1997, 2002; Der and Nickrent, 2008; Su et al., 2015). Most species in Santalales are hemiparasites, including both facultative and obligate (Nickrent, 1997, 2002; Heide–Jørgensen, 2008). Therefore, it provides an ideal system to investigate the reductive plastome evolution in hemiparasitic plants.
In this study, we sequenced and assembled the complete plastomes of three facultative hemiparasites: Pyrularia edulis (Cervantesiaceae), Osyris wightiana, and Santalum album (Santalaceae), and two obligate hemiparasites: Viscum liquidambaricolum, and Viscum ovalifolium (Viscaceae) in Santalales using a genome skimming approach (Straub et al., 2012). We aim to (1) characterize the newly sequenced plastomes, (2) determine structural shifts, gene loss and/or pseudogenization events in hemiparasites by comparing with autotrophic lineages, and (3) investigate the extent and progression of the plastome reduction in both facultative and obligate hemiparasites.
Materials and Methods
Plant Sampling, DNA Extraction, and Illumina Sequencing
Specimen and leaf tissues of P. edulis, O. wightiana, S. album, V. liquidambaricolum, and V. ovalifolium were sampled with vouchers deposited in the herbarium of Kunming Institute of Botany, CAS (information see Supplementary Table S1). Genomic DNA was extracted from ca. 50 mg of silica gel dried leaves using a modified CTAB method (Doyle and Doyle, 1987). Purified DNA was sheared to fragments with an average length of 350 bp, followed by ligation of adaptors for library amplification according to the manufacturer’s guideline (Illumina, San Diego, CA, United States). The shotgun library for each species was sequenced using a 2 × 150 Illumina HiSeq 2500 system at BGI (Wuhan, Hubei, China).
Plastome Assembly, Annotation and Comparison
Original shotgun reads were filtered using the FASTX–Toolkit1 to remove adaptors and reads with ambiguous bases. Both reference-based and de novo strategies were employed to assemble the plastomes. Firstly, the clean reads were assembled using CLC genome assembler v. 4.0β (CLC Inc., Aarhus, Denmark) with default parameter setting. The plastome of Osyris alba was used as reference to P. edulis, O. wightiana and S. album, and the plastome of Viscum album was selected as reference to both V. ovalifolium and V. liquidambaricolum. Genbank accessions of the reference plastomes are provided in Supplementary Table S2. All contigs were aligned to the reference by BLAST searches. The plastid contigs were organized according to the reference and connected with overlapping terminal sequences to yield the entire plastome. Secondly, the program NOVOPlasty v2.7.0 (Dierckxsens et al., 2017) was used for de novo assembly of plastomes with the k–mer size set at 31. The plastid rbcL gene of Taxillus chinensis (KY996492) was used as the seed to recover the plastomes. The plastomes of a given species recovered from different assembly strategies were compared using Geneious V10.2 (Kearse et al., 2012) to validate the accuracy of assembly.
Annotation of plastid genes were performed with Dual Organellar Genome Annotator database (Wyman et al., 2004). All protein-coding genes were checked with a BLAST search against the NCBI protein database. The protein-coding genes with one or more frame shift mutations or premature stop codons were annotated as pseudogenes. Start and stop codons and intron/exon boundaries for protein-coding genes were checked manually. Genes putatively annotated as transfer RNA (tRNA) were further verified by tRNAscan-SE 1.21 (Schattner et al., 2005) with default parameters. All fully annotated plastomes were deposited in the NCBI GenBank database under the accession number MK675807–MK675811 (Supplementary Table S1).
The published plastomes of Santalales (Petersen et al., 2015; Su and Hu, 2016; Li et al., 2017; Yang et al., 2017; Liu et al., 2018; Shin and Lee, 2018; Zhu et al., 2018; Jiang et al., 2019; Chen et al., 2020) were downloaded from NCBI GenBank (Supplementary Table S2). Together with the newly generated plastomes, the gene content, boundaries of IRs/LSC and IRs/SSC of Santalales hemiparasites were compared using Geneious V10.2 (Kearse et al., 2012). Any putative gene losses detected in the newly generated plastomes were further verified by extracting intact sequences of the corresponding genes from the autotrophic Erythropalum scandens plastome and conducting local BLAST searches against the sequencing reads of each species. To investigate structural rearrangement in Santalales plastomes, the plastomes were progressively aligned by the multiple genome alignment software Mauve 2.3.1 (Darling et al., 2010), with E. scandens as the reference.
Phylogenetic Analysis
A phylogenetic tree was inferred with complete plastome DNA sequences. The plastomes of Colobanthus quitensis (KT737381) and Nyssa sinensis (KX904873) were selected as the outgroup because of the close relationships of Santalales to Caryophyalles and Cornales (Der and Nickrent, 2008; Su et al., 2015). Plastome sequences were aligned using MAFFT (Kazutaka and Standley, 2013), and manually edited where necessary. The most appropriated model of sequence substitution for plastomes (GTR + G) was selected using Modeltest v3.7 (Posada, 1998) with the Akaike information criterion (Posada and Buckley, 2004). We treated the whole plastome as a single inherited unit. Because RAxML can accommodate only one DNA substitution model, we used PartitionFinder v. 2.1.1 (Lanfear et al., 2017) to examine whether the selected mode is commonly suitable for LSC, SSC, and IRs, with the “greedy” search algorithm. We used maximum-likelihood analysis (ML) to reconstruct phylogenetic relationships. The ML analysis was performed using RAxML–HPC BlackBox v8.1.24 (Stamatakis, 2006; Miller et al., 2010) with 10 independent ML searches conducted and the branch support determined by computing 1,000 non-parametric bootstrap (BS) replicates.
Results
Features of the Newly Sequenced Plastomes
Illumina paired-end sequencing generated over 30 million clean reads for each species. The mean coverage of the plastome sequencing was 6,518.67X for O. wightiana, 573.28X for P. edulis, 4314.96X for S. album, 134.61X for V. liquidambaricolum, and 592.48X for V. ovalifolium. The high levels of sequencing coverage guaranteed the accuracy of plastome assembly (Supplementary Table S3). For each species, the reference–based and de novo strategies generated almost identical plastomes, with only slight differences in plastome sizes (<5 bp).
Similar to other Santalales hemiparasites, the five newly sequenced plastomes had a typical quadripartite structure, consisting of a pair of IRs, a LSC, and a SSC (Figure 1). The size of these plastomes ranged from 128,601 bp (V. liquidambaricolum) to 147,544 bp (O. wightiana), with the overall GC content varying from 36.1% (V. liquidambaricolum and V. ovalifolium) to 38.3% (P. edulis) (Table 1). Among the five plastomes, the total gene numbers as well as protein–coding, rRNA, and tRNA genes that constituted each are all slightly different (Table 2).
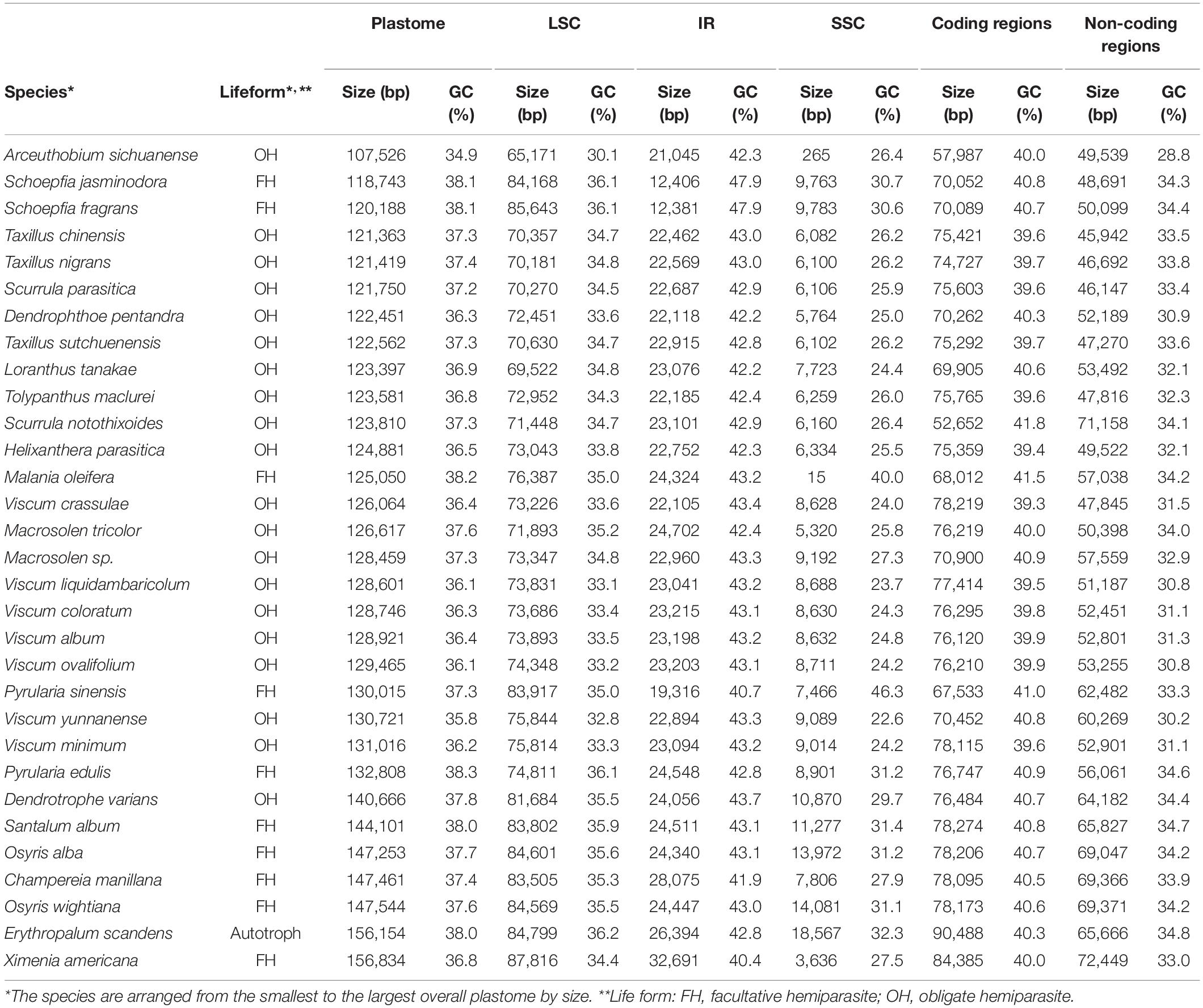
Table 1. Comparison of size and GC content (GC%) of complete plastomes, LSC, IR, SSC, coding and non-coding regions among Santalales hemiparasites and the autotrophic outgroup.
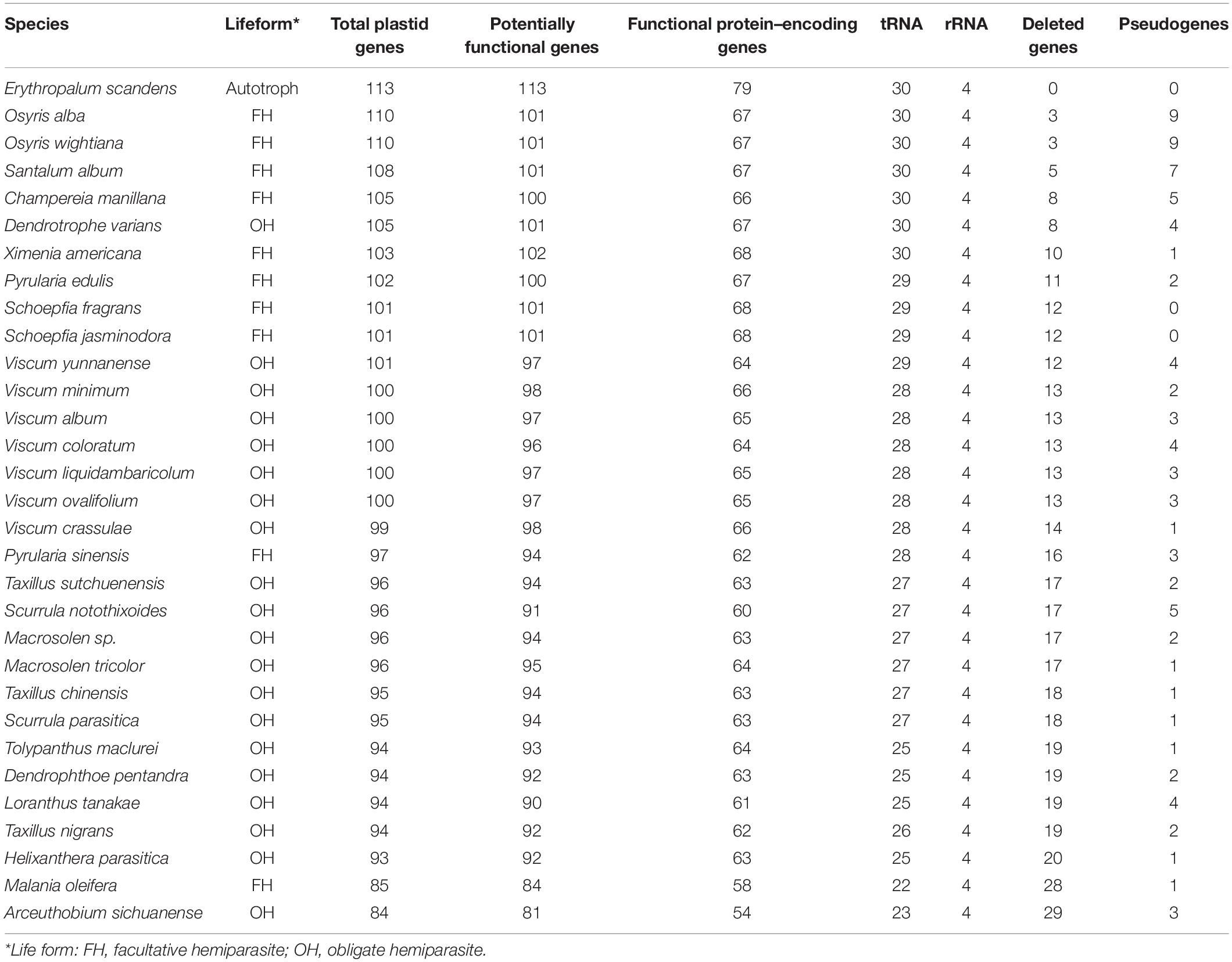
Table 2. Comparison of the plastome gene content of Santalales hemiparasites with Erythropalum scandens.
The plastomes of O. wightiana and S. album retained 101 potentially functional genes (Table 2). The pseudogenization of infA, ndhA–E, ndhG–H, and ndhK, as well as the deletion of ndhF, ndhI, and ndhJ, were observed in O. wightiana. In S. album, infA, ndhB–E, ndhG, and ndhK were identified as pseudogenes, and ndhA and ndhH had been deleted. Although the loss of all ndh genes except for ndhB (pseudogenized) was detected in P. edulis, V. liquidambaricolum, and V. ovalifolium, the additional loss of infA, trnG-UCC, and trnV-UAC, as well as the pseudogenization of ccsA, were only identified in V. liquidambaricolum and V. ovalifolium (Supplementary Table S4). Also, the loss of trnR-ACG and pseudogenization of matK was observed in P. edulis and V. ovalifolium. These gene losses were confirmed using corresponding sequences of E. scandens for local BLAST searches against the Illumina reads of each species. As the result of gene loss and pseudogenization, a total of 100, 97 and 95 putatively functional genes were observed in P. edulis, V. liquidambaricolum, and V. ovalifolium, respectively.
Comparison of Plastome Size and GC Content of Santalales Hemiparasites
Based on the plastomes sequenced in this study, as well as those reported previously, we compared the plastome size and GC content of Santalales hemiparasites with those of E. scandens (Table 1). All hemiparasite plastomes, except for that of Ximenia americana (156,834 bp), are smaller than the 156,154 bp of the autotrophic outgroup. The LSC length of the Santalales hemiparasites ranged from 65,171 bp (Arceuthobium sichuanense) to 87,816 bp (X. americana), whereas the length of IR and SSC regions varied from 12,381 bp (Schoepfia fragrans) to 32,691 bp (X. americana), and 15 bp (Malania oleifera) to 14,081 bp (O. wightiana), respectively. These regions of the hemiparasites (except for X. americana), were reduced in size to varying degrees compared to those of E. scandens (84,799 bp in LSC, 26,394 bp in each IR, and 18,576 bp in SSC). Also, the hemiparasite plastomes had varying GC content from 34.9% (A. sichuanense) to 38.3% (P. edulis). In each plastome, LSC, SSC, and IR regions had an uneven distribution of GC content. Specifically, the IR regions had the highest GC (40.4–47.9%), followed by LSC (30.1–36.1%). The SSC region exhibited the lowest GC content (23.7–40.0%).
Structural Shifts in Santalales Hemiparasitic Plastomes
The junctions of IR/LSC and IR/SSC in the plastomes of Santalales hemiparasites are variable (Figure 1). The SSC region has been extremely contracted to 15 bp in M. oleifera (Ximeniaceae), and 265 bp in A. sichuanense (Viscaceae). A significant IR contraction to the ycf2 at the IR/LSC junctions was observed in the plastomes of Schoepfiaceae. The IR/LSC boundaries of all Loranthaceae species are in rpl2, and the IR/SSC junctions fall into trnL-UAG and ycf1. Within Santalaceae, the IR falls into rps19 in LSC, and in ccsA and the trnR-trnN intergenic spacer in SSC. The plastomes of Cervantesiaceae and C. manillana (Opiliaceae) share the same IR/LSC boundaries with species of Santalaceae, while their IRb/SSC junction shifted to the intergenic spacer between trnN-GUU and rrn5S, and trnL-UAG and trnN-GUU. The expansion of IR regions in Viscaceae reached rpl22-trnH-rps3 cluster in the IR/LSC boundaries, and their IR/SSC fall into ycf1, as well as the intergenic spacer between trnN-GUU and rpl32. The result of the multiple plastome alignment is illustrated in Figure 2. Compared with the autotrophic E. scandens plastome, no structural rearrangement was found in the LSC and IR regions of Santalales hemiparasites. However, multiple rearrangements were found in the IR/SSC boundaries, and SSC regions in species of Amphorogynaceae, Cervantesiaceae, Santalaceae, and Viscaceae.
Comparison of Plastome Gene Content and Organization
The examined Santalales hemiparasites had divergent gene content in their plastomes (Table 2 and Supplementary Table S4 and Figure 3). The plastomes of Dendrotrophe varians, O. alba, O. wightiana, S. album, S. fragrans, and Schoepfia jasminodora encoded the highest number of potentially functional genes with a total of 101, including 67 protein-coding, 30 tRNA, and 4 rRNA genes. Comparatively, the lowest number of putatively functional genes was observed in A. sichuanense, which has 54 protein-coding, 23 tRNA, and 4 rRNA genes. The functional or physical losses of the plastid-encoded NAD(P)H–dehydrogenase (NDH) complex is commonly shared by all Santalales hemiparasites, while the loss of plastid infA was identified in Cervantesiaceae, Loranthaceae, Opiliaceae, Santalaceae, and Viscaceae. In addition, the deletion of trnV–UAC genes was shared by species of Loranthaceae, Schoepfiaceae, Viscaceae, and Ximenaceae. The further loss of trnG-UCC was observed in Loranthaceae, Viscaceae, and Ximeniaceae. The family specific losses of rpl32, rps15, rps16, and trnK-UUU were observed in Loranthaceae and Ximeniaceae, and the further deletion of essential photosynthetic genes (psaC, psbA, I, K, L and M, petB, and ccsA) was identified in Ximeniaceae.
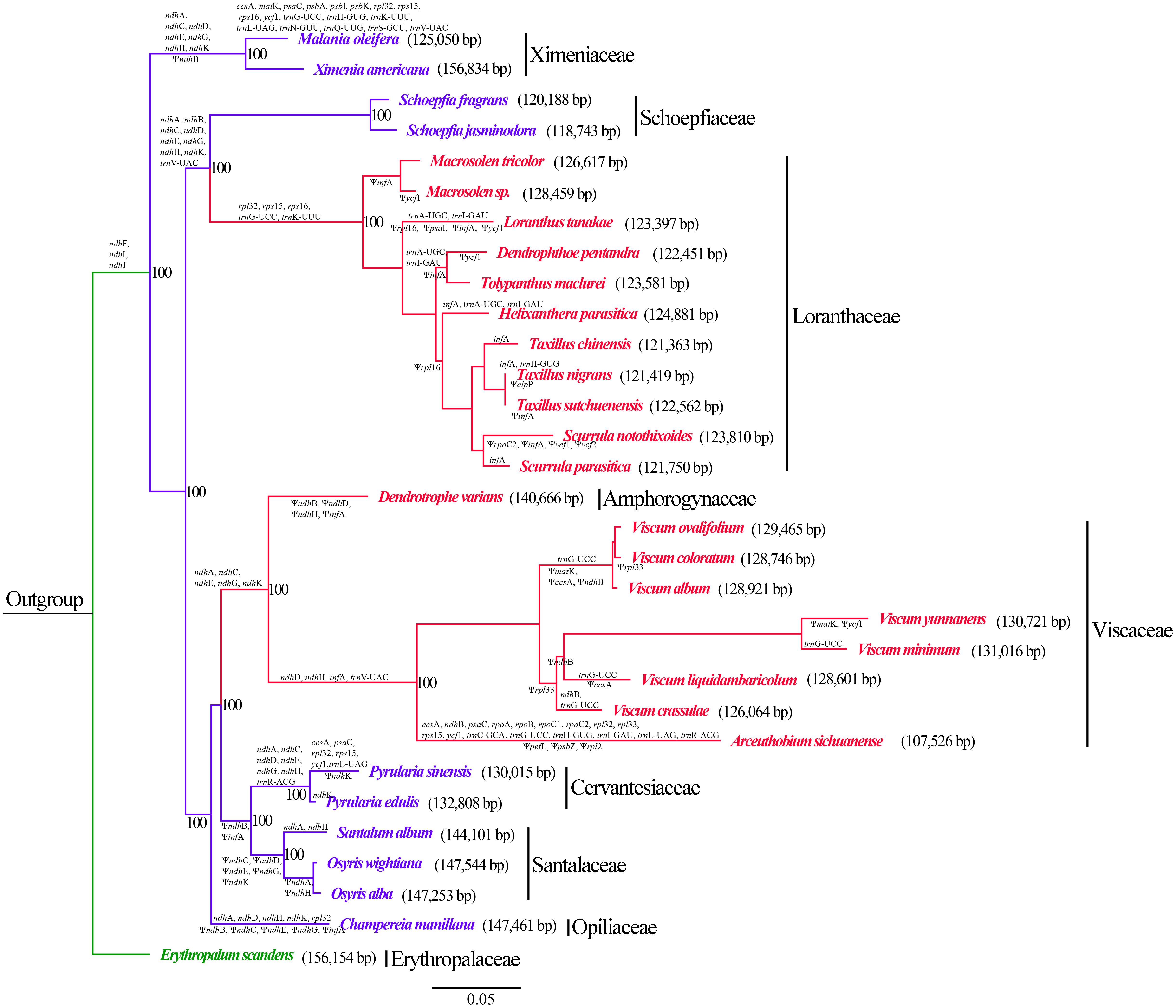
Figure 3. Phylogenetic relationships within Santalales hemiparasites. The branches are labeled with specific gene loss (genes above branches) and pseudogenization (genes under branches). Plastome size of each species are shown in the parentheses behind the botanical name. The number at each node is the maximum-likelihood bootstrap percentage. The branch lengths are proportional to the substitution rate. Green lineages: autotrophy outgroup; blue lineages: facultative hemiparasites; red lineages: obligate hemiparasites.
Phylogenetic Analysis
Maximum-likelihood analysis (Figure 3) resolved the autotrophic taxa E. scandens (Erythropalaceae) as the early diverging clade of the sandalwood order, and recovered the hemiparasites in three major clades (BS = 100%). Notably, the obligate hemiparasites did not form a monophyletic group, with obligate hemiparasitism evolving at least twice from facultative parasitism. Of those hemiparasites, Ximeniaceae (M. oleifera and X. Americana) was resolved as the first diverging branch. Within the second clade, Loranthaceae were recovered as monophyletic (BS = 100%), which was sister to Schoepfiaceae (BS = 100%). Within the third clade, C. manillana (Opiliaceae) is sister to clade comprised of Santalaceae + Cervantesiaceae, D. varians (Amphorogynaceae), and Viscaceae. The predicted gene deletions and gene inactivation shared by specific branches is shown in the tree topology. We also compared the plastome size among close species. The results indicate that closely related taxa possess similar-sized plastomes as well as relatively analogous gene content.
Discussion
Plastome Downsizing and Structural Shifts
The lifestyle transition from autotrophy to parasitism often triggers reductive plastome evolution (Wicke and Naumann, 2018). Compared with the autotrophic E. scandens, the plastomes of Santalales hemiparasites have reduced in size by approximately 6–31%. Among them, X. americana possessed the largest plastome (156,834 bp). Contrarily, in the most reduced plastome (107,526 bp) of A. sichuanense, a total of 29 genes have been lost. The observation of more genes missing and a smaller sized plastome (Table 2), suggests that gene loss can be partly responsible for the plastome downsizing in Santalales hemiparasites.
We also found that the decrease of plastome size in Santalales is accompanied with a length reduction of non-coding regions, including intergenic regions between functional genes and introns (Table 1). A similar phenomenon has been shown in the hemiparasitic Cuscuta species (McNeal et al., 2007). A previous study proposed that the downsizing of non-coding regions have evolved to prompt the efficiency of energy and nutrition utilization, particularly in disadvantageous environments (Wu et al., 2009). Because of their nutritional reliance on host plants, reduction of non-coding regions may reinforce their fitness to the partial heterotrophic lifestyle.
Inverted repeat expansion and contraction frequently occur in angiosperm plastomes. These events often cause the lineage-specific gain or loss of a small number of genes in the IR/LSC, and IR/SSC junctions (Plunkett and Downie, 2000; Chumley et al., 2006). Although large-scale IR expansions and contractions are only observed in a few autotrophic species (Chumley et al., 2006), dramatic IR shifts commonly occur in the plastomes of both holoparasites (Bungard, 2004; Maier et al., 2007; McNeal et al., 2007; Krause, 2008; Naumann et al., 2016; Samigullin et al., 2016; Sidonie et al., 2016; Schneider et al., 2018) and hemiparasites (Li et al., 2013, 2017; Su and Hu, 2016; Cho et al., 2018; Frailey et al., 2018; Liu et al., 2018; Park et al., 2018; Shin and Lee, 2018; Jiang et al., 2019; Chen et al., 2020). Similarly, significant IR and SSC contractions were found in Schoepfiaceae, M. oleifera and A. sichuanense (Figure 1), respectively. Moreover, multiple rearrangements occurred in the SSC regions in hemiparasitic plastomes of Amphorogynaceae, Cervantesiaceae, Santalaceae, and Viscaceae (Figure 2). This suggests that the evolution of parasitism has caused prominent structural changes in the plastomes of some Santalales hemiparasites.
Missing and Pseudogenized Genes
The lifestyle transition from autotrophy to parasitism always leads to prevalent gene losses from the plastomes (Neuhaus and Emes, 2000; Wicke et al., 2013, 2016; Wicke and Naumann, 2018). Compared to autotrophic species, Santalales hemiparasites have experienced significant gene deletion and pseudogenization (Table 2). The series of reductive mutations involves ndh genes, infA, matK, rpl32, rpl33, rps15, rps16, ycf1, as well as several photosynthesis-associated genes (psaC, psbA, I, K, L and M, petB, and ccsA) and tRNAs (Figure 3 and Supplementary Table S4).
The ndh genes encode subunits of the NADH dehydrogenase complex, which mediates photosystem I electron recycle and facilitates chlororespiration in plant cells (Yamori and Shikanai, 2016). In general, the ndh genes are the earliest functional losses in the plastomes of hemiparasites (Maier et al., 2007; Wicke et al., 2013, 2016; Wicke and Naumann, 2018). In Santalales hemiparasites, all eleven ndh (A–K) genes have either been deleted or pseudogenized, suggesting that the plastid NDH pathway is not essential in these species. It is noteworthy that the physical or functional losses of these plastid–encoded ndh genes does not occur exclusively in parasitic or heterotrophic plants. The degeneration of ndh loci have been observed in a wide spectrum of autotrophic lineages, such as Gnetales and Pinaceae of gymnosperm (Wakasugi et al., 1994; McCoy et al., 2008; Braukmann et al., 2009; Wu et al., 2009), the early diverging eudicots (Sun et al., 2016, 2017), the eudicot families Cactaceae (Sanderson et al., 2015) and Geraniaceae (Blazier et al., 2011), the aquatic monocot Najas (Peredo et al., 2013), and epiphytic orchids (Chang et al., 2006; Lin et al., 2015, 2017). Given the independent loss of this pathway in many plant groups, Frailey et al. (2018) proposed that the plastid ndh genes may have been selected against in these photoautotrophic lineages. Also, a previous study revealed the NDH complex–related genes have been concomitantly deleted from plastid and nuclear genomes in some orchids, and proposed that the mutation in photoautotrophic plants may increase the possibility to evolve a heterotrophic life history (Lin et al., 2017). Given that all ndh genes are retained in their autotrophic relative (E. scandens), we hypothesize that the degradation of these genes from plastomes of Santalales hemiparasites may be associated with the lifestyle shift from photoautotroph to hemiparasitism. The eco-physiological consequences of the functional and physical losses of the plastid NDH pathway in Santalales hemiparasites, especially under stress conditions, merit to be furtherly examined (Chen et al., 2020).
Another commonly degraded plastid gene in Santalales is infA; this mutation was detected in all Santalales hemiparasites except for Ximeniaceae and Schoepfiaceae. The loss or pseudogenization of infA occurs in the plastomes of many holoparasitic plants (Wicke et al., 2011, 2013, 2016; Wicke and Naumann, 2018). With respect to hemiparasitic plants, reduction of this gene has been exclusively observed in Santalales (Petersen et al., 2015; Li et al., 2017; Yang et al., 2017; Liu et al., 2018; Shin and Lee, 2018; Zhu et al., 2018; Jiang et al., 2019; Chen et al., 2020). Given that pseudogenization or deletion of infA gene has also been identified in a wide diversity of photoautotrophic plant lineages (Shinozaki et al., 1986; Millen et al., 2001; Ahmed et al., 2012), previous studies revealed that infA is likely to be one of the most mobile plastid genes in flowering plants, which are often transferred to and maintained in the nucleus (Millen et al., 2001; Ahmed et al., 2012). Therefore, it is possible that infA has been likewise transferred to the nucleus, and the degradation of this gene in Santalales hemiparasites may not be correlated to the evolution of parasitism.
Besides the functional or physical losses of ndh loci and infA, further plastome degradations were found in some Santalales hemiparasites. For example, the loss of rps15, rps16, and rpl32 was commonly shared by all Loranthaceae and Ximeniaceae species (Figure 3). Similarly, losses of these plastid ribosomal protein–coding genes have been observed in diverse lineage of autotrophic angiosperms (e.g., Saski et al., 2005; Jansen et al., 2007; Wicke et al., 2011; Sabir et al., 2014; Park et al., 2015; Schwarz et al., 2015). A line of evidence indicates that such genes are transferred to the nucleus in these plants (Park et al., 2015). One possibility is that the plastid ribosomal protein-coding genes have likewise been transferred to the nucleus, and the reduction of these genes in Santalales hemiparasites may not be resulted from the evolution of parasitism. In addition, losses of tRNA genes were commonly observed in Santalales hemiparasites. With smaller size, the import of tRNAs from the cytoplasm may be relatively easy (Wicke and Naumann, 2018). Therefore, plastid tRNA genes may be dispensable even at an early parasitic stage. Following the degradation of ndh genes, those non-essential genes such as tRNAs tend to be deleted from hemiparasitic plastomes.
Plastid genes that encode photosystem (psa/psb), cytochrome complex (pet), and heme attachment (ccsA) are key genes involved in photosynthesis (Wicke et al., 2011; Wicke and Naumann, 2018). Loss or pseudogenization of those core photosynthesis genes had been commonly found in holoparasitic angiosperms (e.g., Funk et al., 2007; McNeal et al., 2007; Wicke et al., 2016; Wicke and Naumann, 2018). According to the model of reductive plastome evolution associated with parasitism (Wicke et al., 2016; Wicke and Naumann, 2018), functional losses of the photosynthesis–associated genes (e.g., psa/psb genes, pet genes, ccsA, cemA) may occur around the boundary to holoparasitism. However, the observation of ccsA pseudogenization in Viscaceae species, as well as the loss of psaC, psbA, I, K, L and M, petB, and ccsA in M. oleifera, imply that the degradation of these core photosynthesis genes might initiate in hemiparasites that still heavily rely on photosynthesis. Because of their great importance for photosynthesis, further studies are needed to clarify whether these genes have been functionally transferred to the nuclear genome (Chen et al., 2020).
Lineage–Specific Plastome Degradation
A previous study suggested that the varying nutritional dependences on a host may influence the reductive evolution of hemiparasitic plastomes (Petersen et al., 2015). Distinct from facultative hemiparasite, an obligate hemiparasite cannot complete the life cycle without coming into contact with a host (Heide–Jørgensen, 2008). As a result, their plastomes may be expected to possess a higher level of degradation. Similar to a previous study (Chen et al., 2020), we found that the range of plastome size of the Santalales facultative hemiparasites (118,743–156,834 bp) largely overlaps the range for obligated ones (107,526–140,666 bp). We also observed that some facultative and obligate plants exhibited the same degree of gene deletion and pseudogenization. For instance, Pyrularia species (facultative) and D. varians (obligate hemiparasites) maintained almost identical gene content. In addition, a total of 28 genes has been deleted from the facultative hemiparasite, M. oleifera. The level of plastome degradation is higher than the majority of obligate hemiparasites (except for A. sichuanense) in Santalales. This suggests that obligate hemiparasitism may not lead to a higher level of physical or functional loss of plastid genes than facultative one. Therefore, the degree of plastome degradation may not be correlated with the lifeform of facultative or obligate.
The relationships within the sandalwood order recovered in this study received high support values (Figure 3), which are highly congruent with previous studies (Der and Nickrent, 2008; Nickrent et al., 2010; Su et al., 2015; Chen et al., 2020). Based on the phylogenetic relationships recovered in this study, we found that closely related taxa, for instance, within the same genera or family tend to possess high level of similarity in plastome size, structure, and gene content. We hence hypothesize that plastome degradation in Santalales hemiparasites may evolve in a lineage–specific manner.
Data Availability Statement
The newly sequenced plastomes in this study were deposited in the NCBI GenBank database under the accession numbers MK675807–MK675811 (Supplementary Table S1).
Author Contributions
YJ, XG, HW, and WS designed the research. XG, CL, and GZ collected and analyzed the data. XG and YJ wrote the manuscript. HC, XZ, and JL discussed the results and revised the manuscript.
Funding
This research was financially supported by the NSFC–Joint Foundation of Yunnan Province (U1802287), National Natural Science Foundation of China (31060052 and 31872673), and the Major Program of National Natural Science Foundation of China (31590823).
Conflict of Interest
The authors declare that the research was conducted in the absence of any commercial or financial relationships that could be construed as a potential conflict of interest.
Acknowledgments
We are grateful to Zhangming Wang, Shenyan Huang, and Youcai Shi for their help in collecting samples used in the study, and to Lifang Yang, Jin Yang, Zhenyan Yang, Lei Jin, and Zhijie Ruan for their assistant in data analysis.
Supplementary Material
The Supplementary Material for this article can be found online at: https://www.frontiersin.org/articles/10.3389/fgene.2020.00597/full#supplementary-material
TABLE S1 | Voucher information and GenBank accession.
TABLE S2 | Lifeform and GenBank accession of published Santalales plastomes.
TABLE S3 | Summary of Illumina sequencing of the five Santalales hemiparasites.
TABLE S4 | Comparison of plastome gene content among Santalales hemiparasites.
Footnotes
References
Ahmed, I., Biggs, P. J., Matthews, P. J., Collins, L. J., Hendy, M. D., and Lockhart, P. J. (2012). Mutational dynamics of aroid chloroplast genomes. Genome Biol. Evol. 4, 1316–1323. doi: 10.1093/gbe/evs110
Barkman, T. J., Mcneal, J. R., Lim, S. H., Coat, G., Croom, H. B., Young, N. D., et al. (2007). Mitochondrial DNA suggests at least 11 origins of parasitism in angiosperms and reveals genomic chimerism in parasitic plants. BMC Evol. Biol. 7:248. doi: 10.1186/1471-2148-7-248
Blazier, J. C., Guisinger–Bellian, M. M., and Jansen, R. K. (2011). Recent loss of plastidencoded ndh genes within Erodium (Geraniaceae). Plant Mol. Biol. 76, 263–272. doi: 10.1007/s11103-011-9753-5
Braukmann, T. W. A., Kuzmina, M., and Stefanovic, S. (2009). Loss of all plastid ndh genes in Gnetales and conifers: extent and evolutionary significance for the seed plant phylogeny. Curr. Genet. 55, 323–337. doi: 10.1007/s00294-009-0249-7
Bungard, R. A. (2004). Photosynthetic evolution in parasitic plants: insight from the chloroplast genome. Bioessays 26, 235–247. doi: 10.1002/bies.10405
Chang, C. C., Lin, H. C., Lin, I. P., Chow, T. Y., Chen, H. H., Chen, W. H., et al. (2006). The chloroplast genome of Phalaenopsis aphrodite (Orchidaceae): Comparative analysis of evolutionary rate with that of grasses and its phylogenetic implications. Mol. Biol. Evol. 23, 279–291. doi: 10.1093/molbev/msj029
Chen, X., Fang, D., Wu, C., Liu, B., Liu, Y., Sahu, S. K., et al. (2020). Comparative plastome analysis of root–and stem–feeding parasites of Santalales untangle the footprints of feeding mode and lifestyle transitions. Genome Biol. Evol. 12, 3663–3676. doi: 10.1093/gbe/evz271
Cho, W. B., Choi, B. H., Kim, J. H., Lee, D. H., and Lee, J. H. (2018). Complete plastome sequencing reveals an extremely diminished SSC region in hemiparasitic Pedicularis ishidoyana (Orobanchaceae). Ann. Bot. Fenici. 55, 171–183. doi: 10.5735/085.055.0122
Chumley, T. W., Palmer, J. D., Mower, J. P., Fourcade, H. M., Calie, P. J., Boore, J. L., et al. (2006). The complete chloroplast genome sequence of Pelargonium x hortorum: organization and evolution of the largest and most highly rearranged chloroplast genome of land plants. Mol. Biol. Evol. 23, 2175–2190. doi: 10.1093/molbev/msl089
Daniell, H., Lin, C. S., Yu, M., and Chang, W. J. (2016). Chloroplast genomes: diversity, evolution, and applications in genetic engineering. Genome Biol. 17:134.
Darling, A. E., Mau, B., and Perna, N. T. (2010). ProgressiveMauve: multiple genome alignment with gene gain, loss and rearrangement. PLoS One 5:e11147. doi: 10.1371/journal.pone.0011147
Der, J. P., and Nickrent, D. L. (2008). A molecular phylogeny of Santalaceae (Santalales). Syst. Bot. 33, 107–116. doi: 10.1600/036364408783887438
Dierckxsens, N., Mardulyn, P., and Smits, G. (2017). NOVOPlasty: de novo assembly of organelle genomes from whole genome data. Nucleic Acids Res. 45, e18. doi: 10.1093/nar/gkw955
Doyle, J. J., and Doyle, J. L. (1987). A rapid DNA isolation procedure for small quantities of fresh leaf tissue. Phytochem. Bull. 19, 11–15.
Frailey, D. C., Chaluvadi, S. R., Vaughn, J. N., Coatney, C. G., and Bennetzen, J. L. (2018). Gene loss and genome rearrangement in the plastids of five hemiparasites in the family Orobanchaceae. BMC Plant Biol. 18:30.
Funk, H., Berg, S., Krupinska, K., Maier, U., and Krause, K. (2007). Complete DNA sequences of the plastid genomes of two parasitic flowering plant species, Cuscuta reflexa and Cuscuta gronovii. BMC Plant Biol. 7:45. doi: 10.1186/1471-2229-7-45
Jansen, R. K., Cai, Z., Raubeson, L. A., Daniell, H., De Pamphilis, C. W., Leebens–Mack, J., et al. (2007). Analysis of 81 genes from 64 plastid genomes resolves relationships in angiosperms and identifies genome–scale evolutionary patterns. Proc. Natl. Acad. Sci. U.S.A. 104, 19369–19374. doi: 10.1073/pnas.0709121104
Jiang, D., Ma, R., Li, J., Mao, Q., Miao, N., and Mao, K. (2019). Characterization of the complete chloroplast of Scurrula parasitica. Mitochondrial DNA 4, 247–248. doi: 10.1080/23802359.2018.1501294
Kazutaka, K., and Standley, D. M. (2013). MAFFT multiple sequence alignment software version 7: improvements in performance and usability. Mol. Biol. Evol. 30, 772–780. doi: 10.1093/molbev/mst010
Kearse, M., Richard, M., Amy, W., Steven, S. H., Matthew, C., Shane, S., et al. (2012). Geneious Basic: an integrated and extendable desktop software platform for the organization and analysis of sequence data. Bioinformatics 28, 1647–1649. doi: 10.1093/bioinformatics/bts199
Krause, K. (2008). From chloroplasts to “cryptic” plastids: evolution of plastid genomes in parasitic plants. Curr. Genet. 54, 111–121. doi: 10.1007/s00294-008-0208-8
Lanfear, R., Frandsen, P. B., Wright, A. M., Senfeld, T., and Calcott, B. (2017). PartitionFinder 2: new methods for selecting partitioned models of evolution for molecular and morphological phylogenetic analyses. Mol Biol Evol. 34, 772–773. doi: 10.1093/molbev/msw260
Li, X., Zhang, T. C., Qiao, Q., Ren, Z., Zhao, J., Yonezawa, T., et al. (2013). Complete chloroplast genome sequence of holoparasite Cistanche deserticola (Orobanchaceae) reveals gene loss and horizontal gene transfer from its host Haloxylon ammodendron (Chenopodiaceae). PLoS One 8:e58747. doi: 10.1371/journal.pone.0058747
Li, Y., Zhou, J. G., Chen, X. L., Cui, Y. X., Xu, Z. C., Li, Y. H., et al. (2017). Gene losses and partial deletion of small single–copy regions of the chloroplast genomes of two hemiparasitic Taxillus species. Sci. Rep. 7:12834.
Lin, C. S., Chen, J. J., Chiu, C. C., Hsiao, H. C., Yang, C. J., Jin, X. H., et al. (2017). Concomitant loss of NDH complex–related genes within chloroplast and nuclear genomes in some orchids. Plant J. 90, 994–1006. doi: 10.1111/tpj.13525
Lin, C. S., Chen, J. J., Huang, Y. T., Chan, M. T., Daniell, H., Chang, W. J., et al. (2015). The location and translocation of ndh genes of chloroplast origin in the Orchidaceae family. Sci. Rep. 5:9040. doi: 10.1038/srep09040
Liu, S. S., Hu, Y. H., Maghuly, F., Porth, I. M., and Mao, J. F. (2018). The complete chloroplast genome sequence annotation for Malania oleifera, a critically endangered and important bioresource tree. Conserv. Genet. Res. 11, 271–274. doi: 10.1007/s12686-018-1005-4
Maier, U. G., Krupinska, K., Berg, S., Funk, H. T., and Krause, K. (2007). Complete DNA sequences of the plastid genomes of two parasitic flowering plant species, Cuscuta reflexa and Cuscuta gronovii. BMC Plant Biol. 7, 1–12.
McCoy, S. R., Kuehl, J. V., Boore, J. L., and Raubeson, L. A. (2008). The complete plastid genome sequence of Welwitschia mirabilis: an unusually compact plastome with accelerated divergence rates. BMC Evol. Biol. 8:130. doi: 10.1186/1471-2148-8-130
McNeal, J. R., Kuehl, J. V., Boore, J. L., and Pamphilis, C. W. D. (2007). Complete plastid genome sequences suggest strong selection for retention of photosynthetic genes in the parasitic plant genus Cuscuta. BMC Plant Biol. 7:57. doi: 10.1186/1471-2229-7-57
Millen, R. S., Olmstead, R. G., Adams, K. L., Palmer, J. D., Lao, N. T., Heggie, L., et al. (2001). Many parallel losses of infA from chloroplast DNA during angiosperm evolution with multiple independent transfers to the nucleus. Plant Cell 13, 645–658. doi: 10.3732/ajb.0800085
Miller, M. A., Pfeiffer, W., and Schwartz, T. (2010). Creating the CIPRES science gateway for inference of large phylogenetic trees. Gateway Comput. Environ. Workshop 14, 1–8. doi: 10.1109/GCE.2010.5676129
Naumann, J., Der, J. P., Wafula, E. K., Jones, S. S., Wagner, S. T., Honaas, L. A., et al. (2016). Detecting and characterizing the highly divergent plastid genome of the nonphotosynthetic parasitic plant Hydnora visseri (Hydnoraceae). Genome Biol. Evol. 8, 345–363. doi: 10.1093/gbe/evv256
Neuhaus, H. E., and Emes, M. J. (2000). Nonphotosynthetic metabolism in plastids. Annu. Rev. Plant Physiol. Plant Mol. Biol. 51, 111–140. doi: 10.1146/annurev.arplant.51.1.111
Nickrent, D. L. (1997). The Parasitic Plant Connection. (Updated on 15 May 2018). Carbondale, IL: Southern Illinois University.
Nickrent, D. L. (2002). “Plantas parásitas en el mundo,” in Plantas Parásitas de la Península Ibérica e Islas Baleares, eds J. A. López–Sáez, P. Catalán, and L. Sáez (Madrid: Mundi–Prensa Libros), 7–27.
Nickrent, D. L., Malécot, V., Vidal–Russell, R., and Der, J. P. (2010). A revised classification of Santalales. Taxon 59, 538–558. doi: 10.2307/25677612
Palmer, J. D. (1985). Comparative organization of chloroplast genomes. Annu. Rev. Genet. 19, 325–354. doi: 10.1146/annurev.ge.19.120185.001545
Park, I., Yang, S., and Kin, W. J. (2018). The complete chloroplast genome of Cuscuta pentagona Engelm. Mithchodr. DNA Part B. 3, 523–524. doi: 10.1080/23802359.2018.1467229
Park, S., Jansen, R. K., and Park, S. (2015). Complete plastome sequence of Thalictrum coreanum (Ranunculaceae) and transfer of the rpl32 gene to the nucleus in the ancestor of the subfamily Thalictroideae. BMC Plant Bio. 15:40. doi: 10.1186/s12870-015-0432-6
Peredo, E. L., King, U. M., and Les, D. H. (2013). The plastid genome of Najas flexilis: adaptation to submersed environments is accompanied by the complete loss of the NDH complex in an aquatic angiosperm. PLoS One 8:e68591. doi: 10.1371/journal.pone.0068591
Petersen, G., Cuenca, A., and Seberg, O. (2015). Plastome evolution in hemiparasitic mistletoes. Genome Biol. Evol. 7, 2520–2532. doi: 10.1093/gbe/evv165
Plunkett, G. M., and Downie, S. R. (2000). Expansion and contraction of the chloroplast inverted repeat in Apiaceae Subfamily Apioideae. Syst. Bot. 25, 648–667. doi: 10.2307/2666726
Posada, D. (1998). MODELTEST: testing the model of DNA substitution. Bioinformatics 14, 817–818. doi: 10.1093/bioinformatics/14.9.817
Posada, D., and Buckley, T. R. (2004). Model selection and model averaging in phylogenetics: advantages of akaike information criterion and Bayesian approaches over likelihood ratio tests. Syst. Biol. 53, 793–808. doi: 10.2307/4135365
Sabir, J., Schwarz, E. N., Ellison, N., Zhang, J., Baeshen, N. A., Mutwakil, M., et al. (2014). Evolutionary and biotechnology implications of plastid genome variation in the inverted–repeat lacking clade of legumes. Plant Biotechnol. J. 12, 743–754. doi: 10.1111/pbi.12179
Samigullin, T. H., Logacheva, M. D., Penin, A. A., and Vallejoroman, C. M. (2016). Complete plastid genome of the recent holoparasite Lathraea squamaria reveals earliest stages of plastome reduction in Orobanchaceae. PLoS One. 11:e0150718. doi: 10.1371/journal.pone.0150718
Sanderson, M. J., Copetti, D., Búrquez, A., Bustamante, E., Charboneau, J. L. M., Eguiarte, L. E., et al. (2015). Exceptional reduction of the plastid genome of saguaro cactus (Carnegiea gigantea): loss of the ndh gene suite and inverted repeat. Am. J. Bot. 102, 1115–1127. doi: 10.3732/ajb.1500184
Saski, C., Lee, S. –B., Daniell, H., Wood, T. C., Tomkins, J., Kim, H. –G., et al. (2005). Complete chloroplast genome sequence of Glycine max and comparative analyses with other legume genomes. Plant Mol. Biol. 59, 309–322. doi: 10.1007/s11103-005-8882-0
Schattner, P., Brooks, A. N., and Lowe, T. M. (2005). The tRNAscan–SE, snoscan and snoGPS web servers for the detection of tRNAs and snoRNAs. Nucleic Acids Res. 33, 686–689. doi: 10.1093/nar/gki366
Schneider, A. C., Chun, H., Stefanović, S., and Baldwin, B. G. (2018). Punctuated plastome reduction and host–parasite horizontal gene transfer in the holoparasitic plant genus Aphyllon. Proc. R. Soc. B. 285:20181535. doi: 10.1098/rspb.2018.1535
Schwarz, E. N., Ruhlman, T. A., Sabir, J. S., Hajrah, N. H., Alharbi, N. S., Al–Malki, A. L., et al. (2015). Plastid genome sequences of legumes reveal parallel inversions and multiple losses of rps16 in papilionoids. J. System. Evol. 53, 458–468. doi: 10.1111/jse.12179
Shin, H. W., and Lee, N. S. (2018). Understanding plastome evolution in hemiparasitic Santalales: complete chloroplast genomes of three species, Dendrotrophe varians, Helixanthera parasitica, and Macrosolen cochinchinensis. PLoS One. 13:e0200293. doi: 10.1371/journal.pone.0200293
Shinozaki, K., Ohme, M., Tanaka, M., Wakasugi, T., Hayshida, N., Matsubayashi, T., et al. (1986). The complete nucleotide sequence of the tobacco chloroplast genome: its gene organization and expression. EMBO J. 5, 2043–2049. doi: 10.1002/j.1460-2075.1986.tb04464.x
Sidonie, B., Natalie, C., Luo, S., Sun, G., Shahin, Z., Andreas, G., et al. (2016). Assembled plastid and mitochondrial genomes, as well as nuclear genes, place the parasite family Cynomoriaceae in the Saxifragales. Mol. Biol. Evol. 8, 2214–2230. doi: 10.1093/gbe/evw147
Stamatakis, A. (2006). RAxML–VI–HPC: maximum likelihood–based phylogenetic analysis with thousands of taxa and mixed models. Bioinformatics 22, 2688–2690. doi: 10.1093/bioinformatics/btl446
Straub, S. C. K., Parks, M., Weithmier, K., Fishbein, M., Cronn, R. C., and Liston, A. (2012). Navigating the tip of the genomic iceberg: next–generation sequencing for plant systematics. Am J Bot. 99, 349–364. doi: 10.3732/ajb.1100335
Su, H. J., and Hu, J. M. (2016). The complete chloroplast genome of hemiparasitic flowering plant Schoepfia jasminodora. Mitochondrial DNA 1, 767–769. doi: 10.1080/23802359.2016.1238753
Su, H. J., Hu, J. M., Anderson, F. E., Der, J. P., and Nickrent, D. L. (2015). Phylogenetic relationships of Santalales with insights into the origins of holoparasitic Balanophoraceae. Taxon 64, 491–506. doi: 10.12705/643.2
Sun, Y., Moore, M. J., Lin, N., Adelalu, K. F., Meng, A., Jian, S., et al. (2017). Complete plastome sequencing of both living species of Circaeasteraceae (Ranunculales) reveals unusual rearrangements and the loss of the ndh gene family. BMC Genomics 18:592.
Sun, Y. X., Moore, M. J., Zhang, S. J., Soltis, P. S., Soltis, D. E., Zhao, T. T., et al. (2016). Phylogenomic and structural analyses of 18 complete plastomes across nearly all families of early–diverging eudicots, including an angiosperm wide analysis of IR gene content evolution. Mol. Phylogenet Evol. 96, 93–101. doi: 10.1016/j.ympev.2015.12.006
Tešitel, J. (2016). Functional biology of parasitic plants: a review. Plant Ecol Evol. 149, 5–20. doi: 10.5091/plecevo.2016.1097
Wakasugi, T., Tsudzuki, J., Ito, S., Nakashima, K., Tsudzuki, T., and Sugiura, M. (1994). Loss of all ndh genes as determined by sequencing the entire chloroplast genome of the black pine Pinus thunbergii. Proc. Natl. Acad. Sci. U.S.A. 91, 9794–9798. doi: 10.2307/2365708
Westwood, J. H., Yoder, J. I., Timko, M. P., and Depamphilis, C. W. (2010). The evolution of parasitism in plants. Trends Plant Sci. 15, 227–235. doi: 10.1016/j.tplants.2010.01.004
Wicke, S., Müller, K. F., Depamphilis, C. W., Quandt, D., Bellot, S., and Schneeweiss, G. M. (2016). Mechanistic model of evolutionary rate variation en route to a nonphotosynthetic lifestyle in plants. Proc. Natl. Acad. Sci. U.S.A. 113, 9045–9050. doi: 10.1073/pnas.1607576113
Wicke, S., Müller, K. F., Depamphilis, C. W., Quandt, D., Wickett, N. J., Zhang, Y., et al. (2013). Mechanisms of functional and physical genome reduction in photosynthetic and nonphotosynthetic parasitic plants of the broomrape family. Plant Cell. 25, 3711–3725. doi: 10.1105/tpc.113.113373
Wicke, S., and Naumann, J. (2018). Molecular evolution of plastid genomes in parasitic flowering plants. Adv. Bot. Res. 85, 315–347. doi: 10.1016/bs.abr.2017.11.014
Wicke, S., Schneeweiss, G. M., de Pamphilis, C. W., Müller, K. F., and Quandt, D. (2011). The evolution of the plastid chromosome in land plants: gene content, gene order, gene function. Plant Mol. Biol. 76, 273–297. doi: 10.1007/s11103-011-9762-4
Wu, C. S., Lai, Y. T., Lin, C. P., Wang, Y. N., and Chaw, S. M. (2009). Evolution of reduced and compact chloroplast genomes (cpDNAs) in gnetophytes: selection toward a lower–cost strategy. Mol. Phylogenet. Evol. 52, 115–124. doi: 10.1016/j.ympev.2008.12.026
Wyman, S. K., Jansen, R. K., and Boore, J. L. (2004). Automatic annotation of organellar genomes with DOGMA. Bioinformatics 20, 3252–3255. doi: 10.1093/bioinformatics/bth352
Yamori, W., and Shikanai, T. (2016). Physiological functions of cyclic electron transport around photosystem I in sustaining photosynthesis and plant growth. Annu. Rev. Plant Biol. 67, 81–106. doi: 10.1146/annurev-arplant-043015-112002
Yang, G.-S., Wang, Y.-H., Wang, Y.-H., and Shen, S.-K. (2017). The complete chloroplast genome of a vulnerable species Champereia manillana (Opiliaceae). Conserv. Genet. Resour. 9, 415–418. doi: 10.1007/s12686-017-0697-1
Keywords: parasitism, plastome degradation, gene loss, pseudogenization, Cervantesiaceae, Santalaceae, Viscaceae
Citation: Guo X, Liu C, Zhang G, Su W, Landis JB, Zhang X, Wang H and Ji Y (2020) The Complete Plastomes of Five Hemiparasitic Plants (Osyris wightiana, Pyrularia edulis, Santalum album, Viscum liquidambaricolum, and V. ovalifolium): Comparative and Evolutionary Analyses Within Santalales. Front. Genet. 11:597. doi: 10.3389/fgene.2020.00597
Received: 09 April 2019; Accepted: 18 May 2020;
Published: 16 June 2020.
Edited by:
Federico Luebert, University of Chile, ChileReviewed by:
Liping Zeng, University of California, Riverside, United StatesDeise J. P. Goncalves, The University of Texas at Austin, United States
Copyright © 2020 Guo, Liu, Zhang, Su, Landis, Zhang, Wang and Ji. This is an open-access article distributed under the terms of the Creative Commons Attribution License (CC BY). The use, distribution or reproduction in other forums is permitted, provided the original author(s) and the copyright owner(s) are credited and that the original publication in this journal is cited, in accordance with accepted academic practice. No use, distribution or reproduction is permitted which does not comply with these terms.
*Correspondence: Wenhua Su, d2hzdUB5bnUuZWR1LmNu; Hengchang Wang, aGN3YW5nQHdiZ2Nhcy5jbg==; Yunheng Ji, aml5aEBtYWlsLmtpYi5hYy5jbg==