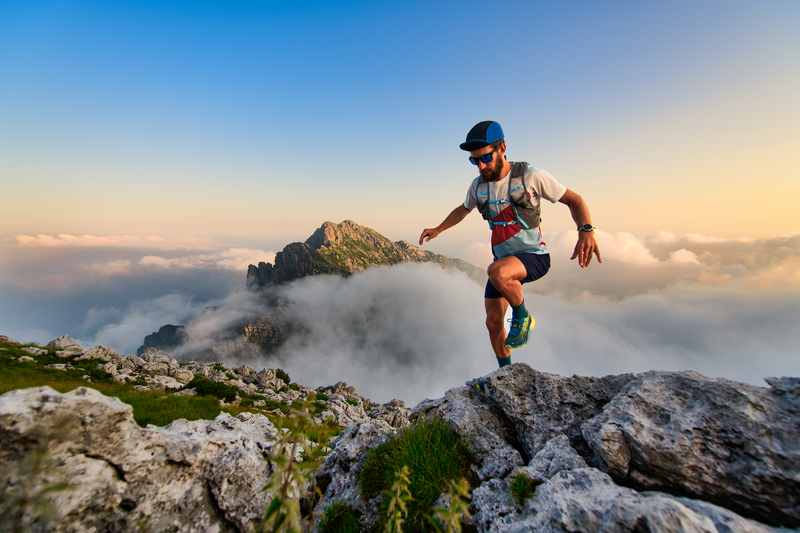
94% of researchers rate our articles as excellent or good
Learn more about the work of our research integrity team to safeguard the quality of each article we publish.
Find out more
MINI REVIEW article
Front. Genet. , 28 August 2019
Sec. Epigenomics and Epigenetics
Volume 10 - 2019 | https://doi.org/10.3389/fgene.2019.00764
This article is part of the Research Topic Pioneering the Nexus of Chromatin and Metabolism View all 19 articles
The effect of one carbon metabolism on DNA methylation has been well described, bridging nutrition, metabolism, and epigenetics. This modification is mediated by the metabolite S-adenosyl methionine (SAM), which is also the methyl-donating substrate of histone methyltransferases. Therefore, SAM levels that are influenced by several nutrients, enzymes, and metabolic cofactors also have a potential impact on histone methylation. Although this modification plays a major role in chromatin accessibility and subsequently in gene expression in healthy or diseased states, its role in translating nutritional changes in chromatin structure has not been extensively studied. Here, we aim to review the literature of known mechanistic links between histone methylation and the central one carbon metabolism.
In the last decade, the connection between one carbon metabolism and DNA methylation in embryonic development, cancer, and neurodegenerative diseases has been well established (reviewed in (Liu and Ward, 2010; Rush et al., 2014). Such metabolically induced changes in DNA methylation are inherited by offsprings supporting the importance of nutrition in chromatin accessibility within generations (Morgan et al., 1999; Waterland and Jirtle, 2003). As the central factors involved in one carbon metabolism also act as critical cofactors of histone modifiers, it is highly likely that histone methylation is tightly connected to the cell’s metabolic state (Locasale and Cantley, 2011; Lu and Thompson, 2012; Sharma and Rando, 2017). Methylated histones are recognized by the so-called chromatin readers that recruit other molecules resulting in a change of gene activity (Taverna et al., 2007). Methylation of histone 3 (H3) at lysine 4 (K4), K36 or K79 are generally associated with active chromatin, while methylation at K9, K27, and histone 4 (H4) K20 are frequently associated with repressed chromatin. The different methylation sites and methylation degrees are thought to play specific roles at different stages of transcription (Greer and Shi, 2012), making histone methylation a key factor in the regulation of cellular physiology (Sarg et al., 2002; Nottke et al., 2009; Pedersen and Helin, 2010).
Most importantly, SAM levels are influenced by dietary intake, especially in the case of mammals. Nutrients serve as a substrate for one-carbon metabolic pathway, making methylation highly dependent on the availability of certain nutrients. Since the impact of nutrition in DNA methylation is well known, we try to focus and summarize the molecular mechanisms bridging one carbon metabolites and its cofactors with histone methylation.
Methylation is mediated by the metabolite S-adenosyl methionine (SAM) (Mentch et al., 2015), the synthesis of which is catalyzed from methionine and ATP by methionine adenosyltransferase enzymes (MATs) [or SAM synthetases (SAM-S); will be referred to as MAT] (Cantoni, 1953). MAT enzymes are encoded by the MAT1A and MAT2A genes, where the former is only expressed in the liver while the latter in most mammalian tissues. SAM is used as a methyl-donor by various cytosolic or nuclear methyltransferases, catalyzing the formation of a methylated substrate and S-adenosylhomocysteine (SAH) (Bailey and Gregory, 1999; Tong et al., 2009; Teperino et al., 2010; Mentch et al., 2015). SAH, a potent inhibitor of most known methyltransferases, is hydrolyzed to homocysteine (Hcy) and adenine by S-adenosylhomocysteine hydrolase (AHCY) to prevent its accumulation (Obeid and Herrmann, 2009; Tehlivets et al., 2013). As the increased concentration of Hcy can also have a negative effect on many methyltransferases, it is efficiently remethylated back to methionine in a folate- and/or choline-dependent manner (Yudkoff, 2012).
The folate derivative N-5,10-methylenetetrahydrofolate is synthesized by C-1-tetrahydrofolatesynthase (MTHFD1) and serine hydroxy methyltransferase (SHMT2) (MacFarlane et al., 2009). In serum, it is converted to 5-methyltetrahydrofolate, the predominant form of folate. Ultimately, a cobalamin (vitamin B12)-dependent methionine synthase (MTR or MS) catalyzes the transfer of one methyl group from 5-methyltetrahydrofolate to Hcy. During the choline-dependent remethylation of methionine, choline is oxidized to betaine, which can also provide one methyl group to Hcy. This latter reaction is catalyzed by the enzyme betaine-homocysteine methyltransferase (BHMT), localized only in the mitochondria of liver and kidney (Blom and Smulders, 2011; Muskiet et al., 2018). The remaining 50% of Hcy, which is not converted to methionine, enters the transsulfuration pathway resulting in the generation of cysteine (Škovierová et al., 2016). The first reaction of this pathway is the condensation of Hcy and serine (Ser) to cystathionine that is further hydrolyzed to cysteine and 2-oxobutyrate. 2-Oxobutyrate is decarboxylated to propionyl-CoA and converted to succinyl-CoA, an intermediate of the TCA cycle.
In addition to the regulation of enzymes involved in remethylation of methionine and SAM synthesis, intracellular SAM concentration can also be regulated by SAM metabolizing enzymes such as glycine N-methyltransferase (GNMT), which catalyzes the transfer of a methyl group from SAM to glycine to form sarcosine (Luka et al., 2009). Interestingly, N-5-methyltetrahydrofolate has also been shown to inhibit GNMT (Wang et al., 2011a). Although the binding between GNMT and N-5-methyltetrahydrofolate has been well characterized in the literature, the impact of GNMT expression in methyl-folate-dependent reactions has not yet been elucidated.
In the following section, we will focus on studies involving the components of one carbon metabolism and how nutrient limitation or enzyme disruption affects histone methylation.
MAT (or Sam-S) is arguably the most important enzyme in the methionine-folate cycle. Organisms such as Saccharomyces pombe and Drosophila melanogaster have a single gene responsible (called sam1 or Sam-S, respectively) for SAM synthesis, making them a simpler model to study the physiological and molecular effects of MAT disruption (Larsson et al., 1996; Hayashi et al., 2018). For instance, genetic ablation of MAT in S. pombe decreases SAM levels and the corresponding histone methylation, causing defects in cell growth and cell cycle progression (Hayashi et al., 2018). Similarly, in S. cerevisae auxotroph, low methionine concentration results in slower cell division and reduced H3K4 di- and trimethylation. This effect is irrespective of the growth rate or the methyltransferase activity of the enzyme. This observation was also extended to human K562 cells, where both H3K9me3 and H3K4me3 levels are affected (Sadhu et al., 2013). In D. melanogaster, the effects vary from developmental regulation, temperature-sensitive sterility in females to changes in position-effect variegation (Larsson et al., 1996). In mammalian cells, MATIIα reduction leads to a derepression of the COX2 gene, which is generally repressed via SETDB1-mediated H3K9me3 methylation. MATIIα reduction also results in an overall decrease of H3K4me3 that potentially leads to a general loss in transcriptional activity (Kera et al., 2013). In addition, MATIIα was also found to act as a corepressor of the MafK transcription factor to maintain the repressed state of the HO-1 gene (Katoh et al., 2011). A relatively recent study observed similar effects on HCT116 cells upon methionine restriction and MATIIα knockdown. In both cases, global histone methylation levels are reduced, whereas the levels of H3K9 and K27 mono-methylation remains unchanged (Haws and Denu, 2017). Furthermore, methionine restriction (MR) was shown to have a direct impact on both SAM and H3K4 methylation (Mentch et al., 2015). This was indeed observed upon MATIIα disruption in mammalian cells and S. pombe (Kera et al., 2013; Hayashi et al., 2018). Methionine refeeding leads to a reestablishment of the H3K4me levels and similar effects have been observed in a more recent study as well (Dai et al., 2018).
Another SAM-buffering enzyme, which has been underexplored in the context of histone methylation, is GNMT. Increase in GNMT expression causes SAM depletion and sarcosine (N-methylglycine) induction (Obata et al., 2014). This can result in changes of histone methylation status, although such studies have been negligible. Interestingly, GNMT was found to be one of the many folate-binding proteins of the liver and was structurally proven to bind 5-methyl-THF pentaglutamate that inhibits its methyltransferase activity (Cook and Wagner, 1984; Luka et al., 2007). Furthermore, GNMT induction stimulates the remethylation of Hcy by improving folate retention, while the absence of GNMT reduces hepatic folate levels and MTR expression (Wang et al., 2011a). GNMT can also regulate the transsulfuration pathway of Hcy, inducing an increase in cysteine and removal of excess Hcy under high methionine condition (Wang et al., 2011b). In addition, a recent study described a reduced gluconeogenesis in GNMT knockout mice due to a complex metabolic reprogramming towards pathways that utilize elevated SAM levels (Hughey et al., 2018).
Folate is another important metabolite in the one carbon cycle. As mentioned in the previous section, apart from its well-documented role in DNA methylation (Crider et al., 2012; Ly et al., 2012), studies on its impact on histone methylation are sparse. Like most intermediary metabolites, folate can be observed in the nucleus (Zamierowski and Wagner, 1977), where it has been shown to bind and regulate a number of enzymes. One of them is LSD1, which demethylates H3K4me1/2. The importance of folate binding has been suggested to protect LSD1 from inhibition by formaldehyde, a by-product of the demethylase reaction (Luka et al., 2011). This observation was supported by a crystal structure showing the binding of folate in proximity to LSD1’s active site (Luka et al., 2014). In liver samples of mouse fed with a folate-deficient diet, H3K4me is substantially increased, suggesting a reduced activity of LSD1 (Garcia et al., 2016). Moreover, rats fed with folate-deficient diet show a three-fold lower SAM concentration in the liver compared to controls, and a resupplementation of folate positively correlates with SAM concentration (Miller et al., 1994). On the other hand, in a S. cerevisae auxotroph strain of folate (fol3Δ), SAM levels do not change between wild-type and low folate cultures, but a prominent increase was indeed observed in high folate conditions (Sadhu et al., 2013). This suggests the possibility of organism-specific changes in histone methylation under low folate conditions or the regulation of SAM via a methionine or an SAM-independent mechanism. Nevertheless, lower folate level leads to a decrease in H3K4me2/3 and consequently affects gene expression. Interestingly, H3K79me was found to be more resilient than H3K4me. The reasons for this are yet to be explored but may be due to differences in Km of the respective methyltransferases for SAM (Sadhu et al., 2013). Surprisingly, basic enzymatic parameters for most histone methyltransferases are not known or vary substantially among different laboratories (Eskeland et al., 2004; Patnaik et al., 2004). Another possible cause for the different susceptibilities of methylation sites is an allosteric regulation of enzymes by additional modifications on the same or proximal nucleosome. Examples for this are the influence of H2B ubiquitination on H3K4 and K79 methylation (Soares and Buratowski, 2013) and the induction of PRC2 activity by the recognition of a preexisting H3K27 methylation (Margueron et al., 2009).
Reports suggest that nutritional folate reduction also results in an increase in the level of plasma Hcy (pHcy) in rats (Miller et al., 1994). Indeed, rats exposed to diets of high methionine, B vitamin deficient, or both show increased plasma Hcy levels (Esse et al., 2013). These diets also induce an increase in SAH levels in the liver, a reduction in H3 arginine 8 dimethylation (H3R8me2) in the brain, and upregulated EZH2 expression and subsequent H3K27me3 at the CFTR promoter (Yang et al., 2018).
The link between Hcy, folate, and SAM levels is through MTHFR that converts Hcy to methionine and maintains intracellular SAM concentration. Indeed, MTHFR has been shown to be an important regulator of heterochromatin maintenance. A knockdown of MTHFR in HeLa cells causes a decrease in H3K9me3 and an increase in centromeric satellite repeat expression (Xiahou et al., 2014). In a subsequent study, cells depleted of MTHFR accumulate in S-phase due to decreased H4K20me3 levels. This, however, is rescued by overexpressing the H4K20 trimethylase SUV4-20H2 (Zhang et al., 2003; Li et al., 2017). Whether this effect is directly due to a reduction in SAM level is questionable, since depletion of SAM by MATIIα/β knockdown results in a G1 arrest by prevention of S phase entry (Lin et al., 2014). On the other hand, serine provides methyl groups for the conversion of THF to methylene-THF that serves as a substrate for MTHFR. The enzyme SHMT accomplishes the conversion of serine to glycine, and it has been shown in yeast (Shm2) to occur via a complex called SESAME, along with PHGDH (Ser32), SAM-synthetases, pyruvate kinase (Pyk), and acetyl-CoA synthetase. This complex interacts with Set1, an H3K4 methyltransferase, and regulates H3K4me3 in the promoter of Pyk leading to its subsequent autoregulation (Li et al., 2015).
Hcy remethylation can also be accomplished via betaine that is obtained from choline, as catalyzed by the betaine homocysteine S-methyltransferase (BHMT). Dietary choline supplementation in rats was shown to have a strong effect on fetal histone methylation. Specifically, H3K9me2/3 and H3K4me2 are directly and indirectly proportional to choline intake, respectively. Choline intake also downregulates G9a and SUV39H1 (both H3K9 methyltransferases), suggesting that the presence of choline might result in a transcriptionally open environment (Davison et al., 2009). Upon choline deprivation of mouse neural-progenitor cells, H3K9me2 is modified at specific genomic locus, causing poor recruitment of G9a (Mehedint et al., 2010). On the contrary, betaine shows a positive correlation with H3K4me3 level in neuroblastoma cells. The corresponding enzyme, BHMT, believed to be present primarily in adult kidneys and liver, was also found to be expressed in adult central nervous system (CNS), suggesting that betaine can serve as a source of methyl group for Hcy remethylation in other tissues as well (Singhal et al., 2015). A summary of enzymes/metabolites and their impact on histone modifications is given in Table 1.
Histone methylation status can also be influenced by levels of vitamin B12, MTHFD1, MTR, and ACHY/SAHH. Although their role in DNA methylation has been documented in some cases, very few studies have been carried out to explicitly investigate their impact on histone methylation.
Studies on transmethylation and one carbon cycle have so far been investigated in the context of DNA methylation. However, in recent years, their impact on histone posttranslational modifications is becoming more and more evident. For instance, the role of GNMT in transmethylation/folate pathway is quite known, but it is surprising that its role in histone methylation has not yet been characterized, although their influence on SAM levels is well established. Moreover, through the roles of Hcy in transsulfuration and GNMT in reprogramming liver metabolism, folate–methionine cycle can now be evidently linked to two important metabolic substrates of posttranslational modifications, SAM, and acetyl-CoA. Studies on the interplay of these two key metabolites and their corresponding changes in histone tail modification would further improve our understanding of the connection between nutrition, metabolism, and epigenetics.
In the last decade, mass spectrometry has offered a more accurate large-scale quantitative analysis of histone modifications than the traditionally used Western blot (Völker-Albert et al., 2018). A new mass spectrometry method for histone posttranslational modification (PTM) analysis provides the possibility of high throughput screening of 200 PTMs in less than a minute (Sidoli et al., 2019a). Furthermore, methods that combine metabolomics and histone PTM analysis have already been developed for acetyl-CoA and histone acetylation (Sidoli et al., 2019b). These developments may not only help to broaden our view of how metabolism and epigenetic processes are connected but also open new pathways for pharmacological intervention in diseases.
Indeed, a surge in systematic effort to develop cancer drugs, inhibitors of methionine, and folate pathway enzymes has been observed in the last years. For instance, 3-deazaneplanocin A (DZNep), an inhibitor of ACHY, regulates the expression of both EZH2, a H3K27 trimethylase, and SETDB1, a H3K9 trimethylase. This drug has been shown to reduce H3K9me2 in human lung cancer cells and H3K27me2 in chondrosarcomas (Miranda et al., 2009; Lee and Kim, 2013; Girard et al., 2014), in addition to reactivating PRC2 repressed genes and inducing apoptosis in cancer cells via FBXO32 (Tan et al., 2007). Similar drugs that inhibit MTHFD1, such as LY345899, have been proposed to be potent drugs for anticancer therapy (Gustafsson, 2017). Interestingly, Temozolomide (TMZ), a drug that has been used to induce DNA methylation and has anticancer properties, was found to affect methylation of H3K4 in vitro (Wang et al., 2016). More recently, a MAT2A inhibitor known as AG-270 has been used for the treatment of patients with solid tumors or lymphoma in phase 1 clinical trials (Agios pharmaceuticals, Inc., 2018). Whether the effect of these drugs on histone methylation is an important aspect of their function or is only a bystander effect is yet to be determined.
MS, AV, and AI participated in drafting the article and revising it critically for important intellectual content; and gave final approval of the version to be submitted. The corresponding author takes primary responsibility for communication with the journal and editorial office during the submission process, throughout peer review, and during publication.
This work has received funding from the European Union's Horizon 2020 research and innovation programme under the Marie Skłodowska-Curie grant agreement No 675610; QBM and SFB1309
The authors declare that the research was conducted in the absence of any commercial or financial relationships that could be construed as a potential conflict of interest.
Agios pharmaceuticals, Inc. (2018). Study of AG-270 in subjects with advanced solid tumors or lymphoma with MTAP loss—full text view—ClinicalTrials.gov. Available at: https://clinicaltrials.gov/ct2/show/NCT03435250 [Accessed May 29, 2019].
Bailey, L. B., Gregory, J. F. (1999). Polymorphisms of methylenetetrahydrofolate reductase and other enzymes: metabolic significance, risks and impact on folate requirement. J. Nutr. 129, 919–922. doi: 10.1093/jn/129.5.919
Blom, H. J., Smulders, Y. (2011). Overview of homocysteine and folate metabolism. With special references to cardiovascular disease and neural tube defects. J. Inherit. Metab. Dis. 34, 75–81. doi: 10.1007/s10545-010-9177-4
Cantoni, G. L. (1953). S-Adenosylmethionine; a new intermediate formed enzymatically from L-methionine and adenosinetriphosphate. J. Biol. Chem. 204, 403–416.
Cook, R. J., Wagner, C. (1984). Glycine N-methyltransferase is a folate binding protein of rat liver cytosol. Proc. Natl. Acad. Sci. U. S. A. 81, 3631–3634. doi: 10.1073/pnas.81.12.3631
Crider, K. S., Yang, T. P., Berry, R. J., Bailey, L. B. (2012). Folate and DNA methylation: a review of molecular mechanisms and the evidence for folate’s role. Adv. Nutr. 3, 21–38. doi: 10.3945/an.111.000992
Dai, Z., Mentch, S. J., Gao, X., Nichenametla, S. N., Locasale, J. W. (2018). Methionine metabolism influences genomic architecture and gene expression through H3K4me3 peak width. Nat. Commun. 9, 1955. doi: 10.1038/s41467-018-04426-y
Davison, J. M., Mellott, T. J., Kovacheva, V. P., Blusztajn, J. K. (2009). Gestational choline supply regulates methylation of histone H3, expression of histone methyltransferases G9a (Kmt1c) and Suv39h1 (Kmt1a), and DNA methylation of their genes in rat fetal liver and brain. J. Biol. Chem. 284, 1982–1989. doi: 10.1074/jbc.M807651200
Eskeland, R., Czermin, B., Boeke, J., Bonaldi, T., Regula, J. T., Imhof, A. (2004). The N-terminus of Drosophila SU(VAR)3–9 mediates dimerization and regulates its methyltransferase activity. Biochem. 43 (12), 3740–3749. doi: 10.1021/bi035964s
Esse, R., Florindo, C., Imbard, A., Rocha, M. S., De Vriese, A. S., Smulders, Y. M., et al. (2013). Global protein and histone arginine methylation are affected in a tissue-specific manner in a rat model of diet-induced hyperhomocysteinemia. Biochim. Biophys. Acta - Mol. Basis Dis. 1832, 1708–1714. doi: 10.1016/j.bbadis.2013.05.013
Garcia, B. A., Luka, Z., Loukachevitch, L. V., Bhanu, N. V., Wagner, C. (2016). Folate deficiency affects histone methylation. Med. Hypotheses 88, 63–67. doi: 10.1016/j.mehy.2015.12.027
Girard, N., Bazille, C., Lhuissier, E., Benateau, H., Llombart-Bosch, A., Boumediene, K., et al. (2014). 3-Deazaneplanocin A (DZNep), an inhibitor of the histone methyltransferase EZH2, induces apoptosis and reduces cell migration in chondrosarcoma cells. PLoS One 9, e98176. doi: 10.1371/journal.pone.0098176
Greer, E. L., Shi, Y. (2012). Histone methylation: a dynamic mark in health, disease and inheritance. Nat. Rev. Genet. 13, 343–357. doi: 10.1038/nrg3173
Gustafsson, R. (2017). Crystal structure of the emerging MTHFD2 in complex with substrate-based inhbitor. AACR. 77 (4), 937–948. doi: 10.1158/0008-5472.CAN-16-1476
Haws, S. A., Denu, J. M. (2017). Regulation of histone methylation via methionine metabolism. FASEB J. 31, 755.9–755.9.
Hayashi, T., Teruya, T., Chaleckis, R., Morigasaki, S., Yanagida, M. (2018). S-Adenosylmethionine synthetase is required for cell growth, maintenance of G0 phase, and termination of quiescence in fission yeast. iScience 5, 38–51. doi: 10.1016/j.isci.2018.06.011
Hughey, C. C., Trefts, E., Bracy, D. P., Wasserman, D. H., Donahue, E. P., James, F. D. (2018). Glycine N-methyltransferase deletion in mice diverts carbon flux from gluconeogenesis to pathways that utilize excess methionine cycle intermediates. J. Biol. Chem. 293, 11944–11954. doi: 10.1074/jbc.RA118.002568
Katoh, Y., Ikura, T., Hoshikawa, Y., Tashiro, S., Ito, T., Ohta, M., et al. (2011). Methionine adenosyltransferase II serves as a transcriptional corepressor of Maf oncoprotein. Mol. Cell. 41, 554–566. doi: 10.1016/j.molcel.2011.02.018
Kera, Y., Katoh, Y., Ohta, M., Matsumoto, M., Takano-Yamamoto, T., Igarashi, K. (2013). Methionine adenosyltransferase II-dependent histone H3K9 methylation at the COX-2 gene locus. J. Biol. Chem. 288, 13592–13601. doi: 10.1074/jbc.M112.429738
Larsson, J., Zhang, J., Rasmuson-Lestander, Å. (1996). Mutations in the Drosophila melanogaster gene encoding S-adenosylmethionine suppress position-effect variegation. Genetics 143, 887–896.
Lee, J.-K., Kim, K.-C. (2013). DZNep, inhibitor of S-adenosylhomocysteine hydrolase, down-regulates expression of SETDB1 H3K9me3 HMTase in human lung cancer cells. Biochem. Biophys. Res. Commun. 438, 647–652. doi: 10.1016/j.bbrc.2013.07.128
Li, S., Swanson, S. K., Gogol, M., Florens, L., Washburn, M. P., Workman, J. L., et al. (2015). Serine and SAM responsive complex SESAME regulates histone modification crosstalk by sensing cellular metabolism. Mol. Cell. 60, 408–421. doi: 10.1016/j.molcel.2015.09.024
Li, X., Nai, S., Ding, Y., Geng, Q., Zhu, B., Yu, K., et al. (2017). Polo-like kinase 1 (PLK1)-dependent phosphorylation of methylenetetrahydrofolate reductase (MTHFR) regulates replication via histone methylation. Cell Cycle 16, 1933–1942. doi: 10.1080/15384101.2017.1363942
Lin, D., Chung, B. P., Kaiser, P. (2014). S-Adenosylmethionine limitation induces p38 mitogen-activated protein kinase and triggers cell cycle arrest in G1. J. Cell Sci. 127, 50–59. doi: 10.1242/jcs.127811
Liu, J. J., Ward, R. L. (2010). Folate and one-carbon metabolism and its impact on aberrant DNA methylation in cancer. Adv. Genet. 71, 79–121. doi: 10.1016/B978-0-12-380864-6.00004-3
Liu, M., Barnes, V., Pile, L. (2015). Disruption of methionine metabolism in drosophila melanogaster impacts histone methylation and results in loss of viability. G3 (Bethesda), 6 (1), 121–132. doi: 10.1523/JNEUROSCI.4349-14.2015
Locasale, J. W., Cantley, L. C. (2011). Metabolic flux and the regulation of mammalian cell growth. Cell Metab. 14, 443–451. doi: 10.1016/j.cmet.2011.07.014
Lu, C., Thompson, C. B. (2012). Metabolic regulation of epigenetics. Cell Metab. 16, 9–17. doi: 10.1016/J.CMET.2012.06.001
Luka, Z., Moss, F., Loukachevitch, L. V., Bornhop, D. J., Wagner, C. (2011). Histone demethylase LSD1 is a folate-binding protein. Biochemistry 50, 4750–4756. doi: 10.1021/bi200247b
Luka, Z., Mudd, S. H., Wagner, C. (2009). Glycine N-methyltransferase and regulation of S-adenosylmethionine levels. J. Biol. Chem. 284, 22507–22511. doi: 10.1074/jbc.R109.019273
Luka, Z., Pakhomova, S., Loukachevitch, L. V., Calcutt, M. W., Newcomer, M. E., Wagner, C. (2014). Crystal structure of the histone lysine specific demethylase LSD1 complexed with tetrahydrofolate. Protein Sci. 23, 993–998. doi: 10.1002/pro.2469
Luka, Z., Pakhomova, S., Loukachevitch, L. V., Egli, M., Newcomer, M. E., Wagner, C. (2007). 5-Methyltetrahydrofolate is bound in intersubunit areas of rat liver folate-binding protein glycine N-methyltransferase. J. Biol. Chem. 282, 4069–4075. doi: 10.1074/jbc.M610384200
Ly, A., Hoyt, L., Crowell, J., Kim, Y.-I. (2012). Folate and DNA Methylation. Antioxid. Redox Signal. 17, 302–326. doi: 10.1089/ars.2012.4554
MacFarlane, A. J., Perry, C. A., Girnary, H. H., Gao, D., Allen, R. H., Stabler, S. P., et al. (2009). Mthfd1 is an essential gene in mice and alters biomarkers of impaired one-carbon metabolism. J. Biol. Chem. 284, 1533–1539. doi: 10.1074/jbc.M808281200
Margueron, R., Justin, N., Ohno, K., Sharpe, M. L., Son, J., Drury, W. J., III, et al. (2009). Role of the polycomb protein EED in the propagation of repressive histone marks. Nature 461, 762–767. doi: 10.1038/nature08398
Mehedint, M. G., Niculescu, M. D., Craciunescu, C. N., Zeisel, S. H. (2010). Choline deficiency alters global histone methylation and epigenetic marking at the Re1 site of the calbindin 1 gene. FASEB J. 24, 184–195. doi: 10.1096/fj.09-140145
Mentch, S. J., Mehrmohamadi, M., Huang, L., Liu, X., Gupta, D., Mattocks, D., et al. (2015). Histone methylation dynamics and gene regulation occur through the sensing of one-carbon metabolism. Cell Metab. 22, 861–873. doi: 10.1016/j.cmet.2015.08.024
Miller, J. W., Nadeau, M. R., Smith, J., Smith, D., Selhub, J. (1994). Folate-deficiency-induced homocysteinaemia in rats: disruption of S-adenosylmethionine’s co-ordinate regulation of homocysteine metabolism. Biochem. J. 298(Pt 2), 415–419. doi: 10.1042/bj2980415
Miranda, T. B., Cortez, C. C., Yoo, C. B., Liang, G., Abe, M., Kelly, T. K., et al. (2009). DZNep is a global histone methylation inhibitor that reactivates developmental genes not silenced by DNA methylation. Mol. Cancer Ther. 8, 1579–1588. doi: 10.1158/1535-7163.MCT-09-0013
Morgan, H. D., Sutherland, H. G. E., Martin, D. I. K., Whitelaw, E. (1999). Epigenetic inheritance at the agouti locus in the mouse. Nat. Genet. 23, 314–318. doi: 10.1038/15490
Muskiet, F. A., Velzing-Aarts, F. V., Fokkema, M. R., Ueland, P. M., Holm, P. I., van der Dijs, F. P. (2018). Plasma choline and betaine and their relation to plasma homocysteine in normal pregnancy. Am. J. Clin. Nutr. 81, 1383–1389. doi: 10.1093/ajcn/81.6.1383
Nottke, A., Colaiacovo, M. P., Shi, Y. (2009). Developmental roles of the histone lysine demethylases. Development 136, 879–889. doi: 10.1242/dev.020966
Obata, F., Kuranaga, E., Tomioka, K., Ming, M., Takeishi, A., Chen, C.-H., et al. (2014). Necrosis-driven systemic immune response alters SAM metabolism through the FOXO-GNMT axis. Cell Rep. 7, 821–833. doi: 10.1016/j.celrep.2014.03.046
Obeid, R., Herrmann, W. (2009). Homocysteine and lipids: S-adenosyl methionine as a key intermediate. FEBS Lett. 583, 1215–1225. doi: 10.1016/j.febslet.2009.03.038
Patnaik, D., Chin, H. G., Estève, P.-O., Benner, J., Jacobsen, S. E., Pradhan, S. (2004). Substrate specificity and kinetic mechanism of mammalian G9a histone H3 methyltransferase. J. Biol. Chem. 279, 53248–53258. doi: 10.1074/jbc.M409604200
Pedersen, M. T., Helin, K. (2010). Histone demethylases in development and disease. Trends Cell Biol. 20, 662–671. doi: 10.1016/j.tcb.2010.08.011
Rush, E. C., Katre, P., Yajnik, C. S. (2014). Vitamin B12: one carbon metabolism, fetal growth and programming for chronic disease. Eur. J. Clin. Nutr. 68, 2–7. doi: 10.1038/ejcn.2013.232
Sadhu, M. J., Guan, Q., Li, F., Sales-Lee, J., Iavarone, A. T., Hammond, M. C., et al. (2013). Nutritional control of epigenetic processes in yeast and human cells. Genetics 195, 831–844. doi: 10.1534/genetics.113.153981
Sarg, B., Koutzamani, E., Helliger, W., Rundquist, I., Lindner, H. H. (2002). Postsynthetic trimethylation of histone H4 at lysine 20 in mammalian tissues is associated with aging. J. Biol. Chem. 277, 39195–39201. doi: 10.1074/jbc.M205166200
Sharma, U., Rando, O. J. (2017). Metabolic inputs into the epigenome. Cell Metab. 25, 544–558. doi: 10.1016/j.cmet.2017.02.003
Sidoli, S., Kori, Y., Lopes, M., Yuan, Z.-F., Kim, H. J., Kulej, K., et al. (2019a). One minute analysis of 200 histone post-translational modifications by direct injection mass spectrometry. Genome Res. 29, 978–987. doi: 10.1101/gr.247353.118
Sidoli, S., Trefely, S., Garcia, B. A., Carrer, A. (2019b). Integrated analysis of acetyl-CoA and histone modification via mass spectrometry to investigate metabolically driven acetylation. Methods Mol. Biol. 1928, 125–147. doi: 10.1007/978-1-4939-9027-6_9
Singhal, N. K., Li, S., Arning, E., Alkhayer, K., Clements, R., Sarcyk, Z., et al. (2015). Changes in methionine metabolism and histone H3 trimethylation are linked to mitochondrial defects in multiple sclerosis. J. Neurosci. 35, 15170–15186. doi: 10.1523/JNEUROSCI.4349-14.2015
Škovierová, H., Vidomanová, E., Mahmood, S., Sopková, J., Drgová, A., Červeňová, T., et al. (2016). The molecular and cellular effect of homocysteine metabolism imbalance on human health. Int. J. Mol. Sci. 17, 1–18. doi: 10.3390/ijms17101733
Soares, L., Buratowski, S. (2013). Histone crosstalk: H2Bub and H3K4 Methylation. Mol. Cell 49 (6), 1019–1020. doi: 10.1016/j.molcel.2013.03.012
Tan, J., Yang, X., Zhuang, L., Jiang, X., Chen, W., Puay, L. L., et al. (2007). Pharmacologic disruption of polycomb-repressive complex 2-mediated gene repression selectively induces apoptosis in cancer cells. Genes Dev. 21, 1050–1063. doi: 10.1101/gad.1524107
Taverna, S. D., Li, H., Ruthenburg, A. J., Allis, C. D., Patel, D. J. (2007). How chromatin-binding modules interpret histone modifications: lessons from professional pocket pickers. Nat. Struct. Mol. Biol. 14, 1025–1040. doi: 10.1038/nsmb1338
Tehlivets, O., Malanovic, N., Visram, M., Pavkov-Keller, T., Keller, W. (2013). S-adenosyl-L-homocysteine hydrolase and methylation disorders: yeast as a model system. Biochim. Biophys. Acta—Mol. Basis Dis. 1832, 204–215. doi: 10.1016/j.bbadis.2012.09.007
Teperino, R., Schoonjans, K., Auwerx, J. (2010). Histone methyl transferases and demethylases; can hey link metabolism and transcription? Cell Metab. 12, 321–327. doi: 10.1016/j.cmet.2010.09.004
Tong, X., Zhao, F., Thompson, C. B. (2009). The molecular determinants of de novo nucleotide biosynthesis in cancer cells. Curr. Opin. Genet. Dev. 19, 32–37. doi: 10.1016/j.gde.2009.01.002
Völker-Albert, M. C., Schmidt, A., Forne, I., Imhof, A. (2018). Analysis of histone modifications by mass spectrometry. Curr. Protoc. Protein Sci. 92, e54. doi: 10.1002/cpps.54
Wang, T., Pickard, A. J., Gallo, J. M. (2016). Histone methylation by Temozolomide; a classic DNA methylating anticancer drug. Anticancer Res. 36, 3289–3299.
Wang, Y.-C., Chen, Y.-M., Lin, Y.-J., Liu, S.-P., Chiang, E.-P. I. (2011a). GNMT expression increases hepatic folate contents and folate-dependent methionine synthase-mediated homocysteine remethylation. Mol. Med. 17, 486–494. doi: 10.2119/molmed.2010.00243
Wang, Y.-C., Tang, F.-Y., Chen, S.-Y., Chen, Y.-M., Chiang, E.-P. I. (2011b). Glycine-N methyltransferase expression in HepG2 cells is involved in methyl group homeostasis by regulating transmethylation kinetics and DNA methylation. J. Nutr. 141, 777–782. doi: 10.3945/jn.110.135954
Waterland, R. A., Jirtle, R. L. (2003). Transposable elements: targets for early nutritional effects on epigenetic gene regulation. Mol. Cell. Biol. 23, 5293–5300. doi: 10.1128/MCB.23.15.5293-5300.2003
Xiahou, Z., Zhao, H., Zhu, B., Zhao, H., Peng, B., Xu, X. (2014). MTHFR promotes heterochromatin maintenance. Biochem. Biophys. Res. Commun. 447, 702–706. doi: 10.1016/j.bbrc.2014.04.082
Yang, A., Jiao, Y., Yang, S., Deng, M., Yang, X., Mao, C., et al. (2018). Homocysteine activates autophagy by inhibition of CFTR expression via interaction between DNA methylation and H3K27me3 in mouse liver. Cell. Death Dis. 9, 1–14. doi: 10.1038/s41419-017-0216-z
Yudkoff, M. (2012). Disorders of amino acid metabolism. Basic Neurochem. 737–754. doi: 10.1016/B978-0-12-374947-5.00042-0
Zamierowski, M. M., Wagner, C. (1977). Identification of folate binding proteins in rat liver. J. Biol. Chem. 252, 933–938
Keywords: folate, s-adenosylmethionine, histone methlyation, one-carbon metabolism, methioine
Citation: Serefidou M, Venkatasubramani AV and Imhof A (2019) The Impact of One Carbon Metabolism on Histone Methylation. Front. Genet. 10:764. doi: 10.3389/fgene.2019.00764
Received: 17 March 2019; Accepted: 18 July 2019;
Published: 28 August 2019.
Edited by:
Carles Canto, Nestle Institute of Health Sciences (NIHS), SwitzerlandReviewed by:
Lori A. Pile, Wayne State University, United StatesCopyright © 2019 Serefidou, Venkatasubramani and Imhof. This is an open-access article distributed under the terms of the Creative Commons Attribution License (CC BY). The use, distribution or reproduction in other forums is permitted, provided the original author(s) and the copyright owner(s) are credited and that the original publication in this journal is cited, in accordance with accepted academic practice. No use, distribution or reproduction is permitted which does not comply with these terms.
*Correspondence: Magdalini Serefidou, bS5zZXJlZmlkb3VAZ21haWwuY29t
†These authors have contributed equally to this work.
Disclaimer: All claims expressed in this article are solely those of the authors and do not necessarily represent those of their affiliated organizations, or those of the publisher, the editors and the reviewers. Any product that may be evaluated in this article or claim that may be made by its manufacturer is not guaranteed or endorsed by the publisher.
Research integrity at Frontiers
Learn more about the work of our research integrity team to safeguard the quality of each article we publish.