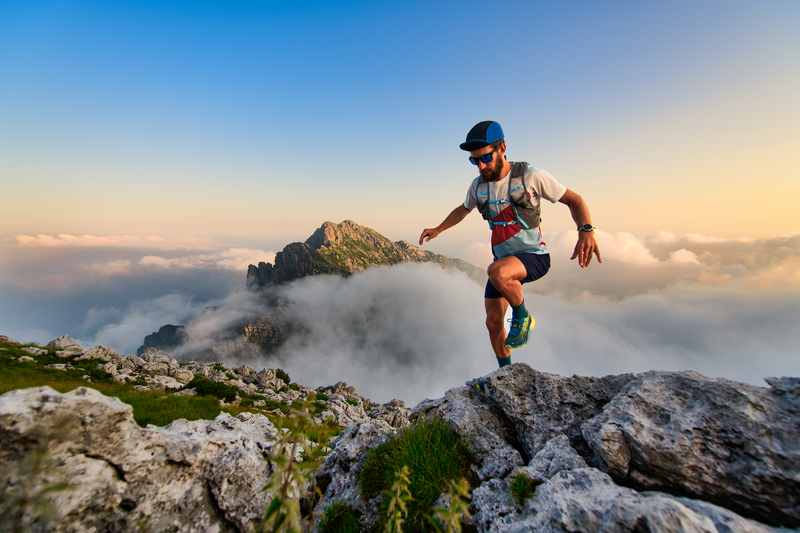
94% of researchers rate our articles as excellent or good
Learn more about the work of our research integrity team to safeguard the quality of each article we publish.
Find out more
ORIGINAL RESEARCH article
Front. Genet. , 13 September 2019
Sec. Evolutionary and Population Genetics
Volume 10 - 2019 | https://doi.org/10.3389/fgene.2019.00840
Bivalves exhibit an astonishing diversity of sexual systems, with genetic and environmental determinants of sex, and possibly the only example of mitochondrial genes influencing sex determination pathways in animals. In contrast to all other animal species in which strict maternal inheritance (SMI) of mitochondria is the rule, bivalves possess a system known as doubly uniparental inheritance (DUI) of mitochondria in which maternal and paternal mitochondria (and their corresponding female-transmitted or F mtDNA and male-transmitted or M mtDNA genomes) are transmitted within a species. Species with DUI also possess sex-associated mtDNA-encoded proteins (in addition to the typical set of 13), which have been hypothesized to play a role in sex determination. In this study, we analyzed the sex-biased transcriptome in gonads of two closely-related freshwater mussel species with different reproductive and mitochondrial transmission modes: the gonochoric, DUI species, Utterbackia peninsularis, and the hermaphroditic, SMI species, Utterbackia imbecillis. Through comparative analysis with other DUI and non-DUI bivalve transcriptomes already available, we identify common male and female-specific genes, as well as SMI and DUI-related genes, that are probably involved in sex determination and mitochondrial inheritance in this animal group. Our results contribute to the understanding of what could be the first animal sex determination system involving the mitochondrial genome.
Only one putative sex determination system involving mitochondria and their genome (mtDNA) has been reported in animals (Breton et al., 2011; Breton et al., 2018). It implicates the unorthodox system of doubly uniparental inheritance (DUI) of mitochondria in bivalve molluscs, which is also the only known exception to strict maternal inheritance (SMI) of mitochondria in animals (Birky, 2001; Breton and Stewart, 2015). Over 100 DUI species have been reported to date (Gusman et al., 2016). In these taxa, both maternal and paternal mitochondria (and their corresponding female-transmitted or F mtDNA and male-transmitted or M mtDNA) are transmitted uniparentally through eggs and sperm, respectively (see Breton et al., 2007; Passamonti and Ghiselli, 2009; Zouros, 2013 for reviews). In all DUI species, the F mtDNA is found in female gonads and in the somatic tissues of both sexes, whereas the M mtDNA is found in sperm and is sometimes detected in small quantities in male soma (see Breton et al., 2017b for a review). The M and F mtDNAs can be extremely divergent, with up to 40% intraspecific DNA divergence (Doucet-Beaupré et al., 2010; Guerra et al., 2017). The mechanism(s) by which such molecular divergence of mtDNAs is tolerated within a species are of considerable interest considering that heteroplasmy, i.e. the presence of two or more different mtDNAs within an organism, can cause severe disease or death in humans (Picard et al., 2016; Wallace, 2016).
Species with DUI also possess sex-associated mtDNA-encoded proteins (in addition to the typical set of 13) that are not apparently homologous to any other known genes (i.e. they are mitochondrial ORFans) (Breton et al., 2009; Breton et al., 2011; Milani et al., 2013a). Specifically, the F mtDNA contains the F-orf gene and the M mtDNA contains the M-orf gene, both of which are relatively conserved across species within a family (Breton et al., 2009; Breton et al., 2011; Milani et al., 2013a; Mitchell et al., 2016). In freshwater mussels (Unionida), gonochorism is perfectly correlated with the presence of DUI and these novel sex-specific proteins, whereas hermaphroditic species lack the M mtDNA (i.e. they possess SMI of mtDNA) and have macromutations in the region of their mtDNA genome that is homologous to the F-orf gene of the F mtDNA in their gonochoric relatives (Breton et al., 2011; Breton et al., 2014). In keeping with the transition from gonochorism to hermaphroditism, the mitochondrial genome present in these species is referred to as an H mtDNA and the gene of interest is known as an H-orf (Breton et al., 2011). These mtORFans have been hypothesized to be part of the sex determination system (Breton et al., 2011), and if true, this would make DUI the first animal sex determination system involving the mtDNA (heteromorphic sex chromosomes are absent in bivalves). However, we are still far from fully understanding the underlying link between DUI and sex determination.
A first transcriptomic study of mature male and female gonads of the DUI clam Ruditapes philippinarum proposed a relationship between sex, mitochondrial inheritance, and ubiquitination (Ghiselli et al., 2012). Ubiquitination is well known for being involved in the elimination of paternal mitochondria in mammals (Sutovsky et al., 1999). It is also known to play a role in sex determination in Caenorhabditis elegans and Drosophila (Ghiselli et al., 2012). Ghiselli et al. (2012) found a relationship between sex and differential expression of several ubiquitination genes in R. philippinarum, making them likely candidate genes for the regulation of sex‐specific aspects of the DUI system. Following this work, Milani et al. (2013a) focused on three of these genes that showed the most significant transcriptional bias and the highest levels of transcription (baculoviral IAP repeat‐containing 4 [birc]; proteasome subunit alpha 6 [psa]; AN1 zinc finger ubiquitin‐like domain [anubl1]). They hypothesized that these genes could play a role in sex determination and be responsible for the maintenance or degradation of sperm mitochondria during embryo development in DUI species. In addition, Capt et al. (2018) hypothesized that if a modification of the ubiquitination mechanism is responsible for the retention of the paternal mtDNA in male bivalves, then molecular signatures of this modification should be discernable in all DUI species. The same three differentially expressed candidate genes were indeed identified in their comparative analysis of male and female gonad transcriptomes for the two DUI freshwater mussel species Venustaconcha ellipsiformis and Utterbackia peninsularis (Capt et al., 2018). This study also revealed that DNA methylation could be involved in the regulation of DUI (Capt et al., 2018) and/or in sex determination and differentiation, as seen in other species (Liu et al., 2015; Capt et al., 2018).
Ubiquitination is not the only process involved in SMI of mitochondria in animals. The mechanisms underlying SMI are widely variable and suggest recurrent loss and restoration and/or several independent origins of this nearly ubiquitous phenomenon (Birky, 2001; Breton and Stewart, 2015). For example, paternal mtDNA can be eliminated by a nuclease-mediated mechanism either during spermatogenesis or after fertilization, or paternal mitochondria can be selectively degraded by autophagy after fertilization (Sato and Sato, 2017). To better understand the molecular basis of DUI, Punzi et al. (2018) selected classes of proteins known to be involved in the maintenance of SMI and compared their features in two closely-related gonochoric clam species differing in their mitochondrial inheritance mechanism, that is, the SMI species Ruditapes decussatus and the DUI species R. philippinarum. Their analysis of gonad transcriptomes focused on nucleases and polymerases, ubiquitination, and proteins involved in autophagy/mitophagy. Their results provided no evidence for a role of nucleases/polymerases or autophagic machinery in the enforcement of SMI in R. decussatus, but transcripts of ubiquitinating enzymes were retrieved, providing additional support to previous transcriptomic studies (Punzi et al., 2018).
In the present study, we take advantage of the existence of closely-related gonochoric–DUI and hermaphroditic–SMI freshwater mussel species to better understand the genetic mechanisms that govern DUI and sex determinations in bivalves. Transcriptomic analyses were performed on three types of gonads, which will also be referred to as “sexes”, i.e. hermaphroditic gonad samples of Utterbackia imbecillis and available male and female gonadal transcriptome data from the species U. peninsularis (Capt et al., 2018). We produced a hybrid assembly that included all libraries from both species, which was used as a reference to map the sequencing reads of each sex. Male–female (MF), hermaphrodite–female (HF) and hermaphrodite–male (HM) comparisons were performed to identify candidate genes involved in sex determination and/or mitochondrial inheritance. In light of information from previous studies (Ghiselli et al., 2012; Milani et al., 2013a; Capt et al., 2018; Punzi et al., 2018), particular attention was given to the following gene ontology (GO) categories to identify candidate genes putatively involved in sex determination or mitochondrial inheritance: reproduction, ubiquitination, nucleases, autophagy/mitophagy and methylation.
Adult U. imbecillis mussels used for this study were collected in the Suwannee River (Florida, USA; lat 29.58684, long -82.94095) in July 2014. All mussels were kept alive during their shipment to the Université de Montréal (QC, Canada). Mussels were dissected and gonads inspected under the microscope. Only mature gonads were kept in the current study and frozen at -80°C until further analyses.
Total RNA extraction from frozen gonad tissues was performed as described in Capt et al. (2018) on eight U. imbecillis samples. Quality and quantity of extracted RNA was assessed via electrophoresis on a 1% agarose gel and using a BioDrop μLITE spectrophotometer; one sample with an absorbance reading A260/A280 below two was discarded (i.e. seven “high quality” RNA samples remained). Because U. imbecillis specimen can be confused visually with U. peninsularis (both species can be found in this sampling site), that these samples were indeed hermaphrodites was validated by verifying the presence of the mitochondrial hermaphrodite open reading frame (h-orf) specific to U. imbecillis (Breton et al., 2011) by PCR (primer sequences: UttimbHorf_F 5’-ATCTTTCTAATTAAGTGTATACG-3’ and UttimbHorf_R 5’-TTGGGCTAGGTTTGTTACGG-3’) and sequencing. Three PCR-confirmed U. imbecillis samples were sent to the Institute for Research in Immunology and Cancer Institute (IRIC, Université de Montréal, Montréal, Canada) for Illumina library preparation (mRNA-Seq) and paired-end (2 × 100 bp) sequencing (Illumina HiSeq2000; San Diego, CA). Paired-end sequencing was chosen for these three samples (no replicate) because it increases the quality of de novo assembly in non-model species and allows for more accurate isoform analyses (Conesa et al., 2016). The same procedure was used in Capt et al. (2018) for U. peninsularis (four female and four male gonad samples without replicates).
Paired-end reads were trimmed with Trimmomatic (v0.32; Bolger et al., 2014) with the options ILLUMINACLIP : TruSeq3-PE.fa:2:30:10 LEADING:3 TRAILING:3 SLIDINGWINDOW:6:10 MINLEN:36. Illumina paired-end adaptors were removed and trailing and leading sequence ends were trimmed if quality (Phred score) dropped below 30. If mean quality dropped below 10 in a six base wide sliding window, nucleotides were replaced by N, and finally, if a trimmed read dropped below a length of 36bp, both paired-end reads were removed. The quality of reads was controlled and visually inspected with FASTQC (v0.11.2; Andrews, 2010), as described in Capt et al. (2018).
Two distinct de novo assemblies were performed with the trimmed reads for each species, using Trinity (Haas et al., 2013). The analytical pipeline used to obtain the transcriptome of U. imbecillis and the main reference transcriptome, which is referred to as the “hybrid” transcriptome for the rest of the study, is the same as the one previously described for U. peninsularis (Capt et al., 2018), i.e. with a minimum length fixed at 300pb for each contig, and other parameters left as default. This was done to allow TransDecoder to recognize Open Reading Frames (ORFs) and reduce the rate of false positives (https://transdecoder.github.io/). The hybrid transcriptome was obtained with the reads of eleven individuals (i.e. our three U. imbecillis hermaphrodites and four male and four female U. peninsularis), and allowed for differential gene expression analysis among the three sexes to avoid bias that would show up from comparing two different transcriptomes. A similar approach was used to study head transcriptomes of two fruit fly species of the genus Anastrepha (Rezende et al., 2016). Most analyses were thus performed on the hybrid transcriptome whereas the gonochoric (U. peninsularis) and the hermaphrodite (U. imbecillis) transcriptomes were used to assess the validity of the hybrid transcriptome, or to check for the presence of species-specific genes. The hybrid transcriptome underwent a clustering of contigs that shared >90% similarity, using CD-HIT-EST (v4.6; Li and Godzik, 2006). This step was added to avoid having multiple orthologous copies of genes from U. imbecillis and U. peninsularis in the hybrid assembly, and consequently false-positives in differential gene expression analyses. The cutoff choice was based on the divergence between mitochondrial female and hermaphrodite nucleotide sequences.
The degree of completeness of the transcriptomes was assessed with Benchmarking Universal Single-Copy Ortholog (BUSCO) software (busco_v3, mode -tran). Two core gene databases, metazoa (978 genes) and eukaryota (303 genes), were used and compared with the Trinity-based de novo assemblies. This comparison measures the presence of near-universal highly conserved single-copy orthologs, and provides information about the completeness of the transcriptomes based on what is expected, depending on the database (Simão et al., 2015).
Raw reads from each individual were aligned to the reference hybrid transcriptome using Bowtie2 (v2.2.4; Langmead and Salzberg, 2012) with default parameters. Next, reads were sorted, indexed, and the corresponding numbers of mapped reads by contig were extracted with Samtools software (v1.1; Li et al., 2009), following the pipeline in Capt et al. (2018). Results were normalized based on Robinson and Oshlack (2010). Given that we previously observed that both DESeq2 (Love et al., 2014) and edgeR (Robinson and Oshlack, 2010) provide similar gene expression patterns (Capt et al., 2018), only edgeR (version edgeR_3.12.0) was used in the current study to simplify analyses and interpretation. EdgeR selects for a more restricted number of differentially expressed genes (DEGs) and increases identification of true positives (Soneson and Delorenzi, 2013; Zhang ZH et al., 2014). DESeq2 was used only to acquire fragments per kilobase of exon per million fragments sequenced (FPKM) values, using “robust = F” to sum the raw counts. DEGs were obtained with edgeR by selecting all contigs with a FPKM value greater than 1 and a fold-change ≥1 or ≤ -1 in one of the three sexes, for each paired-comparison. Gene expression comparisons were performed two per two between hermaphrodites and males (HM), hermaphrodites and females (HF), and males and females (MF). To do so, contrast was fixed at 1 for one sex for each comparison, -1 for the other, and 0 for the sex absent in the comparison, and no intercept was given for the design because there was no ‘control’ value in the current design.
A False Discovery Rate (FDR) adjustment (Benjamini and Hochberg, 1995) was performed to account for multiple comparisons; only values with a corrected p-value < 0.05 were kept as DEG. Unigenes that were expressed only in one sex, i.e. with a FPKM value >1 in one sex and a FPKM value of 0 for the two others, were considered as sex-specific. When genes were hermaphrodite-specific, the associated annotation was checked in the U. imbecillis transcriptome to confirm its validity and specific role in hermaphrodites. A Principal Component Analysis (PCA) was also performed on regularized log transform (rlog) values of the most expressed genes, to verify if individuals were clustering according to their sex.
Assembled contigs from each transcriptome were searched against protein and nucleotide sequences databases. First, potential ORFs, and their linked protein-coding sequences, were predicted using TransDecoder (r20140704; Haas and Papanicolaou, 2017). If several ORFs were found in one contig, the longest was selected. This new TransDecoder list of ORFs allowed a BLASTp search (e-value <1e-5) against protein databases (Swiss-prot and TrEMBL [uniref90 cluster]) available in the Universal Protein Resource (UniProt, April 2018). Swiss-prot is a high quality, manually annotated and non-redundant protein sequence database that focuses mainly on model species to ensure quality annotation. Uniref90 provides computationally generated annotations unfound in Swiss-prot and allows a higher annotation success, but the database is not manually curated. To maximize the hybrid transcriptome annotation success, an additional BLAT search (BLAST-like alignment tool) was performed against NCBI non-redundant (NR) protein database. A BLASTx search (e-value <1e-5) on unigene sequences against Swiss-prot nucleotide database was also performed to complete the annotation [this additional step was also done for U. peninsularis (Capt et al., 2018).
Gene ontology enrichment analyses were performed by combining annotations and differential gene expression information using GOseq (v1.28.0; Young et al., 2010). After FDR correction on p-values, GO terms significantly over-represented were identified with a FDR corrected p-value <0.05.
A total of 188,337,818 paired-end reads was produced for the three U. imbecillis samples and 348,652,616 for male and female U. peninsularis samples (Capt et al., 2018), giving a total of 536,990,434 paired-end reads for both species. The FASTQ raw sequences are accessible under accession numbers SRR8782945-7 (U. imbecillis) and SRR6279376-83 (U. peninsularis; Capt et al., 2018) in the National Centre for Biotechnology Information (NCBI) Sequence Read Archive (SRA) database.
After the trimming process, 439,968,848 high-quality raw reads (81.9%) were retained to build a hybrid assembly of 215,908 transcripts (after the 90% clustering with CD-HIT; before this step, the hybrid assembly provided 245,596 transcripts). The final hybrid transcriptome had an average contig length of 798 bp and a N50 of 1,034. The number of transcripts and median contig length were similar to the values obtained for U. peninsularis (Capt et al., 2018). Complete statistics for all transcriptomes are available in Table 1.
Table 1 De novo assembly quality and annotation statistics of Utterbackia imbecillis, Utterbackia peninsularis and the hybrid transcriptomes.
Supplementary Figure S1 shows the results of the completeness assessment performed using BUSCO. This analysis resulted in 97.8% completely recovered genes for U. imbecillis (20.6% duplicated, 1.6% fragmented, 0.6% missing), and 98.4% completely recovered genes for the hybrid transcriptome (32.2% duplicated, 0.6% fragmented, and 1% missing). Similar results were also obtained for U. peninsularis (Capt et al., 2018). These results validated the completeness of our assemblies and allowed further and reliable analyses of gene expression from the hybrid transcriptome.
Transdecoder recognized 30,964 ORFs from the hybrid transcriptome, which were used to perform BLASTp searches against Swiss-Prot and Uniref90, and BLAT searches against NCBI NR. A total success annotation of 12.3, 15, and 9% were respectively obtained with these three approaches (Table 1). BLASTx against Swiss-prot annotated 9% of the unigenes. Most hits came from mollusk species (data not shown), particularly from the oyster Crassostrea gigas for which whole-genome sequencing and annotation were recently completed (Zhang et al., 2012). When compared with the the recently reported genomes for the freshwater mussels Venustaconcha ellipsiformis (Bivalvia Unionida: Renaut et al., 2018) and Limnoperna fortunei (Bivalvia: Mytilidae; Uliano-Silva et al., 2018), both annotated and assessed for their completeness using the same approach that we did (i.e., respectively with Benchmarking Universal Single-Copy Ortholog [BUSCO] software and BLASTing against uniprot and NCBI NR), our results with BUSCO were comparable and even a bit better [81.9–85% of complete BUSCOs for Renaut et al. (2018) and Uliano-Silva et al. (2018) and 97.7% complete BUSCOs for the present study]. Similarly, Uliano-Silva et al. (2018) annotated 26,198 out of 60,717 predicted protein-coding genes (43%) and Renaut et al. (2018) annotated around 39% of predicted protein genes (>300bp) using BLAST against Uniprot and, for example, we annotated 24,982 of our U. imbecillis transcripts (out of 130,160 = 19.2%). Our percentage of annotation is rather low because we considered all our transcripts (as in other transcriptomic studies). However, if we consider only ORFs identified by Transdecoder (i.e., 31,679 ORFs >300bp from our transcriptome), our percentage of annotation would have been comparable to Uliano-Silva et al. (2018) and Renaut et al. (2018). Also, our results were similar to what was obtained for the gonadal transcriptome of U. peninsularis (Capt et al., 2018). Success annotation in U. imbecillis was also similar to what was obtained for U. peninsularis and the hybrid transcriptome (Table 1). Very few hits came from bacteria or trematodes species, indicating that our gonad samples were not contaminated. Overall, our results are similar to those reported in other transcriptomics studies of bivalve species (Shi et al., 2015; Patnaik et al., 2016; Yarra et al., 2016; Wang et al., 2017), and confirm the difficulty to annotate non-model species, given that few well-characterized model organisms are closely related to bivalves.
Differential gene expression analyses showed that the highest number of differentially expressed genes (DEGs) was observed between hermaphrodites and females (16,573 for the HF comparison), with 9,818 genes up-regulated in hermaphrodites and 6,755 in females (Figure 1). We found 12,352 DEGs between males and females (MF comparison), with 7,710 genes up-regulated in males and 4,642 in females. Finally, 9,762 genes were differentially expressed between hermaphrodites and males (HM comparison), with 5,626 genes up-regulated in hermaphrodites and 4,136 in males. A higher number of DEGs in hermaphrodites is in agreement with previous studies (e.g. Shi et al., 2018), and can be explained by the production of both gametes in hermaphrodite gonads. Figure 1 shows the overlap in DEGs for each comparison. For example, for the HF comparison 16,573 DEGs were found, 6,370 of which were also DEGs in the MF comparison, 1,682 were also DEGs in the HM comparison, and 1,423 were DEGs in all comparisons. The Principal Component Analysis (PCA) performed on regularized log transform (rlog) values of the most expressed genes confirmed that individuals clustered according to their sex (Figure 2), reinforcing the reliability of our results and suggesting low biological variability among samples of the same sex.
Figure 1 Differential expression analysis of Utterbackia spp unigenes. Venn diagram shows differentially expressed genes (DEGs) between sexes: hermaphrodite–female (HF in green), hermaphrodite–male (HM in pink) and male-female (MF in orange) comparisons. The figure shows the number of DEGs unique for one comparison (e.g. 2,877 for MF), the number of DEGs shared between two comparisons (e.g. 5,188 between MF and HM comparisons) and the number of DEGs shared by all comparisons (1,423).
Figure 2 Principal Component Analysis (PCA) performed on regularized log transform (rlog) values of the first thousand most expressed genes per pairwise comparison between the three sexes (males, females and hermaphrodites). The four males are shown in blue, the four females in red and the three hermaphrodites in green.
To unravel potential candidate genes involved in sex determination and/or DUI in Utterbackia spp., we first focused on sex-specific genes (i.e. those expressed only in one sex and not in the two others). Table 2 shows sex-specific genes based on FPKM values. Our analyses revealed 567 genes specific to hermaphrodites, only eleven of which were annotated. DUI individuals showed fewer sex-specific genes with 59 for males (7 annotated) and 100 for females (10 annotated) (Table 2). The detection of the h-orf in hermaphrodite gonads, a mitochondrial gene specific to hermaphroditic freshwater mussel species (Breton et al., 2011), supports the hypothesis that this gene could have an essential role in the development of hermaphrodites in freshwater mussels (Breton et al., 2011; Mitchell et al., 2016). Similarly, the detection of genes involved in ubiquitination in hermaphrodite gonads reinforces the hypothesis that this process could be involved in mitochondrial inheritance and sex determination in bivalves (Ghiselli et al., 2012; Capt et al., 2018; Punzi et al., 2018). However, the few annotated genes in Table 2 (i.e. only 4% of the sex-specific genes were annotated and many of these were uncharacterized proteins) clearly point to the need for further studies of these sex-specific factors to elucidate the molecular mechanisms of sex determination and mitochondrial inheritance in freshwater mussels.
As a second step to unravel potential candidate genes involved in sex determination and/or DUI in Utterbackia spp, we searched for the 20 most up-regulated differentially expressed genes, based on their log2 Fold-Change (log2FC) values, in each pairwise-comparison; Male–Female (MF), Hermaphrodite–Male (HM) and Hermaphrodite–Female (HF) (Supplementary Table S1). For the MF comparison, our results were highly similar to those obtained in our previous study comparing male and female U. peninsularis gonadal transcriptomes (Capt et al., 2018), with up-regulated genes in males including factors involved in spermatogenesis, sperm motility and ubiquitination processes, and up-regulated genes in females including C-type lectin domains, which are known to be involved in gamete recognition and polyspermy blocking during fertilization. One particular new female-biased gene found in the present study was Temptin (c175440_g1_i1; up-regulated mostly in females, and also to a lesser extent in hermaphrodites compared to males [MF; log2FC = -14.7, HM; log2FC = +11.3, HF; log2FC = -3.4]). Temptin is a water-borne protein pheromone that provokes stimulation of mating behavior in some mollusk species [e.g. the sea slug Aplysia sp. (Cummins et al., 2004) and the gastropod Biomphalaria glabrata (Pila et al., 2017)]. Because pheromones are thought to play a critical role in triggering spawning in bivalves (e.g. Rice et al., 2002), it is possible that such a pheromone produced by eggs could stimulate male mussels to spawn and/or attract sperm in marsupia gill chambers where eggs are fertilized in freshwater mussels (Haag, 2012).
Several top DEGs identified in the MF comparison were also present in hermaphrodites (i.e. many up-regulated genes in the hermaphrodites compared to females were “male-type” genes such as testis-specific kinases, whereas many up-regulated genes in the hermaphrodites compared to males were “female-type” genes such as C-type lectin domains and Temptin). These results suggest similar factors involved in gonadal differentiation between the gonochoric U. peninsularis and hermaphrodite U. imbecillis.
To decipher potential candidate genes involved in mitochondrial inheritance in Utterbackia spp, we clustered all the genes found up-regulated in hermaphrodites compared to males and females grouped together (i.e. SMI vs. DUI). Only annotated genes were kept, and if a similar annotation was also found in male- or female-specific DEGs, it was discarded from the list. This step allowed us to focus on genes with a unique role in hermaphrodites, thus potentially revealing genes specific to SMI of mitochondria (Supplementary Table S2). We found 167 genes specifically over-expressed in hermaphrodite gonads. Of these, one unigene was involved in autophagy, seven in nuclease activity and six were involved in polymerase activity, representing three gene ontology categories previously described as being linked to mitochondrial inheritance strategies (Punzi et al., 2018). A similar pipeline was applied for males to find potential candidates directly linked to DUI and retention of sperm mitochondria in male gonads (Supplementary Table S2). We found 153 genes specifically over-expressed in males. One of them has a role in methylation events and another in ubiquitination activity. All of these results are discussed in the section “candidate genes involved in mitochondrial inheritance”.
Figure 3 shows the top ten enriched GO (biological processes and molecular function) categories for each MF, HM, and HF pairwise comparison. For the complete GOseq results, see Supplementary Table S1. Overall, GOseq analyses between males and females revealed 110 gene ontology categories significantly enriched out of 621 listed. HF and HM comparisons showed reduced numbers of enriched categories, with 41 (out of 526) and 25 (out of 456), respectively (Table S1). The top ten enriched categories were similar for MF and HF comparisons, potentially revealing male gonad specific processes and functions, and included microtubule dynamics (GO:0007017, GO:0007018, GO:0005815, GO:0005874, GO:0044450) and mitotic events (GO:190304, GO:0007067) (Figure 3). Microtubule dynamics is important in spermatogenesis and male germ cell development by supervising the division of sperm cells and by modulating sperm shape and motility, leading to correct male fertility (O’Donnell et al., 2012; O’Donnell and O’Bryan, 2014). The up-regulation of microtubule dynamic process in males and hermaphrodites compared to females is thus not surprising. Similar results were also found in previous studies comparing male and female gonad transcriptomes in gonochoric bivalves (Ghiselli et al., 2012; Zhang N et al., 2014; Tong et al., 2015; Shi et al., 2015; Li et al., 2016; Capt et al., 2018). In species with DUI, a role of microtubules in sperm mitochondria aggregation in male gonads has also been suggested (Obata and Komaru, 2005; Ghiselli et al., 2011; Milani et al., 2016), but this still remains to be confirmed. In the HM comparison, over-represented GO categories mostly belonged to response to biotic and external stimuli (GO:0009605, GO:0009607, GO:0043207, GO:0051707, GO:0009617) and ribosome cellular component and activity (GO:0005840, GO:0030529, GO:0044391, GO:0022625, GO: 0015934, GO:0003735). Up-regulation of these GO categories in hermaphrodites could be related to some epigenetic mechanisms with key roles in the differentiation of gametes in hermaphroditic individuals (e.g. Gomot and Griffond, 1993).
Figure 3 Top ten of most significantly enriched Gene Ontology (GO) categories in each comparison; hermaphrodite–male (HM), hermaphrodite–female (HF), and male–female (MF), involved in both biological processes (A) and molecular functions (B). Differentially expressed genes (DEGs) numbers involved in each GO categories are shown by x-axis; female-biased genes are illustrated in pink, male-biased in blue and hermaphrodite-biased in purple. Complete GO terms are written here, *; cellular component assembly involved in morphogenesis, *2; nucleobase-containing compound kinase activity, *3; nucleoside diphosphate kinase activity, *4; phosphotransferase activity, phosphate group as acceptor, *5; nucleobase-containing compound kinase activity.
Our approach to identify candidate genes involved in sex determination consisted of searches in the GO categories, terms that contained ‘reproduction’ and ‘sex’. In addition, candidate genes previously identified as sex-determining genes in the literature in bivalves and in model organisms C. elegans, Drosophila melanogaster and Mus musculus were searched in differentially expressed genes (Table 3; see also Capt et al., 2018). Of the 26 key sex-determining pathway genes examined, putative homologs were found for 15 genes or gene families in freshwater mussel species (Table 3). Based on log2FC values, three main factors were up-regulated in males and hermaphrodites compared to females, i.e. Dsx/Dmrt1/mab3, Sry and Fem1c, all of which are all known to play an important role in male sex determination (Wallis et al., 2008; Kopp, 2012). In contrast, Foxl2, which is a well-known gene implied in oogenesis differentiation in a large variety of species (Uhlenhaut and Treier, 2006), was up-regulated in both females and hermaphrodites when compared to males. Overall, our results are quite similar to what has been observed in previous transcriptomic studies of freshwater mussels or other bivalve species (Capt et al., 2018). One novelty in the present study is the discovery of a highly up-regulated gene in females and hermaphrodites compared to males; Zglp1, which codes for a GATA-4 protein and has a role in early-gonadal differentiation in mammals (Viger et al., 1998).
Table 3 Candidate genes involved in sex determination pathway in freshwater mussels and other bivalves.
To identify candidate genes involved in mitochondrial inheritance, we used the approach proposed by Punzi et al. (2018), i.e. to compare our SMI (U. imbecillis) vs. our DUI (U. peninsularis) species, while considering presence and transcription patterns of proteins belonging to pathways known to be involved in mitochondrial inheritance in animal species. We thus analyzed transcripts belonging to the GO categories “nucleases/polymerases,” “autophagy and mitophagy,” and “ubiquitination pathway” and compared our results with those obtained for the marine clam SMI species Ruditapes decussatus and the DUI species R. philippinarum (Ghiselli et al., 2012; Milani et al., 2013b; Punzi et al., 2018). As mentioned above, we also searched the hermaphrodite-specific and male-specific DEGs for genes related to these categories. Furthermore, because autophagy and mitophagy pathways rely on evolutionarily conserved core components, we used the compiled lists of orthologs related to these processes generated previously (Lee and Han, 2017; Punzi et al., 2018) and used successfully by Punzi et al. (2018) for the bivalve species Ruditapes philippinarum and R. decussatus, to search for their presence in Utterbackia spp based on our annotations. Finally, we discuss transcripts belonging to “methylation GO categories” because our previous study identified this process as potentially important in influencing mtDNA inheritance and/or regulating sex determination and differentiation in bivalves (Capt et al., 2018).
The proposed mode of action for ubiquitination in SMI is to tag paternal mitochondria for degradation during spermatogenesis and/or after fertilization (Sato and Sato, 2017; Punzi et al., 2018), and a modification of this mechanism has been proposed to be responsible for DUI of mitochondria in bivalves (Ghiselli et al., 2012; Milani et al., 2013b). For example, three main male-biased ubiquitination-related factors [birc — baculoviral IAP repeat-containing 4 (inhibitor of apoptosis), psa — proteasome subunit alpha 6, and anubl — AN1 zinc finger ubiquitin-like domain] have previously been hypothesized to be responsible for the maintenance/degradation of spermatozoon mitochondria during embryo development of the DUI species R. philippinarum (Ghiselli et al., 2012; Milani et al., 2013b). These three factors were also reported as male-biased in the freshwater mussel Utterbackia peninsularis (Capt et al., 2018). In the present study, a transcriptional bias (up-regulation) for ubiquitination-related genes is thus expected in males and hermaphrodites compared to females. Our GOseq results revealed seven ubiquitination-related categories enriched (but not significantly) in DUI males (Table S3). The only DEG corresponding to our expectation was annotated as protein MAD2A and is involved in mitotic events (Tables S2 and S3). In our complete list of DEGs, several baculoviral IAP repeat-containing protein-coding genes were found, including male-biases birc genes such as birc-5, which was up-regulated in males but also in hermaphrodites when compared to females (Table 4). Anubl1 was also found up-regulated in males and hermaphrodites compared to females (Table 4). According to previous studies (Milani et al., 2013b; Capt et al., 2018), birc could be an additional mitochondrial tag in DUI species that would protect the M midpiece mitochondria from degradation and anubl1 could be a factor tagging sperm mitochondria that differentiate them from egg mitochondria in DUI embryos. Our results indeed suggest that these genes are mainly male-related but their link to paternal mitochondrial inheritance remains to be clearly demonstrated since they were also found in hermaphrodite and female individuals, both of which lack the M mtDNA. One other ubiquitination-related factor up-regulated in males and hermaphrodites compared to females was the F-box only protein 39 (Table 4). F-box proteins are important components of the protein degradation pathway involving ubiquitination (Ho et al., 2006). Hermaphrodite-specific ubiquitination-related genes were also found (Table S2), reinforcing the hypothesis that this process might be involved in mitochondrial inheritance in freshwater mussels. Finally, nine unigenes were annotated with psa, but only one of them was overexpressed in males and hermaphrodites compared to females (Table 4), supporting the hypothesis that subunits alpha of the proteasome may have a role in mitochondrial inheritance and/or male sex determination or differentiation in bivalve species (Milani et al., 2013b).
The proposed mode of action of nucleases and polymerases is to degrade mtDNA during spermatogenesis to prevent transmission of paternal mtDNA (Sato and Sato, 2017; Punzi et al., 2018). For example, endonuclease G (endoG gene) has been shown to eliminate paternal mitochondrial nucleoids during spermatozoid production in D. melanogaster, thus resulting in SMI of mitochondria (Chan and Schon, 2012;DeLuca and O’Farrell, 2012). Tamas, a gene that codes for one subunit of the mtDNA polymerase (Pol γ-α), has also been identified as a key factor in this process (Yu et al., 2017). Here, a transcriptional bias for such genes (an up-regulation) is expected in SMI hermaphrodites compared to DUI males and females. Our GOseq results and hermaphrodite-specific DEGs revealed 13 genes that corresponded to this situation (Supplementary Table S3), including ten genes coding for Gag or Pol proteins. These genes represent signatures of retrovirus and transposon activity, suggesting a key role for these proteins in hermaphrodite gonads. One promising gene overexpressed in hermaphrodites compared to DUI males and females was exoG (Table 4), which is a paralog of endoG described in higher eukaryotes (Cymerman et al., 2008). It must be noted that endoG was also found but was up-regulated in males and females compared to hermaphrodites (data not shown). We propose that exoG could represent a potential candidate involved in SMI of mitochondria in hermaphroditic freshwater mussels. Like in Drosophila, paternal mtDNA could be mostly degraded by a nuclease-mediated mechanism during the process of spermatogenesis, and paternal mitochondrial structure could be degraded by ubiquitination-related mechanisms after fertilization (Sato and Sato, 2017).
The proposed mode of action of autophagy and mitophagy is to degrade mitochondria during spermatogenesis and/or after fertilization to prevent transmission of paternal mtDNA (Sato and Sato, 2017; Punzi et al., 2018). If these processes are involved in mitochondria degradation during spermatogenesis to maintain SMI, it is expected that they will be up-regulated in SMI hermaphrodites compared to DUI males and females. In addition with GO terms searches, and because autophagy and mitophagy are well-conserved processes, specific autophagy-orthologs listed in Lee and Han (2017) and Punzi et al. (2018) were also searched in our DEGs. Only two genes involved in mitophagy/autophagy processes were indeed up-regulated in the SMI species (Table 4 and Supplementary Table S3): (1) TANK-binding kinase 1, a protein involved in mitophagy regulation in the PINK1-Parkin pathway (Heo et al., 2015), and (2) Ras-like GTP-binding protein YPT1, which is linked to organelle inheritance in yeast (Lewandowska et al., 2013). Combined with exoG, these proteins could belong to the pre-fertilization mechanisms involved in the strict maternal inheritance of mitochondria in hermaphroditic freshwater mussels.
On the other hand, if autophagy and mitophagy are involved in sperm mitochondria degradation after fertilization, it is expected that they will be over-expressed in DUI females and SMI hermaphrodites compared to DUI males. None of our data fulfilled these criteria, a result similar to what was found in Ruditapes spp. (Punzi et al., 2018).
As previously suggested, methylation could play an important role in mitochondrial inheritance and/or sex determination in animal species, including freshwater mussels (Liu et al., 2015; Capt et al., 2018). In Chlamydomonas reinhardtii, there are two mating types (mt+ and mt-), and uniparental inheritance of chloroplast DNA (cpDNA) is achieved by active elimination of the mt-cpDNA, with a possible role of DNA methylation in guaranteeing strict uniparental inheritance by enhancing the replication of the mt+ cpDNA (while unmethylated cpDNAs is being degraded) (Nishimura, 2010). If methylation is involved in DUI of mitochondria in bivalve species, with methylated paternal mtDNA being protected from degradation, we could expect an up-regulation of genes related to this process in DUI males compared to SMI hermaphrodites and DUI females. Our GOseq results and male-specific DEGs revealed two annotated genes fulfilling these criteria, i.e. two methyltransferases, protein arginine N methyltransferase 1/6 that methylates proteins and putative methyltransferase C9orf114 homolog isoform X1 that methylates RNA (Supplementary Table S3). Interestingly, the methyltransferase DNMT1, for which a mitochondrial isoform has been described (Maresca et al., 2015), was found to be overexpressed in males and hermaphrodites compared to females. If DNMT1 is differentially expressed in males and hermaphrodites’ male-parts gonads, it is not related with the assumption that paternal methylated mtDNA would be protected from nucleases, except if another mechanism in SMI hermaphrodites can overcome this event, for example through the endonuclease activity of exoG. Further studies will be necessary to unravel this possibility.
In this study, we reported the transcriptome of male, female, and hermaphrodite gonads of two freshwater mussel species, i.e. the gonochoric, DUI species Utterbackia peninsularis and the hermaphrodite, SMI species U. imbecillis. The results were used to generate a “hybrid transcriptome,” which allowed analyzing differential gene expression among the three sexes. Our results were also compared to published transcriptomes of bivalve species showing different mitochondrial transmission modes (DUI and SMI). This approach permitted us to highlight sex-specific and sex-biased expression patterns, as well as expression patterns associated to SMI or DUI within bivalves having alternative reproductive and mitochondrial transmission modes. Our results revealed similar combinations of genes with conserved sex-linked roles in gonochoric and simultaneous hermaphrodite freshwater mussel species and suggested that steps of the sex-determination pathway are conserved in distantly-related bivalve species. Our results also revealed new candidate genes showing potential roles for SMI or DUI of mitochondria in bivalves, including nucleases and factors involved in autophagy/mitophagy and methylation. Further investigation of these genes will help to unravel the mechanisms underlying mitochondrial inheritance and sex determination in bivalves.
The datasets generated for this study can be found in National Centre for Biotechnology Information (NCBI) Sequence Read Archive (SRA) database, SRR8782945-7.
CC, SR, and SB designed experiments. NJ conducted sampling. CC performed laboratory experiments. CC and SR analyzed data. CC, SR, NJ, DS and SB wrote the manuscript. SB conceived and managed the project. All authors read and approved the final version of the manuscript.
Financial support for this study has been provided by Natural Sciences and Engineering Research Council Discovery Grant awarded to S Breton [grant number RGPIN/435656-2013]. Any use of trade, firm, or product names is for descriptive purposes only and does not imply endorsement by the U.S.
The authors declare that the research was conducted in the absence of any commercial or financial relationships that could be construed as a potential conflict of interest.
We would like to thank Stefano Bettinazzi for his very helpful support and advice and Chase Smith for comments on a previous version of this manuscript. We also thank two anonymous reviewers for suggestions.
The Supplementary Material for this article can be found online at: https://www.frontiersin.org/articles/10.3389/fgene.2019.00840/full#supplementary-material
Andrews, S. (2010). FastQC: a quality control tool for high throughput sequence data. Babraham Bioinform. Available from: http://www.bioinformatics.babraham.ac.uk/projects/fastqc/
Benjamini, Y., Hochberg, Y. (1995). Controlling the false discovery rate: a practical and powerful approach to multiple testing. J. R. Stat. Soc. Series B. Stat. Methodol. 57, 289–300. doi: 10.1111/j.2517-6161.1995.tb02031.x
Birky, C. W., Jr. (2001). The inheritance of genes in mitochondria and chloroplasts: laws, mechanisms, and models. Annu. Rev. Genet. 35, 125–148. doi: 10.1146/annurev.genet.35.102401.090231
Bolger, A. M., Lohse, M., Usadel, B. (2014). Trimmomatic: a flexible trimmer for Illumina sequence data. Bioinformatics 30 (15), 2114–2120. doi: 10.1093/bioinformatics/btu170
Breton, S., Bouvet, K., Auclair, G., Ghazal, S., Sietman, B. E., Johnson, N., et al. (2017b). The extremely divergent maternally- and paternally-transmitted mitochondrial genomes are co-expressed in somatic tissues of two freshwater mussel species with doubly uniparental inheritance of mtDNA. PLoS One. 12 (8), e0183529. doi: 10.1371/journal.pone.0183529
Breton, S., Doucet-Beaupré, H., Stewart, D. T., Hoeh, W. R., Blier, P. U. (2007). The unusual system of doubly uniparental inheritance of mtDNA: isn’t one enough? Trends Genet. 23 (9), 465–474. doi: 10.1016/j.tig.2007.05.011
Breton, S., Doucet-Beaupré, H., Stewart, D. T., Piontkivska, H., Karmakar, M., Bogan, A. E., et al. (2009). Comparative mitochondrial genomics of freshwater mussels (Bivalvia: Unionoida) with doubly uniparental inheritance of mtDNA: gender-specific open reading frames and putative origins of replication. Genetics 183 (4), 1575–1589. doi: 10.1534/genetics.109.110700
Breton, S., Capt, C., Guerra, D., Stewart, D. T. (2018). Sex-Determining Mechanisms in Bivalves. In: Transitions Between Sexual Systems.(Salmon Tower Building New York City, USA: Springer International Publishing). p. 165–192.
Breton, S., Milani, L., Ghiselli, F., Guerra, D., Stewart, D. T., Passamonti, M. (2014). A resourceful genome: updating the functional repertoire and evolutionary role of animal mitochondrial DNAs. Trends Genet. 30, 555–564. doi: 10.1016/j.tig.2014.09.002
Breton, S., Stewart, D. T. (2015). Atypical mitochondrial inheritance patterns in eukaryotes. Genome 58 (10), 423–431. doi: 10.1139/gen-2015-0090
Breton, S., Stewart, D. T., Shepardson, S., Trdan, R. J., Bogan, A. E., Chapman, E. G. (2011). Novel protein genes in animal mtDNA: a new sex determination system in freshwater mussels (Bivalvia: Unionoida)? Mol. Biol. Evol. 28 (5), 1645–1659. doi: 10.1093/molbev/msq345
Capt, C., Renaut, S., Ghiselli, F., Milani, L., Johnson, N. A., Sietman, B. E., et al. (2018). Deciphering the link between Doubly Uniparental Inheritance of mtDNA and sex determination in bivalves: clues from comparative transcriptomics. Genome Biol. Evol. 10 (2), 577–590. doi: 10.1093/gbe/evy019
Chan, D. C., Schon, E. A. (2012). Eliminating mitochondrial DNA from sperm. Dev. Cell. 22 (3), 469–470. doi: 10.1016/j.devcel.2012.02.008
Conesa, A., Madrigal, P., Tarazona, S., Gomez-Cabrero, D., Cervera, A., McPherson, A., et al. (2016). A survey of best practices for RNA-seq data analysis. Genome Biol. 17, 13. doi: 10.1186/s13059-016-0881-8
Cummins, S. F., Nichols, A. E., Amare, A., Hummon, A. B., Sweedler, J. V., Nagle, G. T. (2004). Characterization of Aplysia enticin and temptin, two novel water-borne protein pheromones that act in concert with attractin to stimulate mate attraction. J. Biol. Chem. 279 (24), 25614–25622. doi: 10.1074/jbc.M313585200
Cymerman, I. A., Chung, I., Beckmann, B. M., Bujnicki, J. M., Moiss, G. (2008). EXOG, a novel paralog of Endonuclease G in higher eukaryotes. Nucleic Acids Res. 36 (4), 1369–1379. doi: 10.1093/nar/gkm1169
DeLuca, S. Z., O’Farrell, P. (2012). Barriers to male transmission of mitochondrial DNA in sperm development. Dev. Cell 22 (3), 660–668. doi: 10.1016/j.devcel.2011.12.021
Doucet-Beaupré, H., Breton, S., Chapman, E. G., Blier, P. U., Bogan, A. E., Stewart, D. T., et al. (2010). Mitochondrial phylogenomics of the Bivalvia (Mollusca): searching for the origin and mitogenomic correlates of doubly uniparental inheritance of mtDNA. BMC Evol. Biol. 10, 50. doi: 10.1186/1471-2148-10-50
Ghiselli, F., Milani, L., Chang, P. L., Hedgecock, D., Davis, J. P., Nuzhdin, S. V., et al. (2012). De novo assembly of the manila clam Ruditapes philippinarum transcriptome provides new insights into expression bias, mitochondrial doubly uniparental inheritance and sex determination. Mol. Biol. Evol. 29 (2), 771–786. doi: 10.1093/molbev/msr248
Ghiselli, F., Milani, L., Passamonti, M. (2011). Strict sex-specific mtDNA segregation in the germ line of the DUI species Venerupis philippinarum (Bivalvia: Veneridae). Mol. Biol. Evol. 28 (2), 949–961. doi: 10.1093/molbev/msq271
Gomot, L., Griffond, B. (1993). Action of epigenetic factors on the expression of hermaphroditism in the snail Helix aspersa. Comp Biochem Physiol - Part A Physiol. 104 (2), 195–199. doi: 10.1016/0300-9629(93)90303-L
Guerra, D., Plazzi, F., Stewart, D. T., Bogan, A. E., Hoeh, W. R., Breton, S. (2017). Evolution of sex-dependent mtDNA transmission in freshwater mussels (Bivalvia: Unionida). Sci. Rep. 7, 1551. doi: 10.1038/s41598-017-01708-1
Gusman, A., Lecomte, S., Stewart, D. T., Passamonti, M., Breton, S. (2016). Pursuing the quest for better understanding the taxonomic distribution of the system of doubly uniparental inheritance of mtDNA. Peer J. 4, e2760. doi: 10.7717/peerj.2760
Haag, W. R. (2012). “Host use and host infection strategies,” in North American freshwater mussels: natural history, ecology, and conservation (Cambridge, England: Cambridge University Press), 140–179. doi: 10.1017/CBO9781139048217.006
Haas, B. J., Papanicolaou, A. (2017). TransDecoder. Retrieved from http://transdecoder.github.io.
Haas, B. J., Papanicolaou, A., Yassour, M., Grabherr, M., Blood, P. D., Bowden, J., et al. (2013). De novo transcript sequence reconstruction from RNA-seq using the Trinity platform for reference generation and analysis. Nat. Protoc. 8 (8), 1494–1512. doi: 10.1038/nprot.2013.084
Heo, J. M., Ordureau, A., Paulo, J. A., Rinehart, J., Harper, J. W. (2015). The PINK1-PARKIN mitochondrial ubiquitylation pathway drives a program of OPTN/NDP52 recruitment and TBK1 activation to promote mitophagy. Mol. Cell 60 (1), 7–20. doi: 10.1016/j.molcel.2015.08.016
Ho, M. S., Tsai, P. I., Chien, C. T. (2006). F-box proteins: the key to protein degradation. J. Biomed. Sci. 13 (2), 181–191. doi: 10.1007/s11373-005-9058-2
Kopp, A. (2012). Dmrt genes in the development and evolution of sexual dimorphism. Trends Genet. 28 (4), 175–184. doi: 10.1016/j.tig.2012.02.002
Langmead, B., Salzberg, S. L. (2012). Fast gapped-read alignment with Bowtie 2. Nat. Rev. Clin. Oncol. 9 (4), 357–359. doi: 10.1038/nmeth.1923
Lee, S. R., Han, J. (2017). Mitochondrial nucleoid: shield and switch of the mitochondrial genome. Oxid. Med. Cell Longev. 2017, 8060949. doi: 10.1155/2017/8060949
Lewandowska, A., Macfarlane, J., Shaw, J. M. (2013). Mitochondrial association, protein phosphorylation, and degradation regulate the availability of the active Rab GTPase Ypt11 for mitochondrial inheritance. Mol. Biol. Cell 24 (8), 1185–1195. doi: 10.1091/mbc.e12-12-0848
Li, H., Handsaker, B., Wysoker, A., Fennell, T., Ruan, J., Homer, N., et al. (2009). The sequence alignment/map format and SAMtools. Bioinformatics 25 (16), 2078–2079. doi: 10.1093/bioinformatics/btp352
Li, W., Godzik, A. (2006). Cd-hit: a fast program for clustering and comparing large sets of protein or nucleotide sequences. Bioinformatics 22 (13), 1658–1659. doi: 10.1093/bioinformatics/btl158
Li, Y., Zhang, L., Sun, Y., Ma, X., Wang, J., Li, R., et al. (2016). Transcriptome sequencing and comparative analysis of ovary and testis identifies potential key sex-related genes and pathways in scallop Patinopecten yessoensis. Mar. Biotechnol. 18 (4), 453–465. doi: 10.1007/s10126-016-9706-8
Liu, H., Lamm, M. S., Rutherford, K., Black, M. A., Godwin, J. R., Gemmell, N. J. (2015). Large-scale transcriptome sequencing reveals novel expression patterns for key sex-related genes in a sex-changing fish. Biol. Sex. Differ. 6, 26. doi: 10.1186/s13293-015-0044-8
Love, M. I., Huber, W., Anders, S. (2014). Moderated estimation of fold change and dispersion for RNA-seq data with DESeq2. Genome Biol. 15, 550. doi: 10.1186/s13059-014-0550-8
Maresca, A., Zaffagnini, M., Caporali, L., Carelli., V., Zanna, C. (2015). DNA methyltransferase 1 mutations and mitochondrial pathology: is mtDNA methylated? Front. Genet. 6, 90. doi: 10.3389/fgene.2015.00090
Milani, L., Ghiselli, F., Guerra, D., Breton, S., Passamonti, M. (2013a). A comparative analysis of mitochondrial ORFans: new clues on their origin and role in species with doubly uniparental inheritance of mitochondria. Genome Biol. Evol. 5 (7), 1408–1434. doi: 10.1093/gbe/evt101
Milani, L., Ghiselli, F., Nuzhdin, S. V., Passamonti, M. (2013b). Nuclear genes with sex bias in Ruditapes philippinarum (Bivalvia, veneridae): Mitochondrial inheritance and sex determination in DUI species. J. Exp. Zool. Mol. Dev. Evol. 320B, 442–454. doi: 10.1002/jez.b.22520
Milani, L., Ghiselli, F., Passamonti, M. (2016). Mitochondrial selfish elements and the evolution of biological novelties. Curr. Zool. 62 (6), 687–697. doi: 10.1093/cz/zow044
Mitchell, A., Guerra, D., Stewart, D. T., Breton, S. (2016). In silico analyses of mitochondrial ORFans in freshwater mussels (Bivalvia: Unionoida) provide a framework for future studies of their origin and function. BMC Genomics. 17, 597. doi: 10.1186/s12864-016-2986-6
Nishimura, Y. (2010). Uniparental inheritance of cpDNA and the genetic control of sexual differentiation in Chlamydomonas reinhardtii. J. Plant. Res. 132 (2), 149–162. doi: 10.1007/s10265-009-0292-y
Obata, M., Komaru, A. (2005). Specific location of sperm mitochondria in mussel Mytilus galloprovincialis zygotes stained by MitoTracker. Dev. Growth. Differ. 47 (4), 255–263. doi: 10.1111/j.1440-169X.2005.00801.x
O’Donnell, L., Rhodes, D., Smith, S. J., Merriner, J., Clark, B. J., Borg, C., et al. (2012). An essential role for katanin p80 and microtubule severing in male gamete production. PLoS Genet. 8 (5), e1002698. doi: 10.1371/journal.pgen.1002698
O’Donnell, L., O’Bryan, M. K. (2014). Microtubules and spermatogenesis. Semin. Cell Dev. Biol. 30, 45–54. doi: 10.1016/j.semcdb.2014.01.003
Passamonti, M., Ghiselli, F. (2009). Doubly uniparental inheritance: two mitochondrial genomes, one precious model for organelle DNA inheritance and evolution. DNA Cell Biol. 28 (2), 79–89. doi: 10.1089/dna.2008.0807
Patnaik, B. B., Wang, T. H., Kang, S. W., Hwang, H. J., Park, S. Y., Park, E. B., et al. (2016). Sequencing, de novo assembly, and annotation of the transcriptome of the endangered freshwater pearl bivalve, Cristaria plicata, provides novel insights into functional genes and marker discovery. PLoS One. 11, e0148622. doi: 10.1371/journal.pone.0148622
Punzi, E., Milani, L., Ghiselli, F., Passamonti, M. (2018). Lose it or keep it: (how bivalves can provide) insights into mitochondrial inheritance mechanisms. J. Exp. Zool. Mol. Dev. Evol. 330 (1), 41–51. doi: 10.1002/jez.b.22788
Picard, M., Wallace, D. C., Burelle, Y. (2016). The rise of mitochondria in medicine. Mitochondrion 30, 105–116. doi: 10.1016/j.mito.2016.07.003
Pila, E. A., Peck, S. J., Hanington, P. C. (2017). The protein pheromone temptin is an attractant of the gastropod Biomphalaria glabrata. J. Comp. Physiol. A. Neuroethol. Sens. Neural. Behav. Physiol. 203 (10), 855–866. doi: 10.1007/s00359-017-1198-0
Renaut, S., Guerra, D., Hoeh, W. R., Stewart, D. T., Bogan, A. E., Ghiselli, F., et al. (2018). Hybrid de novo assembly of the draft genome of the freshwater mussel Venustaconcha ellipsiformis (Bivalvia: Unionida). Genome Biol. Evol. 10, 1637–1646. doi: 10.1093/gbe/evy117
Rezende, V. B., Congrains, C., Lima, A. L. A., Campanini, E. B., Nakamura, A. N., Lima de Oliveira, J. (2016). Head transcriptomes of two closely related species of fruit flies of the Anastrepha fraterculus group reveals divergent genes in species with extensive gene flow. G3 6 (10), 3283–3295. doi: 10.1534/g3.116.030486
Rice, P., Ray, S. M., Painter, S. J., Nagle, G. T. (2002). An intrinsic membrane protein in oyster sperm stimulates spawning behaviors in Crassostrea virginica: implications for aquaculture. J. Shellfish Res. 21, 715–718.
Robinson, M. D., Oshlack, A. (2010). A scaling normalization method for differential expression analysis of RNA-seq data. Genome Biol. 11, 3. doi: 10.1186/gb-2010-11-3-r25
Sato, K., Sato, M. (2017). Multiple ways to prevent transmission of paternal mitochondrial DNA for maternal inheritance in animals. J. Biochem. 162 (4), 247–253. doi: 10.1093/jb/mvx052
Shi, J., Hong, Y., Sheng, J., Peng, K., Wang, J. (2015). De novo transcriptome sequencing to identify the sex-determination genes in Hyriopsis schlegelii. Biosci. Biotechnol. Biochem. 0, 1–9. 6. doi: 10.1080/09168451.2015.1025690
Shi, Y., Liu, W., He, M. (2018). Proteome and transcriptome analysis of ovary, intersex gonads, and testis reveals potential key sex reversal/differentiation genes and mechanism in scallop Chlamys nobilis. Mar. Biotechnol. 20 (2), 220–245. doi: 10.1007/s10126-018-9800-1
Simão, F. A., Waterhouse, R. M., Ioannidis, P., Kriventseva, E. V., Zdobnov, E. M. (2015). BUSCO: assessing genome assembly and annotation completeness with single-copy orthologs. Bioinformatics 31 (19), 3210–3212. doi: 10.1093/bioinformatics/btv351
Soneson, C., Delorenzi, M. (2013). A comparison of methods for differential expression analysis of RNA-seq data. BMC Bioinform. 14, 91. doi: 10.1186/1471-2105-14-91
Sutovsky, P., Moreno, R. D., Ramalho-Santos, J., Dominko, T., Simerly, C., Schatten, G. (1999). Ubiquitin tag for sperm mitochondria. Nature 402 (6760), 371–372. doi: 10.1038/46466
Tong, Y., Zhang, Y., Huang, J., Xiao, S., Zhang, Y., Li, J., et al. (2015). Transcriptomics analysis of Crassostrea hongkongensis for the discovery of reproduction-related genes. PLoS One. 10 (8), e0134280. doi: 10.1371/journal.pone.0134280
Uhlenhaut, N. H., Treier, M. (2006). Foxl2 function in ovarian development. Mol. Genet. Metab. 88 (3), 225–234. doi: 10.1016/j.ymgme.2006.03.005
Uliano-Silva, M., Dondero, F., Otto, T. D., Costa, I., Lima, N. C. B., Americo, J. A., et al. (2018). A hybrid-hierarchical genome assembly strategy to sequence the invasive golden mussel, Limnoperna fortunei. GigaScience 7, 101. doi: 10.1093/gigascience/gix128
Viger, R. S., Mertineit, C., Trasler, J. M., Nemer, M. (1998). Transcription factor GATA-4 is expressed in a sexually dimorphic pattern during mouse gonadal development and is a potent activator of the Mullerian inhibiting substance promoter. Development 125 (14), 2665–2675.
Wallace, D. C. (2016). Mitochondrial DNA in evolution and disease. Nature 535, 498–500. doi: 10.1038/nature18902
Wallis, M. C., Waters, P. D., Graves, J. A. M. (2008). Sex determination in mammals — before and after the evolution of SRY. Cell Mol. Life Sci. 65, 3182–3195. doi: 10.1007/s00018-008-8109-z
Wang, X., Liu, Z., Wu, W. (2017). Transcriptome analysis of the freshwater pearl mussel (Cristaria plicata) mantle unravels genes involved in the formation of shell and pearl. Mol. Genet. Genomics. 292 (2), 343–352. doi: 10.1007/s00438-016-1278-9
Yarra, T., Gharbi, K., Blaxter, M., Peck, L. S., Clark, M. S. (2016). Characterization of the mantle transcriptome in bivalves: Pecten maximus, Mytilus edulis and Crassostrea gigas. Mar. Genomics. 27, 9–15. doi: 10.1016/j.margen.2016.04.003
Young, M. D., Wakefield, M. J., Smyth, G. K., Oshlack, A. (2010). Gene ontology analysis for RNA-seq: accounting for selection bias. Genome Biol. 11, 2. doi: 10.1186/gb-2010-11-2-r14
Yu, Z., O’Farrell, P. H., Yakubovich, N., DeLuca, S. Z. (2017). The mitochondrial DNA polymerase promotes elimination of paternal mitochondrial genomes. Curr. Biol. 27 (7), 1033–1039. doi: 10.1016/j.cub.2017.02.014
Zhang, G., Fang, X., Guo, X., Li, L., Luo, R., Xu, F., et al. (2012). The oyster genome reveals stress adaptation and complexity of shell formation. Nature 490, 49–54. doi: 10.1038/nature11413
Zhang, N., Xu, F., Guo, X. (2014). Genomic analysis of the Pacific oyster (Crassostrea gigas) reveals possible conservation of vertebrate sex determination in a mollusc. G3 4, 2207–2217. doi: 10.1534/g3.114.013904
Zhang, Z. H., Jhaveri, D. J., Marshall, V. M., Bauer, D. C., Edson, J., Narayanan, R. K., et al. (2014). A comparative study of techniques for differential expression analysis of RNA-seq data. PLoS One. 9, e1360. doi: 10.1371/journal.pone.0103207
Keywords: comparative transcriptomics, mitochondrial DNA, hermaphroditism, ubiquitination, mitophagy, nucleases, methylation, doubly uniparental inheritance
Citation: Capt C, Renaut S, Stewart DT, Johnson NA and Breton S (2019) Putative Mitochondrial Sex Determination in the Bivalvia: Insights From a Hybrid Transcriptome Assembly in Freshwater Mussels. Front. Genet. 10:840. doi: 10.3389/fgene.2019.00840
Received: 26 March 2019; Accepted: 13 August 2019;
Published: 13 September 2019.
Edited by:
Jacob A. Tennessen, Harvard University, United StatesReviewed by:
Francesco Dondero, University of Eastern Piedmont, ItalyCopyright © 2019 Capt, Renaut, Stewart, Johnson and Breton. This is an open-access article distributed under the terms of the Creative Commons Attribution License (CC BY). The use, distribution or reproduction in other forums is permitted, provided the original author(s) and the copyright owner(s) are credited and that the original publication in this journal is cited, in accordance with accepted academic practice. No use, distribution or reproduction is permitted which does not comply with these terms.
*Correspondence: Charlotte Capt, Y2hhcmxvdHRlLmNhcHRAdW1vbnRyZWFsLmNh; Sophie Breton, cy5icmV0b25AdW1vbnRyZWFsLmNh
Disclaimer: All claims expressed in this article are solely those of the authors and do not necessarily represent those of their affiliated organizations, or those of the publisher, the editors and the reviewers. Any product that may be evaluated in this article or claim that may be made by its manufacturer is not guaranteed or endorsed by the publisher.
Research integrity at Frontiers
Learn more about the work of our research integrity team to safeguard the quality of each article we publish.