- 1Department of Immunology, The University of Texas Southwestern Medical Center, Dallas, TX, United States
- 2Department of Pediatrics, The University of Washington and Seattle Children’s Hospital, Seattle, WA, United States
Chromosome 22q11.2 deletion syndrome (22q11.2del) is a complex, multi-organ disorder noted for its varying severity and penetrance among those affected. The clinical problems comprise congenital malformations; cardiac problems including outflow tract defects, hypoplasia of the thymus, hypoparathyroidism, and/or dysmorphic facial features. Additional clinical issues that can appear over time are autoimmunity, renal insufficiency, developmental delay, malignancy and neurological manifestations such as schizophrenia. The majority of individuals with 22q11.2del have a 3 Mb deletion of DNA on chromosome 22, leading to a haploinsufficiency of ~106 genes, which comprise coding RNAs, noncoding RNAs, and pseudogenes. The consequent haploinsufficiency of many of the coding genes are well described, including the key roles of T-box Transcription Factor 1 (TBX1) and DiGeorge Critical Region 8 (DGCR8) in the clinical phenotypes. However, the haploinsufficiency of these genes alone cannot account for the tremendous variation in the severity and penetrance of the clinical complications among those affected. Recent RNA and DNA sequencing approaches are uncovering novel genetic and epigenetic differences among 22q11.2del patients that can influence disease severity. In this review, the role of coding and non-coding genes, including microRNAs (miRNA) and long noncoding RNAs (lncRNAs), will be discussed in relation to their bearing on 22q11.2del with an emphasis on TBX1.
Genetics of 22q11.2 Deletion Syndrome
Chromosome 22q11.2 deletion syndrome (22q11.2del) (OMIM #’s 188400, 192430, 611867) is the most common chromosomal microdeletion reported in humans, affecting ~1/4,000 individuals (Botto et al., 2003; Kobrynski and Sullivan, 2007; Fung et al., 2015; Mcdonald-Mcginn et al., 2015). Screening fetuses for 22q11.2del by prenatal procedures reveals an even higher frequency of ~1/1,000, suggesting a high morbidity in utero (Wapner et al., 2012; Grati et al., 2015). The disease is multi-syndromic of contrasting severity and penetrance among those affected. It can include congenital heart disease (CHD; especially conotruncal malformations, tetralogy of Fallot, aortic arch abnormalities, truncus arteriosus, ventricular septal defects and vascular rings) and abnormalities of the palate (clefts and velopharyngeal incompetence) (Kobrynski and Sullivan, 2007; Fung et al., 2015; Guna et al., 2015; Mcdonald-Mcginn et al., 2015; Meechan et al., 2015; Morsheimer et al., 2017; Sullivan, 2019). Hypoplasia of the thymus, hypoparathyroidism, dysmorphic facial features, renal and/or skeletal anomalies are also common (Kobrynski and Sullivan, 2007; Mcdonald-Mcginn et al., 2015; Sullivan, 2019). Those in whom an immune deficiency is identified are often classed as having DiGeorge syndrome (Conley et al., 1979; Kobrynski and Sullivan, 2007; Markert et al., 2007; Marcovecchio et al., 2019). DiGeorge syndrome is first suggested following newborn screens for detecting the levels T-cell receptor excision circles (TRECs) as a measure of T cell output from the thymus (Table 1) (Botto et al., 2003; Kobrynski and Sullivan, 2007; Mcdonald-Mcginn et al., 2015). Low TRECs can be an indicator of DiGeorge syndrome, with the diagnosis of 22q11.2del subsequently established by FISH or chromosomal microarray technologies (Kwan et al., 2014; Van Der Spek et al., 2015; Schmid et al., 2017; Ravi et al., 2018). The heterogeneous congenital problems for 22q11.2del patients arise from defective remodeling of the pharyngeal region during embryogenesis (Sullivan, 2004; Bassett et al., 2005; Kobrynski and Sullivan, 2007; Fung et al., 2015; Guna et al., 2015; Mcdonald-Mcginn et al., 2015; Baldini et al., 2016). Impacted is the second heart field and the pharyngeal arch arteries, which form the outgrowth vessels of the heart, as well as the pharyngeal pouches (PP), with the 3rd PP forming the thymic lobes and inferior parathyroids (Lindsay et al., 1999; Jerome and Papaioannou, 2001; Lindsay et al., 2001; Merscher et al., 2001; Xu et al., 2004; Chen et al., 2012; Alfano et al., 2019). Over time, individuals with 22q11.2del often exhibit developmental delay and autoimmune manifestations, with their malignancy risk higher compared to the general population. Autism and autism spectrum, anxiety, attention deficit disorders and psychiatric illnesses like schizophrenia are common (Mcdonald-Mcginn et al., 1999; Kobrynski and Sullivan, 2007; Karayiorgou et al., 2010; Morsheimer et al., 2017; Sullivan, 2019; Zinkstok et al., 2019). Males and females with 22q11.2del are equally affected, regardless of their racial/ethnic grouping (Óskarsdóttir et al., 2005; Kobrynski and Sullivan, 2007; Mcdonald-Mcginn et al., 2015; Kruszka et al., 2017). The least concordant phenotypes occur in children of African descent (Kruszka et al., 2017). Not surprisingly given the numerous organ systems affected, 22q11.2del patients have a diminished life expectancy (Repetto et al., 2014). Mid-aged 22q11.2del individuals have a median life expectancy of 42 years compared to normal sibling controls (60–70 yrs. of age) (Mcdonald-Mcginn et al., 2006; Bassett et al., 2009; Bassett et al., 2011; Repetto et al., 2014). With such a wide gamut of problems, a multi-disciplinary clinical approach is often needed to provide adequate care for children and adolescents with 22q11.2del. This can definitely improve the overall quality of life for 22q11.2del patients, which is lower than normal (Fung et al., 2015; Mcdonald-Mcginn et al., 2015). Yet, health care costs for 22q11.2del patients can reach a staggering $1,000,000 during their first 20 years of life (Brenner et al., 2016).
Ninety percent of individuals with 22q11.2del have a 3 Mb microdeletion on chromosome 22, resulting in a hemizygosity of approximately 106 genes (Figure 1) (Guna et al., 2015; Mcdonald-Mcginn et al., 2015). Forty-six of these are protein coding, 24 are pseudogenes and the remainder comprise non-coding RNAs; 7 microRNAs (miRNAs), 12 long noncoding RNAs (lncRNAs), 2 small nucleolar RNAs (snoRNAs), and additional undefined transcripts. About 5-8% of 22q11.2del individuals have a smaller, nested deletion of 1.5 Mb (Figure 1). This leads to a haploinsufficiency of 30 coding genes, as well as 6 and 9 of the miRNAs and lncRNAs affected with the longer deletion, respectively. Both types of deletions are due to erroneous chromosomal rearrangements during meiosis. These exchanges involve 8 large, paralogous low copy repeats (LCRs), or segmental duplications (A–H) that are distributed along a 5.6 Mb segment of chromosome 22q11.2 (Figure 1) (Shaikh et al., 2001; Hwang et al., 2014). DNA recombination of the LCRs, which are 96% sequence identical, during cross-over exchanges results in erroneous deletions or duplications (Shaikh et al., 2000; Shaikh et al., 2001; Bittel et al., 2009). Distributed within or around the LCRs are 8 long intergenic noncoding RNAs (lncRNAs). These lncRNAs contain Translocation Breakpoint Type A (TBTA) sequences with homology to FAM230C, a lncRNA on chromosome 13 (Delihas, 2018). Such lncRNAs may contribute to the deletions via the translocation breakpoint sequences. The most frequent deletion in 22q11.2del patients spans LCR A-D (3 Mb), with a less frequent deletion between LCR A-B (1.5 Mb) (Figure 1). These are referred to as proximal deletions and are causal to the majority of clinical phenotypes ascribed to 22q11.2del. The actual breakpoint location within the LCR does not have a major role in 22q11.2del phenotypes (Bertini et al., 2017). A rare number of individuals have deletions between LCR B-D or LCR C-D, which are referred to as central deletions. These deletions can also cause CHD and/or neurological abnormalities (Saitta et al., 1999; Burnside, 2015). Even more infrequent are deletions between LCR C-E, LCR D-E, and LCR E-F, although the reported clinical phenotypes are not characteristic of 22q11.2del (Saitta et al., 1999; Shaikh et al., 2007; Burnside, 2015; Guna et al., 2015).
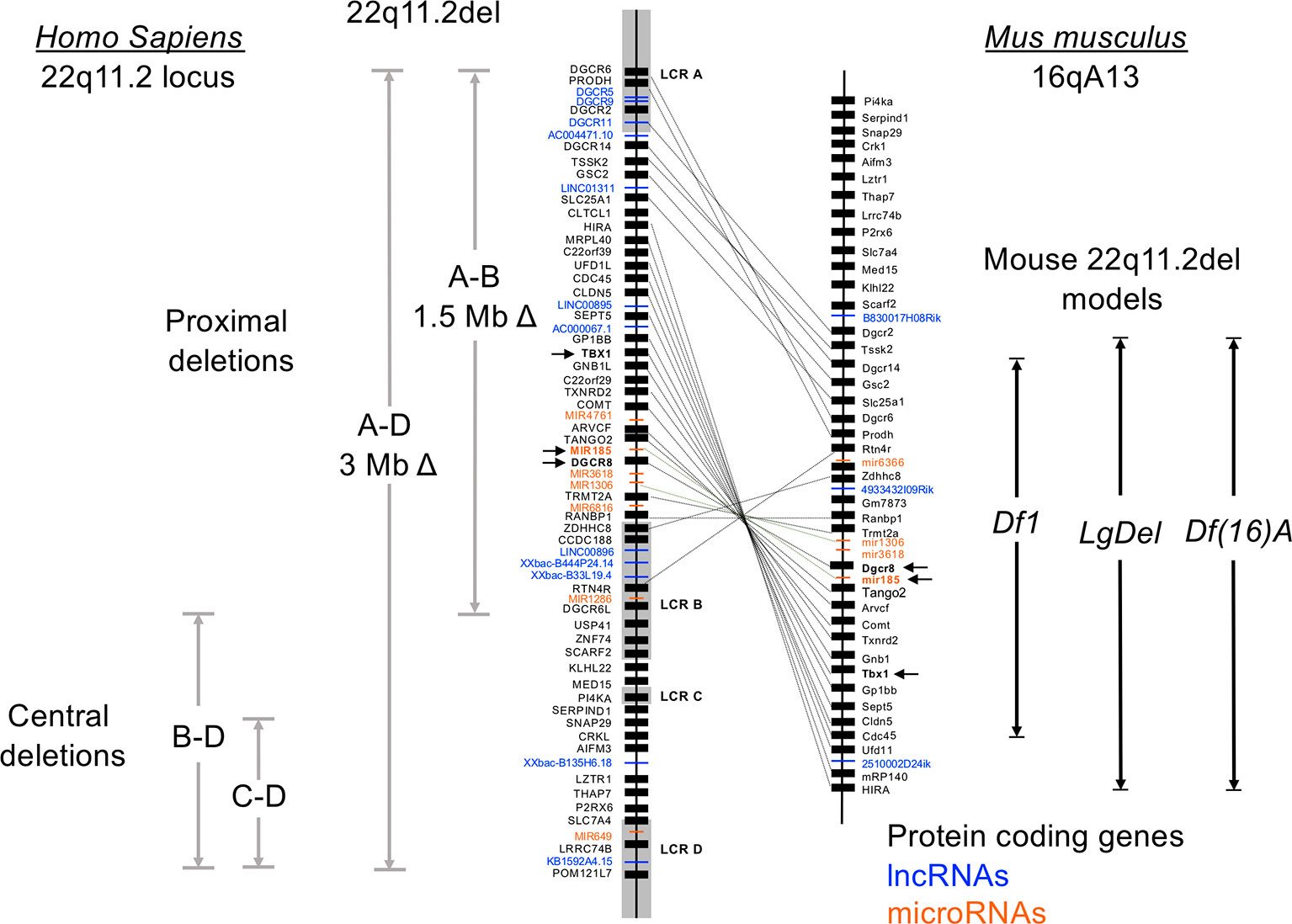
Figure 1 Genetic organization of the human chromosome 22q11.2 locus and synteny on murine chromosome 16. Human chromosome 22 is approximately 51 million base pairs. The region affected by the 22q11.2 chromosomal deletions and duplications spans approximately 4 Mb, with 8 low copy repeats (LCR A-LCR H) causal to this distributed throughout this region. LCR A-LCR D are shown. Recombination of these highly homologous sequences (also called segmental duplications) results in proximal, central, and distal (not shown) deletions affecting both coding and noncoding DNA segments. The proximal deletions are responsible for the classic clinical features of 22q11.2del, which includes DiGeorge syndrome. The location of the coding genes, and noncoding RNAs including miRNAs and lncRNAs, are shown for the proximal region of chromosome 22q11.2. The corresponding genes on the murine locus are connected with lines, which reveals a flipping of the locus and a break containing distinct genes. Three of the more commonly used mouse models of 22q11.2del that replicate many clinical features of 22q11.2del are shown, named as the Df16(A), Df1 and Lgdel lines.
The role of many of the genes whose haploinsufficiency contributes to the congenital malformations, immune system complications, neurological issues and other clinical phenotypes are well-described and discussed in many reviews (Zemble et al., 2010; Bassett et al., 2011; Mcdonald-Mcginn et al., 2015; Meechan et al., 2015; Morsheimer et al., 2017; Morrow et al., 2018; Sullivan, 2019; Zinkstok et al., 2019). Among some of the more well-characterized genes are T-Box 1 Transcription Factor (TBX1), DiGeorge Syndrome Critical Region 8 (DGCR8), Crk-like Adaptor Protein L (CRKL), Proline Dehydrogenase (PRODH), Reticulon 4 Receptor (RTN4R), Zinc-finger DHHC-type Containing 8 (ZDHHC8), Catechol-O-Methyl Transferase (COMT), Guanine Nucleotide Binding Protein b-polypeptide 1-Like (GNB1L), Septin 5 (SEP5) and Glycoprotein Ib Platelet Subunit Beta (GP1BB). Yet, the haploinsufficiency of these genes is clearly unable to explain the heterogeneous penetrance and severity of clinical phenotypes that differ from patient to patient. This is even noted among family members with the same mutation in TBX1 and with genetically identical in-bred strains of mice used as models of 22q11.2del syndrome. In this review, we present recent findings revealing how variations in the expression of TBX1, the functions of DGCR8, and the miRNAs and long noncoding RNAs within and outside the 22q11.2 locus can influence the severity of the clinical manifestations of 22q11.2del (Tables 1–3). We also review data identifying gene duplications, CNVs, SNPs, and other chromosomal abnormalities that impact 22q11.2del patients and/or result in overlapping 22q11.2-like phenotypes in some individuals.
The Genetic and Epigenetic Regulation Coupled to TBX1 Functions
The congenital malformations associated with 22q11.2del are often linked to the haploinsufficiency of TBX1 (Lindsay et al., 1999; Jerome and Papaioannou, 2001; Lindsay et al., 2001; Merscher et al., 2001; Zhang and Baldini, 2008; Fulcoli et al., 2016). TBX1 is expressed at specific regions in the pharyngeal apparatus and in the cardiac progenitors of the second heart field, regulating the expression of nearly 2000 genes (Xu et al., 2004; Chen et al., 2012; Fulcoli et al., 2016). Many excellent reviews detailing the transcriptional role of TBX1 in the patterning of the pharyngeal arches and pouches have been published (Yagi et al., 2003; Zemble et al., 2010; Bassett et al., 2011; Fung et al., 2015; Mcdonald-Mcginn et al., 2015; Meechan et al., 2015; Baldini et al., 2016; Sullivan, 2019). While one might expect that a simple haploinsufficiency of TBX1 should consistently lead to similar congenital malformations, this is certainly not what happens. It is becoming apparent that genetic and epigenetic changes, both within and outside chromosome 22q11.2, can dramatically sway the clinical phenotypes of 22q11.2del (Lindsay et al., 1999; Jerome and Papaioannou, 2001; Lindsay et al., 2001; Merscher et al., 2001; Zhang and Baldini, 2008; Fulcoli et al., 2016).
To understand how such genetic and epigenetic factors impact TBX1 and how this may affect the clinical phenotypes requires some knowledge of how TBX1 functions. Recent evidence indicates that TBX1 interacts with members of the Histone-lysine N-methyltransferase (KMT2)-family (Fulcoli et al., 2016). The KMT2 enzymes activate transcription by mono-methylating lysine residues on Histone 3 (H3K4) on chromatin (Figure 2) (Fulcoli et al., 2016). The TBX1-KMT2 complex regulates the low-level expression of thousands of genes by marking chromatin. In addition, TBX1 interacts with the SWI-SNF-like BAF complex to support chromatin remodeling (Figure 2) (Chen et al., 2012). These two types of interactions would suggest that small fluctuations in the levels of TBX1 could modulate the expression of thousands of transcripts, with those impacted likely stochastic from cell to cell. Thus, variations in TBX1 levels could underlie the severity of the malformations in the pharyngeal region. What patient to patient differences exist that could affect TBX1 expression? One is with DiGeorge Critical Region 6 (DGCR6), a gene also encoded on the frequently deleted segment of chromosome 22q11.2. DGCR6 down-regulates TBX1, impacting neural crest migration within the pharyngeal region (Figure 2, Table 3) (Groot et al., 2004; Chakraborty et al., 2012). As neural crest cells establish the vasculature of the pharyngeal arch arteries along with the capsule of the thymus, changes in their location would affect the morphogenesis of this region. While the transcript levels of DGCR6 as well as its homolog, DGCR6L should be reduced 50% due to their respective haploinsufficiency in 22q11.2del patients, their levels are extremely variable in 22q11.2del patients (Chakraborty et al., 2012). Some individuals actually have much higher levels of DGCR6 compared to normal controls (Chakraborty et al., 2012). Such dramatic expression variations in DGCR6 and DGCR6L are epigenetically determined as there is no evidence of maternal or paternal imprinting (Chakraborty et al., 2012). Noteworthy, either deletions or duplications of DGCR6, in combination with an adjacent gene, PRODH, are linked to CHD (Gao et al., 2015b). These findings suggest that fluctuations in DGCR6 will affect TBX1 levels, and as a consequence, patterning of the pharyngeal region. While most TBX1 is localized in the nucleus, some is present in the cytosol where a portion is complexed with SMAD1 (Fulcoli et al., 2009). This interaction limits the levels of a SMAD1-SMAD4 complex, preventing effective Bone Morphogenic Protein 4 (BMP4) signaling (Figure 2) (Fulcoli et al., 2009). As Bmp4 has a critical role in the septation of the outflow tracts and remodeling of the arch arteries, disruption of its signaling by modulating TBX1 levels is certain to impact these processes (Liu et al., 2004). Bmp4 also regulates early thymus and parathyroid morphogenesis (Gordon et al., 2008). TBX1 interacts with several additional proteins, albeit the role of these complexes are less well-defined (Baldini et al., 2016). In summary, alterations in the levels and/or subcellular distribution of TBX1 will certainly impact the severity of the congenital problems.
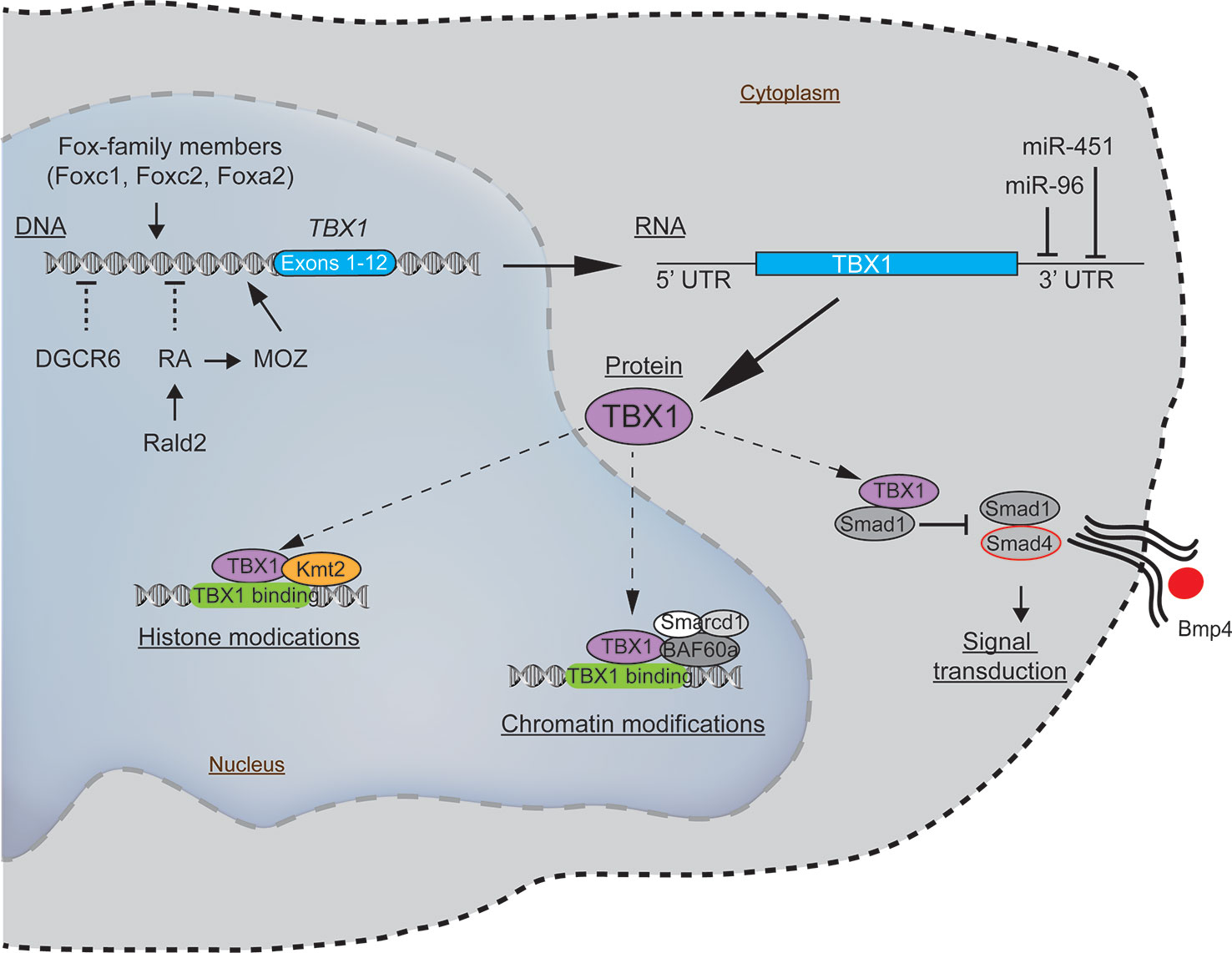
Figure 2 Molecular pathways intersecting with TBX1 with the potential to modulate the clinical severity and penetrance of 22q11.2del. The expression of TBX1, a key gene coupled to the patterning of the pharyngeal apparatus, is positively regulated by members of the Fox-family of transcription factors and MOZ. Negative regulators of TBX1 include the defined teratogens, retinoic acid (RA), gestational diabetes, and miR-96 and miR-451a, the latter two miRNAs that target the 3' untranslated region of TBX1, resulting in its degradation. While most TBX1 resides in the nucleus, wherein it can regulate gene expression, a fraction of TBX1 is localized in the cytosol, where it negatively impacts bone morphogenic protein signaling via a complex with SMAD4.
The consequence of varying TBX1 levels in relation to the severity of the congenital malformations in humans is further supported by comparisons with the diverse 22q11.2del mouse models. Multiple mouse lines were originally created with deletions of varying length on chromosome 16 (orthologous to human chromosome 22q11.2) to identify causal genes in the disorder (Figure 1) (Jones et al., 1999; Kimber et al., 1999; Lindsay et al., 1999; Puech et al., 2000; Jerome and Papaioannou, 2001; Lindsay et al., 2001; Merscher et al., 2001). Comparing the phenotypes in several such mouse lines confirms that changes in Tbx1 levels affects the extent and severity of the CHD, thymic hypoplasia, and hypoparathyroidism (Kimber et al., 1999; Lindsay et al., 1999; Puech et al., 2000; Jerome and Papaioannou, 2001; Lindsay and Baldini, 2001; Lindsay et al., 2001; Merscher et al., 2001; Taddei et al., 2001). Yet, relative to humans, mice actually have a lower penetrance of several of the congenital defects in the setting of either the 22q11.2del or just a Tbx1 haploinsufficiency, most evident with the number of embryos exhibiting a hypoplasia of the thymus. This corresponds to the levels of TBX1, since reducing Tbx1 to 35% normal in the embryos results in a highly penetrant thymic hypoplasia (Zhang and Baldini, 2008). Are there strategies to circumvent the reduced levels of TBX1? Yes, as treating pregnant mice with a histone demethylase inhibitor (tranylcypromine) can increase the methylation levels of H3K4 and partially rescue the cardiovascular anomalies in the Tbx1-mutant embryos (Fulcoli et al., 2016).
There are several additional regulators of TBX1 expression that likely have a bearing on 22q11.2del. One is retinoic acid (RA), a metabolite of vitamin A that functions as a natural morphogen involved in the patterning of the 3rd, 4th, and 6th pharyngeal arch arteries and 3rd PP (Figure 2) (Niederreither et al., 2003; Vermot et al., 2003; Cipollone et al., 2006; Voss et al., 2012). Vitamin A deficiency during pregnancy remains a public health issue in developing countries due to its impact on the developing fetus, particularly eye development (Bastos Maia et al., 2019). High levels of RA can act as a teratogen that causes overlapping 22q11.2del-like phenotypes. This was discovered after pregnant mothers were prescribed RA (tretinoin, isotretinoin) as a clinical treatment for acne, with their infants born with 22q11.2-like congenital malformations (Kucera, 1971; Lammer et al., 1985; Lipson et al., 1993; Markert et al., 2007; Mills, 2010; Sacks et al., 2012; Browne et al., 2014). Mechanistically, RA suppresses TBX1 expression (Roberts et al., 2005; Ryckebusch et al., 2010; Smith and Tallquist, 2010). This was confirmed by using mice created with a haploinsufficiency of retinaldehyde dehydrogenase 2 (Rald2), an enzyme required for RA synthesis. Rald2+/- embryos produce less RA, increasing the levels of Tbx1. In the setting of a Tbx1 haploinsufficiency, the severity of the heart defects was reduced in the Rald2+/- background (Ryckebusch et al., 2010). RA also acts to epigenetically alter chromatin accessibility by positively regulating the expression of Monocytic Leukemia Zinc Finger (MOZ/Myst3/Kat6a), a histone acetyltransferase. MOZ, in turn, positively regulates TBX1 levels, with Moz-knock-out mice and those with loss-of-function mutations having less Tbx1, along with Tbx2, Tbx5, and Tbx9 (Roberts et al., 2005; Jenkinson et al., 2007; Voss et al., 2012). In humans, heterozygous MOZ mutations lead to a syndromic disorder comprising CHD, intellectual disability, and dysmorphic facial features (Tham et al., 2015). In summary, too much RA leads to similar clinical phenotypes as 22q11.2del, likely through its ability to reduce the expression of various TBX-family members including TBX1 (Lindsay et al., 2001; Merscher et al., 2001; Ensenauer et al., 2003; Mokate et al., 2006; Zweier et al., 2007; Zhang and Baldini, 2008).
Gestational diabetes is a distinct teratogen affecting pregnancy, with newborns having congenital features overlapping with 22q11.2del (Kucera, 1971; Mills, 2010; Sacks et al., 2012; Dornemann et al., 2017). In fact, about 18% of infants with a thymic aplasia who did not have deletions on chromosome 22q11.2 and required a thymic tissue transplant were born to mothers with a clinical history of gestational diabetes (Markert et al., 2007). Experimental induction of diabetes in pregnant mice and rats similarly results in a thymic hypoplasia along with intrauterine growth impairment (Padmanabhan and Shafiullah, 2004). So how might elevated blood sugar levels lead to 22q11.2del-like presentations? While this is not yet known, there are some emerging clues. Pregestational diabetes reduces the expression of a regulator of RA, Cytochrome P450 family 26 subfamily A member 1 (Cyp26a1), in the caudal region (tailbud) of developing embryos (Lee et al., 2017). Cyp26a1 catabolizes RA in various tissues to control the levels of this product, and consequently morphogenesis. One speculation is that elevations in blood sugar levels during gestation may increase the levels of RA in the pharyngeal region, thereby diminishing TBX1 levels. It is also known that gestational diabetes affects histone modifications and DNA methylation patterns in fetal cord blood, implying an epigenetic effect that may contribute to the 22q11.del phenotypes (Haertle et al., 2017). These emerging findings suggest that better control of gestational diabetes may aid in diminishing the severity of certain congenital malformations, particularly for embryos who have 22q11.2del.
DiGeorge Syndrome Critical Region 8 and microRNA Contributions to 22q11.2del
DiGeorge Syndrome Critical Region 8 (DGCR8) is another key gene whose haploinsufficiency in 22q11.2del patients can influence the severity of their clinical problems. It is a nuclear miRNA binding protein required for the biogenesis of microRNAs (miRNAs) (Landthaler et al., 2004; Yi et al., 2009; Schofield et al., 2011). MiRNAs are a family of ~2000 small noncoding RNAs (18-22 nucleotides) that bind to diverse mRNA transcripts, targeting the latter for degradation (Lewis et al., 2005; Grosshans and Filipowicz, 2008; Lodish et al., 2008; Bartel, 2009; Xiao and Rajewsky, 2009; Krol et al., 2010; Salmena et al., 2011; Alles et al., 2019). DGCR8 binds to primary miRNA (pri-miRNA) transcripts in the nucleus in conjunction with DROSHA, an endoribonuclease (Ribonuclease III) that cleaves pri-miRNAs into precursor miRNAs (pre-miRNAs) (Lee et al., 2003; Landthaler et al., 2004). These pre-miRNAs are subsequently exported from the nucleus for further processing into miRNAs. Importantly, such miRNAs regulate cellular homeostasis in almost all tissues, are very stress responsive, and facilitate tissue repair and regeneration (Bartel, 2009; Leung and Sharp, 2010; Mendell and Olson, 2012). Thus, a 50% reduction in DGCR8 expression due to 22q11.2del would be predicted to modulate the expression of hundreds of miRNAs. Consistent with this, 22q11.2del patients have a miRNA dysregulation in the peripheral blood, with many miRNAs reduced in expression relative to normal controls (Table 2) (De La Morena et al., 2013). This dysregulation includes a hypervariable miRNA expression pattern that differs from patient to patient, and interestingly, a coordinated expression of multiple distinct clusters of miRNAs among most 22q11.2del individuals (De La Morena et al., 2013; Sellier et al., 2014). The distinct miRNA clustering may emanate from physiological responses to the clinical problems.
In mouse models, the haploinsufficiency of Dgcr8 causes a 30-50% reduction in the overall expression of miRNAs screened in neurons (Stark et al., 2008). These miRNA losses worsen as the mice age. One consequence so far described is an abnormal calcium response in the hippocampal pyramidal neurons (CA3-CA1) that leads to premature synaptic neurotransmitter release (Earls et al., 2010; Earls et al., 2012). Such synaptic disruptions are characteristic of schizophrenia, a frequent presentation in the 22q11.2del patients (Karayiorgou et al., 1995; Karayiorgou et al., 2010; Xu et al., 2013). The neurological miRNA deficiencies in the Dgcr8+/- mice are similar in the mouse models of 22q11.2del, establishing that a 50% reduction in Dgcr8 is key to the miRNA losses (Earls et al., 2012). Interestingly, DGCR8 has functions unrelated to miRNA processing, instead regulating splicing processes in embryonic stem cells, a finding that may reveal more complexities concerning 22q11.2del (Cirera-Salinas et al., 2017).
In addition to DGCR8, the frequently deleted segment of chromosome 22q11.2 leads to a haploinsufficiency of 7 miRNAs (Figure 1, Table 2). Six are clustered near DGCR8. MiR-185 has the strongest connection to the clinical phenotypes of 22q11.2del, detected at 0.4 X normal levels in peripheral blood samples (De La Morena et al., 2013). It is expressed in cardiac tissue, immune cells, and in the brain, targeting Marginal Zone B1 Protein (Mzb1), Bruton’s Tyrosine Kinase (Btk), Calmodulin Kinase 4 (Camk4), Sarco/endoplasmic Reticulum Ca2+-ATPase (Serca2), and Nuclear Factor of Activated T cells 3c (Nfat3c) (Earls et al., 2012; Belkaya et al., 2013; Kim et al., 2015). A common theme regarding these miR-185 targets is calcium signaling, central to many cellular processes (Table 2). The haploinsufficiency of miR-185 in hippocampal neurons results in premature synaptic transmissions, partly through changes in Serca2 and Nfatc levels, with these genes controlling or being regulated by intracellular calcium changes, respectively (Earls et al., 2012). Moreover, enforced expression of miR-185 in thymocytes attenuates T cell development by a disruption of the T cell receptor controlled calcium response (Belkaya et al., 2013). MiR-185 also controls cardiac hypertrophy by targeting Nfatc in myocardial cells (Kim et al., 2015). In B cells, the haploinsufficiency of miR-185 is linked to increased autoantibody production, and this may involve increases in the levels of Bruton’s tyrosine kinase (Btk), a target of miR-185 (Belver et al., 2010). Notably, 22q11.2del patients can have clinical phenotypes related to the disruption of all of these pathways; abnormal neurotransmitter release, low T cell output, cardiac hypertrophy, and autoimmunity. Two additional miRNAs, miR-3618 and miR-1306, are located at the 5’ untranslated region and within exon 1 of DGCR8, respectively (Table 2). While miR-1306 is encoded in most eukaryotic species, miR-3618 is restricted to primates (Merico et al., 2014). MiR-1306 targets F-box/Leucine rich repeat 5 (FBLX5), affecting epithelial to mesenchymal transitions and TGF beta-receptor 2, which is involved in the TGF-beta-SMAD4 signaling process (Figure 2) (He et al., 2018; Yang et al., 2019). Other haploinsufficient miRNAs include miR-1286, selectively encoded in advanced primates, with in situ hybridization revealing its expression in the occipital cortex (Nowakowski et al., 2018). MiR-649, located within the LCRD segment of 22q11.2, targets MALT1, a component of a complex of proteins that activate NF-κB in lymphocytes. MiR-649 enhances Herpes Simplex Virus susceptibility, likely through effects on the NF-κB pathway (Zhang et al., 2017). Several miRNA target prediction programs have been applied to the 7 miRNAs encoded on chromosome 22q11.1 to identify linked pathways that relate to clinical phenotypes. An overlapping set of these miRNAs can target TBX1, KAT8 regulatory NSL complex subunit 1 (KANSL1), Glucose Transporter 3 (GLUT3, SLC2A3), and Ras Responsive Element Binding protein 1 (RREB1) (Bertini et al., 2017; León et al., 2017). Several of these targets are discussed in a subsequent section in relation to their roles in 22q11.2del. Four of the miRNAs regulate transcripts linked to schizophrenia (Merico et al., 2014).
Interestingly, there are 2 miRNAs encoded on other chromosomes that target TBX1. One is miR-96, mutations in which cause a non-syndromic hearing loss in humans (Figure 2) (Mencia et al., 2009; Gao et al., 2015a). In a feed-back loop, TBX1 represses miR-96, suggesting that alterations in the levels of TBX1 may impact hearing loss, consistent with the auditory problems reported for some but not all 22q11.2del patients (Gao et al., 2015a). A second is miR-451, which is involved in wound healing and has been shown to target TBX1 transcripts (Sun and Jiang, 2018; Munk et al., 2019). Ongoing research efforts to identify the different miRNA targets are uncovering multiple intersecting pathways connected with the developmental and neurological manifestation of 22q11.2del (Table 2). The fact that the expression of many distinct miRNAs fluctuate over time and are variable from patient to patient likely explains some of the post-natal complications that differ among 22q11.2del patients (De La Morena et al., 2013). Future directions for such patients may be clinical interventions to improve DGCR8 functions, with recent experiments reporting that protoporphyrins can enhance miRNA biogenesis in DGCR8-haploinsufficient cells (Barr et al., 2015; Sachar et al., 2016).
Emerging Noncoding RNAs and Their Potential Roles in 22q11.2del
Adding further complexity to 22q11.del are the 12 long noncoding RNAs (lncRNAs) embedded in the frequently deleted segment of chromosome 22q11.2 (Table 2). LncRNAs can act as scaffold RNAs, transcriptional assembly hubs, regulators of chromatin accessibility and genome stability (Angrand et al., 2015; Lee et al., 2016; Quinn and Chang, 2016; Du et al., 2019). They exhibit limited evolutionary sequence conservation and are generally expressed at levels much lower than protein coding genes (Cesana et al., 2011; Keniry et al., 2012; Dey et al., 2014; Yang et al., 2014; Zhou et al., 2015). In spite of the accumulating discoveries detailing the functions of numerous lncRNAs, relatively little is known about the 12 that are frequently deleted on chromosome 22q11.2. As the curation of lncRNAs in the human genome is incomplete, additional lncRNAs are likely to be identified on chromosome 22q11.2, while some of those currently compiled may be invalidated. Four lncRNAs, DGCR5, DGCR9, LINC01311, and LINC00896 have high enough RPKM values (Reads per Kilobase of transcripts per Million mapped reads) to suggest functionality, particularly as each is expressed in the biologically relevant tissue (Table 2). Findings to date suggest that several of these lncRNAs could affect the clinical presentations of 22q11.2del, but this is only inferred based on what is known about these lncRNAs in non-22q11.2del patients. DGCR5 and Lnc00896 were up-regulated 9- and 5- fold in patients with lung adenocarcinomas, implying a role in cell growth and survival (Sui et al., 2016). In a separate study of lung cancer patients, DGCR5 was down-regulated, and its target, miR-1180, was increased in expression (Chen et al., 2017). Elevations in miR-1180 reduces apoptosis (Tan et al., 2016). DGCR5 also functions as a competing RNA for miR-23b, causes increases in the targets of this miRNA, PTEN and BTG1 (Xu et al., 2019). Lnc00896 is regulated by miR-139, although the importance of this remains unknown (Sui et al., 2016). DGCR11 is up-regulated 2-fold in hepatocellular cancers, again its role remaining undefined (Zhang et al., 2015). AC004471.10 has a protein coding analog in both the murine and cow genomes, termed Testes Specific Serine Kinase 2 (TSSK2), and lies downstream of TSSK1A (Liu et al., 2017). The significance of this lncRNA-protein coding region homology remains unclear. XXbac-B444P24.14 was identified in a screen for differentially regulated lncRNAs in multiple myeloma (Ronchetti et al., 2018). As with many of the annotated lncRNAs currently curated in the human genome, future studies will need to ascertain the functional contributions of those haploinsufficient due to 22q11.2del and assess the consequences of this on the clinical phenotypes.
Along with the miRNAs and lncRNAs encoded on chromosome 22q11.2 are two small nucleolar RNAs (snoRNAs), SNORA15, and SNORA77 (Table 2). Ranging in size from 60–300 nucleotides, snoRNAs are generated from intronic regions. They assemble with a set of proteins in the nucleolus to facilitate ribosomal RNA processing (Mcmahon et al., 2015). Both SNORA15 and SNORA77 regulate the conversion of uridines to pseudouridines to improve the folding and stabilization of rRNAs (Dupuis-Sandoval et al., 2015). The expression of SNORA15 is altered in cancer, but again how the haploinsufficiency of either of the two snoRNAs affects biological functions in the context of 22q11.2del remains an open question (Yang et al., 2017).
Taken together, it is clear that the haploinsufficiency of diverse noncoding RNAs and the extensive dysregulation of the miRNAs within this group will impact both developmental processes during embryogenesis and post-natal cardiac, immune, and neurological functions. Identifying the pathways affected by these diverse noncoding RNAs may better guide clinical management of the 22q11.2del patients.
Diverse Genetic Polymorphisms Affecting 22q11.2del Phenotypes
Patients with both deletions and duplications of chromosome 22q11.2 and those with loss- or gain- of-function mutations in TBX1 often have overlapping congenital problems that still vary in severity (Ensenauer et al., 2003; Yagi et al., 2003; Xu et al., 2004; Stoller and Epstein, 2005; Zweier et al., 2007; Chen et al., 2012; Reeh et al., 2014; Baldini et al., 2016). Moreover, identical mutations in TBX1 present among several members of the same family resulted in distinct clinical phenotypes (Yagi et al., 2003). These findings further support the idea that selected genetic and/or epigenetic changes can impact the severity of the clinical complications for 22q11.2del patients (Lindsay et al., 1999; Jerome and Papaioannou, 2001; Lindsay et al., 2001; Merscher et al., 2001; Taddei et al., 2001; Xu et al., 2004; Zhang and Baldini, 2008; Chen et al., 2012; Baldini et al., 2016; Fulcoli et al., 2016). This is evident with recent studies revealing numerous genetic differences among the 22q11.2del patients that lie outside the affected locus, potentially affecting disease severity and penetrance (Guo et al., 2015; Mlynarski et al., 2015; Consortium et al., 2016; León et al., 2017). Identified by targeted exome, whole genome, and RNA sequencing strategies, SNPs, copy number variants (CNVs), DNA coding sequence differences and noncoding alterations have been discovered. For example, whole exome sequencing of 184 different 22q11.2del patients was used to identify rare deleterious SNPs (Guo et al., 2015). This screen uncovered polymorphisms in several genes selectively in those 22q11.2del patients with CHD. Several of the SNPs are in genes that regulate histone demethylation, Jumonji Domain Containing 1C (JMJD1C), MYC Induced Nuclear Antigen (MINA), and Lysine-Specific Demethylase (KDM7A). This once again reveals an epigenetic component to 22q11.2del. SNPs have also been reported in Ras Responsive Element Binding protein 1 (RREB1) and SEC24 family member C (SEC24C) selectively in the 22q11.2del with CHD.
CNV analyses have revealed a connection between those 22q11.2del patients who have CHD with a duplication of GLUT3 (also known as SLC2A3), located on chromosome 12p13.3 (Mlynarski et al., 2015). Its duplication is only pathogenic in conjunction with 22q11.2del (Odds ratio = 5.08) (Mlynarski et al., 2015). Of the 5 glucose transporters, GLUT3 has the highest affinity and greatest transport capacity for glucose (Simpson et al., 2008). An independent screen for CNVs in 253 22q11.2del patients identified a duplication of the first 3 exons of the KANSL1 (chromosome 17q21.31), contributing to an increased odds ratio for CHD (OR = 2.75) (León et al., 2017). KANSL1 is part of protein complex that acetylates histones to regulate gene expression (Moreno-Igoa et al., 2015). How the duplication of 3 exons of KANSL1 increases the likelihood of CHD remains uncertain, but a recurring theme of histone modifications in the context of 22q11.2del is evident. KANSL1 is also linked to gene pathways regulated by miRNAs, discussed in a preceding section. In a separate screen for CNVs in miRNAs, deletions and duplications of 11 distinct miRNAs were uncovered in 22q11.2del patients, with at least 1 miRNA affected per individual (Bertini et al., 2017).
Mouse Studies Revealing Additional Candidate Genes Influencing 22q11.2del
Complementing the human sequencing are various mouse studies suggesting additional candidate genes could affect the penetrance and/or severity of 22q11.2del. Among these are Vascular endothelial growth factor (Vegf), Fibroblast growth factor 8 (Fgf8), and Platelet derived growth factor receptor (Pdgfr) (Table 3) (Vitelli et al., 2002; Stalmans et al., 2003; Voss et al., 2012). In mice, reductions in Vegf recapitulate the cardiac defects of 22q11.2del (Stalmans et al., 2003; Lambrechts et al., 2005). Mice haploinsufficient in both Tbx1 and Fgf8 (Tbx1+/−Fgf8+/−) have a higher penetrance of aortic arch artery defects, with Tbx1 regulating Fgf8 expression (Vitelli et al., 2002). These results led one group of investigators to speculate that VEGF and/or FGF8 expression differences in humans could affect the severity of clinical phenotypes in 22q11.2del (Stalmans et al., 2003; Lambrechts et al., 2005). In one case study, a family with low levels of VEGF had several members diagnosed with Tetralogy of Fallot, which occurs in patients with 22q11.2del (Lambrechts et al., 2005). Another possible epigenetic regulator is Pdgfrα, which is expressed in neural crest cells throughout the pharyngeal region (Tallquist and Soriano, 2003; Smith and Tallquist, 2010). Its targeted elimination in mice causes very similar cardiac anomalies as reported for 22q11.2del (Tallquist and Soriano, 2003). Interestingly, Pdgfrβ appears to provide some functional redundancy in the absence of Pdgfrα, as the elimination of both receptors in neural crest cells results in a near complete penetrance of the cardiac anomalies along with a thymic aplasia (Tallquist and Soriano, 2003; Richarte et al., 2007; Smith and Tallquist, 2010). Other candidate genes identified in mouse models include Pax1, Pax3, Rarb, Cyp26b1, Hoxa3, Hoxb1, Hoxb3, Hoxb4, and Hoxd4, each of which is expressed either throughout the entire pharyngeal apparatus or at selected regions. Loss-of-function mutations in human PAX1 cause otofaciocervical syndrome coupled with immunodeficiency, the latter due to the resulting thymic aplasia (Pohl et al., 2013; Paganini et al., 2017). In summary, it is likely that polymorphisms and/or mutations that impact the expression/function of many of the genes coupled to the patterning of the pharyngeal apparatus as well as neurological processes will be identified based on their capacity to modulate the clinical manifestations of 22q11.2del.
A further observation from the different mouse models is the strain dependent severity of the CHD and thymic anomalies, which reveals the effects of genetic differences (Taddei et al., 2001). Thus, the original 129SvEv background in which most of the 22q11.2del mouse models were generated has the lowest penetrance of the congenital malformations (Kimber et al., 1999; Lindsay et al., 1999; Jerome and Papaioannou, 2001; Lindsay et al., 2001; Merscher et al., 2001). Backcrossing these mice onto the C57BL/6 background yields a more penetrant phenotype, particularly in regards to the thymic hypoplasia (Taddei et al., 2001). Of relevance, this strain of mice is more sensitive to stress, as assessed by the higher corticosterone levels in the blood (Ramírez-Rosas et al., 2019). Children with 22q11.2del have higher levels of cortisol relative to age-matched controls (Jacobson et al., 2016). Elevations in cortisol can reduce T cell output from the thymus (Belkaya et al., 2011). Studies in a zebrafish model suggest elevated cortisol levels during embryogenesis are detrimental to cardiac functions (Nesan and Vijayan, 2012). The connection between stress, tissue repair and regeneration, and miRNAs is well-established, and the dysregulation of miRNAs described above will likely be impacted by the cortisol levels in 22q11.2del patients. Finally, the severity and penetrance of the congenital malformations may be related to epigenetic modifiers that affect tissue repair and regeneration during embryogenesis. Notably, the 4th pharyngeal arch artery is either missing and/or poorly developed in nearly 100% of the murine 22q11.2 del embryos when assessed at embryonic e10.5 (equivalent to human embryonic week 7) (Lindsay and Baldini, 2001; Karpinski et al., 2014). However, this penetrance drops dramatically between e12.5-e18.5, such that only 30% of the 22q11.2del pups have CHD at term (Lindsay and Baldini, 2001). This indicates a regenerative or corrective process in genetically identical embryos, which could be explained by epigenetic modifiers, a possibility that requires further analysis.
Independent Chromosomal Deletions in Relation to 22q11.2del
In an analysis of >1400 22q11.2del patients, 1% were found to have secondary mutations at other loci (Michaud et al., 1995; Mcdonald-Mcginn et al., 2013; Kruszka et al., 2017). These secondary mutations, including some affecting the presumed normal allele of chromosome 22q11.2, are resulting in dual diagnoses (Cohen et al., 2018). A few of the more prominent diagnoses include CHARGE (Coloboma, heart defects, atresia choanae, growth retardation, genital and ear anomalies) syndrome, cystic fibrosis, G6PD deficiency, 17q12 deletion syndrome, and von Willebrand disease (Michaud et al., 1995; Mcdonald-Mcginn et al., 2013; Cohen et al., 2018). Whether these secondary mutations at distinct loci influence the expression of genes on chromosome 22q11.2 has not been assessed. There are also reports of patients with 22q11.2del-like phenotypes due to chromosomal deletions at unrelated loci. Among these are microdeletions on 10p13, 4q34, and 3p12.3 (Table 1). Terminal deletions on the short arm of chromosome 10 cause 10p syndrome. Described in over 46 patients, the phenotypes are split into two: DiGeorge syndrome 2 (DGS2) (OMIM# 601362) and hypoparathyroidism, sensorineural deafness and renal dysplasia (HDR) (OMIM# 146255) (Van Esch et al., 2000). DGS2 results from haploinsufficiency of the more proximal region of 10p13-10p14. The resulting cardiac anomalies and thymic hypoplasia are coupled to a haploinsufficiency of BRUNOL3 (NAPOR, CUGBP2, ETR3) (Lichtner et al., 2002). HDR is a separate syndrome involving deletions in the more terminal region of chromosome 10 (10p14-10pter) that result in a hemizygosity of GATA3 (Lindstrand et al., 2010). Chromosomal deletions of the terminal end of 4q34 cause chromosome 4q syndrome, with at least one patient reported with clinical phenotypes of 22q11.2del (Cuturilo et al., 2011; Strehle et al., 2012). A 371-kb interstitial deletion of 3p12.3 was reported for a male child with a clinical presentation of 22q11.2del (Cirillo et al., 2017). Two miRNAs, miR-1243 and miR-4273 along with the Zinc Finger Protein 717 (ZNF717) are affected by this deletion, resulting in DiGeorge-like syndrome and renal insufficiency. Given the clinical overlap among these different microdeletions, it is anticipated that several may affect TBX1 expression and/or modulate histone modifications among cells differentiating within the pharyngeal region.
Summary
Rapid advances in our understanding of the molecular mechanisms underpinning the heterogeneous clinical presentations of 22q11.2del are creating exciting new possibilities for earlier diagnosis and better treatment strategies for affected individuals. The introduction of TREC testing as part of newborn screening throughout the US and in other countries has accelerated the time when infants with 22q11.2del are diagnosed, and this will mean in more rapid and appropriate clinical interventions (Dorsey et al., 2017). Innovations in whole genome sequencing and DNA-based microarrays also make possible an accurate diagnosis of 22q11.2del in the developing fetus using maternal blood sampling (Yatsenko et al., 2015; Schmid et al., 2017). These non-invasive prenatal tests are very sensitive and have low false positive discovery rates (Ravi et al., 2018). Improvements in sequencing limited amounts of fetal DNA from maternal sampling is moving the field to 1st trimester screening (Srinivasan et al., 2013; Helgeson et al., 2015) (ClinicalTrials.gov Identifier: NCT03375359). This is important because the pharyngeal defects arise during this period. Mitigating the damage to the pharyngeal region in TBX1-haploinsufficient embryos at this time point will definitely improve clinical outcomes. In the post-natal period, managing physiological stress should improve vulnerabilities due to miRNA dysregulation, arising from the reduced levels of DGCR8. The identification of small drugs able to modulate the consequences of the haploinsufficiency of both TBX1 and DGCR8 suggest new strategies for reducing the clinical penetrance and severity of 22q11.2del (Barr et al., 2015; Fulcoli et al., 2016). In summary, DNA and RNA sequencing approaches are unveiling new genetic and epigenetic modifiers among the 22q11.2del cohort that have a significant impact on disease penetrance and severity. Identifying and understanding these genetic and epigenetic regulators in an individualized manner for each 22q11.2del patient will direct future care in order to minimize disease severity.
Author Contributions
All authors, QD, MM, and NO contributed to the review of the literature, the writing of the manuscript, and the preparation of data in tables and figures.
Funding
Our work was supported, in part, by grants from the National Institutes of Health R01 (R01 AI114523, R21 AI144140 NO), Beecherl funds from the Department of Immunology at UT Southwestern Medical Center (NO), and the Jeffrey Modell Foundation (MM).
Conflict of Interest
The authors declare that the research was conducted in the absence of any commercial or financial relationships that could be construed as a potential conflict of interest.
Acknowledgments
We would like to thank Drs. M Louise Markert (Duke University) and Robert P. Nelson (Indiana University School of Medicine) for helpful discussions and information. We also thank Dr. Pratibha Bhalla and Ms. Fatma Coskun (UT Southwestern Medical Center) for reviewing the figures in the manuscript.
References
Alfano, D., Altomonte, A., Cortes, C., Bilio, M., Kelly, R. G., Baldini, A. (2019). Tbx1 regulates extracellular matrix-cell interactions in the second heart field. Hum. Mol. Genet. 28, 2295–2308. doi: 10.1093/hmg/ddz058
Alles, J., Fehlmann, T., Fischer, U., Backes, C., Galata, V., Minet, M., et al. (2019). An estimate of the total number of true human miRNAs. Nucleic Acids Res. 47, 3353–3364. doi: 10.1093/nar/gkz097
Angrand, P.-O., Vennin, C., Le Bourhis, X., Adriaenssens, E. (2015). The role of long non-coding RNAs in genome formatting and expression. Front. Genet. 6, 165. doi: 10.3389/fgene.2015.00165
Baldini, A., Fulcoli, F. G., Illingworth, E. (2016). Tbx1: transcriptional and developmental functions. Curr. Topics Dev. Biol 122, 223–243. doi: 10.1016/bs.ctdb.2016.08.002
Barr, I., Weitz, S. H., Atkin, T., Hsu, P., Karayiorgou, M., Gogos, J. A., et al. (2015). Cobalt(III) Protoporphyrin activates the DGCR8 protein and can compensate microRNA processing deficiency. Chem. Biol. 22, 793–802. doi: 10.1016/j.chembiol.2015.05.015
Bartel, D. P. (2009). MicroRNAs: target recognition and regulatory functions. Cell 136. doi: 10.1016/j.cell.2009.01.002
Bassett, A. S., Chow, E. W., Husted, J., Weksberg, R., Caluseriu, O., Webb, G. D., et al. (2005). Clinical features of 78 adults with 22q11 deletion syndrome. Am. J. Med. Genet. A. 138, 307–313. doi: 10.1002/ajmg.a.30984
Bassett, A. S., Chow, E. W. C., Husted, J., Hodgkinson, K. A., Oechslin, E., Harris, L., et al. (2009). Premature death in adults with 22q11.2 deletion syndrome. J. Med. Genet. 46, 324–330. doi: 10.1136/jmg.2008.063800
Bassett, A. S., Mcdonald-Mcginn, D. M., Devriendt, K., Digilio, M. C., Goldenberg, P., Habel, A., et al. (2011). Practical guidelines for managing patients with 22q11.2 deletion syndrome. J. Pediatr. 159, 332–339 e331. doi: 10.1016/j.jpeds.2011.02.039
Bastos Maia, S., Rolland Souza, A. S., Costa Caminha, M. F., Lins Da Silva, S., Callou Cruz, R., Carvalho Dos Santos, C., et al. (2019). Vitamin A and pregnancy: a narrative review. Nutrients 11. doi: 10.3390/nu11030681
Belkaya, S., Silge, R. L., Hoover, A. R., Medeiros, J. J., Eitson, J. L., Becker, A. M., et al. (2011). Dynamic modulation of thymic microRNAs in response to stress. PloS One 6, e27580. doi: 10.1371/journal.pone.0027580
Belkaya, S., Murray, S. E., Eitson, J. L., De La Morena, M. T., Forman, J. A., Van Oers, N. S. (2013). Transgenic expression of microRNA-185 causes a developmental arrest of T cells by targeting multiple genes including Mzb1. J. Biol. Chem. 288, 30752–30762. doi: 10.1074/jbc.M113.503532
Belver, L., De Yebenes, V. G., Ramiro, A. R. (2010). MicroRNAs prevent the generation of autoreactive antibodies. Immunity 33, 713–722. doi: 10.1016/j.immuni.2010.11.010
Bertini, V., Azzarà, A., Legitimo, A., Milone, R., Battini, R., Consolini, R., et al. (2017). Deletion extents are not the cause of clinical variability in 22q11.2 deletion syndrome: does the interaction between DGCR8 and miRNA-CNVs play a major role? Front. Genet. 8, 47. doi: 10.3389/fgene.2017.00047
Bittel, D. C., Yu, S., Newkirk, H., Kibiryeva, N., Holt, A., 3rd, Butler, M. G., et al. (2009). Refining the 22q11.2 deletion breakpoints in DiGeorge syndrome by aCGH. Cytogenet. Genome Res. 124, 113–120. doi: 10.1159/000207515
Botto, L. D., May, K., Fernhoff, P. M., Correa, A., Coleman, K., Rasmussen, S. A., et al. (2003). A population-based study of the 22q11.2 deletion: phenotype, incidence, and contribution to major birth defects in the population. Pediatrics 112, 101–107. doi: 10.1542/peds.112.1.101
Brenner, M. K., Clarke, S., Mahnke, D. K., Simpson, P., Bercovitz, R. S., Tomita-Mitchell, A., et al. (2016). Effect of 22q11.2 deletion on bleeding and transfusion utilization in children with congenital heart disease undergoing cardiac surgery. Pediatr. Res. 79, 318–324. doi: 10.1038/pr.2015.216
Browne, H., Mason, G., Tang, T. (2014). Retinoids and pregnancy: an update. Obstetrician Gynaecolog. 16, 7–11. doi: 10.1111/tog.12075.
Burnside, R. D. (2015). 22q11.21 deletion syndromes: a review of proximal, central, and distal deletions and their associated features. Cytogenet. Genome Res. 0, 89–99. doi: 10.1159/000438708
Cesana, M., Cacchiarelli, D., Legnini, I., Santini, T., Sthandier, O., Chinappi, M., et al. (2011). A long noncoding RNA controls muscle differentiation by functioning as a competing endogenous RNA. Cell 147, 358–369. doi: 10.1016/j.cell.2011.09.028
Chakraborty, D., Bernal, A. J., Schoch, K., Howard, T. D., Ip, E. H., Hooper, S. R., et al. (2012). Dysregulation of DGCR6 and DGCR6L: psychopathological outcomes in chromosome 22q11.2 deletion syndrome. Trans. Psychiatry 2, e105. doi: 10.1038/tp.2012.31
Chen, L., Fulcoli, F. G., Ferrentino, R., Martucciello, S., Illingworth, E. A., Baldini, A. (2012). Transcriptional control in cardiac progenitors: Tbx1 interacts with the BAF chromatin remodeling complex and regulates Wnt5a. PloS Genet. 8, e1002571. doi: 10.1371/journal.pgen.1002571
Chen, E.-G. G., Zhang, J.-S. S., Xu, S., Zhu, X.-J. J., Hu, H.-H. H. (2017). Long non-coding RNA DGCR5 is involved in the regulation of proliferation, migration and invasion of lung cancer by targeting miR-1180. Am. J. Cancer Res. 7, 1463–1475.
Cipollone, D., Amati, F., Carsetti, R., Placidi, S., Biancolella, M., D’amati, G., et al. (2006). A multiple retinoic acid antagonist induces conotruncal anomalies, including transposition of the great arteries, in mice. Cardiovasc. Pathol. Off. J. Soc. Cardiovasc. Pathol. 15, 194–202. doi: 10.1016/j.carpath.2006.04.004
Cirera-Salinas, D., Yu, J., Bodak, M., Ngondo, R. P., Herbert, K. M., Ciaudo, C. (2017). Noncanonical function of DGCR8 controls mESC exit from pluripotency. J. Cell Biol. 216, 355–366. doi: 10.1083/jcb.201606073
Cirillo, E., Giardino, G., Gallo, V., Galasso, G., Romano, R., D’assante, R., et al. (2017). DiGeorge-like syndrome in a child with a 3p12.3 deletion involving MIR4273 gene born to a mother with gestational diabetes mellitus. Am. J. Med. Genet. Part A. 173, 1913–1918. doi: 10.1002/ajmg.a.38242
Cohen, J. L., Crowley, T. B., Mcginn, D. E., Mcdougall, C., Unolt, M., Lambert, M. P., et al. (2018). 22q and two: 22q11.2 deletion syndrome and coexisting conditions. Am. J. Med. Genet. Part A. 176, 2203–2214. doi: 10.1002/ajmg.a.40494
Conley, M. E., Beckwith, J. B., Mancer, J. F., Tenckhoff, L. (1979). The spectrum of the DiGeorge syndrome. J. Pediatr. 94, 883–890. doi: 10.1016/s0022-3476(79)80207-3
Consortium, T., Mlynarski, E. E., Xie, M., Taylor, D., Sheridan, M. B., Guo, T., et al. (2016). Rare copy number variants and congenital heart defects in the 22q11.2 deletion syndrome. Hum. Genet. 135, 273–285. doi: 10.1007/s00439-015-1623-9
Cuturilo, G., Menten, B., Krstic, A., Drakulic, D., Jovanovic, I., Parezanovic, V., et al. (2011). 4q34.1–q35.2 deletion in a boy with phenotype resembling 22q11.2 deletion syndrome. Eur. J. Pediatr. 170, 1465–1470. doi: 10.1007/s00431-011-1533-3
De La Morena, M. T., Eitson, J. L., Dozmorov, I. M., Belkaya, S., Hoover, A. R., Anguiano, E., et al. (2013). Signature MicroRNA expression patterns identified in humans with 22q11.2 deletion/DiGeorge syndrome. Clin. Immunol. 147, 11–22. doi: 10.1016/j.clim.2013.01.011
Delihas, N. (2018). A family of long intergenic non-coding RNA genes in human chromosomal region 22q11.2 carry a DNA translocation breakpoint/AT-rich sequence. PloS One 13, e0195702. doi: 10.1371/journal.pone.0195702
Dey, B. K., Pfeifer, K., Dutta, A. (2014). The H19 long noncoding RNA gives rise to microRNAs miR-675-3p and miR-675-5p to promote skeletal muscle differentiation and regeneration. Genes Dev. 28, 491–501. doi: 10.1101/gad.234419.113
Dornemann, R., Koch, R., Mollmann, U., Falkenberg, M. K., Mollers, M., Klockenbusch, W., et al. (2017). Fetal thymus size in pregnant women with diabetic diseases. J. Perinat. Med. 45, 595–601. doi: 10.1515/jpm-2016-0400
Dorsey, M. J., Dvorak, C. C., Cowan, M. J., Puck, J. M. (2017). Treatment of infants identified as having severe combined immunodeficiency by means of newborn screening. J. Allergy Clin. Immunol. 139, 733–742. doi: 10.1016/j.jaci.2017.01.005
Du, Q., Hoover, A. R., Dozmorov, I., Raj, P., Khan, S., Molina, E., et al. (2019). MIR205HG is a long noncoding RNA that regulates growth hormone and prolactin production in the anterior pituitary. Dev. Cell 49618–631, e615. doi: 10.1016/j.devcel.2019.03.012
Dupuis-Sandoval, F., Poirier, M., Scott, M. S. (2015). The emerging landscape of small nucleolar RNAs in cell biology. Wiley Interdiscip. Rev.: RNA 6, 381–397. doi: 10.1002/wrna.1284
Earls, L., Bayazitov, I., Fricke, R., Berry, R., Illingworth, E., Mittleman, G., et al. (2010). Dysregulation of presynaptic calcium and synaptic plasticity in a mouse model of 22q11 deletion syndrome. J. Neurosci. Off. J. Soc. Neurosci. 30, 15843–15855. doi: 10.1523/JNEUROSCI.1425-10.2010
Earls, L. R., Fricke, R. G., Yu, J., Berry, R. B., Baldwin, L. T., Zakharenko, S. S. (2012). Age-dependent MicroRNA control of synaptic plasticity in 22q11 deletion syndrome and schizophrenia. J. Neurosci. Off. J. Soc. Neurosci. 32, 14132–14144. doi: 10.1523/JNEUROSCI.1312-12.2012
Ensenauer, R. E., Adeyinka, A., Flynn, H. C., Michels, V. V., Lindor, N. M., Dawson, D. B., et al. (2003). Microduplication 22q11.2, an emerging syndrome: clinical, cytogenetic, and molecular analysis of thirteen patients. Am. J. Hum. Genet. 73, 1027–1040. doi: 10.1086/378818
Fulcoli, F. G., Huynh, T., Scambler, P. J., Baldini, A. (2009). Tbx1 regulates the BMP-Smad1 pathway in a transcription independent manner. PloS One 4, e6049–e6049. doi: 10.1371/journal.pone.0006049
Fulcoli, F., Franzese, M., Liu, X., Zhang, Z., Angelini, C., Baldini, A. (2016). Rebalancing gene haploinsufficiency in vivo by targeting chromatin. Nat. Commun. 7, 11688. doi: 10.1038/ncomms11688
Fung, W. L., Butcher, N. J., Costain, G., Andrade, D. M., Boot, E., Chow, E. W., et al. (2015). Practical guidelines for managing adults with 22q11.2 deletion syndrome. Genet. Med. 17, 599–609. doi: 10.1038/gim.2014.175
Gao, S., Moreno, M., Eliason, S., Cao, H., Li, X., Yu, W., et al. (2015a). TBX1 protein interactions and microRNA-96-5p regulation controls cell proliferation during craniofacial and dental development: implications for 22q11.2 deletion syndrome. Hum. Mol. Genet. 24, 2330–2348. doi: 10.1093/hmg/ddu750
Gao, W., Higaki, T., Eguchi-Ishimae, M., Iwabuki, H., Wu, Z., Yamamoto, E., et al. (2015b). DGCR6 at the proximal part of the DiGeorge critical region is involved in conotruncal heart defects. Hum. Genome Variation 2, 15004. doi: 10.1038/hgv.2015.4
Gordon, J., Patel, S. R., Mishina, Y., Manley, N. R. (2008). Evidence for an early role for BMP4 signaling in thymus and parathyroid morphogenesis. Dev. Biol. 339, 141–154. doi: 10.1016/j.ydbio.2009.12.026
Grati, F. R., Molina Gomes, D., Ferreira, J. C. P. B., Dupont, C., Alesi, V., Gouas, L., et al. (2015). Prevalence of recurrent pathogenic microdeletions and microduplications in over 9500 pregnancies. Prenatal Diagnosis 35, 801–809. doi: 10.1002/pd.4613
Groot, A. C., Hierck, B. P., Molin, D. G. M., Boot, M. J., Poelmann, R. E. (2004). A chicken model for DGCR6 as a modifier gene in the DiGeorge critical region. Pediatr. Res. 56, 440–448. doi: 10.1203/01.PDR.0000136151.50127.1C
Grosshans, H., Filipowicz, W. (2008). Molecular biology: the expanding world of small RNAs. Nature 451, 414–416. doi: 10.1038/451414a
Guna, A., Butcher, N. J., Bassett, A. S. (2015). Comparative mapping of the 22q11.2 deletion region and the potential of simple model organisms. J. Neurodev. Disord. 7, 1–16. doi: 10.1186/s11689-015-9113-x
Guo, T., Chung, J. H., Wang, T., Mcdonald-Mcginn, D. M., Kates, W. R., Hawuła, W., et al. (2015). Histone modifier genes alter conotruncal heart phenotypes in 22q11.2 deletion syndrome. Am. J. Hum. Genet. 97, 869–877. doi: 10.1016/j.ajhg.2015.10.013
Haertle, L., El Hajj, N., Dittrich, M., Müller, T., Nanda, I., Lehnen, H., et al. (2017). Epigenetic signatures of gestational diabetes mellitus on cord blood methylation. Clin. Epigenet. 9, 28. doi: 10.1186/s13148-017-0329-3
He, Z.-J., Li, W., Chen, H., Wen, J., Gao, Y.-F., Liu, Y.-J. (2018). miR-1306–3p targets FBXL5 to promote metastasis of hepatocellular carcinoma through suppressing snail degradation. Biochem. Biophys. Res. Commun. 504, 820–826. doi: 10.1016/j.bbrc.2018.09.059
Helgeson, J., Wardrop, J., Boomer, T., Almasri, E., Paxton, W. B., Saldivar, J. S., et al. (2015). Clinical outcome of subchromosomal events detected by whole-genome noninvasive prenatal testing. Prenatal Diagnosis 35, 999–1004. doi: 10.1002/pd.4640
Hwang, V. J., Maar, D., Regan, J., Angkustsiri, K., Simon, T. J., Tassone, F. (2014). Mapping the deletion endpoints in individuals with 22q11.2 deletion syndrome by droplet digital PCR. BMC Med. Genet. 15, 106. doi: 10.1186/s12881-014-0106-5
Jacobson, D., Bursch, M., Lajiness-O’neill, R. (2016). Potential role of Cortisol in social and memory impairments in individuals with 22q11.2 deletion syndrome. J. Pediatr. Genet. 5, 150–157. doi: 10.1055/s-0036-1584549
Jenkinson, W. E., Rossi, S. W., Parnell, S. M., Jenkinson, E. J., Anderson, G. (2007). PDGFRalpha-expressing mesenchyme regulates thymus growth and the availability of intrathymic niches. Blood 109, 954–960. doi: 10.1182/blood-2006-05-023143
Jerome, L. A., Papaioannou, V. E. (2001). DiGeorge syndrome phenotype in mice mutant for the T-box gene, Tbx1. Nat. Genet. 27, 286–291. doi: 10.1038/85845
Jones, N. C., Farlie, P. G., Minichiello, J., Newgreen, D. F. (1999). Detection of an appropriate kinase activity in branchial arches I and II that coincides with peak expression of the treacher collins syndrome gene product, treacle. Hum. Mol. Genet. 8, 2239–2245. doi: 10.1093/hmg/8.12.2239
Karayiorgou, M., Morris, M. A., Morrow, B., Shprintzen, R. J., Goldberg, R., Borrow, J., et al. (1995). Schizophrenia susceptibility associated with interstitial deletions of chromosome 22q11. Proc. Natl. Acad. Sci. U. S. A. 92, 7612–7616. doi: 10.1073/pnas.92.17.7612
Karayiorgou, M., Simon, T. J., Gogos, J. A. (2010). 22q11.2 microdeletions: linking DNA structural variation to brain dysfunction and schizophrenia. Nat. Rev. Neurosci. 11, 402–416. doi: 10.1038/nrn2841
Karpinski, B. A., Maynard, T. M., Fralish, M. S., Nuwayhid, S., Zohn, I. E., Moody, S. A., et al. (2014). Dysphagia and disrupted cranial nerve development in a mouse model of DiGeorge (22q11) deletion syndrome. Dis. Models Mech. 7, 245–257. doi: 10.1242/dmm.012484
Keniry, A., Oxley, D., Monnier, P., Kyba, M., Dandolo, L., Smits, G., et al. (2012). The H19 lincRNA is a developmental reservoir of miR-675 that suppresses growth and Igf1r. Nat. Cell Biol. 14, 659–665. doi: 10.1038/ncb2521
Kim, J. O., Song, D. W., Kwon, E. J., Hong, S.-E., Song, H. K., Min, C. K., et al. (2015). miR-185 plays an anti-hypertrophic role in the heart via multiple targets in the calcium-signaling pathways. PloS One 10, e0122509. doi: 10.1371/journal.pone.0122509
Kimber, W. L., Hsieh, P., Hirotsune, S., Yuva-Paylor, L., Sutherland, H. F., Chen, A., et al. (1999). Deletion of 150 kb in the minimal DiGeorge/velocardiofacial syndrome critical region in mouse. Hum. Mol. Genet. 8, 2229–2237. doi: 10.1093/hmg/8.12.2229
Kobrynski, L. J., Sullivan, K. E. (2007). Velocardiofacial syndrome, DiGeorge syndrome: the chromosome 22q11.2 deletion syndromes. Lancet 370, 1443–1452. doi: 10.1016/S0140-6736(07)61601-8
Krol, J., Loedige, I., Filipowicz, W. (2010). The widespread regulation of microRNA biogenesis, function and decay. Nat. Rev. Genet. 11. doi: 10.1038/nrg2843
Kruszka, P., Addissie, Y. A., Mcginn, D. E., Porras, A. R., Biggs, E., Share, M., et al. (2017). 22q11.2 deletion syndrome in diverse populations. Am. J. Med. Genet. Part A. 173, 879–888. doi: 10.1002/ajmg.a.38199
Kucera, J. (1971). Rate and type of congenital anomalies among offspring of diabetic women. J. Reprod. Med. 7, 73–82.
Kwan, A., Abraham, R. S., Currier, R., Brower, A., Andruszewski, K., Abbott, J. K., et al. (2014). Newborn screening for severe combined immunodeficiency in 11 screening programs in the United States newborn screening for severe combined immunodeficiency newborn screening for severe combined immunodeficiency. JAMA 312, 729–738. doi: 10.1001/jama.2014.9132
Lambrechts, D., Devriendt, K., Driscoll, D. A., Goldmuntz, E., Gewillig, M., Vlietinck, R., et al. (2005). Low expression VEGF haplotype increases the risk for tetralogy of Fallot: a family based association study. J. Med. Genet. 42, 519–522. doi: 10.1136/jmg.2004.026443
Lammer, E. J., Chen, D. T., Hoar, R. M., Agnish, N. D., Benke, P. J., Braun, J. T., et al. (1985). Retinoic acid embryopathy. New Engl. J. Med. 313, 837–841. doi: 10.1056/NEJM198510033131401
Landthaler, M., Yalcin, A., Tuschl, T. (2004). The human DiGeorge syndrome critical region gene 8 and Its D. melanogaster homolog are required for miRNA biogenesis. Curr. Biol. 14, 2162–2167. doi: 10.1016/j.cub.2004.11.001
León, L. E., Benavides, F., Espinoza, K., Vial, C., Alvarez, P., Palomares, M., et al. (2017). Partial microduplication in the histone acetyltransferase complex member KANSL1 is associated with congenital heart defects in 22q11.2 microdeletion syndrome patients. Sci. Rep. 7, 1795. doi: 10.1038/s41598-017-01896-w
Lee, Y., Ahn, C., Han, J., Choi, H., Kim, J., Yim, J., et al. (2003). The nuclear RNase III Drosha initiates microRNA processing. Nature 425. doi: 10.1038/nature01957
Lee, S., Kopp, F., Chang, T.-C., Sataluri, A., Chen, B., Sivakumar, S., et al. (2016). Noncoding RNA NORAD regulates genomic stability by sequestering PUMILIO proteins. Cell 164, 69–80. doi: 10.1016/j.cell.2015.12.017
Lee, L. M. Y., Leung, M. B. W., Kwok, R. C. Y., Leung, Y. C., Wang, C. C., Mccaffery, P. J., et al. (2017). Perturbation of retinoid homeostasis increases malformation risk in embryos exposed to pregestational diabetes. Diabetes 66, 1041–1051. doi: 10.2337/db15-1570
Leung, A. K., Sharp, P. A. (2010). MicroRNA functions in stress responses. Mol. Cell 40, 205–215. doi: 10.1016/j.molcel.2010.09.027
Lewis, B. P., Burge, C. B., Bartel, D. P. (2005). Conserved seed pairing, often flanked by adenosines, indicates that thousands of human genes are microRNA targets. Cell 120, 15–20. doi: 10.1016/j.cell.2004.12.035
Lichtner, P., Attié-Bitach, T., Schuffenhauer, S., Henwood, J., Bouvagnet, P., Scambler, P., et al. (2002). Expression and mutation analysis of BRUNOL3, a candidate gene for heart and thymus developmental defects associated with partial monosomy 10p. J. Mol. Med. 80, 431–442. doi: 10.1007/s00109-002-0331-9
Lindsay, E. A., Baldini, A. (2001). Recovery from arterial growth delay reduces penetrance of cardiovascular defects in mice deleted for the DiGeorge syndrome region. Hum. Mol. Genet. 10, 997–1002. doi: 10.1093/hmg/10.9.997
Lindsay, E. A., Botta, A., Jurecic, V., Carattini-Rivera, S., Cheah, Y. C., Rosenblatt, H. M., et al. (1999). Congenital heart disease in mice deficient for the DiGeorge syndrome region. Nature 401, 379–383. doi: 10.1038/43900
Lindsay, E. A., Vitelli, F., Su, H., Morishima, M., Huynh, T., Pramparo, T., et al. (2001). Tbx1 haploinsufficieny in the DiGeorge syndrome region causes aortic arch defects in mice. Nature 410, 97–101. doi: 10.1038/35065105
Lindstrand, A., Malmgren, H., Verri, A., Benetti, E., Eriksson, M., Nordgren, A., et al. (2010). Molecular and clinical characterization of patients with overlapping 10p deletions. Am. J. Med. Genet. Part A. 152A, 1233–1243. doi: 10.1002/ajmg.a.33366
Lipson, A. H., Collins, F., Webster, W. S. (1993). Multiple congenital defects associated with maternal use of topical tretinoin. Lancet 341, 1352–1353. doi: 10.1016/0140-6736(93)90868-h
Liu, W., Selever, J., Wang, D., Lu, M.-F., Moses, K. A., Schwartz, R. J., et al. (2004). Bmp4 signaling is required for outflow-tract septation and branchial-arch artery remodeling. Proc. Natl. Acad. Sci. U. S. A. 101, 4489–4494. doi: 10.1073/pnas.0308466101
Liu, W.-H., Tsai, Z., Tsai, H.-K. (2017). Comparative genomic analyses highlight the contribution of pseudogenized protein-coding genes to human lincRNAs. BMC Genomics 18, 786. doi: 10.1186/s12864-017-4156-x
Lodish, H. F., Zhou, B., Liu, G., Chen, C. Z. (2008). Micromanagement of the immune system by microRNAs. Nat. Rev. Immunol. 8, 120–130. doi: 10.1038/nri2252
Marcovecchio, G., Bortolomai, I., Ferrua, F., Fontana, E., Imberti, L., Conforti, E., et al. (2019). Thymic Epithelium abnormalities in DiGeorge and down syndrome patients contribute to dysregulation in T cell development. Front. Immunol. 10, 447. doi: 10.3389/fimmu.2019.00447
Markert, M. L., Devlin, B. H., Alexieff, M. J., Li, J., Mccarthy, E. A., Gupton, S. E., et al. (2007). Review of 54 patients with complete DiGeorge anomaly enrolled in protocols for thymus transplantation: outcome of 44 consecutive transplants. Blood 109, 4539–4547. doi: 10.1182/blood-2006-10-048652
Mcdonald-Mcginn, D. M., Kirschner, R., Goldmuntz, E., Sullivan, K., Eicher, P., Gerdes, M., et al. (1999). The Philadelphia story: the 22q11.2 deletion: report on 250 patients. Genet. Couns. 10, 11–24.
Mcdonald-Mcginn, D. M., Reilly, A., Wallgren-Pettersson, C., Hoyme, H. E., Yang, S. P., Adam, M. P., et al. (2006). Malignancy in chromosome 22q11.2 deletion syndrome (DiGeorge syndrome/velocardiofacial syndrome). Am. J. Med. Genet. A. 140, 906–909. doi: 10.1002/ajmg.a.31199
Mcdonald-Mcginn, D. M., Fahiminiya, S., Revil, T., Nowakowska, B. A., Suhl, J., Bailey, A., et al. (2013). Hemizygous mutations in SNAP29 unmask autosomal recessive conditions and contribute to atypical findings in patients with 22q11.2DS. J. Med. Genet. 50, 80–90. doi: 10.1136/jmedgenet-2012-101320
Mcdonald-Mcginn, D. M., Sullivan, K. E., Marino, B., Philip, N., Swillen, A., Vorstman, J. A. S., et al. (2015). 22q11.2 deletion syndrome. Nat. Rev. Dis. Primers 15071. doi: 10.1038/nrdp.2015.71
Mcmahon, M., Contreras, A., Ruggero, D. (2015). Small RNAs with big implications: new insights into H/ACA snoRNA function and their role in human disease. Wiley Interdiscip. Rev.: RNA 6, 173–189. doi: 10.1002/wrna.1266
Meechan, D. W., Maynard, T. M., Tucker, E. S., Fernandez, A., Karpinski, B. A., Rothblat, L. A., et al. (2015). Modeling a model: mouse genetics, 22q11.2 deletion syndrome, and disorders of cortical circuit development. Prog. Neurobiol. 130, 1–28. doi: 10.1016/j.pneurobio.2015.03.004
Mencia, A., Modamio-Hoybjor, S., Redshaw, N., Morin, M., Mayo-Merino, F., Olavarrieta, L., et al. (2009). Mutations in the seed region of human miR-96 are responsible for nonsyndromic progressive hearing loss. Nat. Genet. 41, 609–613. doi: 10.1038/ng.355
Mendell, J. T., Olson, E. N. (2012). MicroRNAs in stress signaling and human disease. Cell 148, 1172–1187. doi: 10.1016/j.cell.2012.02.005
Merico, D., Costain, G., Butcher, N. J., Warnica, W., Ogura, L., Alfred, S. E., et al. (2014). MicroRNA dysregulation, gene networks, and risk for schizophrenia in 22q11.2 deletion syndrome. Front. Neurol. 5, 238. doi: 10.3389/fneur.2014.00238
Merscher, S., Funke, B., Epstein, J. A., Heyer, J., Puech, A., Lu, M. M., et al. (2001). TBX1 is responsible for cardiovascular defects in velo-cardio-facial/DiGeorge syndrome. Cell 104, 619–629. doi: 10.1016/s0092-8674(01)00247-1
Michaud, D., Mcdonald-Mcginn, D., Li, M., Budarf, M. L., Zackai, E. H., Driscoll, D. A., et al. (1995). Identification of a patient with Bernard-Soulier syndrome and a deletion in the DiGeorge/Velo-cardio-facial chromosomal region in 22q11.2. Hum. Mol. Genet. 4, 763–766. doi: 10.1093/hmg/4.4.763
Mills, J. (2010). Malformations in infants of diabetic mothers. Birth Defects Res. Part A.: Clin. Mol. Teratol. 88, 769–778.
Mlynarski, E. E., Sheridan, M. B., Xie, M., Guo, T., Racedo, S. E., Mcdonald-Mcginn, D. M., et al. (2015). Copy-number variation of the glucose transporter gene SLC2A3 and congenital heart defects in the 22q11.2 deletion syndrome. Am. J. Hum. Genet. 96, 753–764. doi: 10.1016/j.ajhg.2015.03.007
Mokate, T., Leask, K., Mehta, S., Sharif, S., Smith, A., Saxena, A., et al. (2006). Non-mosaic trisomy 22: a report of 2 cases. Prenatal Diagnosis 26, 962–965. doi: 10.1002/pd.1537
Moreno-Igoa, M., Hernández-Charro, B., Bengoa-Alonso, A., Pérez-Juana-Del-Casal, A., Romero-Ibarra, C., Nieva-Echebarria, B., et al. (2015). KANSL1 gene disruption associated with the full clinical spectrum of 17q21.31 microdeletion syndrome. BMC Med. Genet. 16, 68. doi: 10.1186/s12881-015-0211-0
Morrow, B. E., Mcdonald-Mcginn, D. M., Emanuel, B. S., Vermeesch, J. R., Scambler, P. J. (2018). Molecular genetics of 22q11.2 deletion syndrome. Am. J. Med. Genet. Part A. 176, 2070–2081. doi: 10.1002/ajmg.a.40504
Morsheimer, M., Whitehorn, T. F., Heimall, J., Sullivan, K. E. (2017). The immune deficiency of chromosome 22q11.2 deletion syndrome. Am. J. Med. Genet. Part A. doi: 10.1002/ajmg.a.38319
Munk, R., Martindale, J. L., Yang, X., Yang, J.-H., Grammatikakis, I., Di Germanio, C., et al. (2019). Loss of miR-451a enhances SPARC production during myogenesis. PloS One 14, e0214301. doi: 10.1371/journal.pone.0214301
Nesan, D., Vijayan, M. M. (2012). Embryo exposure to elevated cortisol level leads to cardiac performance dysfunction in zebrafish. Mol. Cell. Endocrinol. 363, 85–91. doi: 10.1016/j.mce.2012.07.010
Niederreither, K., Vermot, J., Le Roux, I., Schuhbaur, B., Chambon, P., Dolle, P. (2003). The regional pattern of retinoic acid synthesis by RALDH2 is essential for the development of posterior pharyngeal arches and the enteric nervous system. Development 130, 2525–2534. doi: 10.1242/dev.00463
Nowakowski, T. J., Rani, N., Golkaram, M., Zhou, H. R., Alvarado, B., Huch, K., et al. (2018). Regulation of cell-type-specific transcriptomes by microRNA networks during human brain development. Nat. Neurosci. 21, 1784–1792. doi: 10.1038/s41593-018-0265-3
Óskarsdóttir, S., Persson, C., Eriksson, B. O., Fasth, A. (2005). Presenting phenotype in 100 children with the 22q11 deletion syndrome. Eur. J. Pediatr. 164, 146–153. doi: 10.1007/s00431-004-1577-8
Padmanabhan, R., Shafiullah, M. (2004). Effect of maternal diabetes and ethanol interactions on embryo development in the mouse. Mol. Cell. Biochem. 261, 43–56. doi: 10.1023/b:mcbi.0000028736.00532.1e
Paganini, I., Sestini, R., Capone, G. L., Putignano, A. L., Contini, E., Giotti, I., et al. (2017). A novel PAX1 null homozygous mutation in autosomal recessive otofaciocervical syndrome associated with severe combined immunodeficiency. Clin. Genet. 92, 664–668. doi: 10.1111/cge.13085
Pohl, E., Aykut, A., Beleggia, F., Karaca, E., Durmaz, B., Keupp, K., et al. (2013). A hypofunctional PAX1 mutation causes autosomal recessively inherited otofaciocervical syndrome. Hum. Genet. 132, 1311–1320. doi: 10.1007/s00439-013-1337-9
Puech, A., Saint-Jore, B., Merscher, S., Russell, R. G., Cherif, D., Sirotkin, H., et al. (2000). Normal cardiovascular development in mice deficient for 16 genes in 550 kb of the velocardiofacial/DiGeorge syndrome region. Proc. Natl. Acad. Sci. 97, 10090–10095. doi: 10.1073/pnas.97.18.10090
Quinn, J. J., Chang, H. Y. (2016). Unique features of long non-coding RNA biogenesis and function. Nat. Rev. Genet. 17, 47–62. doi: 10.1038/nrg.2015.10
Ramírez-Rosas, E., Velázquez, P. N., Verdugo-Díaz, L., Pérez-Armendáriz, E. M., Juárez-Oropeza, M. A., Paredes-Carbajal, M. C. (2019). Subchronic stress effects on vascular reactivity in C57BL/6 strain mice. Physiol. Behav. 204, 283–289. doi: 10.1016/j.physbeh.2019.03.007
Ravi, H., Mcneill, G., Goel, S., Meltzer, S. D., Hunkapiller, N., Ryan, A., et al. (2018). Validation of a SNP-based non-invasive prenatal test to detect the fetal 22q11.2 deletion in maternal plasma samples. PloS One 13, e0193476. doi: 10.1371/journal.pone.0193476
Reeh, K. A. G., Cardenas, K. T., Bain, V. E., Liu, Z., Laurent, M., Manley, N. R., et al. (2014). Ectopic TBX1 suppresses thymic epithelial cell differentiation and proliferation during thymus organogenesis. Development 141, 2950–2958. doi: 10.1242/dev.111641
Repetto, G. M., Guzmán, M. L., Delgado, I., Loyola, H., Palomares, M., Lay-Son, G., et al. (2014). Case fatality rate and associated factors in patients with 22q11 microdeletion syndrome: a retrospective cohort study. BMJ Open 4. doi: 10.1136/bmjopen-2014-005041
Richarte, A. M., Mead, H. B., Tallquist, M. D. (2007). Cooperation between the PDGF receptors in cardiac neural crest cell migration. Dev. Biol. 306, 785–796. doi: 10.1016/j.ydbio.2007.04.023
Roberts, C., Ivins, S. M., James, C. T., Scambler, P. J. (2005). Retinoic acid down-regulates Tbx1 expression in vivo and in vitro. Dev. Dynamics: Off. Publ. Am. Assoc. Anatomists 232, 928–938. doi: 10.1002/dvdy.20268
Ronchetti, D., Agnelli, L., Pietrelli, A., Todoerti, K., Manzoni, M., Taiana, E., et al. (2018). A compendium of long non-coding RNAs transcriptional fingerprint in multiple myeloma. Sci. Rep. 8, 6557. doi: 10.1038/s41598-018-24701-8
Ryckebusch, L., Bertrand, N., Mesbah, K., Bajolle, F., Niederreither, K., Kelly, R. G., et al. (2010). Decreased levels of embryonic retinoic acid synthesis accelerate recovery from arterial growth delay in a mouse model of DiGeorge syndrome. Circ. Res. 106, 686–694. doi: 10.1161/CIRCRESAHA.109.205732
Sachar, M., Anderson, K. E., Ma, X. (2016). Protoporphyrin IX: the Good, the Bad, and the Ugly. J. Pharmacol. Exp. Ther. 356, 267–275. doi: 10.1124/jpet.115.228130
Sacks, D. A., Hadden, D. R., Maresh, M., Deerochanawong, C., Dyer, A. R., Metzger, B. E., et al. (2012). Frequency of gestational diabetes mellitus at collaborating centers based on IADPSG consensus panel–recommended criteria. Hyperglycemia Adverse Pregnancy Outcome (HAPO) Study 35, 526–528. doi: 10.2337/dc11-1641
Saitta, S. C., Mcgrath, J. M., Mensch, H., Shaikh, T. H., Zackai, E. H., Emanuel, B. S. (1999). A 22q11.2 deletion that excludes UFD1L and CDC45L in a patient with conotruncal and craniofacial defects. Am. J. Hum. Genet. 65, 562–566. doi: 10.1086/302514
Salmena, L., Poliseno, L., Tay, Y., Kats, L., Pandolfi, P. P. (2011). A ceRNA hypothesis: the Rosetta stone of a hidden RNA language? Cell 146, 353–358. doi: 10.1016/j.cell.2011.07.014
Schmid, M., Wang, E., Bogard, P. E., Bevilacqua, E., Hacker, C., Wang, S., et al. (2017). Prenatal screening for 22q11.2 deletion using a targeted microarray-based cell-free DNA test. Fetal Diagnosis Ther. doi: 10.1159/000484317
Schofield, C., Hsu, R., Barker, A., Gertz, C., Blelloch, R., Ullian, E. (2011). Monoallelic deletion of the microRNA biogenesis gene Dgcr8 produces deficits in the development of excitatory synaptic transmission in the prefrontal cortex. Neural Dev. 6, 11. doi: 10.1186/1749-8104-6-11
Sellier, C., Hwang, V. J., Dandekar, R., Durbin-Johnson, B., Charlet-Berguerand, N., Ander, B. P., et al. (2014). Decreased DGCR8 expression and miRNA dysregulation in individuals with 22q11.2 deletion syndrome. PloS One 9, e103884. doi: 10.1371/journal.pone.0103884
Shaikh, T. H., Kurahashi, H., Saitta, S. C., O’hare, A. M., Hu, P., Roe, B. A., et al. (2000). Chromosome 22-specific low copy repeats and the 22q11.2 deletion syndrome: genomic organization and deletion endpoint analysis. Hum. Mol. Genet. 9, 489–501. doi: 10.1093/hmg/9.4.489
Shaikh, T. H., Kurahashi, H., Emanuel, B. S. (2001). Evolutionarily conserved low copy repeats (LCRs) in 22q11 mediate deletions, duplications, translocations, and genomic instability: an update and literature review. Genet. In Med. Off. J. Am. Coll. Med. Genet. 3, 6–13. doi: 10.1097/00125817-200101000-00003
Shaikh, T. H., O’connor, R. J., Pierpont, M. E., Mcgrath, J., Hacker, A. M., Nimmakayalu, M., et al. (2007). Low copy repeats mediate distal chromosome 22q11.2 deletions: sequence analysis predicts breakpoint mechanisms. Genome Res. 17, 482–491. doi: 10.1101/gr.5986507
Simpson, I. A., Dwyer, D., Malide, D., Moley, K. H., Travis, A., Vannucci, S. J. (2008). The facilitative glucose transporter GLUT3: 20 years of distinction. Am. J. Physiol. Endocrinol. Metab. 295, E242–E253. doi: 10.1152/ajpendo.90388.2008
Smith, C. L., Tallquist, M. D. (2010). PDGF function in diverse neural crest cell populations. Cell Adhesion Migration 4, 561–566. doi: 10.4161/cam.4.4.12829
Srinivasan, A., Bianchi, D. W., Huang, H., Sehnert, A. J., Rava, R. P. (2013). Noninvasive detection of fetal subchromosome abnormalities via Deep Sequencing of Maternal Plasma. Am. J. Hum. Genet. 92, 167–176. doi: 10.1016/j.ajhg.2012.12.006
Stalmans, I., Lambrechts, D., Smet, F., Jansen, S., Wang, J., Maity, S., et al. (2003). VEGF: a modifier of the del22q11 (DiGeorge) syndrome? Nat. Med. 9, 173–182. doi: 10.1038/nm819
Stark, K. L., Xu, B., Bagchi, A., Lai, W. S., Liu, H., Hsu, R., et al. (2008). Altered brain microRNA biogenesis contributes to phenotypic deficits in a 22q11-deletion mouse model. Nat. Genet. 40, 751–760. doi: 10.1038/ng.138
Stoller, J. Z., Epstein, J. A. (2005). Identification of a novel nuclear localization signal in Tbx1 that is deleted in DiGeorge syndrome patients harboring the 1223delC mutation. Hum. Mol. Genet. 14, 885–892. doi: 10.1093/hmg/ddi081
Strehle, E.-M., Yu, L., Rosenfeld, J. A., Donkervoort, S., Zhou, Y., Chen, T.-J., et al. (2012). Genotype–phenotype analysis of 4q deletion syndrome: proposal of a critical region. Am. J. Med. Genet. Part A. 158A, 2139–2151. doi: 10.1002/ajmg.a.35502
Sui, J., Li, Y.-H., Zhang, Y.-Q., Li, C.-Y., Shen, X., Yao, W.-Z., et al. (2016). Integrated analysis of long non-coding RNA-associated ceRNA network reveals potential lncRNA biomarkers in human lung adenocarcinoma. Int. J. Oncol. 49, 2023–2036. doi: 10.3892/ijo.2016.3716
Sullivan, K. E. (2004). The clinical, immunological, and molecular spectrum of chromosome 22q11.2 deletion syndrome and DiGeorge syndrome. Curr. Opin. In Allergy Clin. Immunol. 4, 505–512. doi: 10.1097/00130832-200412000-00006
Sullivan, K. E. (2019). Chromosome 22q11.2 deletion syndrome and DiGeorge syndrome. Immunolog. Rev. 287, 186–201. doi: 10.1111/imr.12701
Sun, H., Jiang, P. (2018). MicroRNA-451a acts as tumor suppressor in cutaneous basal cell carcinoma. Mol. Genet. Genomic Med. 6, 1001–1009. doi: 10.1002/mgg3.473
Taddei, I., Morishima, M., Huynh, T., Lindsay, E. A. (2001). Genetic factors are major determinants of phenotypic variability in a mouse model of the DiGeorge/del22q11 syndromes. Proc. Natl. Acad. Sci. U. S. A. 98, 11428–11431. doi: 10.1073/pnas.201127298
Tallquist, M. D., Soriano, P. (2003). Cell autonomous requirement for PDGFRα in populations of cranial and cardiac neural crest cells. Development 130, 507–518. doi: 10.1242/dev.00241
Tan, G., Wu, L., Tan, J., Zhang, B., Tai, W. C.-S., Xiong, S., et al. (2016). MiR-1180 promotes apoptotic resistance to human hepatocellular carcinoma via activation of NF-κB signaling pathway. Sci. Rep. 6, 22328. doi: 10.1038/srep22328
Tham, E., Lindstrand, A., Santani, A., Malmgren, H., Nesbitt, A., Dubbs, H. A., et al. (2015). Dominant mutations in KAT6A cause intellectual disability with recognizable syndromic features. Am. J. Hum. Genet. 96, 507–513. doi: 10.1016/j.ajhg.2015.01.016
Van Der Spek, J., Groenwold, R. H. H., Van Der Burg, M., Van Montfrans, J. M. (2015). TREC based newborn screening for severe combined immunodeficiency disease: a systematic review. J. Clin. Immunol. 35, 416–430. doi: 10.1007/s10875-015-0152-6
Van Esch, H., Groenen, P., Nesbit, M. A., Schuffenhauer, S., Lichtner, P., Vanderlinden, G., et al. (2000). GATA3 haplo-insufficiency causes human HDR syndrome. Nature 406, 419–422. doi: 10.1038/35019088
Vermot, J., Niederreither, K., Garnier, J. M., Chambon, P., Dolle, P. (2003). Decreased embryonic retinoic acid synthesis results in a DiGeorge syndrome phenotype in newborn mice. Proc. Natl. Acad. Sci. U. S. A. 100, 1763–1768. doi: 10.1073/pnas.0437920100
Vitelli, F., Taddei, I., Morishima, M., Meyers, E. N., Lindsay, E. A., Baldini, A. (2002). A genetic link between Tbx1 and fibroblast growth factor signaling. Development 129, 4605–4611.
Voss, A. K., Vanyai, H. K., Collin, C., Dixon, M. P., Mclennan, T. J., Sheikh, B. N., et al. (2012). MOZ regulates the Tbx1 Locus, and Moz mutation partially phenocopies DiGeorge syndrome. Dev. Cell 23, 652–663. doi: 10.1016/j.devcel.2012.07.010
Wapner, R. J., Martin, C. L., Levy, B., Ballif, B. C., Eng, C. M., Zachary, J. M., et al. (2012). Chromosomal microarray versus karyotyping for prenatal diagnosis. New Engl. J. Med. 367, 2175–2184. doi: 10.1056/NEJMoa1203382
Xiao, C., Rajewsky, K. (2009). MicroRNA control in the immune system: basic principles. Cell 136, 26–36. doi: 10.1016/j.cell.2008.12.027
Xu, H., Morishima, M., Wylie, J. N., Schwartz, R. J., Bruneau, B. G., Lindsay, E. A., et al. (2004). Tbx1 has a dual role in the morphogenesis of the cardiac outflow tract. Development 131, 3217–3227. doi: 10.1242/dev.01174
Xu, B., Hsu, P.-K., Stark, K., Karayiorgou, M., Gogos, J. (2013). Derepression of a neuronal inhibitor due to miRNA dysregulation in a schizophrenia-related microdeletion. Cell 152, 262–275. doi: 10.1016/j.cell.2012.11.052
Xu, Y., Zhang, G., Zou, C., Gong, Z., Wang, S., Liu, J., et al. (2019). Long noncoding RNA DGCR5 suppresses gastric cancer progression by acting as a competing endogenous RNA of PTEN and BTG1. J. Cell. Physiol. 234, 11999–12010. doi: 10.1002/jcp.27861
Yagi, H., Furutani, Y., Hamada, H., Sasaki, T., Asakawa, S., Minoshima, S., et al. (2003). Role of TBX1 in human del22q11.2 syndrome. Lancet 362, 1366–1373. doi: 10.1016/s0140-6736(03)14632-6
Yang, L., Froberg, J. E., Lee, J. T. (2014). Long noncoding RNAs: fresh perspectives into the RNA world. Trends Biochem. Sci. 39, 35–43. doi: 10.1016/j.tibs.2013.10.002
Yang, X., Li, Y., Li, L., Liu, J., Wu, M., Ye, M. (2017). SnoRNAs are involved in the progression of ulcerative colitis and colorectal cancer. Digestive Liver Dis. 49, 545–551. doi: 10.1016/j.dld.2016.12.029
Yang, L., Du, X., Liu, L., Cao, Q., Pan, Z., Li, Q. (2019). miR-1306 mediates the feedback regulation of the TGF-β/SMAD signaling pathway in Granulosa cells. Cells 8, 298. doi: 10.3390/cells8040298
Yatsenko, S. A., Peters, D., Saller, D., Chu, T., Clemens, M., Rajkovic, A. (2015). Maternal cell free DNA based screening for fetal microdeletion and the importance of careful diagnostic follow up. Genet. Med. Off. J. Am. Coll. Med. Genet. 17, 836–838. doi: 10.1038/gim.2014.197
Yi, R., Pasolli, H. A., Landthaler, M., Hafner, M., Ojo, T., Sheridan, R., et al. (2009). DGCR8-dependent microRNA biogenesis is essential for skin development. Proc. Natl. Acad. Sci. U. S. A. 106, 498–502. doi: 10.1073/pnas.0810766105
Zemble, R., Luning Prak, E., Mcdonald, K., Mcdonald-Mcginn, D., Zackai, E., Sullivan, K. (2010). Secondary immunologic consequences in chromosome 22q11.2 deletion syndrome (DiGeorge syndrome/velocardiofacial syndrome). Clin. Immunol. 136, 409–418. doi: 10.1016/j.clim.2010.04.011
Zhang, Z., Baldini, A. (2008). In vivo response to high-resolution variation of Tbx1 mRNA dosage. Hum. Mol. Genet. 17, 150–157. doi: 10.1093/hmg/ddm291
Zhang, J., Fan, D., Jian, Z., Chen, G. G., Lai, P. B. S. (2015). Cancer specific long noncoding RNAs show differential expression patterns and competing endogenous RNA potential in Hepatocellular carcinoma. PloS One 10, e0141042. doi: 10.1371/journal.pone.0141042
Zhang, Y., Dai, J., Tang, J., Zhou, L., Zhou, M. (2017). MicroRNA-649 promotes HSV-1 replication by directly targeting MALT1. J. Med. Virol. 89, 1069–1079. doi: 10.1002/jmv.24728
Zhou, J., Yang, L., Zhong, T., Mueller, M., Men, Y., Zhang, N., et al. (2015). H19 lncRNA alters DNA methylation genome wide by regulating S-adenosylhomocysteine hydrolase. Nat. Commun. 6, 10221. doi: 10.1038/ncomms10221
Zinkstok, J. R., Boot, E., Bassett, A. S., Hiroi, N., Butcher, N. J., Vingerhoets, C., et al. (2019). Neurobiological perspective of 22q11.2 deletion syndrome. Lancet Psychiatry. doi: 10.1016/S2215-0366(19)30076-8
Keywords: 22q11.2 deletion syndrome, DiGeorge syndrome, TBX1, noncoding RNAs, microRNAs, epigenetics, haploinsufficiency
Citation: Du Q, de la Morena MT and van Oers NSC (2020) The Genetics and Epigenetics of 22q11.2 Deletion Syndrome. Front. Genet. 10:1365. doi: 10.3389/fgene.2019.01365
Received: 22 July 2019; Accepted: 12 December 2019;
Published: 06 February 2020.
Edited by:
Dan Koboldt, Nationwide Children’s Hospital, United StatesReviewed by:
Marita Bosticardo, National Institute of Allergy and Infectious Diseases, United StatesThomas M Maynard, George Washington University, United States
Copyright © 2020 Du, de la Morena and van Oers. This is an open-access article distributed under the terms of the Creative Commons Attribution License (CC BY). The use, distribution or reproduction in other forums is permitted, provided the original author(s) and the copyright owner(s) are credited and that the original publication in this journal is cited, in accordance with accepted academic practice. No use, distribution or reproduction is permitted which does not comply with these terms.
*Correspondence: Nicolai S. C. van Oers, Tmljb2xhaS52YW5vZXJzQHV0c291dGh3ZXN0ZXJuLmVkdQ==
†Present address: Qiumei Du, Harbor Biomed MA, United States