- 1School of Science and Health, Western Sydney University, Penrith, NSW, Australia
- 2College of Agriculture and Biotechnology, Zhejiang University, Hangzhou, China
- 3Hawkesbury Institute for the Environment, Western Sydney University, Penrith, NSW, Australia
Light is a vital regulator that controls physiological and cellular responses to regulate plant growth, development, yield, and quality. Light is the driving force for electron and ion transport in the thylakoid membrane and other membranes of plant cells. In different plant species and cell types, light activates photoreceptors, thereby modulating plasma membrane transport. Plants maximize their growth and photosynthesis by facilitating the coordinated regulation of ion channels, pumps, and co-transporters across membranes to fine-tune nutrient uptake. The signal-transducing functions associated with membrane transporters, pumps, and channels impart a complex array of mechanisms to regulate plant responses to light. The identification of light responsive membrane transport components and understanding of their potential interaction with photoreceptors will elucidate how light-activated signaling pathways optimize plant growth, production, and nutrition to the prevailing environmental changes. This review summarizes the mechanisms underlying the physiological and molecular regulations of light-induced membrane transport and their potential interaction with photoreceptors in a plant evolutionary and nutrition context. It will shed new light on plant ecological conservation as well as agricultural production and crop quality, bringing potential nutrition and health benefits to humans and animals.
Introduction
Light is one of the most important environmental cues for plant growth and development and other physiological responses via the changes of intensity and spectral quality of light (Steinger et al., 2003; Fan et al., 2013). In natural environments, plants regularly experience rapid changes in the intensity of solar radiation during the day and across seasonal changes. Plant leaves have evolved morphological adaptations and physiological acclimation processes to survive light levels ranging from nearly 0 (i.e., darkness) up to 2,000 µmol m−2 s−1 photons during the peak of midday irradiances. High irradiances generally reduce specific leaf area, increase leaf thickness, alter palisade cell development, and change the position and composition of chloroplasts (Karpinski et al., 1999; Givnish et al., 2004; Walters, 2005; Matos et al., 2009). Light is used as photosynthetic energy source as well as a vital regulator that can affect plant photomorphogenesis through the perception of the spectrum regulated by photoreceptors and potentially the downstream signaling component-membrane transporters. Membrane transport plays a central role virtually in all the aspects of plant ion and solute homeostasis and signaling transduction (Wang et al., 2018). Specialized plant membrane transporters are a promising target to increase crop yields, enhance produce quality, and improve resistance to abiotic and biotic stresses for the sustainable production of nutritious foods (Schroeder et al., 2013). However, the underlying light sensory mechanisms of plants are complex. In this review, we summarize how light regulates plant functions at the molecular, cellular, tissue, and whole-plant levels. We highlight how photoreceptors may have profound regulation on membrane transport in plant cells. We emphasize how coordinated light responses drive ion exchange, membrane transporters, and photoreceptors to promote leaf and fruit developments for improving crop nutrition.
Molecular Physiology of Light Response in Plants and Fruits
Light is used in the photosynthetic machinery, as well as in various regulatory processes such as seed germination, flowering, stomatal development, and membrane transport of guard cells (Assmann et al., 1985; Talbott and Zeiger, 1993; Walters et al., 2003; Fan et al., 2004; Lee et al., 2007b). The responses of plants to light at the whole plant, organ, cell, and molecular levels are evolutionarily conserved (Mullineaux and Karpinski, 2002; Christie, 2007; Shimazaki et al., 2007). Light is heterogeneously distributed throughout the canopy in a horizontal and the vertical planes, but a more homogenous light distribution in the canopy can be advantageous (Li et al., 2014). Moreover, excess light is a stress that can damage DNA and other cellular components, leading to the detrimental effect on photosynthesis (Takahashi and Badger, 2011; Jenkins, 2017; Yin and Ulm, 2017). Plants have mechanisms to protect against excess light that triggers photo-oxidative and harmful damage by minimizing the exposure of photosynthetic tissue to excessive radiation (Martín et al., 2016) and therefore acclimate to the different light wavelengths, showing a high degree of morphological and physiological plasticity (O’carrigan et al., 2014). For example, plants have adapted to excessive light through curling their leaves (Neuner et al., 1999), enhancing their cuticular wax, and altering leaf as well as whole-plant morphology (Horton et al., 1996). Plants can also reduce their specific leaf area (SLA) when photosynthetic photon flux density is increased from 50 to 500 µmol m−2 s−1 (Fan et al., 2013). These alterations in leaf morphology can essentially regulate plant growth, photosynthesis, fruit development, yield, and quality (Azari et al., 2010).
Light has a very important role in determining the nutrient level of plant-based foods, thus affecting human nutrition and health (Davis and Uthus, 2004; Evans et al., 2006). For instance, light treatments can significantly regulate the accumulation of micronutrients and pigments in tomato (O’carrigan et al., 2014). Plant pigments (chlorophyll and carotenoids) are light-sensitive and intrinsic to absorption within photosystems I and II (PSI and PSII) (Gross, 2012). The illumination with high blue light increases the synthesis and accumulation of anthocyanins and carotenoids in pepper plants (Capsicum annuum L.) without affecting the flavonoid contents (Hoffmann et al., 2016). Fruit dry matter and soluble sugar content and the production of lycopene are improved by increasing solar irradiances which attribute to improved tomato fruit quality (Davies et al., 1981), but the excessive light can inhibit the lycopene content (Brandt et al., 2006). Improved amounts of ascorbate, lycopene, carotene, rutin, caffeic acid, and soluble phenol derivatives in fruit have been reported under increased light irradiance (Wilkens et al., 1996; Gautier et al., 2008). Moreover, the accumulation of anthocyanin and soluble sugar in strawberry fruit was promoted with the increases in the expression of aroma-related genes in light accompanied by the regulation of FvMYB10 at transcriptional and post-translation levels (Xu et al., 2018). Naturally occurring plant derivative antioxidants, such as phenols, vitamins, carotenoids, and terpenoids, are likely to be beneficial significantly for health promotion with the reduction of inflammation associated with chronic diseases (Goggs et al., 2005). Plant extracts and their purified compounds are selectively turning off inflammatory cyclooxygenase-2 (COX-2), while preserving housekeeping gene cyclooxygenase-1 (COX-1) (Evans et al., 2006). Thus, nutrient-rich foods are likely to be regulated by light through plant photoreceptors and membrane transport of nutrient to the eatable parts of the plants, which are very multifaceted processes worth investigating in the future.
Plant response to light is modulated by large number of light-regulated genes, which encode proteins including photoreceptors, early signaling components, pleiotropic constitutive photomorphogenic/de-etiolated/fusca (COP/DET/FUS), and many downstream effectors (Quail, 2002; Jiao et al., 2007; Azari et al., 2010). Abrupt changes in gene expression due to excess light have been reported in plants (Rossel et al., 2002; Kimura et al., 2003; Murchie et al., 2005; Adamiec et al., 2008) and green algae (Im et al., 2003; Fischer et al., 2006). Gene expression is severely damaged when light intensities are greater than the maximum potential of the chloroplast electron capacity (Demarsy et al., 2018; Foyer, 2018). Light can enhance the repositioning of the Arabidopsis light-inducible chlorophyll a/b-binding (CAB) locus quickly from the nuclear interior to the nuclear periphery through the red/far-red phytochromes (PHYs) during its transcriptional activation (Feng et al., 2014). Likewise, the light-inducible ribulose-1,5-bisphosphate carboxylase/oxygenase small subunit (RBCS), plastocyanin (PC), and genomes uncoupled 5 (GUN5) showed similar repositioning behavior upon their activation (Feng et al., 2014). Light-intensity-dependent changes of light-harvesting chlorophyll protein complex associated with photosystem II (LHCIIs) are regulated by CAB gene transcription (Escoubas et al., 1995). Moreover, regulation of microRNAs in light stress responses and adaptive mechanisms in plants have been emphasized and may be a potential target for light stress tolerance in crop plants (Yang et al., 2019). Integrative omics strategies, including both proteome and transcriptome, showed that different irradiances coordinate light harvesting, electron transport, and protein synthesis to cope with ever-changing environmental conditions by adjusting the thylakoid membrane proteome in pea (Albanese et al., 2018).
Photoreceptors especially phototropins (PHOTs) can modulate the expression of a large number of photosynthesis-related genes, such as light-harvesting chlorophyll a/b-binding 1 (LHCB1), ribulose-1,5-bisphosphate carboxylase/oxygenase small subunit (RBCS), photosystem II manganese-stabilizing protein (PSBO), photosystem I reaction center subunit IV (PSAE), and photosynthetic electron transfer C (PETC) (López-Juez et al., 2007). Plants grown at high light show up-regulation of RBCS and down-regulation of LHCB1 in Arabidopsis phot1-1 (nph1-1) and phot2-5 (npl1-1 or cav1-5) double mutants (López-Juez et al., 2007). In Arabidopsis, a subset of genes including early light-inducible protein1 (ELIP1) and ELIP2, which encode light stress-related relatives of the LHC protein family and production of anthocyanin pigments 1 and 2 (PAP1) and PAP2, seems to be regulated in excess light or high-intensity blue light by cry1 (cryptochrome 1) mutant (Kleine et al., 2007). In addition, excess light-dependent regulation of 77 genes is changed in the cry1 mutant, and 26 of these genes are also mis-regulated in an elongated hypocotyl5 (hy5) transcription factor mutant (Kleine et al., 2007). The zinc-finger transcription factor ZAT12/RHL41 can be rapidly induced in Arabidopsis to adapt in high light environment (Iida et al., 2000). Notably, blue light modulates the accumulation of anthocyanin in Arabidopsis seedlings, which is CRY-dependent (El-Esawi et al., 2017), and the overexpression of CRY1a increased the accumulation of anthocyanin in tomato (Liu et al., 2018).
In Arabidopsis about 10% (∼2,500) of the genes are modulated by PHYs under long-term light exposure (Tepperman et al., 2006). The PHY-regulated transcription of photoresponsive genes leads to photomorphogenesis via negative transcriptional factors such as PHY-interacting factors (PIFs) and positive transcriptional factors such as HY5 (elongated hypocotyl 5) and HYL (HY5-like) (Lee et al., 2007a; Leivar and Monte, 2014). Nonetheless, recent advances in light-induced modulation of photoreceptors have provided ample knowledge of their molecular interaction with various photosynthesis-related proteins in light signal transduction pathway.
Little is known about the direct link between photoreceptors and membrane transport along the signaling cascade, but circadian clocks appear to be involved in linking photoreceptors and solute transport (Haydon et al., 2011). Light input to the circadian clock and oscillator is mediated by the cryptochromes (CRYs) and PHYs (Somers et al., 1998; Devlin and Kay, 2000). It was reported that blue light and CRY-dependent circadian regulation of the sigma transcription factor (SIG5) form a part of the chloroplast signaling pathway in light stress adaptation (Belbin et al., 2017). In the transcription translation loops, light increases the promoter activity of circadian clock-associated 1 (CCA1) and late elongated hypocotyl (LHY), while blue light directly activates Zeitlupe (ZTL) to bind GIGANTEA (GI). Interaction with GI stabilizes ZTL and ZTL and GI disassociates allows ZTL to target timing of CAB expression 1 (TOC1) for degradation at dusk (Dodd et al., 2007; Kim et al., 2007; Haydon et al., 2011). Therefore, light activates photoreceptors, and circadian regulation of transcripts may further regulate membrane transporters mediating fluxes of ions, sugars, and metabolites for plant nutrition.
Ion Fluxes in Response to Light
Understanding the physiological implications of light-induced electrical signaling at cellular and tissue levels is essential to elucidate the ionic balance and mineral nutrients at whole-plant level. Light can trigger a cascade of electrical events in thylakoid and plasma membranes of green plant tissues (Vredenberg and Tonk, 1975; Hansen et al., 1987; Elzenga et al., 1995; Johannes et al., 1997). At the plasma membrane, light induces voltage changes in Arabidopsis and ion fluxes of tobacco mesophyll protoplasts (Spalding et al., 1992; Blom-Zandstra et al., 1997). The kinetics of H+, Ca2+, K+, and Cl− fluxes of the mesophyll and epidermis of broad bean is related to light-induced changes in the plasma membrane potential (Em) (Shabala and Newman, 1999). Light-activated depolarization in the mesophyll and epidermis is very different (Elzenga et al., 1995; Shabala and Newman, 1999). PHYs can alter the permeability of the plasma membrane to ions from the subsequent changes in Em in response to light (Newman and Briggs, 1972; Racusen and Galston, 1983). However, the mechanisms underlying the light-induced transient Em changes and their ionic basis remain elusive due to the limited number of studies.
Fruit quality that contributes to nutritional attributes can be modulated through light-induced ion fluxes with the coordinated interaction of membrane transporters. Light spectral composition is important factors in determining grape juice acidity and K+ concentrations (Shabala and Wilson, 2001), and fruit pH and K+ levels are modulated by leaf, instead of fruit, exposure to light (Morrison and Noble, 1990). Fluctuations in light intensity can considerably regulate net ion fluxes from and into the berry mesocarp, including changes in fruit apoplastic pH and K+ concentrations, which also play important role in chlorophyll-mediated light transduction to modulate light-dependent ionic exchange (Shabala and Wilson, 2001). It is proposed that K+ enters the cells in exchange for protons derived from organic acids, driven by light-induced membrane-bound H+-ATPase activity (Boulton, 1980a), which results in juice with lower acidity (Boulton, 1980b). However, further studies are required to identify and characterize membrane transporters in fruit tissue that are very different from leaf and root tissues of plants.
Proton Pumps and Light Response in Plants
In plants, proton gradients produced by primary H+-translocating pumps that hydrolyze either ATP [plasma membrane P-type H+ translocating ATPase (H+-ATPase) and tonoplast V-type H+ translocating ATPase (V-ATPase)] or PPi [tonoplast H+ translocating pyrophosphatase (V-PPase)] as the energy source to extrude protons, generating a proton motive force (PMF) to energize the membrane transport (Drozdowicz and Rea, 2001; Palmgren, 2001; Sze et al., 2002; Cheng et al., 2003). Numerous studies have shown an increase in the activity of the H+ pumping out of the cytosol to the apoplast in plants after the onset of illumination. Large light-induced H+ extrusion promotes leaf expansion by increasing cell growth and wall extensibility and regulates photosynthesis and ATP synthesis (Gay and Hurd, 1975; Walters et al., 2003; Christie, 2007; Kangasjärvi et al., 2012). Briefly, the light-induced electrogenic reactions take place in photosynthetic reaction centers of photosystems I and II (PSI and PSII). The light-induced electron transfer in PSII releases H+ into the thylakoid lumen and reduces plastoquinone molecule (PQ), which takes H+ from the outside and delivers them as PQH2 to the inner side of the membrane. Light-driven electrons activate H+ pumping from the stroma into the thylakoid lumen against the electrochemical potential which is driven by PMF across membranes. Protons translocate ATPase for ATP synthesis in response to the pH gradient is then established across the thylakoid membrane (Junge and Jackson, 1982; Muñiz et al., 1995) (Figure 1). It was reported that blue light–stimulated stomatal opening in the leaf is correlated with activation of an electrogenic pump in the guard cell which results in hyperpolarization of 45 mV and outward H+ currents of 5.5 pA, creating an electrochemical gradient for passive ion fluxes (Assmann et al., 1985). Red light also stimulates an outward current mediated by an electrogenic proton pump of Vicia guard cells via the modulation of chloroplasts (Serrano et al., 1988). However, whether there are any interactions between the blue and red light–induced proton pumping and photoreceptors are still not fully explored.
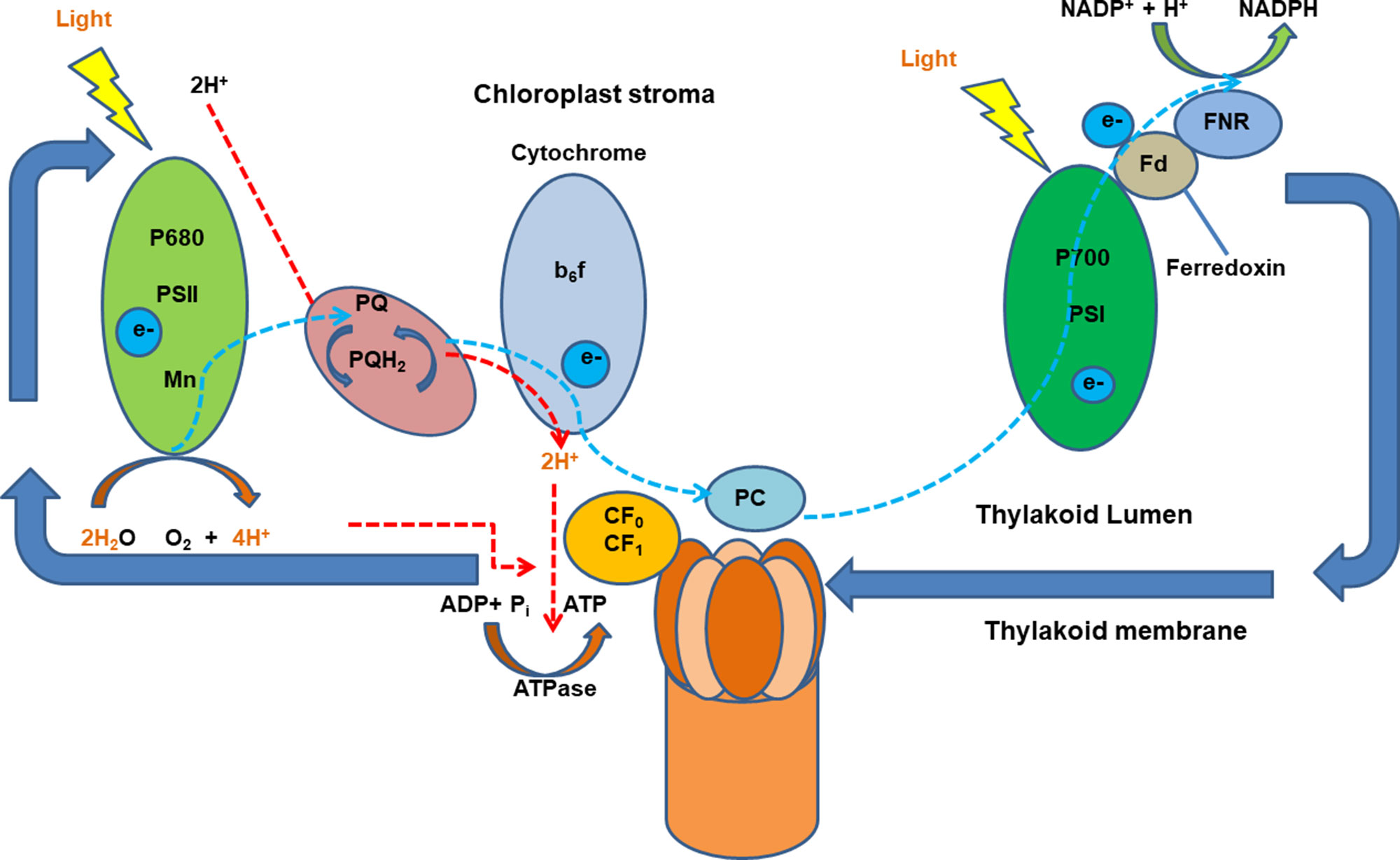
Figure 1 A schematic diagram of light-induced generation of proton motive force in chloroplasts. Light-generated electrons, transferred upon illumination, activate H+ pumping into the thylakoid lumen the electrochemical potential gradient which is driven by proton motive force (PMF) across membranes. Ion channels and transporters can then be regulated by PMF. Adapted and modified from (Muñiz et al., 1995). ADP, adenosine diphosphate; ATP, adenosine triphosphate; ATPase, ATP synthase; b6f, cytochrome b6f complex (plastoquinol–plastocyanin reductase); FNR, feradoxin NADPH reductase; Fd, ferredoxin; NADP/NADPH, nicotinamide adenine dinucleotide phosphate; P700, photosystem I primary donor; PSI, photosystem I; PSII, photosystem II.
Ion Channels and Light Response in Plants
The movement of the ionic substrates is facilitated across the lipid bilayer by the ion channels (Spalding, 2000). Ion channels take part in sensory perception, ion homeostasis, secretion, and Em regulation in all plant cells and play a vital role in the physiology of cells and whole organisms (Assmann et al., 1985; Serrano et al., 1988; Chen et al., 2012b; Hills et al., 2012). An early response of plant cells to blue and red light also results in the hyperpolarization of Em and high proton pump activities (Assmann et al., 1985; Serrano et al., 1988), which affect ion channels. Here, we summarize ion channels that are important for light response in plant cells.
Potassium channels are an essential element controlling plant growth and development and affecting the homeostasis of other elements. In Arabidopsis, plasma membrane inward K+ channels (e.g., KAT1, KAT2, AKT1, AKT2) and outward K+ channels (e.g., GORK, SKOR) mediate K+ fluxes (Blom-Zandstra et al., 1997; Pilot et al., 2003; Zhao et al., 2018a). White light induces an increase in plasma membrane K+-channel activity and a 30- to 70-mV transient membrane depolarization (completed in 2–3 min) in Arabidopsis leaf mesophyll cells (Spalding et al., 1992). Arabidopsis two-pore K+ channels (TPKs) are localized to the tonoplast (TPK1, TPK2, TPK3, and TPK5) and plasma membrane (TPK4) (Voelker et al., 2006; Pfeil et al., 2014). Arabidopsis tpk3 mutant has reduced generation of PMF, which results in altered thylakoid membrane organization, less CO2 assimilation, and reduced dissipation of excess light. Therefore, TPK3 modulates the composition of the PMF, necessary to convert photochemical energy into physiological functions, through ion counterbalancing (Carraretto et al., 2013). Moreover, the links between blue light receptor CRYs and their electrical responses in facilitating K+ channels involved in the blue light perception mechanism are reported (Suh et al., 2000), and red light–induced Em transients in the moss Physcomitrella patens promote K+-channel interaction with PHY signaling (Johannes et al., 1997).
Calcium channels play key role in maintaining cellular response to different environmental stimuli perception. Depolarization-activated Ca2+ channels and hyperpolarization-activated calcium channels have been described and characterized in plants (Véry and Davies, 2000; Miedema et al., 2001), providing a pathway for Ca2+ influx into plant cells (Fairley-Grenot and Assmann, 1992; Marshall et al., 1994; White, 1994; Gelli and Blumwald, 1997). Uptake of Ca2+ in plant cells is accomplished through the depolarization and hyperpolarization-activated calcium channels orchestrated with non-selective cation channel, as well as Ca2+-ATPases and cotransporters acting in concert (Miedema et al., 2001). Early cellular responses to blue and red lights are attributed to the activation of Ca2+ permeable channels to mediate the influx of Ca2+ into cells such as guard cells (Hamilton et al., 2000) and root hair cells (Véry and Davies, 2000). However, full molecular characterization of these Ca2+ channels has yet to be explored in plants. Therefore, the regulations of light and photoreceptors on these Ca2+ channels are most likely to be indirect via the light regulation of PM H+-ATPase and potentially Ca2+-ATPase and ion homeostasis. Their links with light and photoreceptors are a challenge worthy of further discovery.
Plants have at least three families of genes encoding anion channels: slow anion channels (SLACs/SLAHs), rapid anion channels (ALMTs/QUACs), and chloride channels (CLCs) (Spalding, 2000; Roberts, 2006). Both SLACs and ALMTs can be activated by cytosolic Ca2+ and are permeable to a range of anions, including Cl−, malate2−, and NO3− (Chen et al., 2007; Marten et al., 2007). Blue light not red light activates anion channels residing at the plasma membrane of hypocotyl cells of etiolated Arabidopsis seedlings. Anion channel–blocker 15-nitro-2-(3-phenylpropylamino)-benzoic acid (NPPB) inhibits the anion channels and reduces the blue-light-induced depolarization (Cho and Spalding, 1996). Electron transferred to receiver molecule from an excited CRY1 flavoprotein in redox reactions indicate that anion channels may be directly modulated by blue light (Cashmore et al., 1999). The early and important signal transduction role of the anion channels in the blue-light responses of seedling stems are also supported by the findings in Arabidopsis (Noh and Spalding, 1998), pea (Elzenga et al., 1995), and bean (Shabala and Newman, 1999). Illumination triggers a Ca2+-dependent anion channel in the plasma membrane of emerging pea leaf mesophyll cells (Elzenga and Van Volkenburgh, 1997; Lewis et al., 1997). Moreover, an Arabidopsis thylakoid membrane-localized voltage-dependent Cl− channel (VCCN1) fine-tunes PMF via anion influx into the lumen in response to sudden changes to high light, implicating its involvement in photo-protective mechanisms (Herdean et al., 2016). In addition, the independent roles of ion transporters such as Cl− channel e (CLCe), voltage-dependent Cl− channel (VCCN1), and the K+/H+ antiporter (KEA3) have been unraveled in Arabidopsis to fine-tune photosynthesis in the fluctuating light environments (Dukic et al., 2019). However, it is not clear whether any other light receptors are involved in this process.
Co-Transporters and Light Response in Plants
For co-transporters, plants mostly use protons as the coupling ion to transport cations and anions across biological membranes driven by H+ gradient created by H+-ATPase, V-ATPase, and V-PPase (Sze et al., 1999; Gaxiola et al., 2002). For instance, Arabidopsis Ca2+/H+ exchanger 1 (CAX1) mutant cax1 reduces tonoplast Ca2+/H+ antiporter activity about 50%, tonoplast V-ATPase activity 40%, and tonoplast Ca2+-ATPase activity 36% (Cheng et al., 2003). Plants perceive light absorbing far-red (FR) and red light (R) via the PHYA-E. The disrupted gene in long after FR (laf6) mutant encodes ATP-binding-cassette 1 (AtABC1) co-transporter that contains an N-terminal transit peptide targeting to chloroplasts. Mutation in AtABC1 results in an accumulation of protoporphyrin IX and in attenuation of FR-regulated gene expression, which may act as a light-specific signal coordinating communication between plastids and the nucleus (Møller et al., 2001).
Membrane-localized sugar transporters (SUTs, STPs, or SUCs) facilitate the transport, storage, and utilization of sugars for plant growth and development (Gahrtz et al., 1994; Riesmeier et al., 1994; Srivastava et al., 2008). Expression of the AtSTP1 in guard cells showed a strong increase of AtSTP1 expression in the dark and a transient, diurnally regulated increase during the photoperiod around midday (Stadler et al., 2003; Weise et al., 2008). In Solanum tuberosum, StSUT1, StSUT2, and StSUT4 are co-localized, and their RNA levels not only follow a diurnal rhythm but also oscillate in constant light. The phenotype of StSUT4-RNAi plants includes early flowering, higher tuber production, and reduced sensitivity toward light enriched in far-red wavelength, indicating an indirect interaction of PHYs (or potential CRYs, PHOTs) and StSUS4 via the circadian clock genes (Chincinska et al., 2008).
Moreover, co-transporters across the chloroplast thylakoid membrane also play an essential role in light regulated cellular response and plant growth. Arabidopsis K+ efflux antiporter (KEA3) is critical for high photosynthetic efficiency on a shift from dark to low light (or high to low light) and kea3 mutants show prolonged dissipation of absorbed light energy as heat (Armbruster et al., 2014; Kunz et al., 2014). Besides, Arabidopsis K+ efflux antiporters (KEAs) localized to the Golgi apparatus play a pivotal role in the maintenance of ionic and pH homeostasis and in coping with high K+/Na+ stress during quick etiolated growth of seedlings (Wang et al., 2019).
The thylakoid ATP/ADP carrier (TAAC) supply ATP for the nucleotide-dependent reactions in the thylakoid lumen, which facilitate the repair of PSII when plants are under light stress (Yin et al., 2010; Spetea and Lundin, 2012). To develop a comprehensive light signaling network, future investigations are required to discover new transporters responding and/or involved in light-mediated signaling and their interactions with photoreceptors.
Light Response of Photoreceptors
Light signals are sensed and utilized by a few major families of photoreceptors to initiate plant physiological responses through signal networks in integration with other environmental cues (Franklin et al., 2004). In plants, most photoreceptor families (Figure 2) consist of more than one member and share a high degree of resemblance among the different members (Kharshiing and Sinha, 2015). Furthermore, photoreceptors consist of photoreceptive domains binding chromophores to absorb light signals (Figure 2). Thus, the physical light signal is converted to a biochemical signal such as protein–protein interactions and enzyme activation by the photoreceptors to propagate downstream signal transduction (Kong and Okajima, 2016). Photoreceptors play a vital role in plants, regulating most of the spectral responses coupled with altering the expression of up to 1/3 of genes and proteins (Ma et al., 2001; Tepperman et al., 2001; Folta et al., 2003; Tepperman et al., 2004; Wang and Folta, 2013). Light induces extensive reprogramming of gene expression patterns in plants with the association of photoreceptors (Mawphlang and Kharshiing, 2017).
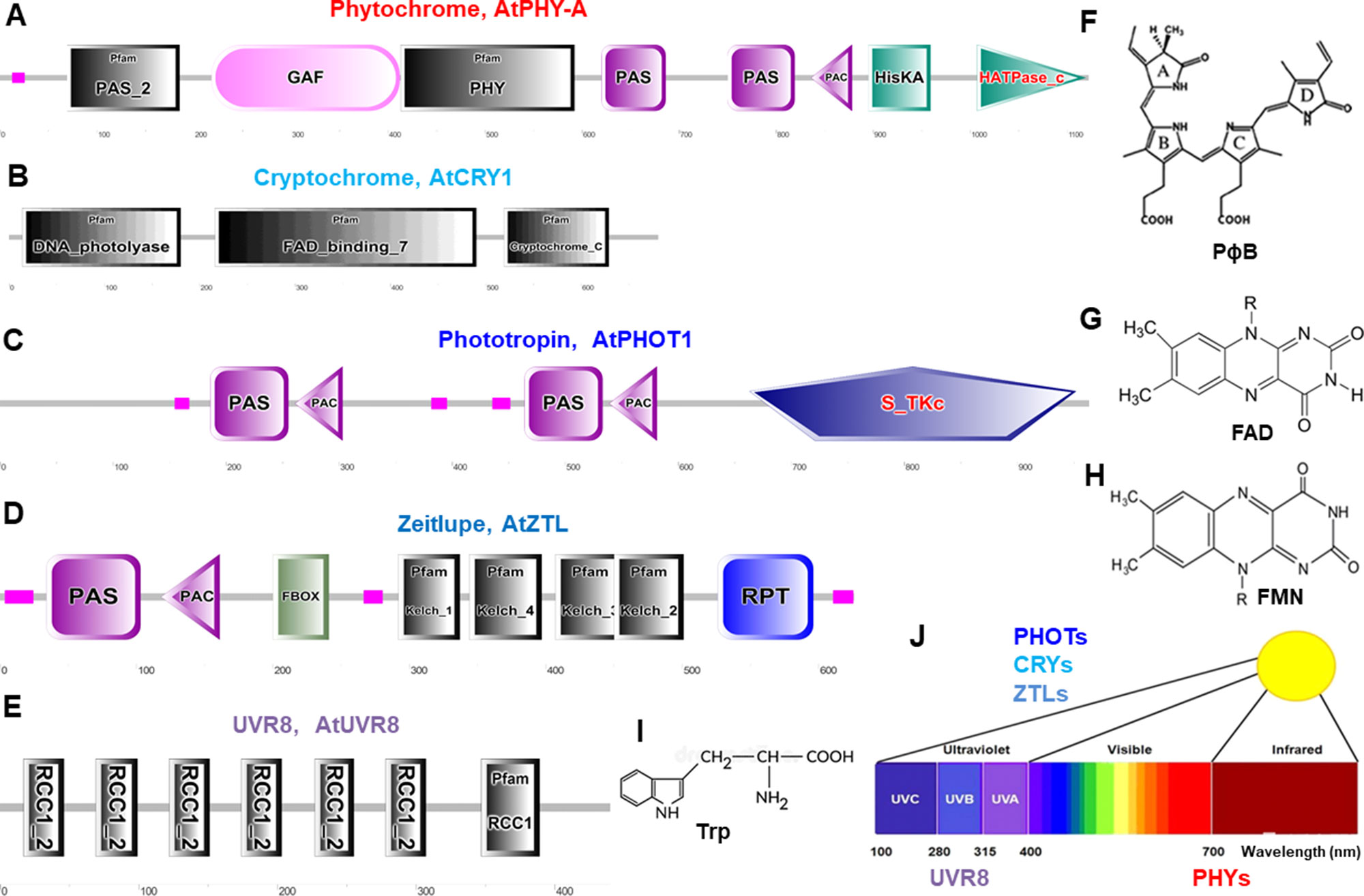
Figure 2 Schematic diagram of domain organization and structure of typical photoreceptors in Arabidopsis. These protein domains are for phytochromes (A), cryptochromes (B), phototropins (C), Zeitlupes (D), and UVR8 (E). Molecular structures are phytochromobilin (PɸB, F), flavin adenine dinucleotide (FAD, G), flavin mononucleotide (FMN, H), and tryptophan (Trp, I). (J), Light spectrum and the photoreceptors. Protein domain mages are generated from http://smart.embl–heidelberg.de/ and molecular structures are from (Galvão and Fankhauser, 2015). PAS, Per-Arndt-Sim; GAF, cGMP phosphodiesterase/adenyl cyclase/Fh1A; PAC, photoactivatable adenylyl cyclase; HisKA, histidine phosphorylation site in the histidine kinase domain; S_TKc, serine/threonine protein kinases protein kinases; F-box, F-box like domain; Kelch, kelch motif; RPT, root phototropism; FAD, flavin-adenine-di-nucleotide, UVR8, UV resistance locus 8; RCC1, regulator of chromosome condensation repeat1.
Phytochromes absorb wavelengths of red (600–700 nm) and far-red light (700–750 nm) and can optimize plant growth and development in changing light (Li et al., 2011; Xu et al., 2015; Hoang et al., 2019) and regulate germination, de-etiolation, shade avoidance responses, and many other plant physiological responses (Kong and Okajima, 2016). PHYs can be homodimeric or heterodimeric proteins comprising of a N-terminal photosensory region covalently bound to a phytochromobilin tetrapyrrole chromophore (PɸB), and a C-terminal output region involved in dimerization (Figures 2A, F) (Burgie and Vierstra, 2014). PHYs are encoded by the PHYA-E gene family in Arabidopsis and other plant species (Sharrock and Quail, 1989; Alba et al., 2000; Staiger, 2008) and are localized in the cytosol in dark to be imported into the nucleus upon activation by light (Nagatani, 2004). Most PHY responses are likely to be the interaction of activated PHYs with transcription factors within the nucleus and triggering responses in the cytosol (Kevei et al., 2007; Rösler et al., 2007; Fankhauser and Chen, 2008).
CRYs absorb wavelengths of blue light/ultraviolet (UV)-A (315–500 nm) and become increasingly evident as key regulators for plant stress responses under environmental fluctuation (Mishra and Khurana, 2017; Carvalho and Damião, 2018), such as inhibition of hypocotyl elongation, photoperiodic control of floral initiation, and circadian rhythms (Christie et al., 2014). CRYs relay light input sensed by a flavin adenine dinucleotide (FAD) chromophore to regulate different biological processes (Figures 2B, G) (Chaves et al., 2011). In Arabidopsis, UV-A and blue light–activated CRY1, CRY2, (Ahmad and Cashmore, 1993; Lin, 1996) CRY3, (Kleine et al., 2003; Staiger, 2008), and CRY-DASH (Drosophila, Arabidopsis, Synechocystis, and human) (Eder and Cosio, 1994) are well characterized. CRYs show different expression pattern and protein stability (Ahmad et al., 1998), and interaction of CRY1 with the E3-ubiquitin ligase constitutive photomorphogenic1 (COP1) is a major event to trigger UV-A and blue light–dependent changes in gene expression (Jiao et al., 2007).
Phototropins, plasma membrane-associated kinases, are activated by blue light/UV-A (315–500 nm) (Christie, 2007; Hart et al., 2019) and play important physiological roles in optimizing plant photosynthetic capacity via mediating differential cell growth in the phototropic response, chloroplast movement, stomatal opening, and leaf flattening (Matsuoka and Tokutomi, 2005; Inoue et al., 2008; Christie et al., 2014). PHOTs’ primary structure consists of an N-terminal photosensory region and a C-terminal AGC type Ser/Thr protein kinase domain. Two flavin mononucleotide (FMN) chromophore bound to light oxygen voltage (LOV1 and LOV2) domains usually sense blue light (Figures 2C, H) (Suetsugu and Wada, 2012). In Arabidopsis blue light–activated PHOT1 (Kharshiing and Sinha, 2015) and PHOT2 have very similar properties (Jarillo et al., 1998; Christie and Briggs, 2001; Briggs and Christie, 2002; Staiger, 2008; Kharshiing and Sinha, 2015; Zhao et al., 2018b). Recently, the generation of a slow-photocycling variants of phot1 or phot2 was found to increase biomass production under light-limiting conditions due to improved sensitivity to light (Hart et al., 2019).
Zeitlupes (ZTL, FKF1, and LKP2) absorb blue light/UV-A (315–500 nm) and contain both photoreceptor and F-box protein activities within the same protein. Land plants encompass an extra family of UV-A/blue light photoreceptors that consist of ZTL, flavin-binding, kelch repeat, F-box (FKF1), and lov kelch protein2 (LKP2) proteins that are collectively named as Zeitlupes (Ito et al., 2012; Suetsugu and Wada, 2012). ZTLs form signaling complexes with factors of the circadian clock and photoperiodic flowering (Zoltowski and Imaizumi, 2014). ZTLs have a single FMN-binding LOV domain followed by an F-box and six-Kelch-repeat domain (Figures 2D, H) (Ito et al., 2012).
UV resistance locus 8 (UVR8) photoreceptor operates through UV-B light (280–315 nm) for UV acclimation in sunlight (Kaiserli and Jenkins, 2007; Rizzini et al., 2011; Rizzini et al., 2011; Christie et al., 2012; Christie et al., 2012; Jenkins, 2014b; Qian et al., 2016; Yin et al., 2016). UVR8 is a UV-B light photoreceptor using tryptophan residues as a chromophore (Figure 2E, I) to mediate phototropic bending, stomatal movement, and entrainment of the circadian clock (Fehér et al., 2011; Jenkins, 2014a; Tossi et al., 2014; Vandenbussche et al., 2014). UVR8 triggers large changes in gene expression leading to morphological adaptations and the production of flavonols to avoid the harmful effects of UV-B light (Favory et al., 2009; Rizzini et al., 2011; Jenkins, 2014b). Tomato SlUVR8 mediates plant acclimation to UV-B stress by orchestrating expression of the UVB-responsive genes such as elongated hypocotyl5 (HY5) and chalcone synthase (CHS) and accumulating UV-absorptive compounds. Furthermore, SlUVR8 enhances fruit chloroplast development accumulating transcription factor golden2-like 2 (SlGLK2) (Li et al., 2018).
Interaction and Evolution of Photoreceptors and Membrane Transporters
Comprehensive knowledge on plant membrane transporters and photoreceptors systems along with the increasing number of sequenced plant genomes have enabled the comparative genomic and evolutionary analysis of many gene families (Cai et al., 2017; Chen et al., 2017; Wang et al., 2018; Zhao et al., 2019). Discovering orthologous membrane transporters and photoreceptors and deciphering their key functions have also led to a growing interest in the manipulation of these genes to enhance crop yield and quality (Boccalandro et al., 2003; Ganesan et al., 2017).
Evolution of Photoreceptors and Membrane Transporters
Photosynthetic organisms use a cluster of photoreceptors to sense the quality, quantity, and direction of light for photosynthesis and consequently growth and development over the long evolutionarily trajectory. Based on the evolutionary analysis of photoreceptors (Lariguet and Dunand, 2005; Zhao et al., 2019) and key membrane transporters (Cai et al., 2017; Chen et al., 2017; Wang et al., 2018; Li et al., 2019) across different land-plant and algal species, we proposed that evolutionary links between these photoreceptors and membrane transporters in various land-plant and algal species may provide clues toward light-induced interaction and potential co-evolution of photoreceptors with membrane transporters.
Photoreceptors have evolved across photosynthetic eukaryotes in land-plant species. Increase in the number of photoreceptors and function during plant evolution are associated with fitness enhancement (Rensing et al., 2016). Plant PHYs, PHOTs, CRYs, UVR8, and ZTLs originated in an ancestor of streptophytes (Li et al., 2015a; Zhao et al., 2019), and these photoreceptors mediated optimization of photosynthesis and photoprotection across green plants (Li et al., 2015b; Demarsy et al., 2018; Zhao et al., 2019). Liverworts, hornworts, and Selaginella have a single PHY, whereas mosses, lycophytes, ferns, and seed plants evolved diverse PHY sub-families due to independent gene duplications (Li et al., 2015a). PHOTs have been independent duplicated in most major land-plant lineages including mosses, lycophytes, ferns, and seed plants but had only single-copy genes in liverworts and hornworts (Li et al., 2015b). Four PHYs, two PHOTs, and five CRYs have been identified in the fern species Adiantum capillus-veneris (Kagawa et al., 2004). In addition, red/far-red and a blue light are sensed by a neochrome, a chimeric photoreceptor kinase combining a PHY photosensitive domain and PHOT, for phototropic response and chloroplast movement in Adiantum capillus-veneris (Nozue et al., 1998; Kawai et al., 2003). In mosses, liverworts, and ferns, chloroplast movement is enhanced by the PHYs, PHOTs, and/or neochromes in both red and blue lights, whereas rotation of the single chloroplast exhibits light-modulated chloroplast avoidance during the exposure of high light in green algae Mougeotia or Mesotaenium (Suetsugu et al., 2017). The liverwort Marchantia polymorphia shows early evolution of ZTLs in the plant lineage in the photoperiodic phase transition (Kubota et al., 2014). Moreover, the high levels of conserved structure of ZTL homologs among monocots and dicots suggest a certain similarity function of these genes across species (Taylor et al., 2010). UVR8 was evolutionarily well conserved within the green lineage, from green algae to angiosperms, and was expressed throughout the plant life cycle (Rizzini et al., 2011; Fernández et al., 2016; Tilbrook et al., 2016; Soriano et al., 2018; Zhao et al., 2019). For instance, in Arabidopsis and Chlamydomonas reinhardtii, only some families of photoreceptors are overlapped and preserved (Demarsy et al., 2018). Arabidopsis contains PHYs (PHYA-E), CRYs (CRY1-2), PHOTs (PHOT1-2), ZTLs, and UVR8 (Heijde and Ulm, 2012; Galvão and Fankhauser, 2015). By comparison, in the C. reinhardtii, UVR8 detects UV-B light whereas blue/UV-A is detected by one PHOT (PHOT1), two CRY-DASHs, one plant-like CRY (pCRY), and one animal-like CRY (aCRY) (Petroutsos, 2017). The latter shows responses both in blue and red lights (Beel et al., 2012; Kianianmomeni and Hallmann, 2014; Tilbrook et al., 2016). However, PHYs and ZTLs are not found in most of the algal species tested (Zhao et al., 2019). Instead, aureochrome (aureo) and opsin are identified in algae by recent genomic studies apart from major photoreceptors (Kianianmomeni and Hallmann, 2014; Essen et al., 2017; Kroth et al., 2017). The functional motifs of UVR8 are widely conserved in green algae, bryophytes, lycophytes, and angiosperms (Fernández et al., 2016), but not in gymnosperms because of redundancy of this photoreceptor or there is an alternative in gymnosperms (Tossi et al., 2019).
Plant membrane transporters have been co-evolved toward higher clades of plant throughout their evolutionary trajectory. The retention of these key membrane transporters and proteins could have had a profound effect on the adaptation of land species during evolution (Chen et al., 2017; Wang et al., 2018). Furthermore, most of the tested algae appeared not to have some families of the membrane transporters compared to plant species. For instance, Arabidopsis plasma membrane H+-ATPase (AHAs) autoinhibited Ca2+-ATPase (ACAs); Na+/H+ antiporter (NHXs), ABCs, CLCs, and CAXs are found amongst plants and algae during evolution, whereas SLACs, ALMTs, and high-affinity K+/Na+ transporter (HKTs) seem to be evolved after the colonization of terrestrial habitats by land plants (Cai et al., 2017; Chen et al., 2017; Wang et al., 2018). The high conservation of transporter protein families points to early acquisition of active control membrane transport mechanisms in plants (Chater et al., 2011; Ruszala et al., 2011; Komatsu et al., 2013; Lind et al., 2015; Chen et al., 2017). Interestingly, homologs of many Arabidopsis transporters such as SLAC1, ALMT12, TPK1, slow vacuolar Ca2+ channel (TPC1), and AHA1 have been found in all land-plant species studied (Cai et al., 2017; Cao, 2019), which are consistent with the evolution of photoreceptors (Zhao et al., 2019). Moreover, comparative genomic analysis revealed the evolutionary conservation of aquaporins, such as the plasma membrane intrinsic proteins (PIPs) family from algae to angiosperm in the long-term natural selection of land plants (Cai et al., 2017; Chen et al., 2017; Wang et al., 2018; Li et al., 2019). In the interaction between photoreceptors and membrane transporters, light-dependent production of assimilates regulates AKT2/3 transcript through a CO2-dependent mechanism in Arabidopsis (Deeken et al., 2000). The moss P. patens displays light-induced action potential changes and its chloroplasts, and PHYs are essential for plasma membrane channels’ activation through changes in cytosolic Ca2+ (Ermolayeva et al., 1996; Koselski et al., 2008). Light-dependent changes in plasma membrane ion transport have also been studied in the algae Eremosphaera viridis (Schönknecht et al., 1998) and C. reinhardtii (Nagel et al., 2002; Nagel et al., 2003), where light regulates phototactic and photophobic responses with the initiation of channelrhodopsins 1 and 2 (ChR1 and ChR2). The currents conducted by channelrhodopsins in high light intensities are likely to depolarize the Chlamydomonas plasma membrane with the mediation mostly of proton/cation flow (Harz and Hegemann, 1991; Harz et al., 1992; Schneider et al., 2015). Are these membrane transporters co-evolved with the photoreceptors during the evolutionary of plants from aquatic to terrestrial life? Is there any direct or indirect regulation of these photoreceptors on the membrane transporters in order to transduce the light signals?
Exploration of the evolution of membrane transporters and photoreceptors can be advantageous with these evolutionary genomics and bioinformatics techniques toward better understanding of their molecular evolutions throughout the evolutionary trajectory. Nevertheless, further research is needed to answer these questions combining the evolutionary analysis with their functional domains, cloning the key genes, and complementing or overexpressing these genes in different plant species.
Regulation of Photoreceptors on Plasma Membrane Transport
Here, we summarize the identified photoreceptors and their interactions with the down-stream signaling components in plants. PHOTs are the obvious candidates for this role as these protein kinases are associated with the plasma membrane (Briggs and Christie, 2002; Sakamoto and Briggs, 2002). CRYs predominantly localized within the nucleus (Wu and Spalding, 2007) are unlikely to directly regulate membrane transport. PHYs are able to affect membrane transport, at least in mosses (Ermolayeva et al., 1996; Ermolayeva et al., 1997). Furthermore, ZTLs mainly regulate circadian rhythms and are unlikely to have a strong influence on membrane transport (Más et al., 2003; Demarsy and Fankhauser, 2009).
PHY-induced inward Ca2+ current immediately starts to depolarize the membrane while concurrently increasing [Ca2+]cyt (Ermolayeva et al., 1996; Ermolayeva et al., 1997). Irradiation of dark-adapted caulonemal filaments of P. patens with red light evoked changes of Em. The transient depolarization was blocked by application of far-red light, K+ channel–blocker tetraethylammonium, the Cl− channel–blocker niflumic acid, removing Ca2+ from the external medium or replacing Ca2+ with Mg2+. Thus, K+, Cl−, and Ca2+ transporters may be involved in the early events in the PHY signaling pathway (Ermolayeva et al., 1996). Moreover, voltage clamp and ion flux measurements showed that K+ and Ca2+ channels are activated at the red light–induced depolarization, indicating red light–induced PHYs and ion channel interaction at the plasma membrane (Ermolayeva et al., 1997). However, research on direct PHY-mediated effects on membrane transporters are yet to be demonstrated.
PHOT1 and PHOT2 regulate membrane transport via the changes of [Ca2+]cyt in plants (Harada et al., 2003). It was suggested that PHOT1 regulates blue light–induced Ca2+ uptake into the cytoplasm from the apoplast in etiolated Arabidopsis seedlings and significant changes in Ca2+ and H+ fluxes (Babourina et al., 2002). It was shown that blue light activates voltage-dependent calcium-permeable channels in the plasma membrane of mesophyll cells. Blue-light stimulated photoreceptors control of Ca2+-channel activity, which was dramatically reduced in phot1-5 and was eliminated in the double mutant phot1-5phot2-1. By contrast, in cry1-3cry2-1 double mutant, the Ca2+ channel remained sensitive to blue light (Stoelzle et al., 2003). PHOTs can also affect ion transport in the long-term through modulation of auxin transport on Zea mays K+ channel 1 (ZMK1) (Fuchs et al., 2003). Although PHOT signaling has been intensively studied, there are still unresolved nodes for the blue light signal to be passed onto membrane transport proteins.
CRYs exported to the cytoplasm upon illumination (Yang et al., 2000) can potentially interact with plasma membrane transporter proteins. Mutant hy4 lacking the CRY1 exhibits a membrane depolarization merely 30% of the wild-type magnitude (Spalding, 2000), which indicates that CRY1 may indirectly modify ionic currents across the plasma membrane (Parks et al., 1998). The excitation of CRY1 by blue light leads to the activation of anion channels at the plasma membrane of etiolated Arabidopsis hypocotyl cells within seconds (Cho and Spalding, 1996), resulting in membrane depolarization (Parks et al., 1998). The rapidity of its action may be explained by the presence of significant fractions of functional CRY1 in the cytoplasm of Arabidopsis hypocotyl cells or the emerging of CRY1-dependent signal from the nucleus to regulate channel gating within the first few seconds of illumination (Guo et al., 1999). However, this link is still largely unresolved due to limited advancement in this area of research.
Photoreceptors and Membrane Transporters in Light Signaling Network
Photoreceptors may coordinate with membrane transporters in light-induced signaling transduction. Here, guard cells are used to demonstrate the role of light-dependent photoreceptors and membrane transport in stomatal opening (Figure 3) (Roelfsema and Hedrich, 2005; Chen et al., 2012a; Inoue and Kinoshita, 2017; Ando and Kinoshita, 2018).
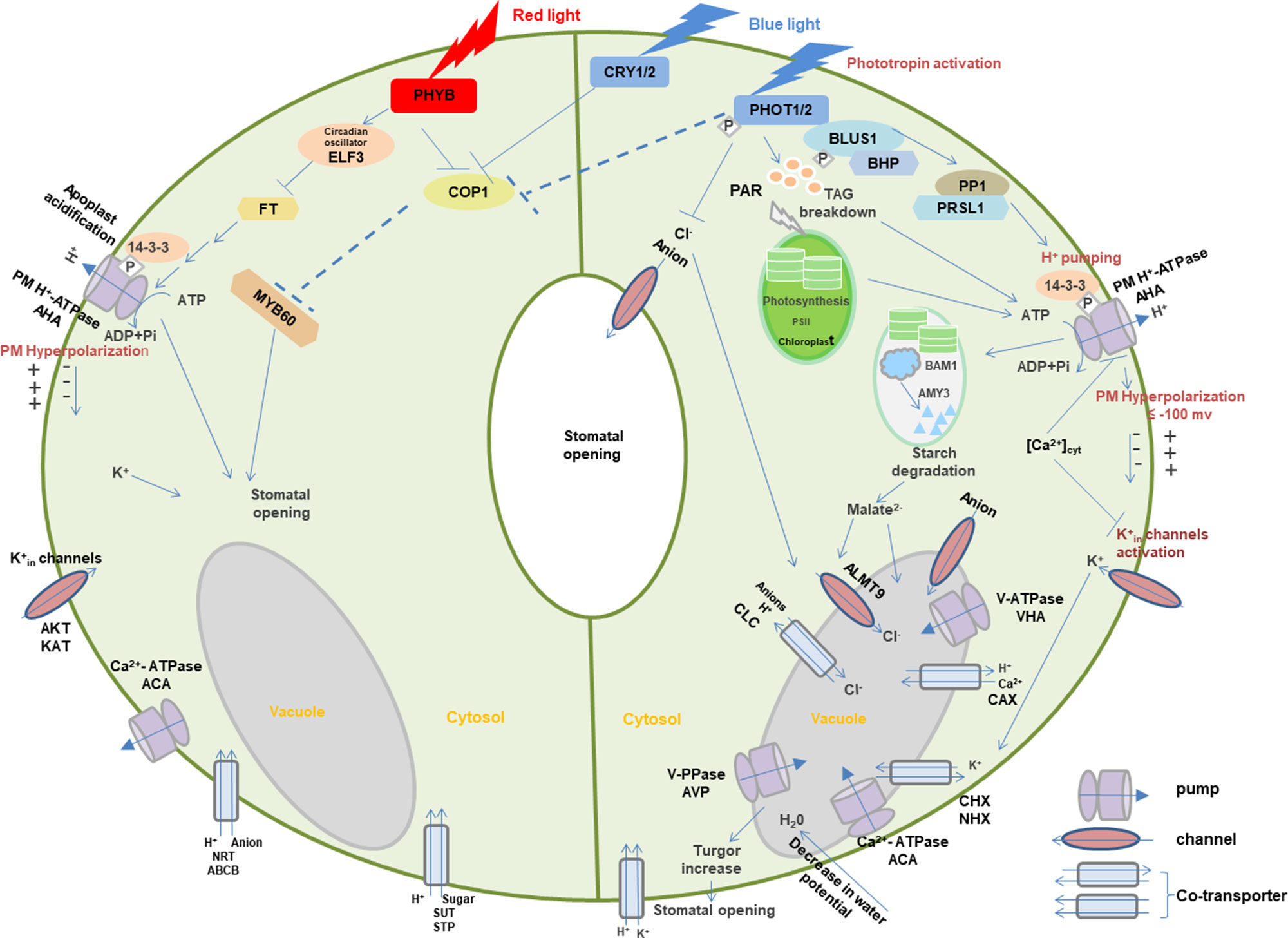
Figure 3 Light-induced interaction between photoreceptors and membrane transporters in stomatal guard cells. In blue light, PHOTs are autophosphorylated and start a signaling cascade to activate H+-ATPase which eventually results in the opening of stomata through the signals transduced downstream with the generation of different substrates such as BLUS1, PP1, and 14-3-3 protein. Blue light–induced H+-ATPase activation causes the hyperpolarization of the plasma membrane followed by the activation of inward-rectifying K+ channels to accumulate K+ ions, resulting in the turgor increase that leads to stomatal opening. Moreover, blue light-mediated signaling downstream of H+-ATPase activity degrades starch in guard cell chloroplasts. Blue light–induced stomatal regulation is also mediated by CRYs. Red light regulates stomatal opening through signaling transduction of PHYB, COP1, ELF3, FT,14-3-3 protein, AHA, KAT, ACA, SUT, NRT, and potentially MYBs. Arrows, T-bars, and dotted T-bar lines represent positive, negative, and hypothetical regulations, respectively. The P in the white rhombus indicates phosphorylation of proteins. PHOT, Phototropin; BLUS1, blue light signaling1; BHP, blue light-dependent H+-ATPase phosphorylation; PP1, type 1 protein phosphatase; PRSL1, regulatory subunit of protein phosphatase 1; 14-3-3, 14-3-3 protein; TAG, triacylglycerol; CRY, cryptochrome; PHY, phytochrome; COP1, constitutive photomorphogenic1; ELF3, early flowering 3; FT, flowering locus t; ALMT, aluminum-activated malate transporter; CLC, anion channel/anion/H+ antiporter; PAR, photosynthetically active radiation; PM, plasma membrane. AHA, plasma membrane H+-ATPase; VHA, vacuolar H+-ATPase; ACA, Ca2+-ATPase; AVP, vacuolar H+/K+-PPase; AKT, KAT, and KC, K+ inward-rectifying channels; CAX, Ca2+/H+ antiporter; CHX, cation/H+ exchanger; NHX, Na+(K+)/H+ antiporter; SUT, sucrose transporter; STP, monosaccharide/H+ symporter; NRT, nitrate transporter; ABCB, ATP-binding Cassette transporter. Models are adapted from (Shimazaki et al., 2007; Chen and Blatt, 2010; Chen et al., 2012a; Inoue and Kinoshita, 2017; Ando and Kinoshita, 2018).
When guard cells are illuminated with blue light, PHOTs are triggered through auto-phosphorylation for the initiation of signaling for stomatal opening (Kinoshita et al., 2001; Christie, 2007). Upon blue light, two Ser residues are auto-phosphorylated in the kinase activation loop of PHOTs, and phosphorylation is essential to transduce signaling downstream through substrate recognition (Inoue et al., 2008; Inoue et al., 2010; Inoue et al., 2011). The activated PHOTs directly phosphorylate another protein kinase blue light signaling1 (BLUS1), which indirectly conveys the signal to type 1 protein phosphatase (PP1) and its regulatory subunit PRSL1 (Takemiya et al., 2006; Takemiya et al., 2012; Takemiya et al., 2013; Takemiya and Shimazaki, 2016). Furthermore, a Raf-like protein kinase, blue light–dependent H+-ATPase phosphorylation (BHP) bound to BLUS1 forms an early signaling complex with PHOTs to facilitate phosphorylation of a second last Thr of the PM H+-ATPase (Hayashi et al., 2017). The signal produced by BLUS1 finally triggers the PM H+-ATPase in guard cells through phosphorylation of Thr in the C terminus with successive binding of the 14-3-3 protein (Shimazaki et al., 2007; Hayashi et al., 2011; Yamauchi et al., 2016). H+-ATPase activation in blue light drives H+ pumping and causes the hyperpolarization of the plasma membrane (Shimazaki et al., 2007; Marten et al., 2010). Besides, red light–induced fluency rate-dependent PM H+-ATPase phosphorylation in guard cells (Ando and Kinoshita, 2018) promotes stomatal opening (Ando and Kinoshita, 2019). This hyperpolarization activates inward-rectifying K+ (K+in) channels (Lebaudy et al., 2008; Kim et al., 2010), which leads to the accumulation of K+. Water potential of guard cells is decreased due to accumulation of ions, resulting in water uptake into the vacuole accompanied with the turgor increase for stomatal opening (Inoue et al., 2010; Marten et al., 2010). Moreover, PHOT-mediated signaling downstream of H+-ATPase activity degrades starch in guard cell chloroplasts (Santelia and Lunn, 2017), which thus leads to stomatal opening possibly through malate synthesis involving β-amylase 1 (BAM1) and α-amylase 3 (AMY3) (Shimazaki et al., 2007; Horrer et al., 2016).
Blue light–induced stomatal regulation is also mediated by CRYs, interacting with PHYB-regulated red light–induced stomatal movement. COP1 plays a negative role in CRYs- and PHYB-induced signaling pathways (Chen et al., 2012a). Furthermore, stomatal aperture may be regulated downstream of the photoreceptors by the transcription factor such as MYB60 (Chen et al., 2012a). Circadian clock protein early flowering 3 (ELF3) and flowering locus t (FT) are also the important intermediates in the red light signaling pathway for stomatal regulation (Hicks et al., 2001). These signals are likely to regulate photoreceptor-mediated stomatal opening in different light via the modulation of ion transport through various channels and co-transporters with the light activation of pumps. However, many signaling components are still missing in the light-induced stomatal regulation, which requires extensive investigation in the future.
Conclusions and Future Perspective
Although a great deal of research has been undertaken to study the effects of light irradiances on the plant growth and development, very little is known about the light-induced mechanisms involved in ion transport regulation of plants. An emphasis on the light-regulated membrane transport processes is necessary to synchronize light responses with receptor recognition, cellular homeostasis, and developmental programming. Establishing crucial links between ion transporters and photoreceptors recognition in light signaling transduction are only beginning to be explored. A large number of genes encoding photoreceptors and their potential downstream interacting transporters are identified in light-induced signaling pathway, but how these proteins interact to achieve rapid signaling transductions are yet to be investigated. Moreover, the knowledge of photoreceptors and downstream signaling pathways is, to a large extent, based on extensive studies in Arabidopsis. Few studies have focused on regulation of membrane transport and their interactions with photoreceptors in other species, especially in crops and early land plants. Thus, a deeper understanding of the molecular evolution and interaction of plasma membrane ion transporters with photoreceptors can shed new light on plant ecological conservation as well as agricultural production and crop quality, bringing potential nutrition and health benefits to animals and humans.
Author Contributions
Z-HC and MB conceived and designed the research. SC and GC conducted the literature search and protein domain analysis. MB and Z-HC wrote the manuscript with contributions from DT and CC.
Conflict of Interest
The authors declare that the research was conducted in the absence of any commercial or financial relationships that could be construed as a potential conflict of interest.
The handling editor is currently organizing a Research Topic with one of the authors, Z-HC, and confirms the absence of any other collaboration.
Acknowledgments
This work is supported by Australian Research Council (ARC) and Chinese 1000-Plan projects to Z-HC, Horticulture Innovation Australia projects (MT13041, VG17003, LP18000), and National Natural Science Foundation of China and Zhejiang University Kuayue Project to SC.
References
Adamiec, M., Drath, M., Jackowski, G. (2008). Redox state of plastoquionone pool regulates expression of Arabidopsis thaliana genes in response to elevated irradiance. Acta Biochim. Polon. Eng. Ed.55, 161.
Ahmad, M., Cashmore, A. R. (1993). HY4 gene of A. thaliana encodes a protein with characteristics of a blue-light photoreceptor. Nature366, 162. doi: 10.1038/366162a0
Ahmad, M., Jarillo, J. A., Cashmore, A. R. (1998). Chimeric proteins between cry1 and cry2 Arabidopsis blue light photoreceptors indicate overlapping functions and varying protein stability. Plant Cell10, 197–207. doi: 10.1105/tpc.10.2.197
Alba, R., Cordonnier-Pratt, M.-M., Pratt, L. H. (2000). Fruit-localized phytochromes regulate lycopene accumulation independently of ethylene production in tomato. Plant Physiol.123, 363–370. doi: 10.1104/pp.123.1.363
Albanese, P., Manfredi, M., Re, A., Marengo, E., Saracco, G., Pagliano, C. (2018). Thylakoid proteome modulation in pea plants grown at different irradiances: quantitative proteomic profiling in a non-model organism aided by transcriptomic data integration. Plant J.96, 786–800. doi: 10.1111/tpj.14068
Ando, E., Kinoshita, T. (2018). Red light-induced phosphorylation of plasma membrane H+-ATPase in stomatal guard cells. Plant Physiol.178, 838–849. doi: 10.1104/pp.18.00544
Ando, E., Kinoshita, T. (2019). Fluence rate dependence of red light-induced phosphorylation of plasma membrane H+-ATPase in stomatal guard cells. Plant Signal. Behav.14, 1561107. doi: 10.1080/15592324.2018.1561107
Armbruster, U., Carrillo, L. R., Venema, K., Pavlovic, L., Schmidtmann, E., Kornfeld, A., et al. (2014). Ion antiport accelerates photosynthetic acclimation in fluctuating light environments. Nat. Commun.5, 5439. doi: 10.1038/ncomms6439
Assmann, S., Simoncini, L., Schroeder, J. (1985). Blue light activates electrogenic ion pumping in guard cell protoplasts of Vicia faba. Nature318, 285–287. doi: 10.1038/318285a0
Azari, R., Tadmor, Y., Meir, A., Reuveni, M., Evenor, D., Nahon, S., et al. (2010). Light signaling genes and their manipulation towards modulation of phytonutrient content in tomato fruits. Biotechnol. Adv.28, 108–118. doi: 10.1016/j.biotechadv.2009.10.003
Babourina, O., Newman, I., Shabala, S. (2002). Blue light-induced kinetics of H+ and Ca2+ fluxes in etiolated wild-type and phototropin-mutant Arabidopsis seedlings. Proc. Natl. Acad. Sci.99, 2433–2438. doi: 10.1073/pnas.042294599
Beel, B., Prager, K., Spexard, M., Sasso, S., Weiss, D., Müller, N., et al. (2012). A flavin binding cryptochrome photoreceptor responds to both blue and red light in Chlamydomonas reinhardtii. Plant Cell24, 2992–3008. doi: 10.1105/tpc.112.098947
Belbin, F. E., Noordally, Z. B., Wetherill, S. J., Atkins, K. A., Franklin, K. A., Dodd, A. N. (2017). Integration of light and circadian signals that regulate chloroplast transcription by a nuclear-encoded sigma factor. New Phytol.213, 727–738. doi: 10.1111/nph.14176
Blom-Zandstra, M., Koot, H., Van Hattum, J., Vogelzang, S. A. (1997). Transient light-induced changes in ion channel and proton pump activities in the plasma membrane of tobacco mesophyll protoplasts. J. Exp. Bot.48, 1623–1630. doi: 10.1093/jxb/48.9.1623
Boccalandro, H. E., Ploschuk, E. L., Yanovsky, M. J., Sánchez, R. A., Gatz, C., Casal, J. J. (2003). Increased phytochrome B alleviates density effects on tuber yield of field potato crops. Plant Physiol.133, 1539–1546. doi: 10.1104/pp.103.029579
Boulton, R. (1980a). A hypothesis for the presence, activity, and role of potassium/hydrogen, adenosine triphosphatases in grapevines. Am. J. Enol. Vitic.31, 283–287.
Boulton, R. (1980b). The relationships between total acidity, titratable acidity and pH in wine. Am. J. Enol. Vitic.31, 76–80.
Brandt, S., Pék, Z., Barna, É., Lugasi, A., Helyes, L. (2006). Lycopene content and colour of ripening tomatoes as affected by environmental conditions. J. Sci. Food Agric.86, 568–572. doi: 10.1002/jsfa.2390
Briggs, W. R., Christie, J. M. (2002). Phototropins 1 and 2: versatile plant blue-light receptors. Trends Plant Sci.7, 204–210. doi: 10.1016/S1360-1385(02)02245-8
Burgie, E. S., Vierstra, R. D. (2014). Phytochromes: an atomic perspective on photoactivation and signaling. Plant Cell26, 4568–4583. doi: 10.1105/tpc.114.131623
Cai, S., Chen, G., Wang, Y., Huang, Y., Marchant, D. B., Wang, Y., et al. (2017). Evolutionary conservation of ABA signaling for stomatal closure. Plant Physiol.174, 732–747. doi: 10.1104/pp.16.01848
Cao, J. (2019). Molecular evolution of the vacuolar iron transporter (VIT) family genes in 14 plant species. Genes10, 144. doi: 10.3390/genes10020144
Carraretto, L., Formentin, E., Teardo, E., Checchetto, V., Tomizioli, M., Morosinotto, T., et al. (2013). A thylakoid-located two-pore K+ channel controls photosynthetic light utilization in plants. Science342, 114–118. doi: 10.1126/science.1242113
Carvalho, R. F., Damião, V. D. A. (2018). Cryptochrome-related abiotic stress responses in plants. Front. Plant Sci.9, 1897. doi: 10.3389/fpls.2018.01897
Cashmore, A. R., Jarillo, J. A., Wu, Y.-J., Liu, D. (1999). Cryptochromes: blue light receptors for plants and animals. Science284, 760–765. doi: 10.1126/science.284.5415.760
Chater, C., Kamisugi, Y., Movahedi, M., Fleming, A., Cuming, A. C., Gray, J. E., et al. (2011). Regulatory mechanism controlling stomatal behavior conserved across 400 million years of land plant evolution. Curr. Biol.21, 1025–1029. doi: 10.1016/j.cub.2011.04.032
Chaves, I., Pokorny, R., Byrdin, M., Hoang, N., Ritz, T., Brettel, K., et al. (2011). The cryptochromes: blue light photoreceptors in plants and animals. Annu. Rev. Plant Biol.62, 335–364. doi: 10.1146/annurev-arplant-042110-103759
Chen, C., Xiao, Y.-G., Li, X., Ni, M. (2012a). Light-regulated stomatal aperture in Arabidopsis. Mol. Plant5, 566–572. doi: 10.1093/mp/sss039
Chen, Z.-H., Chen, G., Dai, F., Wang, Y., Hills, A., Ruan, Y.-L., et al. (2017). Molecular evolution of grass stomata. Trends Plant Sci.22, 124–139. doi: 10.1016/j.tplants.2016.09.005
Chen, Z.-H., Hills, A., Bätz, U., Amtmann, A., Lew, V. L., Blatt, M. R. (2012b). Systems dynamic modeling of the stomatal guard cell predicts emergent behaviors in transport, signaling, and volume control. Plant Physiol.159, 1235–1251. doi: 10.1104/pp.112.197350
Chen, Z., Pottosin, I. I., Cuin, T. A., Fuglsang, A. T., Tester, M., Jha, D., et al. (2007). Root plasma membrane transporters controlling K+/Na+ homeostasis in salt-stressed barley. Plant Physiol.145, 1714–1725. doi: 10.1104/pp.107.110262
Chen, Z. H., Blatt, M. R. (2010). Membrane transport in guard cells. eLS. doi: 10.1002/9780470015902.a0021630
Cheng, N.-H., Pittman, J. K., Barkla, B. J., Shigaki, T., Hirschi, K. D. (2003). The Arabidopsis cax1 mutant exhibits impaired ion homeostasis, development, and hormonal responses and reveals interplay among vacuolar transporters. Plant Cell15, 347–364. doi: 10.1105/tpc.007385
Chincinska, I. A., Liesche, J., Krügel, U., Michalska, J., Geigenberger, P., Grimm, B., et al. (2008). Sucrose transporter StSUT4 from potato affects flowering, tuberization, and shade avoidance response. Plant Physiol.146, 515–528. doi: 10.1104/pp.107.112334
Cho, M. H., Spalding, E. P. (1996). An anion channel in Arabidopsis hypocotyls activated by blue light. Proc. Natl. Acad. Sci.93, 8134–8138. doi: 10.1073/pnas.93.15.8134
Christie, J. M. (2007). Phototropin blue-light receptors. Annu. Rev. Plant Biol.58, 21–45. doi: 10.1146/annurev.arplant.58.032806.103951
Christie, J. M., Arvai, A. S., Baxter, K. J., Heilmann, M., Pratt, A. J., O’hara, A., et al. (2012). Plant UVR8 photoreceptor senses UV-B by tryptophan-mediated disruption of cross-dimer salt bridges. Science335, 1492–1496. doi: 10.1126/science.1218091
Christie, J. M., Blackwood, L., Petersen, J., Sullivan, S. (2014). Plant flavoprotein photoreceptors. Plant Cell Physiol.56, 401–413. doi: 10.1093/pcp/pcu196
Christie, J. M., Briggs, W. R. (2001). Blue light sensing in higher plants. J. Biol. Chem.276, 11457–11460. doi: 10.1074/jbc.R100004200
Davies, J. N., Hobson, G. E., Mcglasson, W. (1981). The constituents of tomato fruit—the influence of environment, nutrition, and genotype. Crit. Rev. Food Sci. Nutr.15, 205–280. doi: 10.1080/10408398109527317
Davis, C. D., Uthus, E. O. (2004). DNA methylation, cancer susceptibility, and nutrient interactions. Exp. Biol. Med.229, 988–995. doi: 10.1177/153537020422901002
Deeken, R., Sanders, C., Ache, P., Hedrich, R. (2000). Developmental and light-dependent regulation of a phloem-localised K+ channel of Arabidopsis thaliana. Plant J.23, 285–290. doi: 10.1046/j.1365-313x.2000.00791.x
Demarsy, E., Fankhauser, C. (2009). Higher plants use LOV to perceive blue light. Curr. Opin. Plant Biol.12, 69–74. doi: 10.1016/j.pbi.2008.09.002
Demarsy, E., Goldschmidt-Clermont, M., Ulm, R. (2018). Coping with ‘dark sides of the sun’through photoreceptor signaling. Trends Plant Sci.23, 260–271. doi: 10.1016/j.tplants.2017.11.007
Devlin, P. F., Kay, S. A. (2000). Cryptochromes are required for phytochrome signaling to the circadian clock but not for rhythmicity. Plant Cell12, 2499–2509. doi: 10.1105/tpc.12.12.2499
Dodd, A., Gardner, M., Hotta, C., Hubbard, K., Dalchau, N., Love, J., et al. (2007). A cADPR-based feedback loop modulates the Arabidopsis circadian clock. Science318, 1789–1792. doi: 10.1126/science.1146757
Drozdowicz, Y. M., Rea, P. A. (2001). Vacuolar H+ pyrophosphatases: from the evolutionary backwaters into the mainstream. Trends Plant Sci.6, 206–211. doi: 10.1016/S1360-1385(01)01923-9
Dukic, E., Herdean, A., Cheregi, O., Sharma, A., Nziengui, H., Dmitruk, D., et al. (2019). K+ and Cl– channels/transporters independently fine-tune photosynthesis in plants. Sci. Rep.9, 8639. doi: 10.1038/s41598-019-44972-z
Eder, J., Cosio, E. G. (1994). Elicitors of plant defense responses. Int. Rev. Cytol.148, 1–36. doi: 10.1016/S0074-7696(08)62404-3
El-Esawi, M., Arthaut, L.-D., Jourdan, N., D’harlingue, A., Link, J., Martino, C. F., et al. (2017). Blue-light induced biosynthesis of ROS contributes to the signaling mechanism of Arabidopsis cryptochrome. Sci. Rep.7, 13875. doi: 10.1038/s41598-017-13832-z
Elzenga, J. T. M., Prins, H. B., Van Volkenburgh, E. (1995). Light-induced membrane potential changes of epidermal and mesophyll cells in growing leaves of Pisum sativum. Planta197, 127–134. doi: 10.1007/BF00239948
Elzenga, J. T. M., Van Volkenburgh, E. (1997). Characterization of a light-controlled anion channel in the plasma membrane of mesophyll cells of pea. Plant Physiol.113, 1419–1426. doi: 10.1104/pp.113.4.1419
Ermolayeva, E., Hohmeyer, H., Johannes, E., Sanders, D. (1996). Calcium-dependent membrane depolarisation activated by phytochrome in the moss Physcomitrella patens. Planta199, 352–358. doi: 10.1007/BF00195726
Ermolayeva, E., Sanders, D., Johannes, E. (1997). Ionic mechanism and role of phytochrome-mediated membrane depolarisation in caulonemal side branch initial formation in the mossPhyscomitrella patens. Planta201, 109–118. doi: 10.1007/BF01007695
Escoubas, J.-M., Lomas, M., Laroche, J., Falkowski, P. G. (1995). Light intensity regulation of cab gene transcription is signaled by the redox state of the plastoquinone pool. Proc. Natl. Acad. Sci.92, 10237–10241. doi: 10.1073/pnas.92.22.10237
Essen, L.-O., Franz, S., Banerjee, A. (2017). Structural and evolutionary aspects of algal blue light receptors of the cryptochrome and aureochrome type. J. Plant Physiol.217, 27–37. doi: 10.1016/j.jplph.2017.07.005
Evans, D. A., Hirsch, J. B., Dushenkov, S. (2006). Phenolics, inflammation and nutrigenomics. J. Sci. Food Agric.86, 2503–2509. doi: 10.1002/jsfa.2702
Fairley-Grenot, K., Assmann, S. (1992). Permeation of Ca2+ through K+ channels in the plasma membrane of Vicia faba guard cells. J. Membr. Biol.128, 103–113. doi: 10.1007/BF00231883
Fan, L.-M., Zhao, Z., Assmann, S. M. (2004). Guard cells: a dynamic signaling model. Curr. Opin. Plant Biol.7, 537–546. doi: 10.1016/j.pbi.2004.07.009
Fan, X.-X., Xu, Z.-G., Liu, X.-Y., Tang, C.-M., Wang, L.-W., Han, X.-L. (2013). Effects of light intensity on the growth and leaf development of young tomato plants grown under a combination of red and blue light. Sci. Hortic.153, 50–55. doi: 10.1016/j.scienta.2013.01.017
Fankhauser, C., Chen, M. (2008). Transposing phytochrome into the nucleus. Trends Plant Sci.13, 596–601. doi: 10.1016/j.tplants.2008.08.007
Favory, J. J., Stec, A., Gruber, H., Rizzini, L., Oravecz, A., Funk, M., et al. (2009). Interaction of COP1 and UVR8 regulates UV-B-induced photomorphogenesis and stress acclimation in Arabidopsis. EMBO J.28, 591–601. doi: 10.1038/emboj.2009.4
Fehér, B., Kozma-Bognár, L., Kevei, É., Hajdu, A., Binkert, M., Davis, S. J., et al. (2011). Functional interaction of the circadian clock and UV RESISTANCE LOCUS 8-controlled UV-B signaling pathways in Arabidopsis thaliana. Plant J.67, 37–48. doi: 10.1111/j.1365-313X.2011.04573.x
Feng, C.-M., Qiu, Y., Van Buskirk, E. K., Yang, E. J., Chen, M. (2014). Light-regulated gene repositioning in Arabidopsis. Nat. Commun.5, 3027. doi: 10.1038/ncomms4027
Fernández, M. B., Tossi, V., Lamattina, L., Cassia, R. (2016). A comprehensive phylogeny reveals functional conservation of the UV-B photoreceptor UVR8 from green algae to higher plants. Front. Plant Sci.7, 1698. doi: 10.3389/fpls.2016.01698
Fischer, B. B., Wiesendanger, M., Eggen, R. I. (2006). Growth condition-dependent sensitivity, photodamage and stress response of Chlamydomonas reinhardtii exposed to high light conditions. Plant Cell Physiol.47, 1135–1145. doi: 10.1093/pcp/pcj085
Folta, K., Pontin, M., Karlin-Neumann, G., Bottini, R., Spalding, E. (2003). Genomic and physiological studies demonstrate roles for auxin and gibberellin in the early phase of cryptochrome 1 action in blue light. Plant J.36, 203–214. doi: 10.1046/j.1365-313X.2003.01870.x
Foyer, C. H. (2018). Reactive oxygen species, oxidative signaling and the regulation of photosynthesis. Environ. Exp. Bot.154, 134–142. doi: 10.1016/j.envexpbot.2018.05.003
Franklin, K. A., Larner, V. S., Whitelam, G. C. (2004). The signal transducing photoreceptors of plants. Int. J. Dev. Biol.49, 653–664. doi: 10.1387/ijdb.051989kf
Fuchs, I., Philippar, K., Ljung, K., Sandberg, G., Hedrich, R. (2003). Blue light regulates an auxin-induced K+-channel gene in the maize coleoptile. Proc. Natl. Acad. Sci.100, 11795–11800. doi: 10.1073/pnas.2032704100
Gahrtz, M., Stolz, J., Sauer, N. (1994). A phloem-specific sucrose-H+ symporter from Plantago major L. supports the model of apoplastic phloem loading. Plant J.6, 697–706. doi: 10.1046/j.1365-313X.1994.6050697.x
Galvão, V. C., Fankhauser, C. (2015). Sensing the light environment in plants: photoreceptors and early signaling steps. Curr. Opin. Neurobiol.34, 46–53. doi: 10.1016/j.conb.2015.01.013
Ganesan, M., Lee, H. Y., Kim, J. I., Song, P. S. (2017). Development of transgenic crops based on photo-biotechnology. Plant Cell Environ.40, 2469–2486. doi: 10.1111/pce.12887
Gautier, H., Diakou-Verdin, V., Bénard, C., Reich, M., Buret, M., Bourgaud, F., et al. (2008). How does tomato quality (sugar, acid, and nutritional quality) vary with ripening stage, temperature, and irradiance. J. Agric. Food Chem.56, 1241–1250. doi: 10.1021/jf072196t
Gaxiola, R. A., Fink, G. R., Hirschi, K. D. (2002). Genetic manipulation of vacuolar proton pumps and transporters. Plant Physiol.129, 967–973. doi: 10.1104/pp.020009
Gay, A., Hurd, R. (1975). The influence of light on stomatal density in the tomato. New Phytol.75, 37–46. doi: 10.1111/j.1469-8137.1975.tb01368.x
Gelli, A., Blumwald, E. (1997). Hyperpolarization-activated Ca2+-permeable channels in the plasma membrane of tomato cells. J. Membr. Biol.155, 35–45. doi: 10.1007/s002329900156
Givnish, T. J., Montgomery, R. A., Goldstein, G. (2004). Adaptive radiation of photosynthetic physiology in the Hawaiian lobeliads: light regimes, static light responses, and whole-plant compensation points. Am. J. Bot.91, 228–246. doi: 10.3732/ajb.91.2.228
Goggs, R., Vaughan-Thomas, A., Clegg, P. D., Carter, S. D., Innes, J. F., Mobasheri, A., et al. (2005). Nutraceutical therapies for degenerative joint diseases: a critical review. Crit. Rev. Food Sci. Nutr.45, 145–164. doi: 10.1080/10408690590956341
Gross, J. (2012). Pigments in vegetables: chlorophylls and carotenoids. Springer Science & Business Media.
Guo, H., Duong, H., Ma, N., Lin, C. (1999). The Arabidopsis blue light receptor cryptochrome 2 is a nuclear protein regulated by a blue light-dependent post-transcriptional mechanism. Plant J.19, 279–287. doi: 10.1046/j.1365-313X.1999.00525.x
Hamilton, D. W., Hills, A., Köhler, B., Blatt, M. R. (2000). Ca2+ channels at the plasma membrane of stomatal guard cells are activated by hyperpolarization and abscisic acid. Proc. Natl. Acad. Sci.97, 4967–4972. doi: 10.1073/pnas.080068897
Hansen, U.-P., Kolbowski, J., Dau, H. (1987). Relationship between photosynthesis and plasmalemma transport. J. Exp. Bot.38, 1965–1981. doi: 10.1093/jxb/38.12.1965
Harada, A., Sakai, T., Okada, K. (2003). Phot1 and phot2 mediate blue light-induced transient increases in cytosolic Ca2+ differently in Arabidopsis leaves. Proc. Natl. Acad. Sci.100, 8583–8588. doi: 10.1073/pnas.1336802100
Hart, J. E., Sullivan, S., Hermanowicz, P., Petersen, J., Diaz-Ramos, L. A., Hoey, D. J., et al. (2019). Engineering the phototropin photocycle improves photoreceptor performance and plant biomass production. Proc. Natl. Acad. Sci.116, 12550–12557. doi: 10.1073/pnas.1902915116
Harz, H., Hegemann, P. (1991). Rhodopsin-regulated calcium currents in Chlamydomonas. Nature351, 489. doi: 10.1038/351489a0
Harz, H., Nonnengasser, C., Hegemann, P. (1992). The photoreceptor current of the green alga Chlamydomonas. Philos. Trans. R. Soc. Lond. B. Biol. Sci.338, 39–52. doi: 10.1098/rstb.1992.0127
Hayashi, M., Inoue, S.-I., Takahashi, K., Kinoshita, T. (2011). Immunohistochemical detection of blue light-induced phosphorylation of the plasma membrane H+-ATPase in stomatal guard cells. Plant Cell Physiol.52, 1238–1248. doi: 10.1093/pcp/pcr072
Hayashi, M., Inoue, S.-I., Ueno, Y., Kinoshita, T. (2017). A Raf-like protein kinase BHP mediates blue light-dependent stomatal opening. Sci. Rep.7, 45586. doi: 10.1038/srep45586
Haydon, M. J., Bell, L. J., Webb, A. A. (2011). Interactions between plant circadian clocks and solute transport. J. Exp. Bot.62, 2333–2348. doi: 10.1093/jxb/err040
Heijde, M., Ulm, R. (2012). UV-B photoreceptor-mediated signalling in plants. Trends Plant Sci.17, 230–237. doi: 10.1016/j.tplants.2012.01.007
Herdean, A., Teardo, E., Nilsson, A. K., Pfeil, B. E., Johansson, O. N., Ünnep, R., et al. (2016). A voltage-dependent chloride channel fine-tunes photosynthesis in plants. Nat. Commun.7, 11654. doi: 10.1038/ncomms11654
Hicks, K. A., Albertson, T. M., Wagner, D. R. (2001). EARLY FLOWERING3 encodes a novel protein that regulates circadian clock function and flowering in Arabidopsis. Plant Cell13, 1281–1292. doi: 10.1105/TPC.010070
Hills, A., Chen, Z.-H., Amtmann, A., Blatt, M. R., Lew, V. L. (2012). OnGuard, a computational platform for quantitative kinetic modeling of guard cell physiology. Plant Physiol.159, 1026–1042. doi: 10.1104/pp.112.197244
Hoang, Q. T. N., Han, Y.-J., Kim, J.-I. (2019). Plant phytochromes and their phosphorylation. Int. J. Mol. Sci.20, 3450. doi: 10.3390/ijms20143450
Hoffmann, A. M., Noga, G., Hunsche, M. (2016). Alternating high and low intensity of blue light affects PSII photochemistry and raises the contents of carotenoids and anthocyanins in pepper leaves. Plant Growth Regul.79, 275–285. doi: 10.1007/s10725-015-0132-0
Horrer, D., Flütsch, S., Pazmino, D., Matthews, J. S., Thalmann, M., Nigro, A., et al. (2016). Blue light induces a distinct starch degradation pathway in guard cells for stomatal opening. Curr. Biol.26, 362–370. doi: 10.1016/j.cub.2015.12.036
Horton, P., Ruban, A., Walters, R. (1996). Regulation of light harvesting in green plants. Annu. Rev. Plant Biol.47, 655–684. doi: 10.1146/annurev.arplant.47.1.655
Iida, A., Kazuoka, T., Torikai, S., Kikuchi, H., Oeda, K. (2000). A zinc finger protein RHL41 mediates the light acclimatization response in Arabidopsis. Plant J.24, 191–203. doi: 10.1046/j.1365-313x.2000.00864.x
Im, C.-S., Zhang, Z., Shrager, J., Chang, C.-W., Grossman, A. R. (2003). Analysis of light and CO2 regulation in Chlamydomonas reinhardtii using genome-wide approaches. Photosyn. Res.75, 111–125. doi: 10.1023/A:1022800630430
Inoue, S.-I., Kinoshita, T. (2017). Blue light regulation of stomatal opening and the plasma membrane H+-ATPase. Plant Physiol.174, 531–538. doi: 10.1104/pp.17.00166
Inoue, S.-I., Kinoshita, T., Matsumoto, M., Nakayama, K. I., Doi, M., Shimazaki, K.-I. (2008). Blue light-induced autophosphorylation of phototropin is a primary step for signaling. Proc. Natl. Acad. Sci.105, 5626–5631. doi: 10.1073/pnas.0709189105
Inoue, S.-I., Matsushita, T., Tomokiyo, Y., Matsumoto, M., Nakayama, K. I., Kinoshita, T., et al. (2011). Functional analyses of the activation loop of phototropin2 in Arabidopsis. Plant Physiol.156, 117–128. doi: 10.1104/pp.111.175943
Inoue, S.-I., Takemiya, A., Shimazaki, K.-I. (2010). Phototropin signaling and stomatal opening as a model case. Curr. Opin. Plant Biol.13, 587–593. doi: 10.1016/j.pbi.2010.09.002
Ito, S., Song, Y. H., Imaizumi, T. (2012). LOV domain-containing F-box proteins: light-dependent protein degradation modules in Arabidopsis. Mol. Plant5, 573–582. doi: 10.1093/mp/sss013
Jarillo, J., Ahmad, M., Cashmore, A. (1998). NPL1: a second member of the NPH serine/threonine kinase family of Arabidopsis. Plant Physiol.117, 719.
Jenkins, G. I. (2014a). Structure and function of the UV-B photoreceptor UVR8. Curr. Opin. Struct. Biol.29, 52–57. doi: 10.1016/j.sbi.2014.09.004
Jenkins, G. I. (2014b). The UV-B photoreceptor UVR8: from structure to physiology. Plant Cell26, 21–37. doi: 10.1105/tpc.113.119446
Jenkins, G. I. (2017). Photomorphogenic responses to ultraviolet-B light. Plant Cell Environ.40, 2544–2557. doi: 10.1111/pce.12934
Jiao, Y., Lau, O. S., Deng, X. W. (2007). Light-regulated transcriptional networks in higher plants. Nat. Rev. Genet.8, 217–230. doi: 10.1038/nrg2049
Johannes, E., Ermolayeva, E., Sanders, D. (1997). Red light-induced membrane potential transients in the moss Physcomitrella patens: ion channel interaction in phytochrome signalling. J. Exp. Bot.48, 599–608. doi: 10.1093/jxb/48.Special_Issue.599
Junge, W., Jackson, J. (1982). The development of electrochemical potential gradients across photosynthetic membranes. Photosyn. Energy Convers. Plants Bact.1, 589–646. doi: 10.1016/B978-0-12-294301-0.50022-1
Kagawa, T., Kasahara, M., Abe, T., Yoshida, S., Wada, M. (2004). Function analysis of phototropin2 using fern mutants deficient in blue light-induced chloroplast avoidance movement. Plant Cell Physiol.45, 416–426. doi: 10.1093/pcp/pch045
Kaiserli, E., Jenkins, G. I. (2007). UV-B promotes rapid nuclear translocation of the Arabidopsis UV-B–specific signaling component UVR8 and activates its function in the nucleus. Plant Cell19, 2662–2673. doi: 10.1105/tpc.107.053330
Kangasjärvi, S., Neukermans, J., Li, S., Aro, E.-M., Noctor, G. (2012). Photosynthesis, photorespiration, and light signalling in defence responses. J. Exp. Bot. 63, 1619–1636. doi: 10.1093/jxb/err402
Karpinski, S., Reynolds, H., Karpinska, B., Wingsle, G., Creissen, G., Mullineaux, P. (1999). Systemic signaling and acclimation in response to excess excitation energy in Arabidopsis. Science284, 654–657. doi: 10.1126/science.284.5414.654
Kawai, H., Kanegae, T., Christensen, S., Kiyosue, T., Sato, Y., Imaizumi, T., et al. (2003). Responses of ferns to red light are mediated by an unconventional photoreceptor. Nature421, 287–290. doi: 10.1038/nature01310
Kevei, E., Schafer, E., Nagy, F. (2007). Light-regulated nucleo-cytoplasmic partitioning of phytochromes. J. Exp. Bot.58, 3113–3124. doi: 10.1093/jxb/erm145
Kharshiing, E., Sinha, S. P. (2015). Plant productivity: can photoreceptors light the way. J. Plant Growth Regul.34, 206–214. doi: 10.1007/s00344-014-9454-9
Kianianmomeni, A., Hallmann, A. (2014). Algal photoreceptors: in vivo functions and potential applications. Planta239, 1–26. doi: 10.1007/s00425-013-1962-5
Kim, T.-H., Böhmer, M., Hu, H., Nishimura, N., Schroeder, J. I. (2010). Guard cell signal transduction network: advances in understanding abscisic acid, CO2, and Ca2+ signaling. Annu. Rev. Plant Biol.61, 561–591. doi: 10.1146/annurev-arplant-042809-112226
Kim, W.-Y., Fujiwara, S., Suh, S.-S., Kim, J., Kim, Y., Han, L., et al. (2007). ZEITLUPE is a circadian photoreceptor stabilized by GIGANTEA in blue light. Nature449, 356. doi: 10.1038/nature06132
Kimura, M., Yamamoto, Y. Y., Seki, M., Sakurai, T., Sato, M., Abe, T., et al. (2003). Identification of Arabidopsis genes regulated by high light–stress using cDNA microarray. Photochem. Photobiol.77, 226–233. doi: 10.1562/0031-8655(2003)0770226IOAGRB2.0.CO2
Kinoshita, T., Doi, M., Suetsugu, N., Kagawa, T., Wada, M., Shimazaki, K.-I. (2001). Phot1 and phot2 mediate blue light regulation of stomatal opening. Nature414, 656–660. doi: 10.1038/414656a
Kleine, T., Kindgren, P., Benedict, C., Hendrickson, L., Strand, Å. (2007). Genome-wide gene expression analysis reveals a critical role for CRYPTOCHROME1 in the response of Arabidopsis to high irradiance. Plant Physiol.144, 1391–1406. doi: 10.1104/pp.107.098293
Kleine, T., Lockhart, P., Batschauer, A. (2003). An Arabidopsis protein closely related to Synechocystis cryptochrome is targeted to organelles. Plant J.35, 93–103. doi: 10.1046/j.1365-313X.2003.01787.x
Komatsu, K., Suzuki, N., Kuwamura, M., Nishikawa, Y., Nakatani, M., Ohtawa, H., et al. (2013). Group A PP2Cs evolved in land plants as key regulators of intrinsic desiccation tolerance. Nat. Commun.4, 2219. doi: 10.1038/ncomms3219
Kong, S.-G., Okajima, K. (2016). Diverse photoreceptors and light responses in plants. J. Plant Res.129, 111–114. doi: 10.1007/s10265-016-0792-5
Koselski, M., Trebacz, K., Dziubinska, H., Krol, E. (2008). Light-and dark-induced action potentials in Physcomitrella patens. Plant Signal. Behav.3, 13–18. doi: 10.4161/psb.3.1.4884
Kroth, P. G., Wilhelm, C., Kottke, T. (2017). An update on aureochromes: phylogeny–mechanism–function. J. Plant Physiol.217, 20–26. doi: 10.1016/j.jplph.2017.06.010
Kubota, A., Kita, S., Ishizaki, K., Nishihama, R., Yamato, K. T., Kohchi, T. (2014). Co-option of a photoperiodic growth-phase transition system during land plant evolution. Nat. Commun.5, 3668. doi: 10.1038/ncomms4668
Kunz, H.-H., Gierth, M., Herdean, A., Satoh-Cruz, M., Kramer, D. M., Spetea, C., et al. (2014). Plastidial transporters KEA1,-2, and-3 are essential for chloroplast osmoregulation, integrity, and pH regulation in Arabidopsis. Proc. Natl. Acad. Sci.111, 7480–7485. doi: 10.1073/pnas.1323899111
Lariguet, P., Dunand, C. (2005). Plant photoreceptors: phylogenetic overview. J. Mol. Evol.61, 559–569. doi: 10.1007/s00239-004-0294-2
Lebaudy, A., Hosy, E., Simonneau, T., Sentenac, H., Thibaud, J. B., Dreyer, I. (2008). Heteromeric K+ channels in plants. Plant J.54, 1076–1082. doi: 10.1111/j.1365-313X.2008.03479.x
Lee, J., He, K., Stolc, V., Lee, H., Figueroa, P., Gao, Y., et al. (2007a). Analysis of transcription factor HY5 genomic binding sites revealed its hierarchical role in light regulation of development. Plant Cell19, 731–749. doi: 10.1105/tpc.106.047688
Lee, S.-H., Tewari, R. K., Hahn, E.-J., Paek, K.-Y. (2007b). Photon flux density and light quality induce changes in growth, stomatal development, photosynthesis and transpiration of Withania somnifera (L.) Dunal. plantlets. Plant Cell Tissue Organ Cult.90, 141–151. doi: 10.1007/s11240-006-9191-2
Leivar, P., Monte, E. (2014). PIFs: systems integrators in plant development. Plant Cell26, 56–78. doi: 10.1105/tpc.113.120857
Lewis, B. D., Karlin-Neumann, G., Davis, R. W., Spalding, E. P. (1997). Ca2+-activated anion channels and membrane depolarizations induced by blue light and cold in Arabidopsis seedlings. Plant Physiol.114, 1327–1334. doi: 10.1104/pp.114.4.1327
Li, F.-W., Melkonian, M., Rothfels, C. J., Villarreal, J. C., Stevenson, D. W., Graham, S. W., et al. (2015a). Phytochrome diversity in green plants and the origin of canonical plant phytochromes. Nat. Commun.6, 7852. doi: 10.1038/ncomms8852
Li, F.-W., Rothfels, C. J., Melkonian, M., Villarreal, J. C., Stevenson, D. W., Graham, S. W., et al. (2015b). The origin and evolution of phototropins. Front. Plant Sci.6, 637. doi: 10.3389/fpls.2015.00637
Li, H., Li, Y., Deng, H., Sun, X., Wang, A., Tang, X., et al. (2018). Tomato UV-B receptor SlUVR8 mediates plant acclimation to UV-B radiation and enhances fruit chloroplast development via regulating SlGLK2. Sci. Rep.8, 6097. doi: 10.1038/s41598-018-24309-y
Li, J., Li, G., Wang, H., Deng, X. W. (2011). Phytochrome signaling mechanisms. Am. Soc. Plant Biol.9, e0148. doi: 10.1199/tab.0148
Li, T., Heuvelink, E., Dueck, T., Janse, J., Gort, G., Marcelis, L. (2014). Enhancement of crop photosynthesis by diffuse light: quantifying the contributing factors. Ann. Bot.114, 145–156. doi: 10.1093/aob/mcu071
Li, W., Zhang, D., Zhu, G., Mi, X., Guo, W. (2019). Combining genome-wide and transcriptome-wide analyses reveal the evolutionary conservation and functional diversity of aquaporins in cotton. BMC Genomics20, 538. doi: 10.1186/s12864-019-5928-2
Lin, C. (1996). CRY2, a second member of the Arabidopsis cryptochrome gene family. Plant Physiol.110, 1047. doi: 10.1104/pp.110.3.1047
Lind, C., Dreyer, I., López-Sanjurjo, E. J., Von Meyer, K., Ishizaki, K., Kohchi, T., et al. (2015). Stomatal guard cells co-opted an ancient ABA-dependent desiccation survival system to regulate stomatal closure. Curr. Biol.25, 928–935. doi: 10.1016/j.cub.2015.01.067
Liu, C. C., Chi, C., Jin, L. J., Zhu, J., Yu, J. Q., Zhou, Y. H. (2018). The bZip transcription factor HY5 mediates CRY1a-induced anthocyanin biosynthesis in tomato. Plant Cell Environ.41, 1762–1775. doi: 10.1111/pce.13171
López-Juez, E., Bowyer, J. R., Sakai, T. (2007). Distinct leaf developmental and gene expression responses to light quantity depend on blue-photoreceptor or plastid-derived signals, and can occur in the absence of phototropins. Planta227, 113–123. doi: 10.1007/s00425-007-0599-7
Ma, L., Li, J., Qu, L., Hager, J., Chen, Z., Zhao, H., et al. (2001). Light control of Arabidopsis development entails coordinated regulation of genome expression and cellular pathways. Plant Cell13, 2589–2607. doi: 10.1105/tpc.010229
Marshall, J., Corzo, A., Leigh, R. A., Sanders, D. (1994). Membrane potential-dependent calcium transport in right-side-out plasma membrane vesicles from Zea mays L. roots. Plant J.5, 683–694. doi: 10.1111/j.1365-313X.1994.00683.x
Marten, H., Konrad, K. R., Dietrich, P., Roelfsema, M. R. G., Hedrich, R. (2007). Ca2+-dependent and-independent abscisic acid activation of plasma membrane anion channels in guard cells of Nicotiana tabacum. Plant Physiol.143, 28–37. doi: 10.1104/pp.106.092643
Marten, I., Deeken, R., Hedrich, R., Roelfsema, M. (2010). Light-induced modification of plant plasma membrane ion transport. Plant Biol.12, 64–79. doi: 10.1111/j.1438-8677.2010.00384.x
Martín, G., Leivar, P., Ludevid, D., Tepperman, J. M., Quail, P. H., Monte, E. (2016). Phytochrome and retrograde signalling pathways converge to antagonistically regulate a light-induced transcriptional network. Nat. Commun.7, 11431. doi: 10.1038/ncomms11431
Más, P., Kim, W.-Y., Somers, D. E., Kay, S. A. (2003). Targeted degradation of TOC1 by ZTL modulates circadian function in Arabidopsis thaliana. Nature426, 567–570. doi: 10.1038/nature02163
Matos, F. S., Wolfgramm, R., Gonçalves, F. V., Cavatte, P. C., Ventrella, M. C., Damatta, F. M. (2009). Phenotypic plasticity in response to light in the coffee tree. Environ. Exp. Bot.67, 421–427. doi: 10.1016/j.envexpbot.2009.06.018
Matsuoka, D., Tokutomi, S. (2005). Blue light-regulated molecular switch of Ser/Thr kinase in phototropin. Proc. Natl. Acad. Sci. U. S. A.102, 13337–13342. doi: 10.1073/pnas.0506402102
Mawphlang, O. I., Kharshiing, E. V. (2017). Photoreceptor mediated plant growth responses: implications for photoreceptor engineering toward improved performance in crops. Front. Plant Sci.8, 1181. doi: 10.3389/fpls.2017.01181
Miedema, H., Bothwell, J. H., Brownlee, C., Davies, J. M. (2001). Calcium uptake by plant cells–channels and pumps acting in concert. Trends Plant Sci.6, 514–519. doi: 10.1016/S1360-1385(01)02124-0
Mishra, S., Khurana, J. P. (2017). Emerging roles and new paradigms in signaling mechanisms of plant cryptochromes. Crit. Rev. Plant Sci.36, 89–115. doi: 10.1080/07352689.2017.1348725
Møller, S. G., Kunkel, T., Chua, N.-H. (2001). A plastidic ABC protein involved in intercompartmental communication of light signaling. Genes Dev.15, 90–103. doi: 10.1101/gad.850101
Morrison, J. C., Noble, A. C. (1990). The effects of leaf and cluster shading on the composition of Cabernet Sauvignon grapes and on fruit and wine sensory properties. Am. J. Enol. Vitic.41, 193–200.
Mullineaux, P., Karpinski, S. (2002). Signal transduction in response to excess light: getting out of the chloroplast. Curr. Opin. Plant Biol.5, 43–48. doi: 10.1016/S1369-5266(01)00226-6
Muñiz, J. J., Pottosin, I. I., Sandoval, L. (1995). Patch-clamp study of vascular plant chloroplasts: ion channels and photocurrents. J. Bioenerg. Biomembr.27, 249–258. doi: 10.1007/BF02110040
Murchie, E., Hubbart, S., Peng, S., Horton, P. (2005). Acclimation of photosynthesis to high irradiance in rice: gene expression and interactions with leaf development. J. Exp. Bot.56, 449–460. doi: 10.1093/jxb/eri100
Nagatani, A. (2004). Light-regulated nuclear localization of phytochromes. Curr. Opin. Plant Biol.7, 708–711. doi: 10.1016/j.pbi.2004.09.010
Nagel, G., Ollig, D., Fuhrmann, M., Kateriya, S., Musti, A. M., Bamberg, E., et al. (2002). Channelrhodopsin-1: a light-gated proton channel in green algae. Science296, 2395–2398. doi: 10.1126/science.1072068
Nagel, G., Szellas, T., Huhn, W., Kateriya, S., Adeishvili, N., Berthold, P., et al. (2003). Channelrhodopsin-2, a directly light-gated cation-selective membrane channel. Proc. Natl. Acad. Sci.100, 13940–13945. doi: 10.1073/pnas.1936192100
Neuner, G., Braun, V., Buchner, O., Taschler, D. (1999). Leaf rosette closure in the alpine rock species Saxifraga paniculata Mill.: significance for survival of drought and heat under high irradiation. Plant Cell Environ.22, 1539–1548. doi: 10.1046/j.1365-3040.1999.00508.x
Newman, I. A., Briggs, W. R. (1972). Phytochrome-mediated electric potential changes in oat seedlings. Plant Physiol.50, 687–693. doi: 10.1104/pp.50.6.687
Noh, B., Spalding, E. P. (1998). Anion channels and the stimulation of anthocyanin accumulation by blue light in Arabidopsis seedlings. Plant Physiol.116, 503–509. doi: 10.1104/pp.116.2.503
Nozue, K., Kanegae, T., Imaizumi, T., Fukuda, S., Okamoto, H., Yeh, K.-C., et al. (1998). A phytochrome from the fern Adiantum with features of the putative photoreceptor NPH1. Proc. Natl. Acad. Sci.95, 15826–15830. doi: 10.1073/pnas.95.26.15826
O’carrigan, A., Babla, M., Wang, F., Liu, X., Mak, M., Thomas, R., et al. (2014). Analysis of gas exchange, stomatal behaviour and micronutrients uncovers dynamic response and adaptation of tomato plants to monochromatic light treatments. Plant Physiol. Biochem.82, 105–115. doi: 10.1016/j.plaphy.2014.05.012
Palmgren, M. G. (2001). Plant plasma membrane H+-ATPases: powerhouses for nutrient uptake. Annu. Rev. Plant Biol.52, 817–845. doi: 10.1146/annurev.arplant.52.1.817
Parks, B. M., Cho, M. H., Spalding, E. P. (1998). Two genetically separable phases of growth inhibition induced by blue light in Arabidopsis seedlings. Plant Physiol.118, 609–615. doi: 10.1104/pp.118.2.609
Petroutsos, D. (2017). “Chlamydomonas photoreceptors: cellular functions and impact on physiology,” in Chlamydomonas: biotechnology and biomedicine (Netherlands: Springer), 1–19. doi: 10.1007/978-3-319-66360-9_1
Pfeil, B. E., Schoefs, B., Spetea, C. (2014). Function and evolution of channels and transporters in photosynthetic membranes. Cell. Mol. Life Sci.71, 979–998. doi: 10.1007/s00018-013-1412-3
Pilot, G., Pratelli, R., Gaymard, F., Meyer, Y., Sentenac, H. (2003). Five-group distribution of the Shaker-like K+ channel family in higher plants. J. Mol. Evol.56, 418–434. doi: 10.1007/s00239-002-2413-2
Qian, C., Mao, W., Liu, Y., Ren, H., Lau, O. S., Ouyang, X., et al. (2016). Dual-source nuclear monomers of UV-B light receptor direct photomorphogenesis in Arabidopsis. Mol. Plant9, 1671–1674. doi: 10.1016/j.molp.2016.10.005
Quail, P. H. (2002). Photosensory perception and signalling in plant cells: new paradigms. Curr. Opin. Cell Biol.14, 180–188. doi: 10.1016/S0955-0674(02)00309-5
Racusen, R., Galston, A. (1983). “Developmental significance of light-mediated electrical responses in plant tissue,” in Photomorphogenesis (Berlin, Heidelberg: Springer), 687–703. doi: 10.1007/978-3-642-68918-5_26
Rensing, S. A., Sheerin, D. J., Hiltbrunner, A. (2016). Phytochromes: more than meets the eye. Trends Plant Sci.21, 543–546. doi: 10.1016/j.tplants.2016.05.009
Riesmeier, J. W., Willmitzer, L., Frommer, W. B. (1994). Evidence for an essential role of the sucrose transporter in phloem loading and assimilate partitioning. EMBO J.13, 1. doi: 10.1002/j.1460-2075.1994.tb06229.x
Rizzini, L., Favory, J.-J., Cloix, C., Faggionato, D., O’hara, A., Kaiserli, E., et al. (2011). Perception of UV-B by the Arabidopsis UVR8 protein. Science332, 103–106. doi: 10.1126/science.1200660
Roberts, S. K. (2006). Plasma membrane anion channels in higher plants and their putative functions in roots. New Phytol.169, 647–666. doi: 10.1111/j.1469-8137.2006.01639.x
Roelfsema, M. R. G., Hedrich, R. (2005). In the light of stomatal opening: new insights into ‘the Watergate’. New Phytol.167, 665–691. doi: 10.1111/j.1469-8137.2005.01460.x
Rösler, J., Klein, I., Zeidler, M. (2007). Arabidopsisfhl/fhy1 double mutant reveals a distinct cytoplasmic action of phytochrome A. Proc. Natl. Acad. Sci.104, 10737–10742. doi: 10.1073/pnas.0703855104
Rossel, J. B., Wilson, I. W., Pogson, B. J. (2002). Global changes in gene expression in response to high light in Arabidopsis. Plant Physiol.130, 1109–1120. doi: 10.1104/pp.005595
Ruszala, E. M., Beerling, D. J., Franks, P. J., Chater, C., Casson, S. A., Gray, J. E., et al. (2011). Land plants acquired active stomatal control early in their evolutionary history. Curr. Biol.21, 1030–1035. doi: 10.1016/j.cub.2011.04.044
Sakamoto, K., Briggs, W. R. (2002). Cellular and subcellular localization of phototropin 1. Plant Cell14, 1723–1735. doi: 10.1105/tpc.003293
Santelia, D., Lunn, J. E. (2017). Transitory starch metabolism in guard cells: unique features for a unique function. Plant Physiol.174, 539–549. doi: 10.1104/pp.17.00211
Schneider, F., Grimm, C., Hegemann, P. (2015). Biophysics of channelrhodopsin. Annu. Rev. Biophys.44, 167–186. doi: 10.1146/annurev-biophys-060414-034014
Schönknecht, G., Bauer, C., Simonis, W. (1998). Light-dependent signal transduction and transient changes in cytosolic Ca2+ in a unicellular green alga. J. Exp. Bot.49, 1–11. doi: 10.1093/jxb/49.318.1
Schroeder, J. I., Delhaize, E., Frommer, W. B., Guerinot, M. L., Harrison, M. J., Herrera-Estrella, L., et al. (2013). Using membrane transporters to improve crops for sustainable food production. Nature497, 60–66. doi: 10.1038/nature11909
Serrano, E. E., Zeiger, E., Hagiwara, S. (1988). Red light stimulates an electrogenic proton pump in Vicia guard cell protoplasts. Proc. Natl. Acad. Sci.85, 436–440. doi: 10.1073/pnas.85.2.436
Shabala, S., Newman, I. (1999). Light-induced changes in hydrogen, calcium, potassium, and chloride ion fluxes and concentrations from the mesophyll and epidermal tissues of bean leaves. Understanding the ionic basis of light-induced bioelectrogenesis. Plant Physiol.119, 1115–1124. doi: 10.1104/pp.119.3.1115
Shabala, S., Wilson, S. (2001). Fluctuations in light intensity modulate ion fluxes from grape berry mesocarp: direct evidence from microelectrode ion flux estimations. Aust. J. Grape and Wine Res. 7, 137–143. doi: 10.1111/j.1755-0238.2001.tb00201.x
Sharrock, R. A., Quail, P. H. (1989). Novel phytochrome sequences in Arabidopsis thaliana: structure, evolution, and differential expression of a plant regulatory photoreceptor family. Genes Dev.3, 1745–1757. doi: 10.1101/gad.3.11.1745
Shimazaki, K.-I., Doi, M., Assmann, S. M., Kinoshita, T. (2007). Light regulation of stomatal movement. Annu. Rev. Plant Biol.58, 219–247. doi: 10.1146/annurev.arplant.57.032905.105434
Somers, D. E., Devlin, P. F., Kay, S. A. (1998). Phytochromes and cryptochromes in the entrainment of the Arabidopsis circadian clock. Science282, 1488–1490. doi: 10.1126/science.282.5393.1488
Soriano, G., Cloix, C., Heilmann, M., Núñez-Olivera, E., Martínez-Abaigar, J., Jenkins, G. I. (2018). Evolutionary conservation of structure and function of the UVR 8 photoreceptor from the liverwort Marchantia polymorpha and the moss Physcomitrella patens. New Phytol.217, 151–162. doi: 10.1111/nph.14767
Spalding, E. (2000). Ion channels and the transduction of light signals. Plant Cell Environ.23, 665–674. doi: 10.1046/j.1365-3040.2000.00594.x
Spalding, E. P., Slayman, C. L., Goldsmith, M. H. M., Gradmann, D., Bertl, A. (1992). Ion channels in Arabidopsis plasma membrane transport characteristics and involvement in light-induced voltage changes. Plant Physiol.99, 96–102. doi: 10.1104/pp.99.1.96
Spetea, C., Lundin, B. (2012). Evidence for nucleotide-dependent processes in the thylakoid lumen of plant chloroplasts–an update. FEBS Letters586, 2946–2954. doi: 10.1016/j.febslet.2012.07.005
Srivastava, A. C., Ganesan, S., Ismail, I. O., Ayre, B. G. (2008). Functional characterization of the Arabidopsis AtSUC2 sucrose/H+ symporter by tissue-specific complementation reveals an essential role in phloem loading but not in long-distance transport. Plant Physiol.148, 200–211. doi: 10.1104/pp.108.124776
Stadler, R., Büttner, M., Ache, P., Hedrich, R., Ivashikina, N., Melzer, M., et al. (2003). Diurnal and light-regulated expression of AtSTP1 in guard cells of Arabidopsis. Plant Physiol.133, 528–537. doi: 10.1104/pp.103.024240
Staiger, D. (2008). Light and plant development. Annual Plant Reviews, Volume 30. Ann. Bot.101, 480–481. doi: 10.1093/aob/mcm309
Steinger, T., Roy, B., Stanton, M. (2003). Evolution in stressful environments II: adaptive value and costs of plasticity in response to low light in Sinapis arvensis. J. Evol. Biol.16, 313–323. doi: 10.1046/j.1420-9101.2003.00518.x
Stoelzle, S., Kagawa, T., Wada, M., Hedrich, R., Dietrich, P. (2003). Blue light activates calcium-permeable channels in Arabidopsis mesophyll cells via the phototropin signaling pathway. Proc. Natl. Acad. Sci.100, 1456–1461. doi: 10.1073/pnas.0333408100
Suetsugu, N., Higa, T., Wada, M. (2017). Ferns, mosses and liverworts as model systems for light-mediated chloroplast movements. Plant Cell Environ.40, 2447–2456. doi: 10.1111/pce.12867
Suetsugu, N., Wada, M. (2012). Evolution of three LOV blue light receptor families in green plants and photosynthetic stramenopiles: phototropin, ZTL/FKF1/LKP2 and aureochrome. Plant Cell Physiol.54, 8–23. doi: 10.1093/pcp/pcs165
Suh, S., Moran, N., Lee, Y. (2000). Blue light activates potassium-efflux channels in flexor cells from Samanea saman motor organs via two mechanisms. Plant Physiol.123, 833–844. doi: 10.1104/pp.123.3.833
Sze, H., Li, X., Palmgren, M. G. (1999). Energization of plant cell membranes by H+-pumping ATPases: regulation and biosynthesis. Plant Cell11, 677–689. doi: 10.1105/tpc.11.4.677
Sze, H., Schumacher, K., Müller, M. L., Padmanaban, S., Taiz, L. (2002). A simple nomenclature for a complex proton pump: VHA genes encode the vacuolar H+-ATPase. Trends Plant Sci.7, 157–161. doi: 10.1016/S1360-1385(02)02240-9
Takahashi, S., Badger, M. R. (2011). Photoprotection in plants: a new light on photosystem II damage. Trends Plant Sci.16, 53–60. doi: 10.1016/j.tplants.2010.10.001
Takemiya, A., Kinoshita, T., Asanuma, M., Shimazaki, K.-I. (2006). Protein phosphatase 1 positively regulates stomatal opening in response to blue light in Vicia faba. Proc. Natl. Acad. Sci.103, 13549–13554. doi: 10.1073/pnas.0602503103
Takemiya, A., Shimazaki, K.-I. (2016). Arabidopsis phot1 and phot2 phosphorylate BLUS1 kinase with different efficiencies in stomatal opening. J. Plant Res.129, 167–174. doi: 10.1007/s10265-015-0780-1
Takemiya, A., Sugiyama, N., Fujimoto, H., Tsutsumi, T., Yamauchi, S., Hiyama, A., et al. (2013). Phosphorylation of BLUS1 kinase by phototropins is a primary step in stomatal opening. Nat. Commun.4, 2094. doi: 10.1038/ncomms3094
Takemiya, A., Yamauchi, S., Yano, T., Ariyoshi, C., Shimazaki, K.-I. (2012). Identification of a regulatory subunit of protein phosphatase 1 which mediates blue light signaling for stomatal opening. Plant Cell Physiol.54, 24–35. doi: 10.1093/pcp/pcs073
Talbott, L. D., Zeiger, E. (1993). Sugar and organic acid accumulation in guard cells of Vicia faba in response to red and blue light. Plant Physiol.102, 1163–1169. doi: 10.1104/pp.102.4.1163
Taylor, A., Massiah, A. J., Thomas, B. (2010). Conservation of Arabidopsis thaliana photoperiodic flowering time genes in onion (Allium cepa L.). Plant Cell Physiol.51, 1638–1647. doi: 10.1093/pcp/pcq120
Tepperman, J. M., Hudson, M. E., Khanna, R., Zhu, T., Chang, S. H., Wang, X., et al. (2004). Expression profiling of phyB mutant demonstrates substantial contribution of other phytochromes to red-light-regulated gene expression during seedling de-etiolation. Plant J.38, 725–739. doi: 10.1111/j.1365-313X.2004.02084.x
Tepperman, J. M., Hwang, Y. S., Quail, P. H. (2006). phyA dominates in transduction of red-light signals to rapidly responding genes at the initiation of Arabidopsis seedling de-etiolation. Plant J.48, 728–742. doi: 10.1111/j.1365-313X.2006.02914.x
Tepperman, J. M., Zhu, T., Chang, H.-S., Wang, X., Quail, P. H. (2001). Multiple transcription-factor genes are early targets of phytochrome A signaling. Proc. Natl. Acad. Sci.98, 9437–9442. doi: 10.1073/pnas.161300998
Tilbrook, K., Dubois, M., Crocco, C. D., Yin, R., Chappuis, R., Allorent, G., et al. (2016). UV-B perception and acclimation in Chlamydomonas reinhardtii. Plant Celltpc. 00287, 02015. doi: 10.1105/tpc.15.00287
Tossi, V., Lamattina, L., Jenkins, G. I., Cassia, R. O. (2014). Ultraviolet-B-induced stomatal closure in Arabidopsis is regulated by the UV RESISTANCE LOCUS8 photoreceptor in a nitric oxide-dependent mechanism. Plant Physiol.164, 2220–2230. doi: 10.1104/pp.113.231753
Tossi, V. E., Regalado, J. J., Iannicelli, J., Burrieza, H. P., Escandon, A. S., Pitta-Alvarez, S. I. (2019). Beyond Arabidopsis: differential UV-B response mediated by UVR8 in diverse species. Front. Plant Sci.10, 780. doi: 10.3389/fpls.2019.00780
Vandenbussche, F., Tilbrook, K., Fierro, A. C., Marchal, K., Poelman, D., Van Der Straeten, D., et al. (2014). Photoreceptor-mediated bending towards UV-B in Arabidopsis. Mol. Plant7, 1041–1052. doi: 10.1093/mp/ssu039
Véry, A.-A., Davies, J. M. (2000). Hyperpolarization-activated calcium channels at the tip of Arabidopsis root hairs. Proc. Natl. Acad. Sci.97, 9801–9806. doi: 10.1073/pnas.160250397
Voelker, C., Schmidt, D., Mueller-Roeber, B., Czempinski, K. (2006). Members of the Arabidopsis AtTPK/KCO family form homomeric vacuolar channels in planta. Plant J.48, 296–306. doi: 10.1111/j.1365-313X.2006.02868.x
Vredenberg, W., Tonk, W. (1975). On the steady-state electrical potential difference across the thylakoid membranes of chloroplasts in illuminated plant cells. (BBA)-Bioenerg.387, 580–587. doi: 10.1016/0005-2728(75)90095-X
Walters, R. G. (2005). Towards an understanding of photosynthetic acclimation. J. Exp. Bot.56, 435–447. doi: 10.1093/jxb/eri060
Walters, R. G., Shephard, F., Rogers, J. J., Rolfe, S. A., Horton, P. (2003). Identification of mutants of Arabidopsis defective in acclimation of photosynthesis to the light environment. Plant Physiol.131, 472–481. doi: 10.1104/pp.015479
Wang, Y., Blatt, M. R., Chen, Z. H. (2018). Ion transport at the plant plasma membrane. eLS, 1–16. doi: 10.1002/9780470015902.a0001307.pub3
Wang, Y., Folta, K. M. (2013). Contributions of green light to plant growth and development. Am. J. Bot.100, 70–78. doi: 10.3732/ajb.1200354
Wang, Y., Tang, R. J., Yang, X., Zheng, X., Shao, Q., Tang, Q. L., et al. (2019). Golgi-localized cation/proton exchangers regulate ionic homeostasis and skotomorphogenesis in Arabidopsis. Plant Cell Environ.42, 673–687. doi: 10.1111/pce.13452
Weise, A., Lalonde, S., Kühn, C., Frommer, W. B., Ward, J. M. (2008). Introns control expression of sucrose transporter LeSUT1 in trichomes, companion cells and in guard cells. Plant Mol. Biol.68, 251–262. doi: 10.1007/s11103-008-9366-9
White, P. J. (1994). Characterization of a voltage-dependent cation-channel from the plasma membrane of rye (Secale cereale L.) roots in planar lipid bilayers. Planta193, 186–193. doi: 10.1007/BF00192529
Wilkens, R. T., Spoerke, J. M., Stamp, N. E. (1996). Differential responses of growth and two soluble phenolics of tomato to resource availability. Ecology77, 247–258. doi: 10.2307/2265674
Wu, G., Spalding, E. P. (2007). Separate functions for nuclear and cytoplasmic cryptochrome 1 during photomorphogenesis of Arabidopsis seedlings. Proc. Natl. Acad. Sci.104, 18813–18818. doi: 10.1073/pnas.0705082104
Xu, P., Zawora, C., Li, Y., Wu, J., Liu, L., Liu, Z., et al. (2018). Transcriptome sequencing reveals role of light in promoting anthocyanin accumulation of strawberry fruit. Plant Growth Regul.86, 121–132. doi: 10.1007/s10725-018-0415-3
Xu, X., Paik, I., Zhu, L., Huq, E. (2015). Illuminating progress in phytochrome-mediated light signaling pathways. Trends Plant Sci.20, 641–650. doi: 10.1016/j.tplants.2015.06.010
Yamauchi, S., Takemiya, A., Sakamoto, T., Kurata, T., Tsutsumi, T., Kinoshita, T., et al. (2016). The plasma membrane H+-ATPase AHA1 plays a major role in stomatal opening in response to blue light. Plant Physiol.171, 2731–2743. doi: 10.1104/pp.16.01581
Yang, B., Tang, J., Yu, Z., Khare, T., Srivastav, A., Datir, S., et al. (2019). Light stress responses and prospects for engineering light stress tolerance in crop plants. J. Plant Growth Regul. doi: 10.1007/s00344-019-09951-8
Yang, H.-Q., Wu, Y.-J., Tang, R.-H., Liu, D., Liu, Y., Cashmore, A. R. (2000). The C termini of Arabidopsis cryptochromes mediate a constitutive light response. Cell103, 815–827. doi: 10.1016/S0092-8674(00)00184-7
Yin, L., Lundin, B., Bertrand, M., Nurmi, M., Solymosi, K., Kangasjärvi, S., et al. (2010). Role of thylakoid ATP/ADP carrier in photoinhibition and photoprotection of photosystem II in Arabidopsis. Plant Physiol.153, 666–677. doi: 10.1104/pp.110.155804
Yin, R., Skvortsova, M. Y., Loubéry, S., Ulm, R. (2016). COP1 is required for UV-B–induced nuclear accumulation of the UVR8 photoreceptor. Proc. Natl. Acad. Sci.113, E4415–E4422. doi: 10.1073/pnas.1607074113
Yin, R., Ulm, R. (2017). How plants cope with UV-B: from perception to response. Curr. Opin. Plant Biol.37, 42–48. doi: 10.1016/j.pbi.2017.03.013
Zhao, C., Haigh, A. M., Holford, P., Chen, Z.-H. (2018a). Roles of chloroplast retrograde signals and ion transport in plant drought tolerance. Int. J. Mol. Sci.19, 963. doi: 10.3390/ijms19040963
Zhao, C., Wang, Y., Chan, K. X., Marchant, B., Franks, P. J., Randall, D., et al. (2019). Evolution of chloroplast retrograde signaling facilitates green plant adaptation to land. Proc. Natl. Acad. Sci. U. S. A. 116, 5015–5020. doi: 10.1073/pnas.1812092116
Zhao, X., Zhao, Q., Xu, C., Wang, J., Zhu, J., Shang, B., et al. (2018b). Phot2-regulated relocation of NPH3 mediates phototropic response to high-intensity blue light in Arabidopsis thaliana. J. Integr. Plant Biol.60, 562–577. doi: 10.1111/jipb.12639
Keywords: light, photoreceptors, membrane transporters, membrane potential, ion flux, crop nutrition
Citation: Babla M, Cai S, Chen G, Tissue DT, Cazzonelli CI and Chen Z-H (2019) Molecular Evolution and Interaction of Membrane Transport and Photoreception in Plants. Front. Genet. 10:956. doi: 10.3389/fgene.2019.00956
Received: 03 August 2018; Accepted: 06 September 2019;
Published: 11 October 2019.
Edited by:
Xusheng Wang, University of North Dakota, United StatesReviewed by:
Shaobai Huang, University of Western Australia, AustraliaBo Ouyang, Huazhong Agricultural University, China
Copyright © 2019 Babla, Cai, Chen, Tissue, Cazzonelli and Chen. This is an open-access article distributed under the terms of the Creative Commons Attribution License (CC BY). The use, distribution or reproduction in other forums is permitted, provided the original author(s) and the copyright owner(s) are credited and that the original publication in this journal is cited, in accordance with accepted academic practice. No use, distribution or reproduction is permitted which does not comply with these terms.
*Correspondence: Zhong-Hua Chen, Wi5DaGVuQHdlc3Rlcm5zeWRuZXkuZWR1LmF1