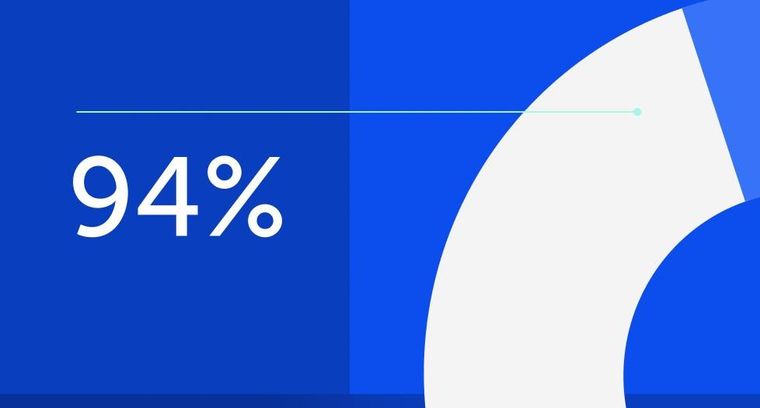
94% of researchers rate our articles as excellent or good
Learn more about the work of our research integrity team to safeguard the quality of each article we publish.
Find out more
REVIEW article
Front. Genet., 14 May 2019
Sec. Genetics of Aging
Volume 10 - 2019 | https://doi.org/10.3389/fgene.2019.00435
This article is part of the Research TopicProceedings of the International Conference on Interventions to Extend Healthspan and LifespanView all 8 articles
Aging is a general degenerative process related to deterioration of cell functions in the entire organism. Mitochondria, which play a key role in energy homeostasis and metabolism of reactive oxygen species (ROS), require lifetime control and constant renewal. This explains recently peaked interest in the processes of mitochondrial biogenesis and mitophagy. The principal event of mitochondrial metabolism is regulation of mitochondrial DNA (mtDNA) transcription and translation, which is a complex coordinated process that involves at least two systems of transcription factors. It is commonly believed that its major regulatory proteins are PGC-1α and PGC-1β, which act as key factors connecting several regulator cascades involved in the control of mitochondrial metabolism. In recent years, the number of publications on the essential role of Nrf2/ARE signaling in the regulation of mitochondrial biogenesis has grown exponentially. Nrf2 is induced by various xenobiotics and oxidants that oxidize some Nrf2 negative regulators. Thus, ROS, in particular H2O2, were found to be strong Nrf2 activators. At present, there are two major concepts of mitochondrial biogenesis. Some authors suggest direct involvement of Nrf2 in the regulation of this process. Others believe that Nrf2 regulates expression of the antioxidant genes, while the major and only regulator of mitochondrial biogenesis is PGC-1α. Several studies have demonstrated the existence of the regulatory loop involving both PGC-1α and Nrf2. In this review, we summarized recent data on the Nrf2 role in mitochondrial biogenesis and its interaction with PGC-1α in the context of extending longevity.
Mitochondria play a key role in the pathogenesis of many diseases (Wallace, 2005; Lin and Beal, 2006; Lindblom et al., 2015; Ratliff et al., 2016; Boengler et al., 2017) and aging (Jang et al., 2018). The number of mitochondria and the amount of mtDNA rapidly increase in the fetus, starting from the blastocyst stage (Pikó and Taylor, 1987) and continuing to grow over the entire period of organism development and maturation (Heerdt and Augenlicht, 1990). However, there is still no consensus on what happens to the number of mitochondria with aging, since the data obtained in different tissues, at different stages, and with different methods are extremely controversial.
A number of authors report an age-related increase of the amount of mtDNA in skeletal muscle (Barrientos et al., 1997; Onyango et al., 2010). Studies based on electron microscopy have not shown any age-related changes in the number of mitochondria in skeletal muscle (Mathieu-Costello et al., 2005; Callahan et al., 2014). However, Corsetti et al. (2008) showed a decrease of the number of mitochondrial copies in skeletal muscles of aged mice. Studies by Kerner et al. (2001) and Chabi et al. (2008) showed a decrease of the marker mitochondrial enzymes activity in skeletal muscle, and the authors concluded that the number of mitochondria decreases in aging. Opposite conclusions made by various research groups may be associated not only with different methods for determining the number of mitochondria, but also with structural, biochemical and functional heterogeneity of skeletal muscles (Hepple, 2014). Studies using electron microscopy showed a decrease of the number of mitochondria in the heart (Tate and Herbener, 1976; Corsetti et al., 2008), whereas others did not reveal any changes (Schmucker and Sachs, 1985). However, there is no doubt that the area of the inner mitochondrial membrane decreases with age in heart (Sachs et al., 1977; Riva et al., 2006). In the brain, age-related decrease of the number of mitochondria was found using electron microscopy (Burns et al., 1979), enzymatic methods (Genova et al., 1997; Lenaz et al., 2000; Dencher et al., 2007) and estimation of expression of genes encoded by mtDNA (Ojaimi et al., 1999; Gureev et al., 2016).
We should emphasize that it is not only the number of mitochondria that is important, but also their functional state, which depends on mitochondrial biogenesis and dynamics, including fission/fusion and mitophagy. The dysregulation of these processes leads to age-related decrease in mitochondrial volume density and oxidative capacity per mitochondrial volume (Conley et al., 2000). The coordination between these processes is controlled by several mutually regulated signaling cascades, one of the most important being the Nrf2/ARE cascade (Holmström et al., 2016). Despite a growing interest in this signaling pathway, there are only a few reviews on the key role of Nrf2 in mitochondrial biogenesis and its interactions with the more studied “master regulator of mitochondrial biogenesis” PGC-1α (Ryoo and Kwak, 2018).
Mammalian mtDNA is a circular DNA molecule of approximately 16.5 kb that possesses its own translational/transcriptional system, including 2 rRNA genes and 22 tRNA genes. It also has the non-coding region, the so-called D-loop, that contains the mtDNA replication origin and the transcription initiation site (Clayton, 2000).
Replication is performed by the mtDNA polymerase γ (POLG) consisting of the catalytic subunit encoded by the POLG gene and auxiliary dimeric subunit encoded by the POLG2 gene (Graziewicz et al., 2006). mtDNA is transcribed by the mitochondrial RNA polymerase POLRMT (Tiranti et al., 1997). The key enhancer protein is TFAM (transcription factor A, mitochondrial), which ensures mtRNA unwinding and flexing required for the POLRMT binding to the mtDNA promoters. TFB2M (transcription factor B2, mitochondrial) acts as a specific dissociation factor that provides interaction between POLRMT and TFAM. Both TFB1M and TFB2M bind rRNA dimethyltransferases and, therefore, can function as rRNA modifiers (Rebelo et al., 2011). It was suggested that the major role of TFB1M is rRNA methylation and not its transcription factor function (Metodiev et al., 2009).
Nuclear respiratory factors NRF1 and NRF2 regulate expression of the electron transfer chain (ETC) subunits encoded by the nuclear genome (Evans and Scarpulla, 1990) and bind to the promoters of genes involved in mtDNA transcription. NRF1 binds to the specific promoter sites and regulates expression of TFAM (Virbasius and Scarpulla, 1994), TFB1M, and TFB2M (Gleyzer et al., 2005). Besides, nuclear respiratory factors, in particular NRF2, regulate expression of other mitochondrial enzymes, e.g., TOMM20 (translocase outer mitochondrial membrane), a key enzyme in the mitochondrial membrane transport (Blesa and Hernández-Yago, 2006). In turn, NRF1 and NRF2 are regulated by transcription coactivators, the most studied of which is PGC-1α (Scarpulla, 2008).
PGC-1α was discovered as a coregulator of PPARγ expressed in the brown fat at low temperatures that mediates adaptive thermogenesis (hence the name PGC-1α—PPAR-Gamma-Coactivator-1α). Later, it was found that PGC-1α acts as a coactivator for a much larger number of genes. It was demonstrated that PGC-1α interacts with both NRF1 and NRF2. Deletion of the N-terminal fragment in NRF1 abolishes the PGC-1α effect on mitochondrial biogenesis (Wu et al., 1999).
PGC-1α is regulated on both the transcription and post-translation levels (Fernandez-Marcos and Auwerx, 2011). Cold exposure activates the sympathetic nervous system through β3-adrenergic receptor (β3-AR), which contributes the activation of protein kinase A (PKA). PKA activates CREB, which regulates the expression of PGC-1α directly (Puigserver et al., 1998; Boss et al., 1999; Herzig et al., 2001). p38 MAPK is another factor that regulates the expression of PGC-1α. It activates myocyte enhancer factor 2 (MEF2), which has the site of binding with the promoter of PGC-1α (Handschin et al., 2003). MEF2 can activate p38 mitogen-activated protein kinase (p38 MAPK) in skeletal muscle (Zhao et al., 1999). Additionally, p38 MAPK can regulate the expression of PGC-1α by activating transcription factor 2 (ATF2) (Akimoto et al., 2005). An increase in the intracellular Ñà2+ concentration in skeletal muscle activates Ñà2+/calmodulin-dependent protein kinase (CaMK), which activates the expression of PGC-1α via CREB (Ojuka, 2004) (Figure 1).
AMP-activated protein kinase (AMPK) is the major factor of PGC-1α post-translation modification for a wide range of tissue. PGC-1α phosphorylation is only one of the mechanisms of mitochondrial biogenesis activation via AMPK (Jäger et al., 2007). AMPK can increase the level of NAD+, which results in SIRT1 phosphorylation. Activated SIRT1 deacetylates PGC-1α, thereby promoting mitochondrial biogenesis (Cantó et al., 2009). p38 MAPK cannot only regulate the expression of PGC-1α, but also phosphorylates and so activates PGC-1α (Puigserver et al., 2001). Another activator of PGC-1α is cGMP, which is upregulated due to the increase in the concentration of nitric oxide (NO) produced by NO synthases iNOS and eNOS (Nisoli et al., 2003) (Figure 1).
Phosphorylation of PGC-1α can also lead to its negative regulation. PGC-1α is phosphorylated by glycogen synthase kinase 3β (GSK3β), which inhibits PGC-1α and contributes to its intranuclear proteasomal degradation (Anderson et al., 2008). However, negative regulation of PGC-1α is carried out because of acetylation to a greater extent. GCN5 (general control of amino acid synthesis 5) is one of the most important molecules that carry out the acetylation of PGC-1α, which subsequently deacetylates by SIRT1 (Rodgers et al., 2005).
PGC-1β does not have such a wide range of action as PGC-1α, in particular it is not activated in brown fat upon cold exposure (Meirhaeghe et al., 2003). However, PGC-1β regulates the expression of NRF1 and mitochondrial biogenesis (Lin et al., 2002). The level of mitochondrial biogenesis is extremely high in transgenic mice, overexpressing PGC-1β, which even leads to the disorganization of the myofibrillar apparatus (Arany et al., 2007).
ARE (antioxidant response element) is a promoter element in various genes. The major activator of ARE was named Nrf2 (NF-E2-p45-related factor 2, encoded by NFE2L2 gene) (Giudice et al., 2010). Nrf2 localizes to the cytoplasm, where it binds the specific inhibitor Keap1. In the absence of activation, Nrf2 is ubiquitinated by the E3-ubiquitin ligase-like domain of Keap1 and degraded by the 26S proteasome (Zhang and Hannink, 2003); therefore, Keap1 acts as a negative regulator of Nrf2. Nrf2 activation requited oxidation of SH-groups in Keap1 (Kansanen et al., 2013). Free Nrf2 is translocated to the nucleus, where it forms a heterodimer with the small protein Maf and binds to AREs in the target gene promoters. In most cases, these are genes coding for proteins with cytoprotective properties, e.g., antioxidant enzymes, proteins of phase II xenobiotic detoxication, and antiinflammatory enzymes. Nrf2 also regulates expression of genes involved in the regulation of redox homeostasis and a number of metabolic enzymes (Dinkova-Kostova and Abramov, 2015).
Another well-described Nrf2 repressor is GSK3β. Unlike most protein kinases, GSK3β is active under non-stress conditions and can phosphorylate Nrf2, thereby suppressing its translocation to the nucleus. However, GSK3β-induced suppression of Nrf2 can be abolished by Akt/PKB that inhibits GSK3β (Tebay et al., 2015). The E3 ubiquitin ligase Hrd1 is the third negative regulator of Nrf2, which contributes to its ubiquitination and degradation (Wu et al., 2014).
The idea that the Nrf2/ARE signaling cascade is involved in mitochondrial biogenesis is relatively new. Only in Piantadosi et al. (2008) for the first time suggested the role of Nrf2 in the activation of mitochondrial biogenesis. The powerful incentive for the development of this field of research was the discovery of four AREs in the NRF1 gene promoter that were capable of Nrf2 binding. The CO-stimulated production of H2O2 results in PTEN oxidation, leading to Akt/PKB activation. Akt phosphorylates and inactivates GSK3β, thereby promoting Nrf2 translocation to the nucleus. In the nucleus, Nrf2 binds to the NRF1 promoter AREs. NRF1 activates TFAM, which is directly involved in the mtDNA replication (Figure 1).
Later, Akt phosphorylation with the following activation of the Nrf2-dependent mitochondrial biogenesis was demonstrated in heart failure treatment (Calvert et al., 2010). CO has a therapeutic effect on Staphylococcus aureus-caused sepsis because of the increase of expression levels of HO-1, both nuclear respiratory factors (NRF1 and NRF2) and TFAM. This effect was detected neither in Nrf2-deficient nor in Akt-deficient mice (MacGarvey et al., 2012).
Different Nrf2 activators were found to regulate mitochondrial biogenesis in different organs. Dimethyl fumarate disrupts the interaction between Keap1 and Nfr2 by alkylating cysteine residues in Keap1, which results in Nrf2 translocation to the nucleus and activation of mitochondrial biogenesis in multiple sclerosis (Hayashi et al., 2017) and MPTP-induced model of Parkinson’s disease (Ahuja et al., 2016). Quercetin and acetyl-L-carnitine induce Nrf2-dependent mitochondrial biogenesis in traumatic brain injury (Li et al., 2016) and hypoxia (Hota et al., 2012), respectively. Methylene blue (Gureev et al., 2016) and β-guanidinopropionic acid (Gureev et al., 2018) promote mitochondrial biogenesis in the brain of middle-aged (15-month-old) mice by inducing mild oxidative and energy stress in the mitochondria. Sulforaphane increases the number of mitochondria in cancer cells (Negrette-Guzmán et al., 2017). The α7-nicotinic acetylcholine receptor agonist PNU282987 activates mitochondrial biogenesis in cultured glial cells (Navarro et al., 2017). Moderate physical exercise induces Nrf2-dependent mitochondrial biogenesis in muscles (Merry and Ristow, 2016; Zoladz et al., 2017) and in the striatum in the 6-OHDA-induced model of parkinsonism (Aguiar et al., 2016). Nrf2-dependent mitochondrial biogenesis is activated during tissue regeneration after burn trauma (Bohanon et al., 2018). Downregulation of the Nox4 gene expression also promotes Nrf2-dependent mitochondrial biogenesis (Bernard et al., 2017) (Table 1).
The key role of Nrf2 in mitochondrial biogenesis has also been demonstrated using Nrf2-deficient animal models. In contrast to the wild-type animals, Nrf2 activators did not increase the amounts of mitochondrial markers in the Nrf2−/− mutants (Athale et al., 2012; MacGarvey et al., 2012; Chen et al., 2014; Ahuja et al., 2016; Navarro et al., 2017). It was found that Nrf2 deficiency impairs mitochondrial biogenesis in the intestine of Nrf2−/− mice (Chen et al., 2014).
A decrease in the intensity of mitochondrial biogenesis due to impaired Nrf2 translocation to the nucleus was observed in the gentamycin-induced nephrotoxicity (Negrette-Guzmán et al., 2015), hypertonia caused by injection of low doses of lipopolysaccharide (Wu K.L.H. et al., 2016), multiple sclerosis (Hayashi et al., 2017), and in placentas of women suffering from diabetes combined with obesity (Duan et al., 2018).
It was found that Nrf2 translocation to the nucleus is strictly regulated by the activity of AMPK. AMPK phosphorylates Nrf2 at Ser50, which results in GSK3β inactivation. Both processes are essential for Nrf2 translocation to the nucleus (Joo et al., 2016). It was demonstrated that berberine (Mo et al., 2014), aldose reductase inhibitor fidarestat (Shukla et al., 2017), and pterostilbene (antioxidant from blueberries) (Kosuru et al., 2018) activate Nrf2, and this activation is controlled by AMPK. AMPK inhibition abolishes Nrf2 activation (Wang et al., 2016).
It is most probable that Nrf2 is indeed controlled by PGC-1α. Aquilano et al. (2013) showed that PGC-1α controls the antioxidant genes through Nrf2 activation; thus, downregulation of PGC-1α expression almost completely inhibits Nrf2 binding to the GCLC gene ARE and decreased the content of SOD2 and GCL proteins. Mice heterozygous by PGC-1α (PGC-1α+/−) exhibit downregulated expression of SOD2 because of the disrupted Nrf2 interaction with the SOD2 gene ARE (Cherry et al., 2014). It was found that PGC-1α knockout dysregulates the Nrf2-dependent mitochondrial biogenesis (Navarro et al., 2017), although the mechanism of direct interaction between PGC-1α and Nrf2 remained unclear until recently. Choi et al. (2017) demonstrated that PGC-1α activates Nrf2 via inhibition of GSK3β. GSK3β is inactivated by p38, which is positively regulated by PGC-1α. Therefore, the PGC-1α/p38/GSK3β/Nrf2 cascade is the most probable pathway connecting these two coregulators of mtDNA transcription.
It is also possible that Nrf2 and PGC-1α form the feedback loop, i.e., Nrf2 directly influences PGC-1α expression. The PGC-1α gene promoter contains two AREs: −1723 (5′-TCTTGATGACATTGCTTCTG-3′) and −226 (5′-CTGATTTGATGGAGCTACTT-3′) (Baldelli et al., 2013). There are data that confirm the existence of the Nrf2/PGC-1α feedback loop. Thus, the siRNA-mediated suppression of Nrf2, as well as Nrf2 knockout, inhibit mitochondrial biogenesis and downregulate PGC-1α expression in hepatocytes (Joe et al., 2015), skeletal muscles (Whitman et al., 2013), and lungs infected with S. aureus (Athale et al., 2012).
There is evidence that both signaling cascades could be activated simultaneously. Metformin activates Nrf2 without involvement of the AMPK/PGC-1α axis, since inhibition of AMPK phosphorylation does not prevent metformin-induced Nrf2 activation (Prasad et al., 2017). It is, however, commonly believed that the major effect of metformin is AMPK activation due to the changes in the AMP/ATP ratio (Pawlyk et al., 2014).
Nrf2 and PGC-1α can be simultaneously activated via the Erk1/2 signaling cascade. Erk1/2 activates both Nrf2 and PGC-1α via phosphorylation of LKB1, which in turn phosphorylates AMPK (Hota et al., 2012). Interestingly, some commonly used pharmaceutical agents, e.g., erythropoietin (EPO), regulate the antioxidant defense via the Erk1/2/Nrf2/ARE axis (Genc et al., 2010; Jin et al., 2011; Wu et al., 2017). On the other hand, it was found that EPO activates mitochondria biogenesis through the Akt/eNOS/PGC-1α (Carraway et al., 2010; Qin et al., 2013) and AMPK/PGC-1α (Wang et al., 2013) pathways. Therefore, EPO can potentially activate both the Nrf2 and PGC-1α cascades. Moreover, both cascades have a common point: Akt phosphorylation can activate Nrf2 via GSK3β phosphorylation (Piantadosi et al., 2008) and directly activate eNOS (Tengan et al., 2012). Methylene blue is another compound which contributes to activation of mitochondrial biogenesis through Nrf2 by stimulation of H2O2 production (Gureev et al., 2016) and changing the relation NAD+/NADH, which leads to PGC-1α activation through phosphorylation by AMPK (Atamna et al., 2015).
An important study on the relation between PGC-1α and Nrf2 in mitochondrial biogenesis was published by Merry and Ristow (2016). It is known that physical exercise induces formation of reactive oxygen species (ROS) (mostly, H2O2) and nitric oxide (NO). The authors demonstrated that treatment of Nrf2−/− cells with the NO and H2O2 donors failed to cause mitochondrial biogenesis activation. siRNA-mediated suppression of PGC-1α prevented NO-induced, but not H2O2-induced, activation of mitochondrial biogenesis (Merry and Ristow, 2016). Therefore, even in the case of the same event (physical exercise), mitochondrial biogenesis can be activated by different mechanisms involving different secondary messengers (Ca2+, NO, H2O2) (Figure 1). Both Nrf2 and PGC-1α were found to be activated simultaneously during mitochondrial biogenesis induced by exercise (Zoladz et al., 2017) and burn trauma (Bohanon et al., 2018).
Simultaneous activation of Nrf2 and PGC-1α pathways may be one of the most promising directions in gerontoprotection. In general, it relates with the fact that these two transcription factors in sum are able to regulate almost all aspects related to the functioning of mitochondria.
The major postulates of the aging theory were published by Harman over 50 years ago. He stated that an increase in the intensity of free-radical processes with age results in the accumulation of oxidative damage and tissue degeneration, i.e., aging (Harman, 2009). Since then, the theory expanded because of new experimental data that do not refute, but modify and refine this theory. There is widespread agreement that one of symptoms of aging is the accumulation of damaged mitochondria (López-Otín et al., 2013; Stefanatos and Sanz, 2018). For this reason, the theory of a mitochondrial “vicious cycle” became a logical continuation of Harman’s theory. Age-related increase of ROS production causes an increase of the frequency of mtDNA mutations, and they lead to ETC dysfunction, which leads to even more ROS production (Wei, 1998). Despite the logicality of this theory, it is not fully supported by experimental data (Stefanatos and Sanz, 2018). In particular, the initiation of mtDNA mutations does not cause an increase of the rate of ROS production, although it led to disruption of the functioning of the respiratory complexes, inhibition of membrane potential generation and ATP synthesis (Hiona et al., 2010). Ubiquinone depletion leads to impaired mitochondrial function, but on the contrary leads to a decrease of the rate of H2O2 production (Wang et al., 2015). These studies suggest that among all age-related mitochondrial dysfunctions, an increase of ROS production is not the most influential.
An age-related decrease of the activity of the mitochondrial respiratory chain complexes was observed almost in all studies. It is noteworthy that there is a decrease in the activity of not all components, but mainly NADH dehydrogenase (Genova et al., 1997; Navarro and Boveris, 2004; Rygiel et al., 2014) and COX (Navarro and Boveris, 2004; Ferguson et al., 2005). The decrease of the COX activity is associated with a shift of the ETC redox balance toward over-reduction. It stimulates electron leakage from ETC and subsequent ROS hyperproduction. A decrease of the complex I activity, on the contrary, may lead to a decrease of ROS production, which also has negative consequences (Stefanatos and Sanz, 2018). ROS, in particular H2O2, are important signaling molecules involved in the inactivation of negative regulators of Nrf2. An age-related decline of ROS production can lead to dysregulation of the feedback system of adaptive responses, including those by Nrf2 (Zhang H. et al., 2015).
Thus, age-related changes in ROS metabolism can cause damage to mtDNA, but mainly due to an imbalance between mitochondrial biogenesis and mitophagy. Both these processes are closely related to the Nrf2/ARE signal pathway (Piantadosi et al., 2008; Murata et al., 2015). The mtDNA mutations result from a violation of mtDNA replication and repair (Krishnan et al., 2008), as well as mitophagic dysfunction (Fivenson et al., 2017). In aging organisms, the ability of the Nrf2/ARE cascade to regulate mitochondrial biogenesis is diminished, as it has been demonstrated in rat kidneys (Pokkunuri et al., 2016) and mouse brain (Gureev et al., 2016). On the other hand, various tested chemical compounds displayed their pharmacological effects only in old rodents, which might indicate that the system of adaptive responses in young animals does not require additional external stimuli. It was found that the geroprotective effects of EPO (Wu et al., 2017), Methylene blue (Gureev et al., 2016), grape powder (Pokkunuri et al., 2016), and β-guanidinopropionic acid (Gureev et al., 2018) can be related to the Nrf2 cascade activation. Several studies demonstrated a decrease in the functional activity of Nrf2 in various organs during aging (Suh et al., 2004; Shih and Yen, 2007; Duan et al., 2009; Ungvari et al., 2011).
The SKN-1 (Skinhead-1) protein is an Nrf2 ortholog in the Caenorhabditis elegans nematode, the most commonly used object for studying longevity. SKN-1 is activated by various external stimuli, including oxidative stress, and induces signaling cascades regulating mitochondrial biogenesis and mitophagy resulting in the renewal of mitochondria and metabolism normalization. In combination with normalization of the mitochondria antioxidant status, coordinated regulation of mitochondrial biogenesis and mitophagy might increase the lifespan of C. elegans (Palikaras et al., 2015). The increase of life expectancy because of maintaining of mitochondrial homeostasis connected with the modulation of mitochondrial biogenesis and mitophagy was shown for tomatidine, activating SCN-1 (Fang et al., 2017). D-β-Hydroxybutyrate increased the activity of ETC complexes because of SCN-1 activation, which can also indirectly testify to the activation of mitochondrial biogenesis (Edwards et al., 2014). However, most of the investigations were focused on gerontoprotection via SKN-1 because of decreasing of oxidative stress and increasing of antioxidant defense. It was demonstrated for hydralazine (Dehghan et al., 2017), resveratrol and its derivatives (Fischer et al., 2017), pyrroloquinoline (Wu J.Z. et al., 2016), vitamin D3 (Mark et al., 2016), catalpol (Seo et al., 2015), curcumin (Liao et al., 2011), as well as S-allylcysteine, S-allylmercaptocysteine (Ogawa et al., 2016), and diallyl trisulfide from garlic extract (Powolny et al., 2011). SKN-1 can also be activated by mild oxidative stress induced by low arsenite doses (Schmeisser et al., 2013).
There are no studies showing the relationship between Nrf2 and the insect’s mitochondrial biogenesis at the moment. Nevertheless, there are data showing that the inhibition of GSK-3β (Castillo-Quan et al., 2016) and Keap1 loss of function (Sykiotis and Bohmann, 2008) cause lifespan extension.
The PGC-1α pathway is also involved in the process of age-related violations of mitochondrial biogenesis. Response of PGC-1α to exercise training decreases in old rats (Derbré et al., 2012). PGC-1α regulators, such as AMPK, SIRT1 and others, are also negatively regulated in the process of aging. The level of SIRT1 decreases in the microglia of old mice (Cho et al., 2015). Gong et al. (2014) showed the strongest age-dependent decrease of SIRT1 level in brain. SIRT1 levels in liver, skeletal muscle and white adipose tissue change less (Gong et al., 2014). The activation of AMPK with the help of pharmacological agents and physical exercise was blunted in the skeletal muscle of old rats (Reznick et al., 2007).
Lin et al. (2005) reported that PGC-1α structural and functional homologs were not found for several lower organisms, for example for worm, fly and yeasts. Later, dPGC-1/Spargel—a structural homolog of PGC-1α—was found for Drosophila melanogaster (Gershman et al., 2007). The importance of dPGC-1 in D. melanogaster mitochondrial biogenesis is shown in numerous studies (Tiefenböck et al., 2010; Rera et al., 2011; Mukherjee et al., 2014; Zhang F. et al., 2015; Wei et al., 2018). dPGC-1-related mitochondrial biogenesis contributes to longevity in Indy (I’m Not Dead Yet) mutant flies (Rogers and Rogina, 2014). Meldonium increased lifespan and survival rate in fly Huntington disease models via upregulation of the dPGC-1 gene (Di Cristo et al., 2018). Silencing of dPGC-1 promotes Parkinsonian phenotypes in flies (Merzetti and Staveley, 2015; Ng et al., 2017).
Aging is one of the factors mediating the development of a broad range of neurodegenerative diseases. The structures of the extrapyramidal system, in particular front departments of substantia nigra pars compacta, are mainly affected in Parkinson’s disease (Lang and Lozano, 1998). Mitochondrial biogenesis is inhibited during Parkinson’s disease (Thomas et al., 2012). For this reason, both Nrf2 and PGC-1α are able to be targets for therapy or slowdown of Parkinson’s disease pathogenesis. It was shown that moderate physical exercises activate Nrf2-dependent mitochondrial biogenesis, which improves Parkinson’s disease symptoms in MPTP (Tsou et al., 2015) and 6-OHDA models (Aguiar et al., 2016). Dimethyl fumarate and monomethyl fumarate contributed to the treatment of MPTP-induced Parkinson’s disease by activating Nrf2-dependent biogenesis (Ahuja et al., 2016). The hereditary form of Parkinson’s disease is primarily associated with mutations in the genes encoding PARKIN and PINK1 proteins (PTEN induced kinase 1), which mediate mitophagy (Geisler et al., 2010). There is a direct connection between Nrf2 and PINK1 as Nrf2 can regulate the expression of PINK1, because 4 ARE regions were detected in the promoter of this gene (Murata et al., 2015). Nrf2 regulates expression of p62/SQSTM1, which acts as an adapter molecule, which then provides interaction of ubiquitinated molecules directly with the autophagosome (Jain et al., 2010). Dimethyl fumarate (Lastres-Becker et al., 2016) and the CCCP (carbonyl cyanide m-chlorophenylhydrazone) (Ivankovic et al., 2016) support p62/SQSTM1-dependent mitophagy by activating Nrf2 and contribute to Parkinson’s disease therapy. At the moment, Inosin (urate precursor—Nrf2 activator) is on the 3rd stage of clinical trials for the treatment of Parkinson’s disease (NCT02642393).
The level of PGC-1α protein decreases during Parkinson diseases in patients (Thomas et al., 2012) and in SH-SY5Y neuroblastoma cells exposed by MPP+ (Zeissler et al., 2016). PGC-1α null mice are much more sensitive to the neurodegenerative effects of MPTP (St-Pierre et al., 2006). The protein PARIS (ZNF746) functions as a repressor because of the KRAB domain and repress the activity of PGC-1α (Shin et al., 2011). The activity of PARIS in normal functioning neurons is repressed by Parkin (Castillo-Quan, 2011), which has ubiquitin Å3-ligase activity (Tanaka et al., 2004). Overexpression of PARIS in the absence of gene Parkin activity causes a decrease of the activity of PGC-1α-dependent mitochondrial biogenesis and a decrease of the mitochondrial number. These effects were not observed after PARIS knockout (Stevens et al., 2015). Also, compounds such as metformin and glitazone can significantly decrease the risk of Parkinson’s disease in diabetes patients via AMPK and PGC-1α (Wahlqvist et al., 2012; Brauer et al., 2015).
The main cause of Alzheimer’s disease is taupathy (hyperphosphorylation of tau protein with the formation of neurofibrillary tangles) and the accumulation of beta-amyloid plaques (Aβ). These processes are accompanied by extensive oxidative stress and neuroinflammation, so it is logical that Nrf2 is involved in these processes (Bahn and Jo, 2019). Nrf2 activators such as Puerarin (Zhou et al., 2014), Triterpenoid CDDO-methylamide (Dumont et al., 2009), β-hydroxybutyrate (Xie et al., 2015) and others contributed to lowering the level of Aβ and improving rodent cognitive parameters (Bahn and Jo, 2019). The effect of Nrf2-dependent reduction of hyperphosphorylated tau was shown for substances such as fisetin (Kim et al., 2016), benfotiamine (Tapias et al., 2018) and dimethyl fumarate (Cuadrado et al., 2018). It was shown that it is possible to reduce the level of Aβ and hyperphosphorylated Tau protein simultaneously for methylene blue (Sun et al., 2016; Zakaria et al., 2016), sulforaphane (Kim et al., 2013) and allicin (Zhu et al., 2015). Methylene blue is currently undergoing clinical trials for the treatment of Alzheimer’s disease (NCT02380573).
The role of PGC-1α during Alzheimer’s disease is very ambiguous. On the one hand, the level of PPARGC1a expression in postmortem tissues and therapeutic preservation of neuronal PPARGC1a expression is able to prevent accumulation of Aβ (Qin et al., 2009). On the other hand, PPARGC1a overexpression can exacerbate Aβ and hyperphosphorylated tau deposition in mice model of Alzheimer’s disease (Dumont et al., 2014).
Another neurodegenerative disease is Huntington’s disease. It is characterized by progressive choreic hyperkinesis and mental disorders caused by striatum atrophy (Joshi and Johnson, 2012). Nrf2 is a promising target for its treatment. Inhibitors of complex II (3-nitropropionic acid and malonate) can damage its structure and form the symptoms observed during Huntington’s disease. Nrf2-deficient mice are more susceptible to inhibitors of complex II, while overexpression of Nrf2, in contrast, protected neurons from the toxicity of malonate (Calkins et al., 2005). Compounds such as synthetic triterpinoids (Stack et al., 2010) and dimethyl fumarate (Ellrichmann et al., 2011) can improve the symptoms of the disease by Nrf2 activating. PGC-1α is also likely to be involved in the pathogenesis of Huntington’s disease. Mutant huntingtin represses PPARGC1a gene transcription (Cui et al., 2006). Huntington’s disease patients are also characterized by a decrease of PGC-1α levels (Weydt et al., 2006), but it is still not clear if this can be used as therapeutic target for the treatment of Huntington’s disease.
Thus, it can be concluded that Nrf2 activation is the most promising direction in the field of neurodegenerative disease therapy. Firstly, it provides antioxidant protection. Reducing the level of oxidative stress can significantly improve the clinical picture of diseases. Secondly, the maintenance of mitochondrial homeostasis, associated with the regulation of the number and functionality of mitochondria, can inhibit degenerative processes in the nervous tissue, significantly slowing the rate of onset of the disease. And although neurodegeneration is considered to be a severe incurable disease, it is believed that slowing down their pathogenesis is a real task, the solution of which will improve the condition of patients, prolong active longevity and delay the terminal phase of the disease as much as possible.
ES organized the article search and preliminary analysis. AG wrote the manuscript. VP revised it critically for important intellectual content. All authors contributed to a manuscript revision.
This research was supported by the Ministry of Education and Science of the Russian Federation (State Assessment No. 6.4656.2017/8.9) to VP, Russian Foundation for Basic Research (No. 19-44-360011 p_a) to VP, a President grant for supporting of leading scientific school (Agreement No. 14.Z57. 18.3451-NSh) to VP, and partly supported by a “Skoltech Systems Biology Fellowship” (1-10-1202) to AG.
The authors declare that the research was conducted in the absence of any commercial or financial relationships that could be construed as a potential conflict of interest.
Aguiar, A. S. Jr., Duzzioni, M., Remor, A. P., Tristão, F. S., Matheus, F. C., Raisman-Vozari, R., et al. (2016). Moderate-intensity physical exercise protects against experimental 6-hydroxydopamine-induced hemiparkinsonism through Nrf2-antioxidant response element pathway. Neurochem. Res. 41, 64–72. doi: 10.1007/s11064-015-1709-8
Ahuja, M., Ammal Kaidery, N., Yang, L., Calingasan, N., Smirnova, N., Gaisin, A., et al. (2016). Distinct Nrf2 signaling mechanisms of fumaric acid esters and their role in neuroprotection against 1-methyl-4-phenyl-1,2,3,6-tetrahydropyridine-induced experimental Parkinson’s-like disease. J. Neurosci. 36, 6332–6351. doi: 10.1523/JNEUROSCI.0426-16
Akimoto, T., Pohnert, S. C., Li, P., Zhang, M., Gumbs, C., Rosenberg, P. B., et al. (2005). Exercise stimulates Pgc-1alpha transcription in skeletal muscle through activation of the p38 MAPK pathway. J. Biol. Chem. 280, 9587–9593. doi: 10.1074/jbc.M408862200
Anderson, R. M., Barger, J. L., Edwards, M. G., Braun, K. H., O’Connor, C. E., Prolla, T. A., et al. (2008). Dynamic regulation of PGC-1alpha localization and turnover implicates mitochondrial adaptation in calorie restriction and the stress response. Aging Cell 7, 101–111. doi: 10.1111/j.1474-9726.2007.00357.x
Aquilano, K., Baldelli, S., Pagliei, B., Cannata, S. M., Rotilio, G., and Ciriolo, M. R. (2013). p53 orchestrates the PGC-1α-mediated antioxidant response upon mild redox and metabolic imbalance. Antioxid Redox Signal. 18, 386–399. doi: 10.1089/ars.2012.4615
Arany, Z., Lebrasseur, N., Morris, C., Smith, E., Yang, W., Ma, Y., et al. (2007). The transcriptional coactivator PGC-1beta drives the formation of oxidative type IIX fibers in skeletal muscle. Cell Metab. 5, 35–46. doi: 10.1016/j.cmet.2006.12.003
Atamna, H., Atamna, W., Al-Eyd, G., Shanower, G., and Dhahbi, J. M. (2015). Combined activation of the energy and cellular-defense pathways may explain the potent anti-senescence activity of methylene blue. Redox Biol. 6, 426–435. doi: 10.1016/j.redox.2015.09.004
Athale, J., Ulrich, A., MacGarvey, N. C., Bartz, R. R., Welty-Wolf, K. E., Suliman, H. B., et al. (2012). Nrf2 promotes alveolar mitochondrial biogenesis and resolution of lung injury in Staphylococcus aureus pneumonia in mice. Free Radic. Biol. Med. 53, 1584–1594. doi: 10.1016/j.freeradbiomed.2012.08.009
Bahn, G., and Jo, D. G. (2019). Therapeutic approaches to Alzheimer’s disease through modulation of NRF2. Neuromolecular Med. 21, 1–11. doi: 10.1007/s12017-018-08523-5
Baldelli, S., Aquilano, K., and Ciriolo, M. R. (2013). Punctum on two different transcription factors regulated by PGC-1α: nuclear factor erythroid-derived 2-like 2 and nuclear respiratory factor 2. Biochim. Biophys. Acta 1830, 4137–4146. doi: 10.1016/j.bbagen.2013.04.006
Barrientos, A., Casademont, J., Cardellach, F., Estivill, X., Urbano-Marquez, A., and Nunes, V. (1997). Reduced steady-state levels of mitochondrial RNA and increased mitochondrial DNA amount in human brain with aging. Brain Res. Mol. Brain Res. 52, 284–289.
Bernard, K., Logsdon, N. J., Miguel, V., Benavides, G. A., Zhang, J., Carter, A. B., et al. (2017). NADPH oxidase 4 (Nox4) suppresses mitochondrial biogenesis and bioenergetics in lung fibroblasts via a nuclear factor erythroid-derived 2-like 2 (Nrf2)-dependent pathway. J. Biol. Chem. 292, 3029–3038. doi: 10.1074/jbc.M116.752261
Blesa, J. R., and Hernández-Yago, J. (2006). Distinct functional contributions of 2 GABP-NRF-2 recognition sites within the context of the human TOMM70 promoter. Biochem. Cell Biol. 84, 813–822. doi: 10.1139/o06-064
Boengler, K., Kosiol, M., Mayr, M., Schulz, R., and Rohrbach, S. (2017). Mitochondria and ageing: role in heart, skeletal muscle and adipose tissue. J. Cachexia Sarcopenia Muscle 8, 349–369. doi: 10.1002/jcsm.12178
Bohanon, F. J., Nunez Lopez, O., Herndon, D. N., Wang, X., Bhattarai, N., Ayadi, A. E., et al. (2018). Burn trauma acutely increases the respiratory capacity and function of liver Mitochondria. Shock 49, 466–473. doi: 10.1097/SHK.0000000000000935
Boss, O., Bachman, E., Vidal-Puig, A., Zhang, C. Y., Peroni, O., and Lowell, B. B. (1999). Role of the beta(3)-adrenergic receptor and/or a putative beta(4)-adrenergic receptor on the expression of uncoupling proteins and peroxisome proliferator-activated receptor-gamma coactivator-1. Biochem. Biophys. Res. Commun. 261, 870–876. doi: 10.1006/bbrc.1999.1145
Brauer, R., Bhaskaran, K., Chaturvedi, N., Dexter, D. T., Smeeth, L., and Douglas, I. (2015). Glitazone treatment and incidence of Parkinson’s disease among people with diabetes: a retrospective cohort study. PLoS Med. 12:e1001854. doi: 10.1371/journal.pmed.1001854
Burns, E. M., Kruckeberg, T. W., Comerford, L. E., and Buschmann, M. T. (1979). Thinning of capillary walls and declining numbers of endothelial mitochondria in the cerebral cortex of the aging primate, Macaca nemestrina. J. Gerontol. 34, 642–650.
Calkins, M. J., Jakel, R. J., Johnson, D. A., Chan, K., Kan, Y. W., and Johnson, J. A. (2005). Protection from mitochondrial complex II inhibition in vitro and in vivo by Nrf2-mediated transcription. Proc. Natl. Acad. Sci. U.S.A. 102, 244–249. doi: 10.1073/pnas.0408487101
Callahan, D. M., Bedrin, N. G., Subramanian, M., Berking, J., Ades, P. A., Toth, M. J., et al. (2014). Age-related structural alterations in human skeletal muscle fibers and mitochondria are sex specific: relationship to single-fiber function. J. Appl. Physiol. 116, 1582–1592. doi: 10.1152/japplphysiol.01362.2013
Calvert, J. W., Elston, M., Nicholson, C. K., Gundewar, S., Jha, S., Elrod, J. W., et al. (2010). Genetic and pharmacologic hydrogen sulfide therapy attenuates ischemia-induced heart failure in mice. Circulation 122, 11–19. doi: 10.1161/CIRCULATIONAHA.109.920991
Cantó, C., Gerhart-Hines, Z., Feige, J. N., Lagouge, M., Noriega, L., Milne, J. C., et al. (2009). AMPK regulates energy expenditure by modulating NAD+ metabolism and SIRT1 activity. Nature 458, 1056–1060. doi: 10.1038/nature07813
Carraway, M. S., Suliman, H. B., Jones, W. S., Chen, C. W., Babiker, A., and Piantadosi, C. A. (2010). Erythropoietin activates mitochondrial biogenesis and couples red cell mass to mitochondrial mass in the heart. Circ Res. 106, 1722–1730. doi: 10.1161/CIRCRESAHA.109.214353
Castillo-Quan, J. I. (2011). Parkin’ control: regulation of PGC-1α through PARIS in Parkinson’s disease. Dis. Model. Mech. 4, 427–429. doi: 10.1242/dmm.008227
Castillo-Quan, J. I., Li, L., Kinghorn, K. J., Ivanov, D. K., Tain, L. S., Slack, C., et al. (2016). Lithium promotes longevity through GSK3/NRF2-dependent hormesis. Cell Rep. 15, 638–650. doi: 10.1016/j.celrep.2016.03.041
Chabi, B., Ljubicic, V., Menzies, K. J., Huang, J. H., Saleem, A., and Hood, D. A. (2008). Mitochondrial function and apoptotic susceptibility in aging skeletal muscle. Aging Cell 7, 2–12. doi: 10.1111/j.1474-9726.2007.00347.x
Chen, H., Hu, Y., Fang, Y., Djukic, Z., Yamamoto, M., Shaheen, N. J., et al. (2014). Nrf2 deficiency impairs the barrier function of mouse oesophageal epithelium. Gut 63, 711–719. doi: 10.1136/gutjnl-2012-303731
Cherry, A. D., Suliman, H. B., Bartz, R. R., and Piantadosi, C. A. (2014). Peroxisome proliferator-activated receptor γ co-activator 1-α as a critical co-activator of the murine hepatic oxidative stress response and mitochondrial biogenesis in Staphylococcus aureus sepsis. J. Biol. Chem. 289, 41–52. doi: 10.1074/jbc.M113.512483
Cho, S. H., Chen, J. A., Sayed, F., Ward, M. E., Gao, F., Nguyen, T. A., et al. (2015). SIRT1 deficiency in microglia contributes to cognitive decline in aging and neurodegeneration via epigenetic regulation of IL-1β. J. Neurosci. 35, 807–818. doi: 10.1523/JNEUROSCI.2939-14.2015
Choi, H. I., Kim, H. J., Park, J. S., Kim, I. J., Bae, E. H., Ma, S. K., et al. (2017). PGC-1α attenuates hydrogen peroxide-induced apoptotic cell death by upregulating Nrf-2 via GSK3β inactivation mediated by activated p38 in HK-2 Cells. Sci. Rep. 7:4319. doi: 10.1038/s41598-017-04593-w
Clayton, D. A. (2000). Vertebrate mitochondrial DNA-a circle of surprises. Exp. Cell Res. 255, 4–9. doi: 10.1006/excr.1999.4763
Conley, K. E., Jubrias, S. A., and Esselman, P. C. (2000). Oxidative capacity and ageing in human muscle. J. Physiol. 526, 203–210.
Corsetti, G., Pasini, E., D’Antona, G., Nisoli, E., Flati, V., Assanelli, D., et al. (2008). Morphometric changes induced by amino acid supplementation in skeletal and cardiac muscles of old mice. Am. J. Cardiol. 101, 26E–34E. doi: 10.1016/j.amjcard.2008.02.078
Cuadrado, A., Kügler, S., and Lastres-Becker, I. (2018). Pharmacological targeting of GSK-3 and NRF2 provides neuroprotection in a preclinical model of tauopathy. Redox Biol. 14, 522–534. doi: 10.1016/j.redox.2017.10.010
Cui, L., Jeong, H., Borovecki, F., Parkhurst, C. N., Tanese, N., and Krainc, D. (2006). Transcriptional repression of PGC-1alpha by mutant huntingtin leads to mitochondrial dysfunction and neurodegeneration. Cell 127, 59–69. doi: 10.1016/j.cell.2006.09.015
Dehghan, E., Zhang, Y., Saremi, B., Yadavali, S., Hakimi, A., Dehghani, M., et al. (2017). Hydralazine induces stress resistance and extends C. elegans lifespan by activating the NRF2/SKN-1 signalling pathway. Nat. Commun. 8:2223. doi: 10.1038/s41467-017-02394-3
Dencher, N. A., Frenzel, M., Reifschneider, N. H., Sugawa, M., and Krause, F. (2007). Proteome alterations in rat mitochondria caused by aging. Ann. N. Y. Acad. Sci. 1100, 291–298. doi: 10.1196/annals.1395.030
Derbré, F., Gomez-Cabrera, M. C., Nascimento, A. L., Sanchis-Gomar, F., Martinez-Bello, V. E., Tresguerres, J. A., et al. (2012). Age associated low mitochondrial biogenesis may be explained by lack of response of PGC-1α to exercise training. Age 34, 669–679. doi: 10.1007/s11357-011-9264-y
Di Cristo, F., Finicelli, M., Digilio, F. A., Paladino, S., Valentino, A., Scialò, F., et al. (2018). Meldonium improves Huntington’s disease mitochondrial dysfunction by restoring peroxisome proliferator-activated receptor γ coactivator 1α expression. J. Cell. Physiol. 234, 9233–9246. doi: 10.1002/jcp.27602
Dinkova-Kostova, A. T., and Abramov, A. Y. (2015). The emerging role of Nrf2 in mitochondrial function. Free Radic. Biol. Med. 88, 179–188. doi: 10.1016/j.freeradbiomed.2015.04.036
Duan, W., Zhang, R., Guo, Y., Jiang, Y., Huang, Y., Jiang, H., et al. (2009). Nrf2 activity is lost in the spinal cord and its astrocytes of aged mice. In Vitro Cell. Dev. Biol. Anim. 45, 388–397. doi: 10.1007/s11626-009-9194-5
Duan, Y., Sun, F., Que, S., Li, Y., Yang, S., and Liu, G. (2018). Prepregnancy maternal diabetes combined with obesity impairs placental mitochondrial function involving Nrf2/ARE pathway and detrimentally alters metabolism of offspring. Obes. Res. Clin. Pract. 12, 90–100. doi: 10.1016/j.orcp.2017.01.002
Dumont, M., Stack, C., Elipenahli, C., Jainuddin, S., Launay, N., Gerges, M., et al. (2014). PGC-1α overexpression exacerbates β-amyloid and tau deposition in a transgenic mouse model of Alzheimer’s disease. FASEB J. 28, 1745–1755. doi: 10.1096/fj.13-236331
Dumont, M., Wille, E., Calingasan, N. Y., Tampellini, D., Williams, C., Gouras, G. K., et al. (2009). Triterpenoid CDDO-methylamide improves memory and decreases amyloid plaques in a transgenic mouse model of Alzheimer’s disease. J. Neurochem. 109, 502–512. doi: 10.1111/j.1471-4159.2009.05970.x
Edwards, C., Canfield, J., Copes, N., Rehan, M., Lipps, D., and Bradshaw, P. C. (2014). D-beta-hydroxybutyrate extends lifespan in C. elegans. Aging 6, 621–644. doi: 10.18632/aging.100683
Ellrichmann, G., Petrasch-Parwez, E., Lee, D. H., Reick, C., Arning, L., Saft, C., et al. (2011). Efficacy of fumaric acid esters in the R6/2 and YAC128 models of Huntington’s disease. PLoS One 6:e16172. doi: 10.1371/journal.pone.0016172
Evans, M. J., and Scarpulla, R. C. (1990). NRF-1: a trans-activator of nuclear-encoded respiratory genes in animal cells. Genes Dev. 4, 1023–1034.
Fang, E. F., Waltz, T. B., Kassahun, H., Lu, Q., Kerr, J. S., Morevati, M., et al. (2017). Tomatidine enhances lifespan and healthspan in C. elegans through mitophagy induction via the SKN-1/Nrf2 pathway. Sci. Rep. 7:46208. doi: 10.1038/srep46208
Ferguson, M., Mockett, R. J., Shen, Y., Orr, W. C., and Sohal, R. S. (2005). Age-associated decline in mitochondrial respiration and electron transport in Drosophila melanogaster. Biochem. J. 390, 501–511. doi: 10.1042/BJ20042130
Fernandez-Marcos, P. J., and Auwerx, J. (2011). Regulation of PGC-1α, a nodal regulator of mitochondrial biogenesis. Am. J. Clin. Nutr. 93, 884S–890S. doi: 10.3945/ajcn.110.001917
Fischer, N., Büchter, C., Koch, K., Albert, S., Csuk, R., and Wätjen, W. (2017). The resveratrol derivatives trans-3,5-dimethoxy-4-fluoro-4’-hydroxystilbene and trans-2,4’,5-trihydroxystilbene decrease oxidative stress and prolong lifespan in Caenorhabditis elegans. J. Pharm. Pharmacol. 69, 73–81. doi: 10.1111/jphp.12657
Fivenson, E. M., Lautrup, S., Sun, N., Scheibye-Knudsen, M., Stevnsner, T., Nilsen, H., et al. (2017). Mitophagy in neurodegeneration and aging. Neurochem. Int. 109, 202–209. doi: 10.1016/j.neuint.2017.02.007
Geisler, S., Holmström, K. M., Treis, A., Skujat, D., Weber, S. S., Fiesel, F. C., et al. (2010). The PINK1/Parkin-mediated mitophagy is compromised by PD-associated mutations. Autophagy 6, 871–878.
Genc, K., Egrilmez, M. Y., and Genc, S. (2010). Erythropoietin induces nuclear translocation of Nrf2 and heme oxygenase-1 expression in SH-SY5Y cells. Cell Biochem. Funct. 28, 197–201. doi: 10.1002/cbf.1639
Genova, M. L., Bovina, C., Marchetti, M., Pallotti, F., Tietz, C., Biagini, G., et al. (1997). Decrease of rotenone inhibition is a sensitive parameter of complex I damage in brain non-synaptic mitochondria of aged rats. FEBS Lett. 410, 467–469.
Gershman, B., Puig, O., Hang, L., Peitzsch, R. M., Tatar, M., and Garofalo, R. S. (2007). High-resolution dynamics of the transcriptional response to nutrition in Drosophila: a key role for dFOXO. Physiol. Genomics 29, 24–34. doi: 10.1152/physiolgenomics.00061.2006
Giudice, A., Arra, C., and Turco, M. C. (2010). Review of molecular mechanisms involved in the activation of the Nrf2-ARE signaling pathway by chemopreventive agents. Methods Mol. Biol. 647, 37–74. doi: 10.1007/978-1-60761-738-9_3
Gleyzer, N., Vercauteren, K., and Scarpulla, R. C. (2005). Control of mitochondrial transcription specificity factors (TFB1M and TFB2M) by nuclear respiratory factors (NRF-1 and NRF-2) and PGC-1 family coactivators. Mol. Cell. Biol. 25, 1354–1366.
Gong, H., Pang, J., Han, Y., Dai, Y., Dai, D., Cai, J., et al. (2014). Age-dependent tissue expression patterns of Sirt1 in senescence-accelerated mice. Mol. Med. Rep. 10, 3296–3302. doi: 10.3892/mmr.2014.2648
Graziewicz, M. A., Longley, M. J., and Copeland, W. C. (2006). DNA polymerase gamma in mitochondrial DNA replication and repair. Chem. Rev. 106, 383–405. doi: 10.1021/cr040463d
Gureev, A. P., Shaforostova, E. A., Starkov, A. A., and Popov, V. N. (2018). β-guanidinopropionic acid stimulates brain mitochondria biogenesis and alters cognitive behavior in nondiseased mid-age mice. J. Exp. Neurosci. 12:1179069518766524. doi: 10.1177/1179069518766524
Gureev, A. P., Syromyatnikov, M. Y., Gorbacheva, T. M., Starkov, A. A., and Popov, V. N. (2016). Methylene blue improves sensorimotor phenotype and decreases anxiety in parallel with activating brain mitochondria biogenesis in mid-age mice. Neurosci. Res. 113, 19–27. doi: 10.1016/j.neures.2016.07.006
Handschin, C., Rhee, J., Lin, J., Tarr, P. T., and Spiegelman, B. M. (2003). An autoregulatory loop controls peroxisome proliferator-activated receptor gamma coactivator 1alpha expression in muscle. Proc. Natl. Acad. Sci. U.S.A. 100, 7111–7116. doi: 10.1073/pnas.1232352100
Harman, D. (2009). About “Origin and evolution of the free radical theory of aging: a brief personal history, 1954-2009”. Biogerontology 10:783. doi: 10.1007/s10522-009-9253-z
Hayashi, G., Jasoliya, M., Sahdeo, S., Saccà, F., Pane, C., Filla, A., et al. (2017). Dimethyl fumarate mediates Nrf2-dependent mitochondrial biogenesis in mice and humans. Hum. Mol. Genet. 26, 2864–2873. doi: 10.1093/hmg/ddx167
Heerdt, B. G., and Augenlicht, L. H. (1990). Changes in the number of mitochondrial genomes during human development. Exp. Cell Res. 186, 54–59.
Hepple, R. T. (2014). Mitochondrial involvement and impact in aging skeletal muscle. Front. Aging Neurosci. 6:211. doi: 10.3389/fnagi.2014.00211
Herzig, S., Long, F., Jhala, U. S., Hedrick, S., Quinn, R., Bauer, A., et al. (2001). CREB regulates hepatic gluconeogenesis through the coactivator PGC-1. Nature 413, 179–183. doi: 10.1038/35093131
Hiona, A., Sanz, A., Kujoth, G. C., Pamplona, R., Seo, A. Y., Hofer, T., et al. (2010). Mitochondrial DNA mutations induce mitochondrial dysfunction, apoptosis and sarcopenia in skeletal muscle of mitochondrial DNA mutator mice. PLoS One 5:e11468. doi: 10.1371/journal.pone.0011468
Holmström, K. M., Kostov, R. V., and Dinkova-Kostova, A. T. (2016). The multifaceted role of Nrf2 in mitochondrial function. Curr. Opin. Toxicol. 1, 80–91. doi: 10.1016/j.cotox.2016.10.002
Hota, K. B., Hota, S. K., Chaurasia, O. P., and Singh, S. B. (2012). Acetyl-L-carnitine-mediated neuroprotection during hypoxia is attributed to ERK1/2-Nrf2-regulated mitochondrial biosynthesis. Hippocampus 22, 723–736. doi: 10.1002/hipo.20934
Ivankovic, D., Chau, K. Y., Schapira, A. H., and Gegg, M. E. (2016). Mitochondrial and lysosomal biogenesis are activated following PINK1/parkin-mediated mitophagy. J. Neurochem. 136, 388–402. doi: 10.1111/jnc.13412
Jäger, S., Handschin, C., St-Pierre, J., and Spiegelman, B. M. (2007). AMP-activated protein kinase (AMPK) action in skeletal muscle via direct phosphorylation of PGC-1alpha. Proc. Natl. Acad. Sci. U.S.A. 104, 12017–12022. doi: 10.1073/pnas.0705070104
Jain, A., Lamark, T., Sjøttem, E., Larsen, K. B., Awuh, J. A., Øvervatn, A., et al. (2010). p62/SQSTM1 is a target gene for transcription factor NRF2 and creates a positive feedback loop by inducing antioxidant response element-driven gene transcription. J. Biol. Chem. 285, 22576–22591. doi: 10.1074/jbc.M110.118976
Jang, J. Y., Blum, A., Liu, J., and Finkel, T. (2018). The role of mitochondria in aging. J. Clin. Invest. 128, 3662–3670. doi: 10.1172/JCI120842
Jin, W., Kong, J., Lu, T., Wang, H., Ni, H., Wu, J., et al. (2011). Erythropoietin prevents secondary brain injury induced by cortical lesion in mice: possible involvement of Nrf2 signaling pathway. Ann. Clin. Lab. Sci. 41, 25–32.
Joe, Y., Zheng, M., Kim, H. J., Uddin, M. J., Kim, S. K., Chen, Y., et al. (2015). Cilostazol attenuates murine hepatic ischemia and reperfusion injury via heme oxygenase-dependent activation of mitochondrial biogenesis. Am. J. Physiol. Gastrointest. Liver Physiol. 309, G21–G29. doi: 10.1152/ajpgi.00307.2014
Joo, M. S., Kim, W. D., Lee, K. Y., Kim, J. H., Koo, J. H., and Kim, S. G. (2016). AMPK facilitates nuclear accumulation of Nrf2 by phosphorylating at serine 550. Mol. Cell. Biol. 36, 1931–1942. doi: 10.1128/MCB.00118-16
Joshi, G., and Johnson, J. A. (2012). The Nrf2-ARE pathway: a valuable therapeutic target for the treatment of neurodegenerative diseases. Recent Pat. CNS Drug Discov. 7, 218–229.
Kansanen, E., Kuosmanen, S. M., Leinonen, H., and Levonen, A. L. (2013). The Keap1-Nrf2 pathway: mechanisms of activation and dysregulation in cancer. Redox Biol. 1, 45–49. doi: 10.1016/j.redox.2012.10.001
Kerner, J., Turkaly, P. J., Minkler, P. E., and Hoppel, C. L. (2001). Aging skeletal muscle mitochondria in the rat: decreased uncoupling protein-3 content. Am. J. Physiol. Endocrinol. Metab. 281, E1054–E1062. doi: 10.1152/ajpendo.2001.281.5.E1054
Kim, H. V., Kim, H. Y., Ehrlich, H. Y., Choi, S. Y., Kim, D. J., and Kim, Y. (2013). Amelioration of Alzheimer’s disease by neuroprotective effect of sulforaphane in animal model. Amyloid 20, 7–12. doi: 10.3109/13506129.2012.751367
Kim, S., Choi, K. J., Cho, S. J., Yun, S. M., Jeon, J. P., Koh, Y. H., et al. (2016). Fisetin stimulates autophagic degradation of phosphorylated tau via the activation of TFEB and Nrf2 transcription factors. Sci. Rep. 6:24933. doi: 10.1038/srep24933
Kosuru, R., Kandula, V., Rai, U., Prakash, S., Xia, Z., and Singh, S. (2018). Pterostilbene decreases cardiac oxidative stress and inflammation via activation of AMPK/Nrf2/HO-1 pathway in fructose-fed diabetic rats. Cardiovasc. Drugs Ther. 32, 147–163. doi: 10.1007/s10557-018-6780-3
Krishnan, K. J., Reeve, A. K., Samuels, D. C., Chinnery, P. F., Blackwood, J. K., Taylor, R. W., et al. (2008). What causes mitochondrial DNA deletions in human cells? Nat. Genet. 40, 275–279. doi: 10.1038/ng.f.94
Lang, A. E., and Lozano, A. M. (1998). Parkinson’s disease. First of two parts. N. Engl. J. Med. 339, 1044–1053. doi: 10.1056/NEJM199810083391506
Lastres-Becker, I., García-Yagüe, A. J., Scannevin, R. H., Casarejos, M. J., Kügler, S., Rábano, A., et al. (2016). Repurposing the NRF2 activator dimethyl fumarate as therapy against synucleinopathy in Parkinson’s disease. Antioxid Redox Signal. 25, 61–77. doi: 10.1089/ars.2015.6549
Lenaz, G., D’Aurelio, M., Merlo Pich, M., Genova, M. L., Ventura, B., and Bovina, C. (2000). Mitochondrial bioenergetics in aging. Biochim. Biophys. Acta 1459, 397–404.
Li, X., Wang, H., Gao, Y., Li, L., Tang, C., Wen, G., et al. (2016). Protective effects of quercetin on mitochondrial biogenesis in experimental traumatic brain injury via the Nrf2 signaling pathway. PLoS One 11:e0164237. doi: 10.1371/journal.pone.0164237
Liao, V. H., Yu, C. W., Chu, Y. J., Li, W. H., Hsieh, Y. C., and Wang, T. T. (2011). Curcumin-mediated lifespan extension in Caenorhabditis elegans. Mech. Ageing Dev. 132, 480–487. doi: 10.1016/j.mad.2011.07.008
Lin, J., Handschin, C., and Spiegelman, B. M. (2005). Metabolic control through the PGC-1 family of transcription coactivators. Cell Metab. 1, 361–370. doi: 10.1016/j.cmet.2005.05.004
Lin, J., Puigserver, P., Donovan, J., Tarr, P., and Spiegelman, B. M. (2002). Peroxisome proliferator-activated receptor gamma coactivator 1beta (PGC-1beta ), a novel PGC-1-related transcription coactivator associated with host cell factor. J. Biol. Chem. 277, 1645–1648. doi: 10.1074/jbc.C100631200
Lin, T. M., and Beal, F. M. (2006). Mitochondrial dysfunction and oxidative stress in neurodegenerative diseases. Nature 443, 787–795. doi: 10.1038/nature05292
Lindblom, R., Higgins, G., Coughlan, M., and de Haan, J. B. (2015). Targeting mitochondria and reactive oxygen species-driven pathogenesis in diabetic nephropathy. Rev. Diabet. Stud. 12, 134–156. doi: 10.1900/RDS.2015.12.134
López-Otín, C., Blasco, M. A., Partridge, L., Serrano, M., and Kroemer, G. (2013). The hallmarks of aging. Cell 153, 1194–1217. doi: 10.1016/j.cell.2013.05.039
MacGarvey, N. C., Suliman, H. B., Bartz, R. R., Fu, P., Withers, C. M., Welty-Wolf, K. E., et al. (2012). Activation of mitochondrial biogenesis by heme oxygenase-1-mediated NF-E2-related factor-2 induction rescues mice from lethal Staphylococcus aureus sepsis. Am. J. Respir. Crit. Care Med. 185, 851–861. doi: 10.1164/rccm.201106-1152OC
Mark, K. A., Dumas, K. J., Bhaumik, D., Schilling, B., Davis, S., Oron, T. R., et al. (2016). Vitamin D promotes protein homeostasis and longevity via the stress response pathway genes skn-1, ire-1, and xbp-1. Cell Rep. 17, 1227–1237. doi: 10.1016/j.celrep.2016.09.086
Mathieu-Costello, O., Ju, Y., Trejo-Morales, M., and Cui, L. (2005). Greater capillary-fiber interface per fiber mitochondrial volume in skeletal muscles of old rats. J. Appl. Physiol. 99, 281–289. doi: 10.1152/japplphysiol.00750.2004
Meirhaeghe, A., Crowley, V., Lenaghan, C., Lelliott, C., Green, K., Stewart, A., et al. (2003). Characterization of the human, mouse and rat PGC1 beta (peroxisome-proliferator-activated receptor-gamma co-activator 1 beta) gene in vitro and in vivo. Biochem. J. 373, 155–165. doi: 10.1042/BJ20030200
Merry, T. L., and Ristow, M. (2016). Nuclear factor erythroid-derived 2-like 2 (NFE2L2, Nrf2) mediates exercise-induced mitochondrial biogenesis and the anti-oxidant response in mice. J. Physiol. 594, 5195–5207. doi: 10.1113/JP271957
Merzetti, E. M., and Staveley, B. E. (2015). spargel, the PGC-1α homologue, in models of Parkinson disease in Drosophila melanogaster. BMC Neurosci. 16:70. doi: 10.1186/s12868-015-0210-2
Metodiev, M. D., Lesko, N., Park, C. B., Cámara, Y., Shi, Y., Wibom, R., et al. (2009). Methylation of 12S rRNA is necessary for in vivo stability of the small subunit of the mammalian mitochondrial ribosome. Cell Metab. 9, 386–397. doi: 10.1016/j.cmet.2009.03.001
Mo, C., Wang, L., Zhang, J., Numazawa, S., Tang, H., Tang, X., et al. (2014). The crosstalk between Nrf2 and AMPK signal pathways is important for the anti-inflammatory effect of berberine in LPS-stimulated macrophages and endotoxin-shocked mice. Antioxid Redox Signal. 20, 574–588. doi: 10.1089/ars.2012.5116
Mukherjee, S., Basar, M. A., Davis, C., and Duttaroy, A. (2014). Emerging functional similarities and divergences between Drosophila Spargel/dPGC-1 and mammalian PGC-1 protein. Front. Genet. 5:216. doi: 10.3389/fgene.2014.00216
Murata, H., Takamatsu, H., Liu, S., Kataoka, K., Huh, N. H., and Sakaguchi, M. (2015). NRF2 regulates PINK1 expression under oxidative stress conditions. PLoS One 10:e0142438. doi: 10.1371/journal.pone.0142438
Navarro, A., and Boveris, A. (2004). Rat brain and liver mitochondria develop oxidative stress and lose enzymatic activities on aging. Am. J. Physiol. Regul. Integr. Comp. Physiol. 287, R1244–R1249. doi: 10.1152/ajpregu.00226.2004
Navarro, E., Gonzalez-Lafuente, L., Pérez-Liébana, I., Buendia, I., López-Bernardo, E., Sánchez-Ramos, C., et al. (2017). Heme-oxygenase I and PCG-1α regulate mitochondrial biogenesis via microglial activation of alpha7 nicotinic acetylcholine receptors using PNU282987. Antioxid Redox Signal. 27, 93–105. doi: 10.1089/ars.2016.6698
Negrette-Guzmán, M., García-Niño, W. R., Tapia, E., Zazueta, C., Huerta-Yepez, S., León-Contreras, J. C., et al. (2015). Curcumin attenuates gentamicin-induced kidney mitochondrial alterations: possible role of a mitochondrial biogenesis mechanism. Evid. Based Complement. Alternat. Med. 2015:917435. doi: 10.1155/2015/917435
Negrette-Guzmán, M., Huerta-Yepez, S., Vega, M. I., León-Contreras, J. C., Hernández-Pando, R., Medina-Campos, O. N., et al. (2017). Sulforaphane induces differential modulation of mitochondrial biogenesis and dynamics in normal cells and tumor cells. Food Chem. Toxicol. 100, 90–102. doi: 10.1016/j.fct.2016.12.020
Ng, C. H., Basil, A. H., Hang, L., Tan, R., Goh, K. L., O’Neill, S., et al. (2017). Genetic or pharmacological activation of the Drosophila PGC-1α ortholog spargel rescues the disease phenotypes of genetic models of Parkinson’s disease. Neurobiol. Aging 55, 33–37. doi: 10.1016/j.neurobiolaging.2017.03.017
Nisoli, E., Clementi, E., Paolucci, C., Cozzi, V., Tonello, C., Sciorati, C., et al. (2003). Mitochondrial biogenesis in mammals: the role of endogenous nitric oxide. Science 299, 896–899. doi: 10.1126/science.1079368
Ogawa, T., Kodera, Y., Hirata, D., Blackwell, T. K., and Mizunuma, M. (2016). Natural thioallyl compounds increase oxidative stress resistance and lifespan in Caenorhabditis elegans by modulating SKN-1/Nrf. Sci. Rep. 6:21611. doi: 10.1038/srep21611
Ojaimi, J., Masters, C. L., Opeskin, K., McKelvie, P., and Byrne, E. (1999). Mitochondrial respiratory chain activity in the human brain as a function of age. Mech. Ageing Dev. 111, 39–47.
Ojuka, E. O. (2004). Role of calcium and AMP kinase in the regulation of mitochondrial biogenesis and GLUT4 levels in muscle. Proc. Nutr. Soc. 63, 275–278. doi: 10.1079/PNS2004339
Onyango, I. G., Lu, J., Rodova, M., Lezi, E., Crafter, A. B., and Swerdlow, R. H. (2010). Regulation of neuron mitochondrial biogenesis and relevance to brain health. Biochim. Biophys. Acta 802, 228–234. doi: 10.1016/j.bbadis.2009.07.014
Palikaras, K., Lionaki, E., and Tavernarakis, N. (2015). Coordination of mitophagy and mitochondrial biogenesis during ageing in C. elegans. Nature 521, 525–528. doi: 10.1038/nature14300
Pawlyk, A. C., Giacomini, K. M., McKeon, C., Shuldiner, A. R., and Florez, J. C. (2014). Metformin pharmacogenomics: current status and future directions. Diabetes 63, 2590–2599. doi: 10.2337/db13-1367
Piantadosi, C. A., Carraway, M. S., Babiker, A., and Suliman, H. B. (2008). Heme oxygenase-1 regulates cardiac mitochondrial biogenesis via Nrf2-mediated transcriptional control of nuclear respiratory factor-1. Circ. Res. 103, 1232–1240. doi: 10.1161/01.RES.0000338597
Pikó, L., and Taylor, K. D. (1987). Amounts of mitochondrial DNA and abundance of some mitochondrial gene transcripts in early mouse embryos. Dev. Biol. 123, 364–374.
Pokkunuri, I., Ali, Q., and Asghar, M. (2016). Grape powder improves age-related decline in mitochondrial and kidney functions in Fischer 344 rats. Oxid. Med. Cell. Longev. 2016:6135319. doi: 10.1155/2016/6135319
Powolny, A. A., Singh, S. V., Melov, S., Hubbard, A., and Fisher, A. L. (2011). The garlic constituent diallyl trisulfide increases the lifespan of C. elegans via skn-1 activation. Exp. Gerontol. 46, 441–452. doi: 10.1016/j.exger.2011.01.005
Prasad, S., Sajja, R. K., Kaisar, M. A., Park, J. H., Villalba, H., Liles, T., et al. (2017). Role of Nrf2 and protective effects of Metformin against tobacco smoke-induced cerebrovascular toxicity. Redox Biol. 12, 58–69. doi: 10.1016/j.redox.2017.02.007
Puigserver, P., Rhee, J., Lin, J., Wu, Z., Yoon, J. C., Zhang, C. Y., et al. (2001). Cytokine stimulation of energy expenditure through p38 MAP kinase activation of PPARgamma coactivator-1. Mol. Cell 8, 971–982.
Puigserver, P., Wu, Z., Park, C. W., Graves, R., Wright, M., and Spiegelman, B. M. (1998). A cold-inducible coactivator of nuclear receptors linked to adaptive thermogenesis. Cell 92, 829–839.
Qin, W., Haroutunian, V., Katsel, P., Cardozo, C. P., Ho, L., Buxbaum, J. D., et al. (2009). PGC-1alpha expression decreases in the Alzheimer disease brain as a function of dementia. Arch. Neurol. 66, 352–361. doi: 10.1001/archneurol.2008.588
Qin, Y. J., Zhang, X. L., Yu, Y. Q., Bian, X. H., and Dong, S. M. (2013). Cardioprotective effect of erythropoietin on sepsis-induced myocardial injury in rats. World J. Emerg. Med. 4, 215–222. doi: 10.5847/wjem.j.1920-8642.2013.03.011
Ratliff, B. B., Abdulmahdi, W., Pawar, R., and Wolin, M. S. (2016). Oxidant mechanisms in renal injury and disease. Antioxid Redox Signal. 25, 119–146. doi: 10.1089/ars.2016.6665
Rebelo, A. P., Dillon, L. M., and Moraes, C. T. (2011). Mitochondrial DNA transcription regulation and nucleoid organization. J. Inherit. Metab. Dis. 34, 941–951. doi: 10.1007/s10545-011-9330-8
Rera, M., Bahadorani, S., Cho, J., Koehler, C. L., Ulgherait, M., Hur, J. H., et al. (2011). Modulation of longevity and tissue homeostasis by the Drosophila PGC-1 homolog. Cell Metab. 14, 623–634. doi: 10.1016/j.cmet.2011.09.013
Reznick, R. M., Zong, H., Li, J., Morino, K., Moore, I. K., Yu, H. J., et al. (2007). Aging-associated reductions in AMP-activated protein kinase activity and mitochondrial biogenesis. Cell Metab. 5, 151–156. doi: 10.1016/j.cmet.2007.01.008
Riva, A., Tandler, B., Lesnefsky, E. J., Conti, G., Loffredo, F., Vazquez, E., et al. (2006). Structure of cristae in cardiac mitochondria of aged rat. Ageing Dev. 127, 917–921. doi: 10.1016/j.mad.2006.09.002
Rodgers, J. T., Lerin, C., Haas, W., Gygi, S. P., Spiegelman, B. M., and Puigserver, P. (2005). Nutrient control of glucose homeostasis through a complex of PGC-1alpha and SIRT1. Nature 434, 113–118. doi: 10.1038/nature03354
Rogers, R. P., and Rogina, B. (2014). Increased mitochondrial biogenesis preserves intestinal stem cell homeostasis and contributes to longevity in Indy mutant flies. Aging 6, 335–350. doi: 10.18632/aging.100658
Rygiel, K. A., Grady, J. P., and Turnbull, D. M. (2014). Respiratory chain deficiency in aged spinal motor neurons. Neurobiol. Aging 35, 2230–2238. doi: 10.1016/j.neurobiolaging.2014.02.027
Ryoo, I. G., and Kwak, M. K. (2018). Regulatory crosstalk between the oxidative stress-related transcription factor Nfe2l2/Nrf2 and mitochondria. Toxicol. Appl. Pharmacol. 359, 24–33. doi: 10.1016/j.taap.2018.09.014
Sachs, H. G., Colgan, J. A., and Lazarus, M. L. (1977). Ultrastructure of the aging myocardium: a morphometric approach. Am. J. Anat. 150, 63–71. doi: 10.1002/aja.1001500105
Scarpulla, R. C. (2008). Transcriptional paradigms in mammalian mitochondrial biogenesis and function. Physiol. Rev. 88, 611–638. doi: 10.1152/physrev.00025.2007
Schmeisser, S., Schmeisser, K., Weimer, S., Groth, M., Priebe, S., Fazius, E., et al. (2013). Mitochondrial hormesis links low-dose arsenite exposure to lifespan extension. Aging Cell 12, 508–517. doi: 10.1111/acel.12076
Schmucker, D. L., and Sachs, H. G. (1985). Age-dependent alterations in rat ventricular myocardium: a quantitative analysis. Mech. Ageing Dev. 31, 89–101.
Seo, H. W., Cheon, S. M., Lee, M. H., Kim, H. J., Jeon, H., and Cha, D. S. (2015). Catalpol modulates lifespan via DAF-16/FOXO and SKN-1/Nrf2 activation in Caenorhabditis elegans. Evid. Based Complement. Alternat. Med. 2015:524878. doi: 10.1155/2015/524878
Shih, P. H., and Yen, G. C. (2007). Differential expressions of antioxidant status in aging rats: the role of transcriptional factor Nrf2 and MAPK signaling pathway. Biogerontology 8, 71–80. doi: 10.1007/s10522-006-9033-y
Shin, J. H., Ko, H. S., Kang, H., Lee, Y., Lee, Y. I., Pletinkova, O., et al. (2011). PARIS (ZNF746) repression of PGC-1α contributes to neurodegeneration in Parkinson’s disease. Cell 144, 689–702. doi: 10.1016/j.cell.2011.02.010
Shukla, K., Sonowal, H., Saxena, A., Ramana, K. V., and Srivastava, S. K. (2017). Aldose reductase inhibitor, fidarestat regulates mitochondrial biogenesis via Nrf2/HO-1/AMPK pathway in colon cancer cells. Cancer Lett. 411, 57–63. doi: 10.1016/j.canlet.2017.09.031
Stack, C., Ho, D., Wille, E., Calingasan, N. Y., Williams, C., Liby, K., et al. (2010). Triterpenoids CDDO-ethyl amide and CDDO-trifluoroethyl amide improve the behavioral phenotype and brain pathology in a transgenic mouse model of Huntington’s disease. Free Radic. Biol. Med. 49, 147–158. doi: 10.1016/j.freeradbiomed.2010.03.017
Stefanatos, R., and Sanz, A. (2018). The role of mitochondrial ROS in the aging brain. FEBS Lett. 592, 743–758. doi: 10.1002/1873-3468.12902
Stevens, D. A., Lee, Y., Kang, H. C., Lee, B. D., Lee, Y. I., Bower, A., et al. (2015). Parkin loss leads to PARIS-dependent declines in mitochondrial mass and respiration. Proc. Natl. Acad. Sci. U.S.A. 112, 11696–11701. doi: 10.1073/pnas.1500624112
St-Pierre, J., Drori, S., Uldry, M., Silvaggi, J. M., Rhee, J., Jäger, S., et al. (2006). Suppression of reactive oxygen species and neurodegeneration by the PGC-1 transcriptional coactivators. Cell 127, 397–408. doi: 10.1016/j.cell.2006.09.024
Suh, J. H., Shenvi, S. V., Dixon, B. M., Liu, H., Jaiswal, A. K., Liu, R. M., et al. (2004). Decline in transcriptional activity of Nrf2 causes age-related loss of glutathione synthesis, which is reversible with lipoic acid. Proc. Natl. Acad. Sci. U.S.A. 101, 3381–3386. doi: 10.1073/pnas.0400282101
Sun, W., Lee, S., Huang, X., Liu, S., Inayathullah, M., Kim, K. M., et al. (2016). Attenuation of synaptic toxicity and MARK4/PAR1-mediated Tau phosphorylation by methylene blue for Alzheimer’s disease treatment. Sci. Rep. 6:34784. doi: 10.1038/srep34784
Sykiotis, G. P., and Bohmann, D. (2008). Keap1/Nrf2 signaling regulates oxidative stress tolerance and lifespan in Drosophila. Dev. Cell 14, 76–85. doi: 10.1016/j.devcel.2007.12.002
Tanaka, K., Suzuki, T., Hattori, N., and Mizuno, Y. (2004). Ubiquitin, proteasome and parkin. Biochim. Biophys. Acta 1695, 235–247. doi: 10.1016/j.bbamcr.2004.09.026
Tapias, V., Jainuddin, S., Ahuja, M., Stack, C., Elipenahli, C., Vignisse, J., et al. (2018). Benfotiamine treatment activates the Nrf2/ARE pathway and is neuroprotective in a transgenic mouse model of tauopathy. Hum. Mol. Genet. 27, 2874–2892. doi: 10.1093/hmg/ddy201
Tate, E. L., and Herbener, G. H. (1976). A morphometric study of the density of mitochondrial cristae in heart and liver of aging mice. J. Gerontol. 31, 129–134.
Tebay, L. E., Robertson, H., Durant, S. T., Vitale, S. R., Penning, T. M., Dinkova-Kostova, A. T., et al. (2015). Mechanisms of activation of the transcription factor Nrf2 by redox stressors, nutrient cues, and energy status and the pathways through which it attenuates degenerative disease. Free Radic. Biol. Med. 88, 108–146. doi: 10.1016/j.freeradbiomed.2015.06.021
Tengan, C. H., Rodrigues, G. S., and Godinho, R. O. (2012). Nitric oxide in skeletal muscle: role on mitochondrial biogenesis and function. Int. J. Mol. Sci. 13, 17160–17184. doi: 10.3390/ijms131217160
Thomas, R. R., Keeney, P. M., and Bennett, J. P. (2012). Impaired complex-I mitochondrial biogenesis in Parkinson disease frontal cortex. J. Parkinsons Dis. 2, 67–76. doi: 10.3233/JPD-2012-11074
Tiefenböck, S. K., Baltzer, C., Egli, N. A., and Frei, C. (2010). The Drosophila PGC-1 homologue Spargel coordinates mitochondrial activity to insulin signalling. EMBO J. 29, 171–183. doi: 10.1038/emboj.2009.330
Tiranti, V., Savoia, A., Forti, F., D’Apolito, M. F., Centra, M., Rocchi, M., et al. (1997). Identification of the gene encoding the human mitochondrial RNA polymerase (h-mtRPOL) by cyberscreening of the Expressed Sequence Tags database. Hum. Mol. Genet. 6, 615–625.
Tsou, Y. H., Shih, C. T., Ching, C. H., Huang, J. Y., Jen, C. J., Yu, L., et al. (2015). Treadmill exercise activates Nrf2 antioxidant system to protect the nigrostriatal dopaminergic neurons from MPP+ toxicity. Exp. Neurol. 263, 50–62. doi: 10.1016/j.expneurol.2014.09.021
Ungvari, Z., Bailey-Downs, L., Gautam, T., Sosnowska, D., Wang, M., Monticone, R. E., et al. (2011). Age-associated vascular oxidative stress, Nrf2 dysfunction, and NF-{kappa}B activation in the nonhuman primate Macaca mulatta. J. Gerontol. A Biol. Sci. Med. Sci. 66, 866–875. doi: 10.1093/gerona/glr092
Virbasius, J. V., and Scarpulla, R. C. (1994). Activation of the human mitochondrial transcription factor A gene by nuclear respiratory factors: a potential regulatory link between nuclear and mitochondrial gene expression in organelle biogenesis. Proc. Natl. Acad. Sci. U.S.A. 91, 1309–1313.
Wahlqvist, M. L., Lee, M. S., Hsu, C. C., Chuang, S. Y., Lee, J. T., and Tsai, H. N. (2012). Metformin-inclusive sulfonylurea therapy reduces the risk of Parkinson’s disease occurring with Type 2 diabetes in a Taiwanese population cohort. Parkinsonism Relat. Disord. 18, 753–758. doi: 10.1016/j.parkreldis.2012.03.010
Wallace, D. C. (2005). A mitochondrial paradigm of metabolic and degenerative diseases, aging, and cancer: a dawn for evolutionary medicine. Annu. Rev. Genet. 39, 359–407. doi: 10.1146/annurev.genet.39.110304.095751
Wang, L., Jia, Y., Rogers, H., Suzuki, N., Gassmann, M., Wang, Q., et al. (2013). Erythropoietin contributes to slow oxidative muscle fiber specification via PGC-1α and AMPK activation. Int. J. Biochem. Cell Biol. 45, 1155–1164. doi: 10.1016/j.biocel.2013.03.007
Wang, L., Zhang, S., Cheng, H., Lv, H., Cheng, G., and Ci, X. (2016). Nrf2-mediated liver protection by esculentoside A against acetaminophen toxicity through the AMPK/Akt/GSK3β pathway. Free Radic. Biol. Med. 101, 401–412. doi: 10.1016/j.freeradbiomed.2016.11.009
Wang, Y., Oxer, D., and Hekimi, S. (2015). Mitochondrial function and lifespan of mice with controlled ubiquinone biosynthesis. Nat. Commun. 6:6393. doi: 10.1038/ncomms7393
Wei, P., Guo, J., Xue, W., Zhao, Y., Yang, J., and Wang, J. (2018). RNF34 modulates the mitochondrial biogenesis and exercise capacity in muscle and lipid metabolism through ubiquitination of PGC-1 in Drosophila. Acta Biochim. Biophys. Sin. 50, 1038–1046. doi: 10.1093/abbs/gmy106
Wei, Y. H. (1998). Mitochondrial DNA mutations and oxidative damage in aging and diseases: an emerging paradigm of gerontology and medicine. Proc. Natl. Sci. Counc. Repub. China B 22, 55–67.
Weydt, P., Pineda, V. V., Torrence, A. E., Libby, R. T., Satterfield, T. F., Lazarowski, E. R., et al. (2006). Thermoregulatory and metabolic defects in Huntington’s disease transgenic mice implicate PGC-1alpha in Huntington’s disease neurodegeneration. Cell Metab. 4, 349–362. doi: 10.1016/j.cmet.2006.10.004
Whitman, S. A., Long, M., Wondrak, G. T., Zheng, H., and Zhang, D. D. (2013). Nrf2 modulates contractile and metabolic properties of skeletal muscle in streptozotocin-induced diabetic atrophy. Exp. Cell Res. 319, 2673–2683. doi: 10.1016/j.yexcr.2013.07.015
Wu, H., Zhao, J., Chen, M., Wang, H., Yao, Q., Fan, J., et al. (2017). The anti-aging effect of erythropoietin via the ERK/Nrf2-ARE pathway in aging rats. J. Mol. Neurosci. 61, 449–458. doi: 10.1007/s12031-017-0885-1
Wu, J. Z., Huang, J. H., Khanabdali, R., Kalionis, B., Xia, S. J., and Cai, W. J. (2016). Pyrroloquinoline quinone enhances the resistance to oxidative stress and extends lifespan upon DAF-16 and SKN-1 activities in C. elegans. Exp. Gerontol. 80, 43–50. doi: 10.1016/j.exger.2016.04.008
Wu, K. L. H., Wu, C. W., Chao, Y. M., Hung, C. Y., and Chan, J. Y. H. (2016). Impaired Nrf2 regulation of mitochondrial biogenesis in rostral ventrolateral medulla on hypertension induced by systemic inflammation. Free Radic. Biol. Med. 97, 58–74. doi: 10.1016/j.freeradbiomed.2016.05.012
Wu, T., Zhao, F., Gao, B., Tan, C., Yagishita, N., Nakajima, T., et al. (2014). Hrd1 suppresses Nrf2-mediated cellular protection during liver cirrhosis. Genes Dev. 28, 708–722. doi: 10.1101/gad.238246.114
Wu, Z., Puigserver, P., Andersson, U., Zhang, C., Adelmant, G., Mootha, V., et al. (1999). Mechanisms controlling mitochondrial biogenesis and respiration through the thermogenic coactivator PGC-1. Cell 98, 115–124. doi: 10.1016/S0092-8674(00)80611-X
Xie, G., Tian, W., Wei, T., and Liu, F. (2015). The neuroprotective effects of β-hydroxybutyrate on Aβ-injected rat hippocampus in vivo and in Aβ-treated PC-12 cells in vitro. Free Radic. Res. 49, 139–150. doi: 10.3109/10715762.2014.987274
Zakaria, A., Hamdi, N., and Abdel-Kader, R. M. (2016). Methylene blue improves brain mitochondrial ABAD functions and decreases Aβ in a neuroinflammatory Alzheimer’s disease mouse model. Mol. Neurobiol. 53, 1220–1228. doi: 10.1007/s12035-014-9088-8
Zeissler, M. L., Eastwood, J., McCorry, K., Hanemann, C. O., Zajicek, J. P., and Carroll, C. B. (2016). Delta-9-tetrahydrocannabinol protects against MPP+ toxicity in SH-SY5Y cells by restoring proteins involved in mitochondrial biogenesis. Oncotarget 7, 46603–46614. doi: 10.18632/oncotarget.10314
Zhang, D. D., and Hannink, M. (2003). Distinct cysteine residues in Keap1 are required for Keap1-dependent ubiquitination of Nrf2 and for stabilization of Nrf2 by chemopreventive agents and oxidative stress. Mol. Cell. Biol. 23, 8137–8151.
Zhang, F., Qi, Y., Zhou, K., Zhang, G., Linask, K., and Xu, H. (2015). The cAMP phosphodiesterase Prune localizes to the mitochondrial matrix and promotes mtDNA replication by stabilizing TFAM. EMBO Rep. 16, 520–527. doi: 10.15252/embr.201439636
Zhang, H., Davies, K. J. A., and Forman, H. J. (2015). Oxidative stress response and Nrf2 signaling in aging. Free Radic. Biol. Med. 88, 314–336. doi: 10.1016/j.freeradbiomed.2015.05.036
Zhao, M., New, L., Kravchenko, V. V., Kato, Y., Gram, H., di Padova, F., et al. (1999). Regulation of the MEF2 family of transcription factors by p38. Mol. Cell. Biol. 19, 21–30.
Zhou, Y., Xie, N., Li, L., Zou, Y., Zhang, X., and Dong, M. (2014). Puerarin alleviates cognitive impairment and oxidative stress in APP/PS1 transgenic mice. Int. J. Neuropsychopharmacol. 17, 635–644. doi: 10.1017/S146114571300148X
Zhu, Y. F., Li, X. H., Yuan, Z. P., Li, C. Y., Tian, R. B., Jia, W., et al. (2015). Allicin improves endoplasmic reticulum stress-related cognitive deficits via PERK/Nrf2 antioxidative signaling pathway. Eur. J. Pharmacol. 762, 239–246. doi: 10.1016/j.ejphar.2015.06.002
Keywords: mitochondrial biogenesis, Nrf2, PGC-1α, aging, active longevity
Citation: Gureev AP, Shaforostova EA and Popov VN (2019) Regulation of Mitochondrial Biogenesis as a Way for Active Longevity: Interaction Between the Nrf2 and PGC-1α Signaling Pathways. Front. Genet. 10:435. doi: 10.3389/fgene.2019.00435
Received: 08 November 2018; Accepted: 24 April 2019;
Published: 14 May 2019.
Edited by:
Elena G. Pasyukova, Institute of Molecular Genetics (RAS), RussiaReviewed by:
Nirmalya Chatterjee, Harvard Medical School, United StatesCopyright © 2019 Gureev, Shaforostova and Popov. This is an open-access article distributed under the terms of the Creative Commons Attribution License (CC BY). The use, distribution or reproduction in other forums is permitted, provided the original author(s) and the copyright owner(s) are credited and that the original publication in this journal is cited, in accordance with accepted academic practice. No use, distribution or reproduction is permitted which does not comply with these terms.
*Correspondence: Artem P. Gureev, Z3VyZWV2QGJpby52c3UucnU=
Disclaimer: All claims expressed in this article are solely those of the authors and do not necessarily represent those of their affiliated organizations, or those of the publisher, the editors and the reviewers. Any product that may be evaluated in this article or claim that may be made by its manufacturer is not guaranteed or endorsed by the publisher.
Research integrity at Frontiers
Learn more about the work of our research integrity team to safeguard the quality of each article we publish.