- 1Industrial Microbiology, Department of Biotechnology Delft, Delft University of Technology, Delft, Netherlands
- 2Delft Bioinformatics Lab, Department of Intelligent Systems, Delft University of Technology, Delft, Netherlands
- 3Infectious Disease and Microbiome Program, Broad Institute of MIT and Harvard, Boston, MA, United States
Saccharomyces pastorianus lager-brewing yeasts are domesticated hybrids of S. cerevisiae x S. eubayanus that display extensive inter-strain chromosome copy number variation and chromosomal recombinations. It is unclear to what extent such genome rearrangements are intrinsic to the domestication of hybrid brewing yeasts and whether they contribute to their industrial performance. Here, an allodiploid laboratory hybrid of S. cerevisiae and S. eubayanus was evolved for up to 418 generations on wort under simulated lager-brewing conditions in six independent sequential batch bioreactors. Characterization of 55 single-cell isolates from the evolved cultures showed large phenotypic diversity and whole-genome sequencing revealed a large array of mutations. Frequent loss of heterozygosity involved diverse, strain-specific chromosomal translocations, which differed from those observed in domesticated, aneuploid S. pastorianus brewing strains. In contrast to the extensive aneuploidy of domesticated S. pastorianus strains, the evolved isolates only showed limited (segmental) aneuploidy. Specific mutations could be linked to calcium-dependent flocculation, loss of maltotriose utilization and loss of mitochondrial activity, three industrially relevant traits that also occur in domesticated S. pastorianus strains. This study indicates that fast acquisition of extensive aneuploidy is not required for genetic adaptation of S. cerevisiae × S. eubayanus hybrids to brewing environments. In addition, this work demonstrates that, consistent with the diversity of brewing strains for maltotriose utilization, domestication under brewing conditions can result in loss of this industrially relevant trait. These observations have important implications for the design of strategies to improve industrial performance of novel laboratory-made hybrids.
Introduction
Saccharomyces yeasts are popular eukaryotic models for studying genome hybridization, chromosome (mis)segregation and aneuploidy (Botstein et al., 1997; Sheltzer et al., 2011). The genus Saccharomyces arose between 10 and 20 million years ago and currently comprises eight described species, as well as interspecies hybrids (Liti et al., 2006; Hittinger, 2013; Naseeb et al., 2017). Absence of a prezygotic barrier between Saccharomyces species facilitates hybridization, although spore viabilities of the resulting hybrids is typically well below 10% (Liti et al., 2006; Hittinger, 2013; Naseeb et al., 2017). Several interspecies Saccharomyces hybrids are tightly associated with domestication in industrial processes. S. pastorianus lager-brewing yeasts are domesticated S. cerevisiae × S. eubayanus hybrids (Libkind et al., 2011). Double and triple hybrids between S. cerevisiae, S. kudriavzevii and S. uvarum are closely associated with wine fermentation (González et al., 2006; Querol and Bond, 2009; Marsit and Dequin, 2015). S. bayanus cider fermentation yeasts are domesticated S. uvarum × S. eubayanus hybrids (Naumov et al., 2001). Reconstruction of the corresponding Saccharomyces hybrids in the laboratory showed improved performance, relative to the parental species. For example, laboratory-made S. cerevisiae × S. eubayanus hybrids combined sugar utilization characteristics of S. cerevisiae and the superior performance at low temperatures of S. eubayanus (Hebly et al., 2015; Krogerus et al., 2017). Similarly, hybrids of S. cerevisiae, S. kudriavzevii and S. uvarum combined traits of their parental species relevant to industrial wine fermentation, such as flocculence, sugar utilization kinetics, stress tolerance and aroma production (Coloretti et al., 2006; Lopandic et al., 2016).
The relevance of laboratory hybridization of Saccharomyces species extends beyond reconstruction of existing, domesticated hybrids. The ability of hybridization to generate extensive phenotypic diversity has raised interest in the development of novel Saccharomyces hybrids for specific industrial processes (Krogerus et al., 2017). For example, an S. cerevisiae × S. paradoxus hybrid produced high concentrations of aromatic compounds that are of interest for wine making (Bellon et al., 2011). Hybrids between S. cerevisiae and S. arboricola or S. mikatae were able to utilize the sugars in wort at low temperatures and produced particularly aromatic beer (Nikulin et al., 2017). Laboratory hybrids of S. cerevisiae and S. kudriavzevii or S. mikatae yielded xylose-consuming strains with high inhibitor tolerance for second generation biofuel production (Peris et al., 2017).
The alloeuploid genomes of laboratory hybrids of Saccharomyces species strongly differ from the extremely aneuploidy genomes of the domesticated strains used in traditional industrial processes. For example, the genomes of S. pastorianus lager-brewing yeasts contain between 45 and 79 chromosomes (Van Den Broek et al., 2015; Okuno et al., 2016), a degree of aneuploidy that is not observed elsewhere in the Saccharomyces genus (Gorter de Vries et al., 2017b). However, it remains unclear when and how domestication resulted in the extensive chromosome copy number variations and phenotypic diversity of current S. pastorianus strains.
Hybrid genomes have a well-documented increased tendency to become aneuploid due to an increased rate of chromosome missegregation during mitosis and/or meiosis (Chambers et al., 1996; Liti et al., 2006). Aneuploidy reduces the efficiency of sporulation and can thereby complicate genetic modification, impeding breeding and targeted strain improvement (Santaguida and Amon, 2015; Gorter de Vries et al., 2017a). In evolutionary contexts, aneuploidy is generally seen as a transient adaptation mechanism, whose positive impacts are eventually taken over by more parsimonious mutations (Yona et al., 2012). When grown mitotically, sporulated hybrid strains were prone to further chromosome missegregation resulting in more extensive chromosome copy number variations (Lopandic et al., 2016). Even genomes of Saccharomyces hybrids that had not undergone meiosis displayed increased rates of mitotic chromosome missegregation during mitosis (Delneri et al., 2003). Indeed, when evolved in lignocellulosic hydrolysates, cultures of S. cerevisiae × S. kudriavzevii and S. cerevisiae × S. mikatae hybrids exhibited segmental and full-chromosome aneuploidy after only 50 generations (Peris et al., 2017). Similarly, when evolved under wine fermentation conditions, S. cerevisiae × S. kudriavzevii hybrids displayed extensive genome reorganizations that led to a significant reduction of their genome content (Pérez Través et al., 2014).
Genetic instability of hybrid genomes could be detrimental to stable, robust industrial performance. Therefore, to assess industrial applicability of new hybrids generated in the laboratory, it is important to determine their genome stability under industrially relevant conditions. Moreover, laboratory evolution under simulated industrial conditions can increase understanding of the selective pressures that shaped the genomes of domesticated microorganisms (Bachmann et al., 2012; Gibbons et al., 2012; Gibbons and Rinker, 2015).
The goal of the present study was to investigate how the previously constructed allodiploid S. cerevisiae × S. eubayanus hybrid IMS0408 (Hebly et al., 2015) evolves under simulated lager-brewing conditions, with a specific focus on genome dynamics and on acquisition or loss of brewing-related phenotypes. To mimic successive lager beer fermentation processes, the hybrid strain was subjected to sequential batch cultivation on industrial wort, in six independent bioreactor setups. After up to 418 generations, the genotypic and phenotype diversity generated in these laboratory evolution experiments was analyzed by characterization of 55 single-cell isolates. After whole-genome resequencing of each isolate using 150 bp paired-end reads, sequence data were mapped to high-quality reference genomes of the parental strains to identify genomic changes. Phenotypic analysis of the isolates focused on the ability to utilize maltotriose, flocculation and the respiratory capacity. We interpreted these results in the context of the domestication history of S. pastorianus brewing strains as well as in relation to genome stability and industrial application of newly generated Saccharomyces hybrids.
Materials and Methods
Yeast Strains and Media
Saccharomyces strains used in this study are listed in Supplementary Table 1. Yeast strains and E. coli strains containing plasmids were stocked in 1 mL aliquots after addition of 30% v/v glycerol to the cultures and stored at −80°C. For preparation of stock cultures and inocula of bioreactors, yeast strains were routinely propagated in shake flasks containing 100 mL YPD (10 g.L−1 yeast extract, 20 g.L−1 yeast peptone and 20 g.L−1 glucose) at 30°C and 200 RPM in an Brunswick Innova43/43R shaker (Eppendorf Nederland B.V., Nijmegen, The Netherlands). For cultivation on solid media, YPD medium was supplemented with 20 g.L−1 Bacto agar (Becton Dickinson, Breda, The Netherlands) and incubation was done at 30 °C. Synthetic medium (SM), containing 3 g.L−1 KH2PO4, 0.5 g.L−1 MgSO4.7H2O, 5 g.L−1 (NH4)2SO4, 1 mL.L−1 of a trace element solution and 1 mL.L−1 of a vitamin solution, was prepared as previously described (Verduyn et al., 1992). SM maltotriose was supplemented with 20 g.L−1 of maltotriose and SM ethanol with 20 mL.L−1 of ethanol. Selection for the amdS marker was performed on SM-AC: SM with 0.6 g·L−1 acetamide and 6.6 g·L−1 K2SO4 instead of (NH4)2SO4 as nitrogen source (Solis-Escalante et al., 2013). For counter selection of the amdS marker, strains were first grown on YPD and then on SM-FAC: SM supplemented with 2.3 g·L−1 fluoroacetamide (Solis-Escalante et al., 2013). Industrial wort was provided by HEINEKEN Supply Chain B.V., Zoeterwoude, the Netherlands, and contained 14.4 g·L−1 glucose, 2.3 g·L−1 fructose, 85.9 g·L−1 maltose, 26.8 g·L−1 maltotriose and 269 mg/L free amino nitrogen. The wort was supplemented with 1.5 mg/L Zn2+ by addition of ZnSO4.7H2O, then autoclaved for 30 min at 121°C and, prior to use, filtered through Nalgene 0.2 μm SFCA bottle-top filters (ThermoFisher Scientific, Waltham, MA). For experiments performed with diluted wort, two volumes of sterile demineralized water were added per volume of wort. To prevent excessive foaming during the aeration phase of the bioreactor experiments, (un)diluted wort was supplemented with 0.2 mL.L−1 of sterile Pluronic PE 6100 antifoam (Sigma-Aldrich, Zwijndrecht, the Netherlands).
Analytical Methods and Statistics
Optical density at 660 nm was measured with a Libra S11 spectophotometer (Biochrom, Cambridge, UK). HPLC analysis of sugar and metabolite concentrations was performed with an Agilent Infinity 1260 chromatography system (Agilent Technologies, Santa Clara, CA) with an Aminex HPX-87 column (Bio-Rad, Lunteren, The Netherlands) at 65°C, eluted with 5 mM H2SO4. Significance of data was assessed by an unpaired two-tailed Student's t-test with a 95% confidence interval.
Laboratory Evolution and Single Colony Isolation
The hybrid yeast strain IMS0408 was evolved under three different conditions in duplicate in Minifors 2 bioreactors (INFORS HT, Velp, the Netherlands) with a working volume of 100 mL: on diluted wort at 30°C (LG30.1 and LG30.2), on diluted wort at 12°C (LG12.1 and LG12.2) and on full-strength wort at 12°C (HG12.1 and HG12.2). Sequential batch cultivation was performed with 10 and 30 mL.min−1 of headspace N2 flushing at 12 and 30°C, respectively. The percentage of CO2 in the outlet gas stream, the culture pH and the dissolved oxygen concentration in the broth were continuously monitored. The end of a batch cultivation cycle was automatically triggered when the percentage of CO2 in the offgas decreased below 75 and 10% of the maximum value reached during that cycle for growth on diluted wort and full-strength wort, respectively. These CO2 percentages correspond to the moment at which sugar utilization was complete in the first batch cycle for each condition, as determined by HPLC measurements. When the CO2 threshold was reached, the reactor was emptied while stirring at 1200 RPM leaving about 7 mL to inoculate the next batch. Upon addition of fresh medium, the broth was stirred at 500 RPM and sparged with 500 mL.min−1 of pressurized air during 5 min for diluted wort or 12 h for wort. During the remainder of each batch cultivation cycle, the medium was not sparged or stirred and the pH was not adjusted. LG30.1 and LG30.2 were carried out for 116 and 117 cycles, respectively, LG12.1 and LG12.2 were carried out for 29 cycles and HG12.1 and HG12.2 were carried out for 13 and 16 cycles, respectively. Culture samples from all six reactors were then streaked on YPD plates and after three subsequent restreaks, frozen stock cultures of single colony isolates were prepared. By default, five isolates were obtained for each culture. For LG12.1 and LG30.1, two different colony morphologies were observed, therefore five elevated and conically-shaped colonies and five regular flat colonies were stocked. The experiments at 12°C on diluted wort were continued for 4 months until a total of 58 and 57 cycles was reached for LG12.1 and LG12.2, respectively, and five single-cell isolates were obtained for each reactor as described above.
Genomic DNA Extraction and Whole Genome Sequencing
Yeast cultures were incubated in 500-mL shake-flasks containing 100 mL YPD at 30°C on an orbital shaker set at 200 RPM until the strains reached stationary phase at an OD660 between 12 and 20. Genomic DNA was isolated using the Qiagen 100/G kit (Qiagen, Hilden, Germany) according to the manufacturer's instructions and quantified using a Qubit® Fluorometer 2.0 (ThermoFisher Scientific). For IMS0408 and the evolved isolates, genomic DNA was sequenced at Novogene Bioinformatics Technology Co., Ltd (Yuen Long, Hong Kong) on a HiSeq2500 sequencer (Illumina, San Diego, CA) with 150 bp paired-end reads using PCR-free library preparation.
Genome Analysis
A high quality reference genome was constructed by combining near-complete assemblies of S. cerevisiae CEN.PK113-7D (Salazar et al., 2017) and S. eubayanus CBS 12357T (Brickwedde et al., 2018). The kanMX marker present in IMS0408 was inserted as an additional contig (Wach et al., 1994). For each evolved strain, raw Illumina reads were aligned against the reference genome using the Burrows–Wheeler Alignment tool (BWA, version 0.7.15-r1142) and further processed using SAMtools (version 1.3.1) and Pilon (version 1.18) for variant calling (Li et al., 2009; Li and Durbin, 2010; Walker et al., 2014). SNPs and INDELs that were also called or which were ambiguous in IMS0408, were disregarded. Copy number was determined based on read coverage analysis. Chromosomal translocations were detected using Breakdancer (version 1.3.6) (Chen et al., 2009). Only translocations which were supported by at least 10% of the reads aligned at that locus and which were absent in strain IMS0408 were considered. All SNPs, INDELs, recombinations and copy number changes were manually confirmed by visualizing the generated.bam files in the Integrative Genomics Viewer (IGV) software (Robinson et al., 2011). A complete list of identified mutations is provided in Supplementary Data File 1. For chimeric open-reading-frame reconstruction, reads aligning within 3 kbp of an identified recombination site and their paired reads were extracted using Python and were assembled using SPAdes (Bankevich et al., 2012). The resulting contigs were aligned against ORFs of the genes affected by the recombination to identify the recombination point, and the complete recombined ORF was reconstructed. Original and recombined ORFs were then aligned and translated using CloneManager (version 9.51, Sci-Ed Software, Denver, CO) to determine whether the translocation had introduced frameshifts or premature stop codons.
DNA Content Determination by Flow Cytometric Analysis
Exponential-phase shake flask cultures on YPD were diluted to an OD660 of 1. A 1 mL sample (~107 cells) was then washed in cold demineralized water and resuspended in 800 μL 70% ethanol while vortexing. After addition of another 800 μL 70% ethanol, fixed cells were stored at 4°C until further staining and analysis. DNA was then stained with SYTOX Green as described previously (Haase and Reed, 2002). Samples were analyzed on a Accuri C6 flow cytometer (BD Biosciences, Franklin Lakes, NJ) equipped with a 488-nm laser and the median fluorescence of cells in the 1n and 2n phases of the cell cycle was determined using FlowJo (BD Biosciences). The 1n and 2n medians of strains CEN.PK113-7D (n), CEN.PK122 (2n), and FRY153 (3n) were used to create a standard curve of fluorescence versus genome size with a linear curve fit, as performed previously (Van Den Broek et al., 2015). The genome size of each tested strain was estimated by averaging predicted genome sizes of the 1n and 2n population in assays on three independent cultures.
Identification of Strains With Respiratory Deficiency
Respiratory competence was assessed through their ability to grow on ethanol. Samples from 24 h shake-flask cultures on YPD (30°C, 200 RPM) were washed twice with demineralized water and used to inoculate duplicate aerobic shake flasks containing 100 mL of SM with 2% ethanol to an OD660 of 0.2. After 72 h incubation at 30°C and 200 RPM, OD660 was measured.
Assay for Calcium-Dependence of Flocculation
Two 100 μL aliquots from overnight cultures on YPD were washed with sterile demineralized water. One aliquot was resuspended in demineralized water and the other in 50 mM EDTA (pH 7.0). Both samples were imaged at 100 x magnification under a Z1 microscope (Carl Zeiss BV, Breda, the Netherlands) to assess flocculence and its reversal by EDTA chelation of calcium ions.
Plasmid Construction
All plasmids were propagated in E. coli DH5α (Table 1). The gRNAs to target ScSFL1 and SeSFL1 (Supplementary Table 2) were designed as previously described (Gorter de Vries et al., 2017a) and ordered as de novo synthesized plasmids pUD711 (ScSFL1) and pUD712 (SeSFL1) at GeneArt (ThermoFisher Scientific). Plasmid pUDP104, expressing gRNAScSFL1 and cas9, was constructed by Golden Gate cloning by digesting pUDP004 and pUD711 using BsaI and ligating with T4 ligase (Engler et al., 2008). Similarly, plasmid pUDP105, expressing gRNASeSFL1 and cas9, was constructed from pUDP004 and pUD712. Correct assembly was verified by restriction analysis using PdmI.
Strain Construction
The ScTEF1p-Venus-ScENO2t repair fragment with flanks for homologous recombination in the ScMAL11 locus was PCR amplified from plasmid pUDE482 using primers 12989 and 12990 (Supplementary Table 2). The ScTDH3p-mTurquoise2-ScADH1t repair fragment with flanks for homologous recombination in the ScSFL1 locus was PCR amplified from plasmid pUDE482 using primers 13564 and 13565. The ScTEF1p-Venus-ScENO2t repair fragment with flanks for homologous recombination in the SeSFL1 locus was PCR amplified from plasmid pUDE481 using primers 13566 and 13567.
All strains were transformed by electroporation as described previously, with 300 ng of gRNA/Cas9 expression plasmid and 1 μg of repair fragment (Gorter de Vries et al., 2017a). Strains IMX1698 (mVenus::ΔScmal11), IMX1824 (mTurquoise2::ΔScsfl1) and IMX1825 (Venus::ΔSesfl1) were constructed by transforming IMS0408 with the appropriate repair fragments and plasmids pUDP045, pUDP104 and pUDP105, respectively. Strain IMX1826 (mTurquoise2::ΔScsfl1 Venus::ΔSesfl1) was constructed by transforming IMX1824 using the appropriate repair fragment and plasmid pUDP105. After electroporation, cells were transferred to 20 mL SM-Ac medium to select successful transformants and incubated at 30°C for 3 to 5 days. After growth was observed, 200 μL of culture was transferred to 20 mL fresh SM-Ac and incubated similarly during 24h. Finally, 200 μL from the second culture was transferred to 20 mL fresh YPD medium to maximize expression of fluorescent proteins. Successfully gene-edited cells were sorted using the BD FACSAria™ II SORP Cell Sorter (BD Biosciences) as described previously (Gorter de Vries et al., 2018a). The plasmids were cured from strains IMX1698, IMX1824, IMX1825, and IMX1826 by subsequent growth on YPD and plating on SM-FAC. After confirmation of the correct genotype by colony PCR, randomly picked colonies were used to prepare frozen stocks.
Biomass Sedimentation Assay
IMS0408, IMS0556, IMS0558, IMS0559, IMS0617, IMX1824, IMX1825, and IMX1826 were grown in triplicate during 72 h in vented 50 mL Bio-One Cellstar Cellreactor tubes (Sigma-Aldrich) on 20 mL YPD at 30°C and 200 RPM until stationary phase. For each sample, the biomass was resuspended by vigorous vortexing and 1 mL was sampled immediately after vortexing from right underneath the meniscus. After 60 s of stationary incubation, another sample was taken by the same procedure. Biomass sedimentation was quantified as the ratio of the OD660 values of the two samples.
Evaluation of Maltotriose Fermentation
Each strain was grown microaerobically in 100 mL serum bottles containing 100 mL medium and shaken at 200 RPM. Medium (full-strength or diluted wort) and incubation temperature (12 or 30°C) were the same as in the evolution experiment from which a strain had been isolated. Strain IMS0408 was included as a control for each condition. Bottles were inoculated to an OD660 of 0.2 from aerobic shake-flask precultures grown on same medium and under the same conditions. During cultivation, 8 to 12 samples were taken at regular intervals for OD660 measurements and metabolite analysis by HPLC. When no further sugar consumption was recorded over an interval of at least 48h, the fermentation was considered finished.
Results
Simulating Domestication Under Lager-Brewing Conditions in Sequential Batch Bioreactors
Industrial lager brewing involves batch cultivation of S. pastorianus on wort, an extract from malted barley, at temperatures between 7 and 15°C. After a brief initial aeration phase to enable oxygen-dependent biosynthesis of unsaturated fatty acids and sterols (Andreasen and Stier, 1953; Gibson et al., 2007), brewing fermentations are not aerated or stirred, leading to anaerobic conditions during the main fermentation (Briggs et al., 2004). To simulate domestication under industrial lager-brewing conditions, a laboratory evolution regime was designed in which the laboratory-made S. cerevisiae x S. eubayanus hybrid IMS0408 was grown at 12°C in sequential batch bioreactors on industrial wort. As in industrial brewing, each cultivation cycle was preceded by an aeration phase, after which cultures were incubated without sparging or stirring until a decline of the CO2 production indicated a cessation of sugar consumption. The bioreactors were then partially emptied, leaving 7% of the culture volume as inoculum for the next aeration and fermentation cycle, which was initiated by refilling the reactor with sterile wort (Figures 1A,B). To mimic the low sugar concentrations during early domestication of S. pastorianus (Meussdoerffer, 2009), parallel duplicate experiments at 12°C were not only performed with full-strength 17 °Plato wort (“High Gravity,” experiments HG12.1 and HG12.2), but also with three-fold diluted wort (“Low Gravity,” experiments LG12.1 and LG12.2). To enable a larger number of generations during 4 months of operation, additional duplicate experiments on three-fold diluted wort were performed at 30°C (LG30.1 and LG30.2).
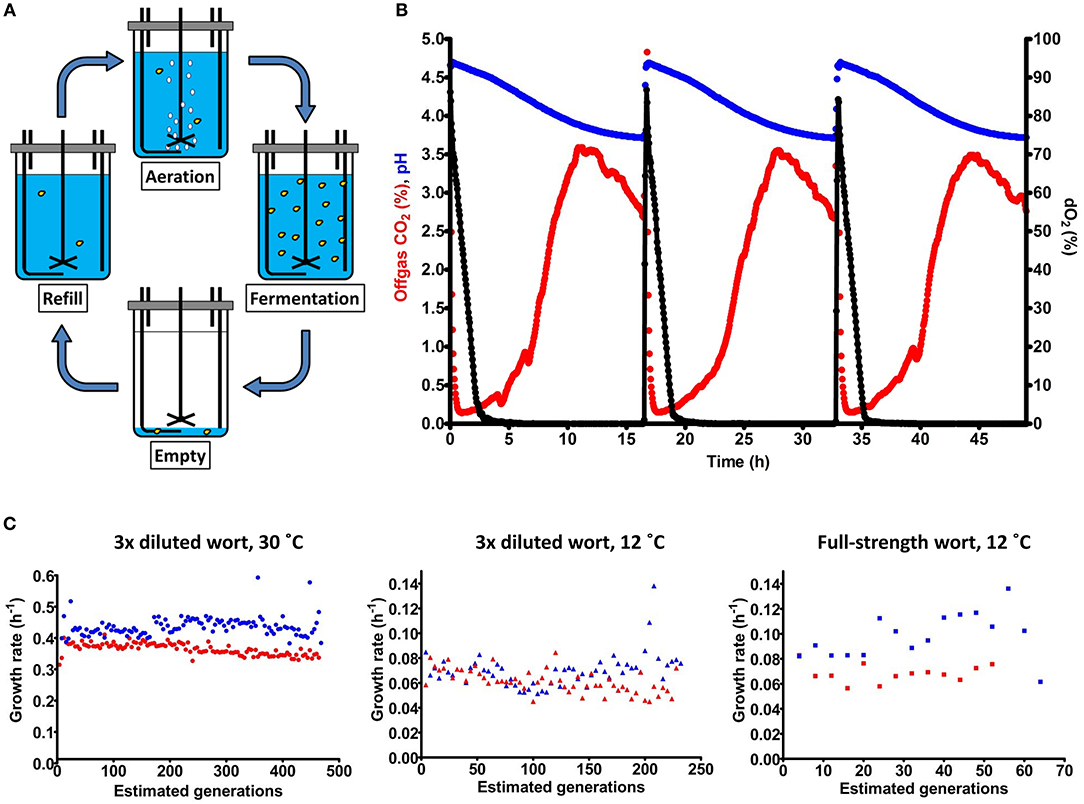
Figure 1. Laboratory evolution mimicking the domestication of lager-brewing yeast. The S. cerevisiae x S. eubayanus laboratory hybrid IMS0408 was grown in duplicate sequential batch bioreactors in three-fold diluted wort at 30°C (LG30.1 and LG30.2) and at 12°C (LG12.1 and LG12.2), and in full-strength wort at 12°C (HG12.1 and HG12.2). (A) Experimental design for simulated sequential lager-beer brewing cycles. Each cycle consisted of four phases: (i) (re)filling of the fermenter with fresh medium up to a total volume of 100 mL, (ii) aeration at 200 mL/min while stirring at 500 RPM, (iii) a batch fermentation phase without sparging or stirring, while flushing the bioreactor headspace with N2 to enable accurate analysis of CO2 production and (iv) removal of broth, leaving 7 mL to inoculate the next cycle. (B) Fermentation profiles of three consecutive cycles from experiment LG30.1, performed at 30°C in three-fold diluted wort. Percentage of CO2 in the off gas, culture pH and dissolved oxygen (dO2) concentration are indicated by red, blue and black symbols, respectively. Due to the lack of stirring and sparging, CO2 was slowly released by the medium; emptying of the reactor was initiated when the offgas CO2 concentration dropped to 70% of its initial value as off-line analyses indicated that, at this point, all fermentable sugars had been consumed (C) Specific growth estimated from CO2 production profiles during each cycle of the evolution lines. LG30.1 (blue circles) and LG30.2 (red circles) were grown on three-fold diluted wort at 30°C; LG12.1 (blue triangles) and LG12.2 (red triangles) were grown on three-fold diluted wort at 12°C. HG12.1 (blue squares) and HG12.2 (red squares) were evolved in full-strength wort at 12°C. Since lack of sparging and stirring precluded exact estimates of specific growth rates, the calculated values should be taken as indicative.
Concentration of the wort and temperature strongly affected the length of the fermentation cycles, which was 17 h for LG30.1 and LG30.2, 93 h for LG12.1 and LG12.2 and 205 h for HG12.1 and HG12.2. Experiments LG30.1 and LG30.2 involved 117 and 118 batch cycles, respectively, LG12.1 and LG12.2 covered 58 and 57 cycles, respectively, and HG12.1 and HG12.2 covered 13 and 16 cycles, respectively. At the inoculum size of 7% of the total culture volume, each cycle corresponded to ~4 generations. Specific growth rates, estimated from CO2 production rates during the exponential growth phase of the batch cycles, were not significantly different during the first and the last five cycles of each experiment (Student's t-test, p > 0.05). Average specific growth rates were 0.35 ± 0.02 h−1 for LG30.1, 0.42 ± 0.03 h−1 for LG30.2, 0.070 ± 0.013 h−1 for LG12.1, 0.062 ± 0.009 h−1 for LG12.2, 0.068 ± 0.007 h−1 for HG12.1 and 0.098 ± 0.018 h−1 for HG12.2. While the initial specific growth rate was clearly higher at 30°C than at 12°C, initial growth rates on diluted and full-strength wort were not significantly different. However, CO2 production from sugars continued much longer in full-strength wort. During brewing fermentation, depletion of nitrogen sources and oxygen limit biomass formation. Complete sugar conversion therefore depends on growth-independent alcoholic fermentation which, apparently, was much slower in cultures grown on full-strength wort. At the end of each evolution experiment, culture samples were streaked on YPD agar and 5 single colonies were isolated for each culture. For experiments LG12.1 and LG12.2, isolates were also made from intermediate samples after 29 cycles. Evolution lines LG30.2 and LG12.2 developed flocculence and isolates from these lines had two distinct colony morphologies: about half of the colonies were elevated and conically-shaped, while the other colonies shared the flat morphology of IMS0408 (Figure 3A). For each of these lines, five random colonies of each morphology were selected.
Prolonged Growth Under Simulated Brewing Conditions Did Not Cause Large Ploidy Changes
Six independent sequential batch fermentation experiments under simulated brewing conditions, covering 52 to 468 generations, yielded 55 isolates. Staining with the DNA-binding fluorescent dye SYTOX Green and flow cytometry indicated genome sizes of the isolates between 17.6 and 23.5 Mbp (Supplementary Table 3). These values did not differ significantly from the 21.3 ± 1.9 Mbp genome size measured for the parental laboratory hybrid IMS0408 and therefore indicated the absence of large changes in genome content such as whole-genome duplications. For a detailed genotypic analysis, the genomes of the 55 isolates were sequenced using 150 bp pair-end reads with 101- to 189-fold coverage. A high quality-reference genome was constructed by combining the chromosome-level contigs from assemblies of CEN.PK113-7D and CBS 12357 generated with nanopore technology, including mitochondrial genome sequences (Salazar et al., 2017; Brickwedde et al., 2018).
Copy number analysis revealed whole-chromosome aneuploidies in only 5/55 isolates (Table 2). Relative to strain IMS0408, the total chromosome number of the isolates had not changed by more than one. Isolate IMS0556 (LG30.1) had gained a copy of ScCHRVIII, IMS0560 (LG30.1) had gained a copy of SeCHRX, IMS0565 (LG30.2) had lost ScCHRXIV and gained a copy of SeCHRXIV, IMS0595 (LG12.1) had gained a copy of SeCHRVIII and IMS0606 (LG12.2) had lost a copy of SeCHRVIII.
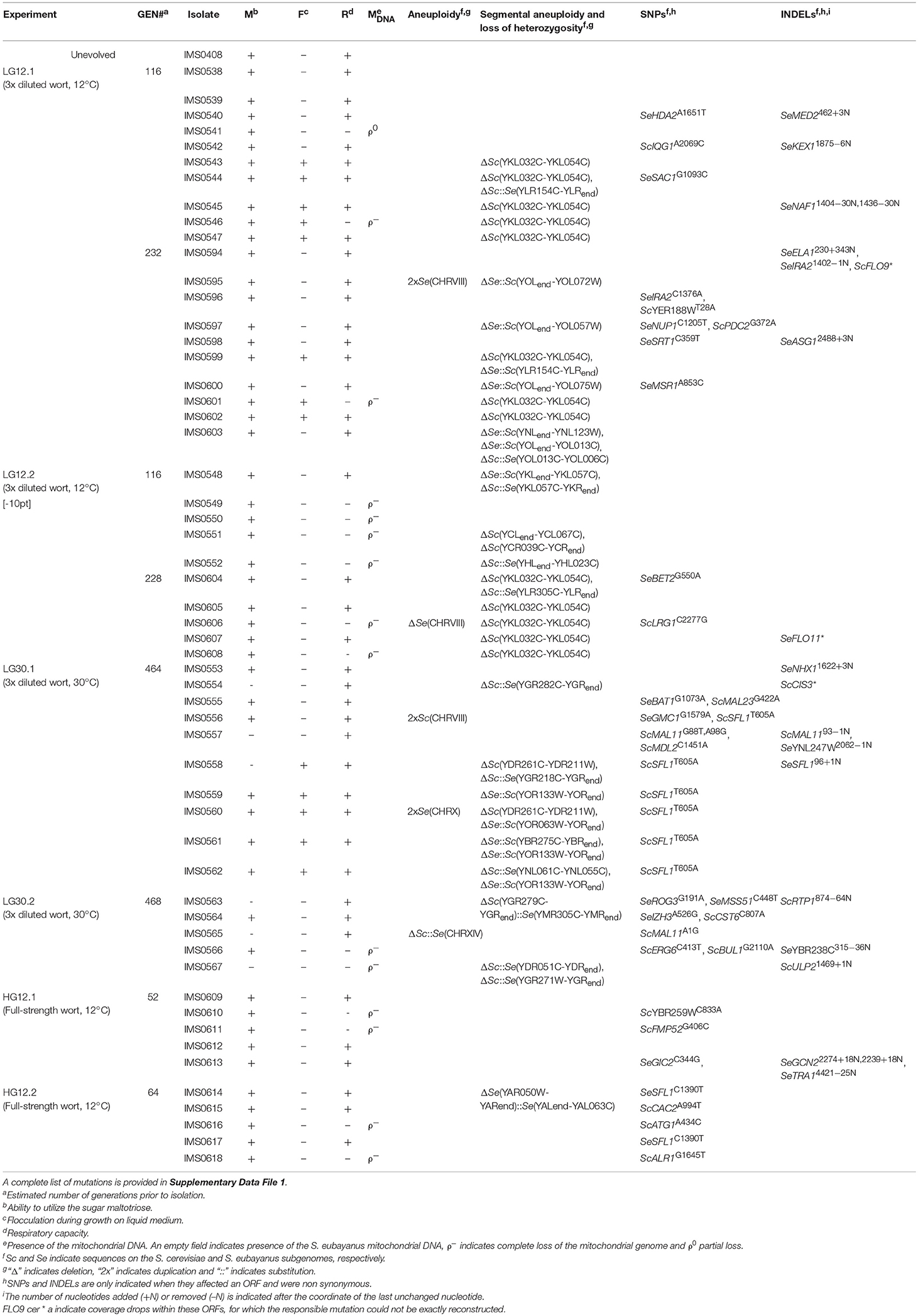
Table 2. Overview of phenotypic and genotypic changes in isolates obtained after laboratory evolution of the allodiploid laboratory hybrid IMS0408 under simulated lager-brewing conditions.
Read alignments to mitochondrial genome sequences were absent from 14/55 isolates, while 1 isolate showed only a partial alignment, indicating complete (ρ−) or partial loss (ρ0) of the mitochondrial genome in 15/55 strains. Loss of respiratory competence was confirmed by the observation that these 15 isolates, in contrast to IMS0408 and isolates containing a full mitochondrial genome, were unable to grow on YP-ethanol (Supplementary Figure 1).
Chromosomal Recombinations Frequently Caused Loss of Heterozygosity
Of the 55 evolved isolates, 29 displayed segmental copy number changes. In total, 20 of the 32 chromosomes in strain IMS0408 were affected in at least one isolate. Of the 55 evolved isolates, 24 showed chromosome segments with increased copy number and 41 showed chromosome segments with decreased copy number (Table 2, Figure 2). Seventeen internal recombinations resulting in deletions were observed: ΔSc(YKL032C-YKL054C) occurred in 13 strains, ΔSc(YDR261C-YDR211W) occurred in two strains, and ΔSc(YCLend-YCL067C) and ΔSc(YCR039C-YCRend) occurred together in one strain. The internal recombinations ΔSc(YKL032C-YKL054C) and ΔSc(YDR261C-YDR211W) both resulted in loss of the sequence between the recombination sites. The recombination occurred between IXR1 and DEF1 in ΔSc(YKL032C-YKL054C) and between Ty-transposons for ΔSc(YDR261C-YDR211W). Finally, the concurrent loss of Sc(YCLend-YCL067C) and Sc(YCR039C-YCRend) indicated loss of both ends of ScCHRIII, including telomeres. This mutation is consistent with a previously-observed circularization of chromosome III by a recombination between the HMLALPHA2 (YCL067C) and MATALPHA2 (YCR039C) loci, leading to loss of both chromosome extremities (Newlon et al., 1991).
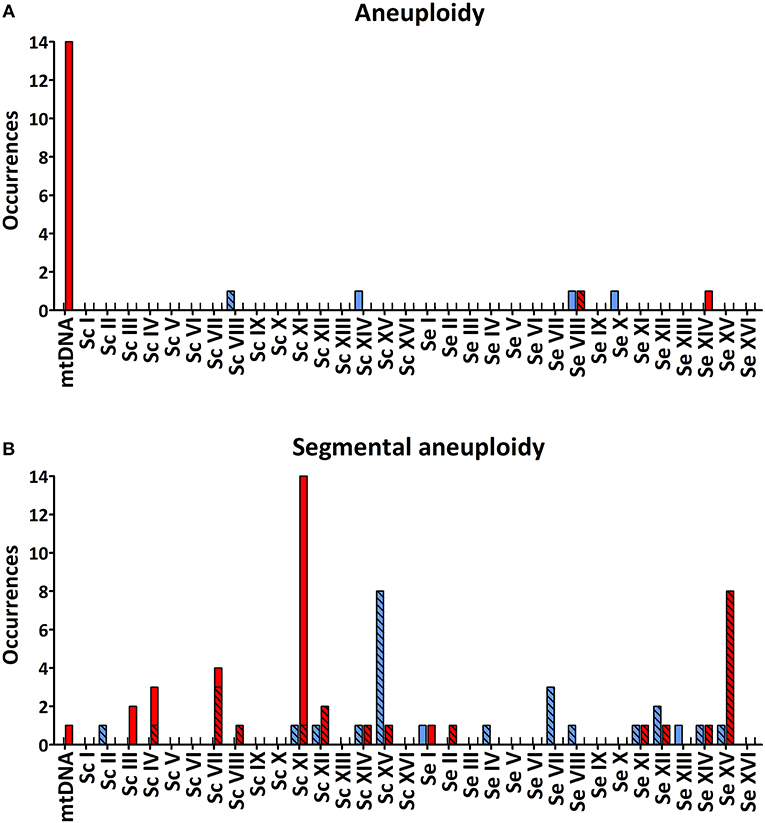
Figure 2. Total number of occurrences of whole-chromosome (A) and segmental (B) aneuploidy for each chromosome of IMS0408 among 55 isolates obtained after laboratory evolution under simulated lager fermentation conditions. For each chromosome, loss of genetic material is indicated in red and duplicated genetic material is indicated in blue. Loss or duplication of S. cerevisiae or S. eubayanus genetic material which was coupled with duplication or loss of the corresponding region of the other subgenome, is indicated by checked bars. S. eubayanus harbors two translocations relative to S. cerevisiae: between chromosomes II and IV, and between chromosomes VIII and XV. For simplicity, copy number affecting these regions were allocated based on the S. cerevisiae genome architecture.
The remaining 24 chromosome-segment duplications and losses reflected inter-chromosomal recombinations: one chromosomal region was replaced by an additional copy of another chromosomal region by a non-conservative recombination. The recombinations ΔSc(YGR279C-YGRend)::Se(YMR305C-YMRend) and ΔSe(YAR050W-YARend)::Se(YALend-YAL063C) occurred between highly similar genes; the paralogs SCW4 and SCW10, and FLO1 and FLO9, respectively. In the remaining 22 cases, recombination occurred between homologous genes of each subgenome. No copy-number conservative chromosome translocations were identified.
Of the 26 observed recombinations, 23 occurred inside ORFs, and thus resulted in chimeric genes (Table 3). The homology between ORFs involved in recombinations varied from < 70 to 100%, with a median homology of 82.41%. Chimeric ORFs were reconstructed by extracting reads from one locus affected by the recombination which were paired to the other locus affected by the recombination from the sequencing data, and using them for a local assembly. This approach allowed for identification of the recombination site at a resolution that, depending on sequence homology of the two ORFs, varied between 2 and 633 nucleotides. Due to length differences and relative INDELs between the original ORFs, recombined ORFs differed in length. However, all recombinations occurred in frame and no premature stop codons were introduced, suggesting that these chimeric ORFs might yield functional proteins.
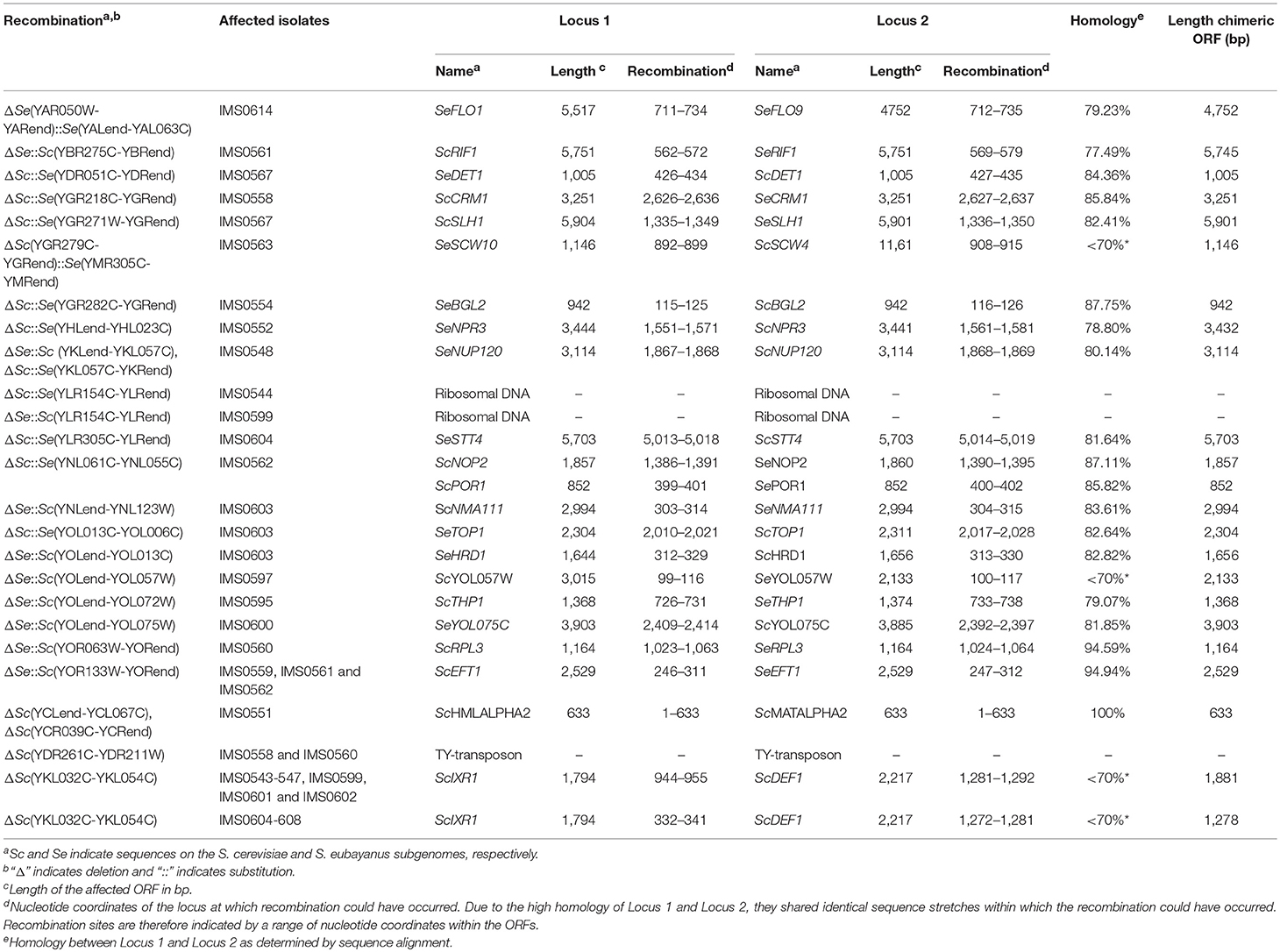
Table 3. Overview of all recombinations observed in 55 isolates obtained after laboratory evolution of strain IMS0408 under simulated lager fermentation conditions.
IRA2, SFL1, and MAL11 Are Mutated in Multiple Evolved Isolates
A total of 76 SNPs and 43 INDELs were identified in the genomes of the 55 isolates (Supplementary Data Files 1A,B), of which 38 SNPs and 17 INDELs occurred in ORFs and were non-synonymous (Table 2). Gene ontology analysis of all genes affected by non-synonymous SNPs or INDELs did not yield a significant enrichment in specific biological processes, molecular functions or cellular components. However, the genes IRA2, SFL1, and MAL11 were affected in more than one strain. IRA2 encodes a RAS GTPase-activating protein, which is disrupted in many S. cerevisiae genomes from the CEN.PK strain family (Tanaka et al., 1990, 1991; Nijkamp et al., 2012). In strain IMS0408, the ScIRA2 was indeed disrupted while the SeIRA2 ORF was intact. However, SeIRA2 was mutated in 6/10 isolates from LG12.1 after 232 generations. SeIRA2 had a frameshift in IMS0594, a premature stop codon in IMS0596 and was completely lost in four isolates due to different loss of heterozygosities: ΔSe::Sc(YOLend-YOL072W) in IMS0595, ΔSe::Sc(YOLend-YOL057W) in IMS0597, ΔSe::Sc(YOLend-YOL075W) in IMS0600 and ΔSe::Sc(YOLend-YOL013C) in IMS0603.
SFL1 encodes a transcriptional repressor of flocculation genes, which was present both on ScCHRXV and SeCHRVIII (Atsushi et al., 1989). ScSFL1 was mutated in 6/10 isolates from LG30.1 after 464 generations, which harbored a non-conservative substitution at the 605th nucleotide, affecting its DNA binding domain (Atsushi et al., 1989). SeSFL1 had a frameshift in IMS0558 (LG30.1), a single nucleotide substitution in IMS0614 and IMS0617 (HG12.2) and was completely lost in four isolates of LG30.1 due to two losses of heterozygosity: ΔSe::Sc(YOR133W-YORend) in IMS0559, IMS0561 and IMS0562, and ΔSe::Sc(YOR063W-YORend) in IMS0560 (Table 2).
ScMAL11, also referred to as AGT1, encodes the only maltose transporter of the MAL gene family which enables efficient uptake of maltotriose in IMS0408 (Alves et al., 2008). ScMAL11 is located on the right arm of ScCHRVII and is absent in the S. eubayanus subgenome of IMS0408, which has no other maltotriose transporters (Hebly et al., 2015; Brickwedde et al., 2018). ScMAL11 had a frameshift in IMS0557 (LG30.1) and lost its start codon in IMS0565 (LG30.2) (Table 2). In addition, ScMAL11 was completely lost due to three different losses of heterozygosity: ΔSc::Se(YGR282C-YGRend) in IMS0554 (LG30.1), ΔSc::Se(YGR218C-YGRend) in IMS0558 (LG30.1) and ΔSc::Se(YGR271W-YGRend) in IMS0567 (LG30.2), and due to the non-conservative recombination ΔSc(YGR279C-YGRend)::Se(YMR305C-YMRend) in IMS0563 (LG30.2).
Mutations in SFL1 Cause Emergence of Calcium-Dependent Flocculation
Eight of the 55 evolved isolates showed SNPs, INDELs or loss of heterozygosity in the flocculation inhibitor gene SFL1. In isolates IMS0558, IMS0559, IMS0560, IMS0561, and IMS0562 from LG30.1, both SeSFL1 and ScSFL1 were mutated, while in isolate IMS0556 from LG30.1 only ScSFL1 was mutated and in isolates IMS0614 and IMS0617 from HG12.2, only SeSFL1 was mutated. Evolved isolates that carried mutations in both ScSFL1 and SeSFL1 formed elevated conically-shaped colonies on YPD agar, while strain IMS0408 and evolved isolates with either an intact SeSFL1 or ScSFL1 did not (Figure 3A). Strains with mutations in both ScSFL1 and SeSFL1 also showed rapid sedimentation in micro-aerobic cultures on wort, which was not observed for the other evolved isolates or for strain IMS0408 (Figure 3B).
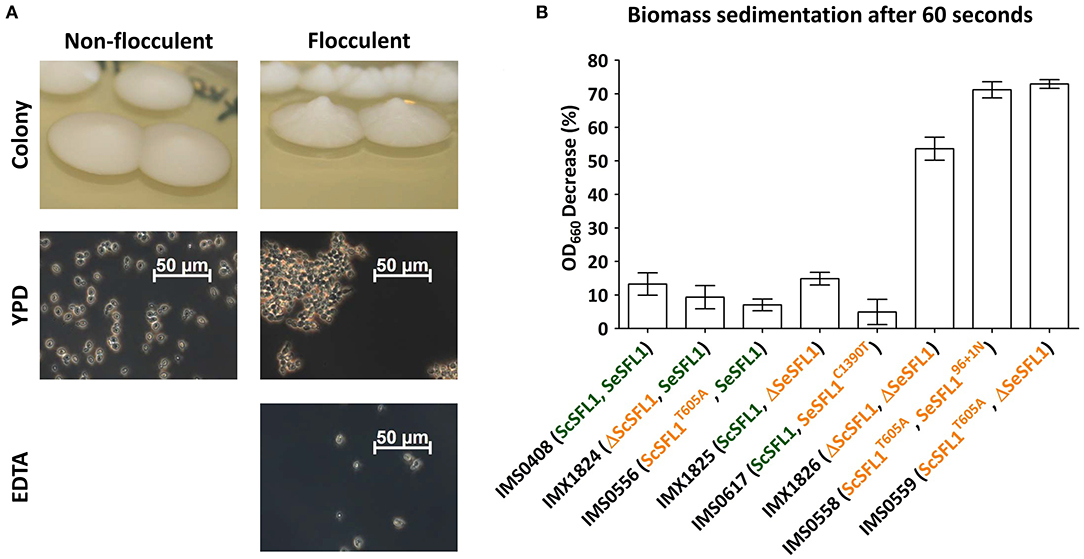
Figure 3. Mutations in ScSFL1 and SeSFL1correlate with flocculation in evolved isolates and reverse engineered strains. (A) Colony morphology and phase-contrast microscopy images (100x) of YPD-grown cell suspensions of the non-evolved, non-flocculent strain (IMS0408), and of a typical flocculent evolved isolate (IMS0558). Resuspension in 50 mM EDTA (pH 7.0) eliminated flocculation. (B) Biomass sedimentation of evolved isolates and engineered strains with mutations in SeSFL1 and/or ScSFL1. Triplicate cultures of all strains were grown on YPD and sedimentation was measured as the decrease in OD660 right underneath the meniscus of a stationary cell suspensions 60 s after the suspension had been vortexed.
Mutations in ScMAL11 Cause Loss of Maltotriose Utilization
All evolved isolates and the unevolved hybrid IMS0408 were grown under brewing conditions at the temperature and wort gravity used during their evolution in micro-aerobic 100 mL bottles to assess their brewing performance. Besides differences in flocculation behavior described above, only 6 isolates evolved on diluted wort at 30°C displayed significantly different brewing performances relative to IMS0408. These six strains all harbored mutations to the ScMAL11 gene, which encodes the sole maltotriose transporter in strain IMS0408. The unevolved IMS0408 consumed 100 ± 0% of the maltotriose in diluted wort, and evolved strains with an intact ScMAL11 genes consumed 98 ± 3%. In contrast, strains IMS0554, IMS0557, IMS0558, IMS0563, IMS0565, and IMS0567, which all harbored mutations in ScMAL11, did not show any maltotriose consumption; instead, the concentration increased by 14 ± 3% on average, presumably due to water evaporation. To test if the mutations affecting ScMAL11 were responsible for the loss of maltotriose utilization, ScMAL11 was deleted in strain IMS0408 using CRISPR-Cas9 gene editing, resulting in strain IMX1698 (mVenus::ΔScmal11). Under the same conditions used to evaluate maltotriose utilization by the evolved strains, strain IMS0408 consumed 97 ± 5% of the maltotriose while strain IMX1698 only consumed 1 ± 0% of the maltotriose. These results confirmed that loss of ScMAL11 function was responsible for loss of maltotriose utilization.
Discussion
Evolution of the laboratory S. cerevisiae x S. eubayanus IMS0408 under simulated lager-brewing conditions yielded a wide array of mutations, including SNPs, INDELs, chromosomal recombinations, aneuploidy and loss of mitochondrial DNA (Table 2, Additional Data File 1). SNPs were the most common type of mutation, with frequencies ranging between 0.004 and 0.039 per division (Figure 4). Based on a genome size of 24.2 Mbp, the rate at which single-nucleotide mutations occurred was between 1.7·10−10 and 1.6·10−9 per nucleotide per cell division, which is similar to a rate of 3.3·10−10 per site per cell division reported for S. cerevisiae (Lynch et al., 2008). At a frequency of 0.003 and 0.010 per cell division, INDELs occurred up to 4.1-fold less frequently than SNPs, in accordance with their two-fold lower occurrence in S. cerevisiae (Lang and Murray, 2008). The higher incidence of both SNPs and INDELs in isolates evolved in full-strength wort (Figure 4) may be related to the higher concentrations of ethanol, a known mutagen, in these cultures (Voordeckers et al., 2015). The rate of loss of mitochondrial DNA varied between 0.0001 and 0.007 per division (Figure 4), and was negatively correlated with the number of generations of selective growth, indicating loss of mitochondrial DNA is selected against. The percentage of respiratory deficient isolates, between 13 and 40% for all evolutions, is consistent with observations during laboratory evolution under oxygen limitation, and has been associated with increased ethanol tolerance (Ibeas and Jimenez, 1997; Taylor et al., 2002). However, loss of reparative capacity is highly undesirable for lager brewing as it impedes biomass propagation (Gibson et al., 2008).
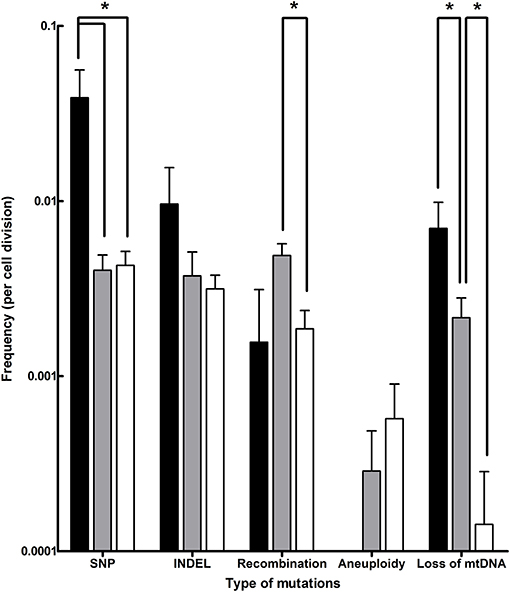
Figure 4. Frequencies of different types of mutations observed in evolved isolates obtained after laboratory evolution of strain IMS0408 under simulated lager fermentation conditions. The mutations identified in all 55 isolates evolved under brewing conditions were classified by type, and the frequency of mutation per cell division was calculated for each isolate based on its estimated number of generations of growth under simulated brewing conditions. Average frequencies of mutation types and standard deviations are shown for isolates evolved on full-strength wort at 12°C (black), on three-fold diluted wort at 12°C (gray) and on threefold diluted wort at 30°C (white). The frequencies are shown on a logarithmic scale, and p-values were determined using Student's t-test. * indicates Significant differences (p-value < 0.05).
The frequency of chromosomal recombinations was estimated between 0.002 and 0.005 per division (Figure 4), which is similar to frequencies reported for S. cerevisiae (Dunham et al., 2002). The observed recombinations were not reciprocal translocations. Instead, in all cases, genetic material was lost due to internal deletions, or genetic material from one chromosome was replaced by an additional copy of genetic material from another chromosome. This abundant loss of heterozygosity is consistent with the evolutionary history of S. cerevisiae (Magwene et al., 2011) and with previously observed loss of genetic material in hybrids (Sipiczki, 2008; Peris et al., 2017; Smukowski Heil et al., 2017, 2018). Under selective conditions, regions from one subgenome can be preferentially affected by loss of heterozygosity due to the fitness effects of genes they harbor. Due to its irreversibility, loss of heterozygosity is a determining mechanism for the evolution of hybrids during domestication (Pérez Través et al., 2014). For example, in an S. cerevisiae x S. uvarum hybrid, the chromosomal region harboring ScPHO84 was preferentially retained at 30°C, while it was lost at the expense of its S. uvarum homolog at 15°C (Smukowski Heil et al., 2017, 2018). Similarly, the superior growth of S. cerevisiae at 30°C relative to S. paradoxus could be linked to S. cerevisiae alleles of eight genes by investigating the effect of loss of heterozygosity in a laboratory hybrid (Weiss et al., 2018). Moreover, the deletion of S. cerevisiae alleles in S. uvarum × S. cerevisiae had strong and varying impacts on fitness under glucose-, sulfate- and phosphate-limitation (Lancaster et al., 2018). Overall, loss of heterozygosity is an irreversible process which enables rapid adaptation of hybrid genomes to the selective pressure of their growth environment. In the present study, loss of heterozygosity notably affected ScMAL11 and SeSFL1, contributing to the acquired flocculation and loss of maltotriose utilization phenotypes. Other observed losses of heterozygosity may be due to genetic drift, or they may yield a selective advantage which we did not identify. Loss of heterozygosity may be advantageous by removing unfavorable dominant alleles, by enabling the expression of favorable recessive alleles, or by resolving redundancy, cross-talk and possible incompatibility between alleles and genes of both subgenomes (Piatkowska et al., 2013; Gibson and Liti, 2015).
Whole-chromosome aneuploidy was the rarest type of mutation, occurring only between 0 and 0.0003 times per division (Figure 4). The emergence of single chromosome aneuploidy in 9% of the isolates after laboratory evolution is similar to observations during laboratory evolution of S. cerevisiae (Yona et al., 2012; Voordeckers et al., 2015; González-Ramos et al., 2016; Gorter de Vries et al., 2017b). However, the observed small extent of aneuploidy starkly contrasts with the massive aneuploidy of S. pastorianus brewing strains (Van Den Broek et al., 2015; Okuno et al., 2016). These differences might of course be attributed to the difference in time scale between 4 months of laboratory evolution and several centuries of domestication. However, they might also be due to differences between the S. cerevisiae × S. eubayanus IMS0408 evolved in this study and the ancestral S. pastorianus hybrid. Firstly, IMS0408 was obtained by crossing a haploid S. cerevisiae strain with a haploid S. eubayanus spore (Hebly et al., 2015). In contrast, the progenitor of brewing strains of S. pastorianus may have resulted from a cross of higher ploidy strains (Okuno et al., 2016). Since higher ploidy leads to higher chromosome missegregation rates, they accumulate chromosome copy number changes faster (Storchova, 2014). In addition, the higher initial ploidy leads to a smaller relative increase of genetic material when an additional copy is gained, which may “buffer” deleterious effects of further changes in ploidy (Torres et al., 2007). Moreover, this laboratory-made hybrid was constructed by crossing a laboratory S. cerevisiae strain with the first discovered S. eubayanus strain, which may differ considerably from the genetic background of the initial S. pastorianus hybrid (Okuno et al., 2016). Therefore, the ancestral S. pastorianus hybrid may have had, or have acquired, mutations that stimulate extensive aneuploidy, such as mutations increasing the rate of chromosome missegregation or mutations increasing the tolerance against aneuploidy associated stresses (Torres et al., 2010). Finally, aneuploidy may have emerged in S. pastorianus by an (aborted) sporulation event, as such events can cause uneven chromosome segregation even in non-hybrid polyploid yeasts (Kim et al., 2017). Regardless of the origin of the extensive aneuploidy of S. pastorianus, our results show that euploid S. cerevisiae × S. eubayanus hybrids are not by definition prone to extensive aneuploidy under brewing-related experimental conditions. For industrial applications, the relative genetic stability of newly generated Saccharomyces hybrid strains reduces the chance of strain deterioration during the many generations involved in large-scale fermentation and/or biomass recycling (Krogerus et al., 2017). Due to the industry practice of limiting yeast biomass re-pitching, the genetic and phenotypic stability observed during 52 generations in 17°P wort at 12°C is sufficient to warrant sufficient strain stability for lager brewing applications.
In addition to showing extensive aneuploidy, S. pastorianus strains harbor numerous chromosomal recombinations. During the laboratory evolution experiments, two types of recombinations were observed: (i) intrachromosomal recombinations resulting in loss of chromosome segments, and (ii) interchromosomal recombinations resulting in loss of one chromosome segment and replacement by an additional copy of a segment from another chromosome, resulting in loss of heterozygosity. While in S. cerevisiae, chromosomal recombinations predominantly occur in repetitive regions of the genome (Dunham et al., 2002; Fontdevila, 2005), here 88% of the observed recombinations occurred within ORFs. The average homology of the recombined ORFs did not exceed the average 85% homology of ORFs in the S. cerevisiae and S. eubayanus subgenomes. Instead, the high rate of recombinations at ORFs could reflect a correlation between transcriptional activity and recombination (Thomas and Rothstein, 1989). In all cases, the reading frames were conserved, resulting in chimeric ORFs which could encode functional chimeric proteins, with altered length and sequences compared to the parental genomes. The potential selective advantage of such chimeric proteins is illustrated by recurring recombinations between ammonium permease MEP2 alleles in a S. cerevisiae and S. uvarum hybrid during laboratory evolution under nitrogen-limited conditions (Dunn et al., 2013). The formation of chimeric ORFs has even led to the emergence of novel gene functions, as illustrated by the formation of a maltotriose transporter by recombination of three non-maltotriose transporter genes in S. eubayanus during laboratory evolution (Brouwers et al., 2018).
The predominant occurrence of recombinations within ORFs in the evolved isolates has also been observed in the genomes of brewing strains of S. pastorianus, which all share identical recombinations at the ZUO1, MAT, HSP82, and XRN1/KEM1 loci (Hewitt et al., 2014; Walther et al., 2014; Okuno et al., 2016). These common recombinations suggest that all S. pastorianus isolates descend from a common ancestor (Monerawela and Bond, 2018). However, S. pastorianus strains may also have emerged from two independent hybridization events, as suggested by the presence of two genetically distinct groups within S. pastorianus (Dunn and Sherlock, 2008; Baker et al., 2015) Indeed, since identical recombinations have been observed in independent evolutions, identical recombinations might reflect parallel evolution due to a strong selective advantage under brewing-related conditions and/or a predisposition of specific loci for recombination (Dunham et al., 2002; Dunn et al., 2013). Of the recombination loci found in the present study, only EFT1 and MAT loci were associated with recombinations in S. pastorianus. Moreover, the recombinations at these loci in the evolved isolates were different from those in S. pastorianus (Hewitt et al., 2014; Walther et al., 2014; Okuno et al., 2016; Monerawela and Bond, 2017). All interchromosomal recombinations observed in this study were unique. These results, obtained under brewing-related conditions, are consistent with the notion that recombination sites are largely aleatory and that all modern S. pastorianus strains share the same recombinations because they descend from a single hybrid ancestor.
Different recombination events resulted in the loss of heterozygosity, in four isolates each, of the right arm of SeCHRXV, including SeSFL1, and of the right arm of ScCHRXI, including ScMAL11. These events directly affected two phenotypes relevant for brewing fermentation: calcium-dependent flocculation, which led to fast biomass sedimentation, and loss of maltotriose utilization. Biomass sedimentation can be strongly selected for in sequential batch bioreactors, as it increases the chance that cells are retained in the bioreactor during the emptying phase (Oud et al., 2013; Hope et al., 2017). A similar selective advantage is likely to have played a role in the early domestication of S. pastorianus, as sedimenting yeast remaining in fermentation vessels was more likely to be used in a next fermentation. Flocculation is a key characteristic of current lager-brewing yeasts (also referred to as bottom-fermenting yeasts), as it simplifies biomass separation at the end of the fermentation (Ferreira et al., 2010). The present study illustrates how this aspect of brewing yeast domestication can be rapidly reproduced under simulated laboratory conditions.
At first glance, loss of the ability to utilize maltotriose, an abundant fermentable sugar in wort, appears to be undesirable from an evolutionary perspective. However, as demonstrated in studies on laboratory evolution of S. cerevisiae in sequential batch cultures on sugar mixtures, the selective advantage of consuming a specific sugar from a sugar mixture correlates with the number of generations of growth on that sugar during each cultivation cycle (Wisselink et al., 2009; Verhoeven et al., 2018). Saccharomyces yeasts, including strain IMS0408 generally prefer glucose and maltose over maltotriose (Alves et al., 2008; Hebly et al., 2015; Brickwedde et al., 2017). As a consequence, maltotriose consumption from wort typically only occurs when growth has already ceased due to oxygen limitation and/or nitrogen source depletion, which results in few or no generations of growth on this trisaccharide. However, loss of maltotriose utilization in six isolates in two independent evolution experiments strongly suggests that loss of ScMAL11 expression was not merely neutral but even conferred a selective advantage. These results are consistent with the existence of many S. pastorianus strains with poor maltotriose utilization and with the truncation of ScMAL11 in all S. pastorianus strains, including good maltotriose utilizers (Vidgren et al., 2009; Gibson et al., 2013). In the latter strains, maltotriose utilization depends on alternative transporters such as Mty1 (Dietvorst et al., 2005; Salema-Oom et al., 2005). It is therefore unclear if a selective advantage of the loss of ScMAL11 reflects specific properties of this gene or its encoded transporter or, alternatively, a general negative impact of maltotriose utilization under brewing-related conditions. In analogy with observations on maltose utilization by S. cerevisiae, unrestricted Mal11-mediated maltotriose-proton symport might cause maltotriose-accelerated death (Postma et al., 1990; Jansen et al., 2004). Alternatively, expression of the Mal11 transporter might compete with superior maltose transporters for intracellular trafficking, membrane integration and/or membrane space (Vidgren, 2010; Libkind et al., 2011). Indeed, laboratory evolution to obtain improved maltotriose utilization resulted in reduced maltose uptake in S. pastorianus (Brickwedde et al., 2017).
Saccharomyces hybrids are commonly applied for industrial applications such as lager beer brewing and wine fermentation (Naumov et al., 2001; González et al., 2006; Libkind et al., 2011). Recently, novel hybrids have been generated, that performed well under a broad range of industrially-relevant conditions (Bellon et al., 2011; Hebly et al., 2015; Lopandic et al., 2016; Krogerus et al., 2017; Nikulin et al., 2017; Peris et al., 2017). While the performance and phenotypic diversity of laboratory hybrids support their application in industrial processes, further strain development of such hybrids could improve their performance (Steensels et al., 2014; Krogerus et al., 2017). Especially in food and beverage fermentation processes, consumer acceptance issues largely preclude use of targeted genetic modification techniques. Laboratory evolution offers an interesting alternative strategy for “non-GMO” strain improvement (Bachmann et al., 2015). However, as exemplified by the loss, in independent laboratory evolution experiments, of MAL11 and of the mitochondrial genome, mutations that yield increased fitness under simulated industrial fermentation conditions are not necessarily advantageous for industrial performance. Therefore, instead of faithfully reconstructing industrial conditions in the laboratory, laboratory evolution experiments should be designed to specifically select for desired phenotypes. For example, a recent study illustrated how maltotriose fermentation kinetics of an S. pastorianus hybrid could be improved by laboratory evolution in carbon-limited chemostats grown on a maltotriose-enriched sugar mixture (Brickwedde et al., 2017).
Data Availability
The datasets generated by genome sequencing for this study can be found in the NCBI database (https://www.ncbi.nlm.nih.gov/) under the Bioproject PRJNA506072.
Author Contributions
AG planned and executed the laboratory evolution, made single colony isolates, prepared genomic DNA and characterized ploidy and respiratory deficiency. AG, AvA, LK and LJ characterized flocculation and maltotriose utilization of the isolates. AG and MV performed the reverse engineering of deletions of SFL1 and MAL11 and characterized the reverse engineered phenotypes. AG and MvdB performed the bioinformatics analysis. AG, JP, and J-MD wrote the manuscript. AS, NB, and TA provided critical feedback throughout the study. J-MD and JP supervised the study. All authors read and approved the final manuscript.
Funding
This work was performed within the BE-Basic R&D Program (grant numbers TKIBE01003 and TKIBE01001, http://www.be-basic.org/), which was granted an FES subsidy from the Dutch Ministry of Economic Affairs, Agriculture and Innovation (EL&I).
Conflict of Interest Statement
The authors declare that the research was conducted in the absence of any commercial or financial relationships that could be construed as a potential conflict of interest.
Acknowledgements
We thank Erik de Hulster, Xavier Hakkaart, and Robert Mans for sharing their expertise in fermentation, Marijke Luttik for her expertise in Flow cytometry and Nikola Gyurchev for assembling plasmids pUDP104 and pUDP105. We are thankful to Niels Kuijpers (HEINEKEN Supply Chain B.V.), and Jan-Maarten Geertman (HEINEKEN Supply Chain B.V.) for their support and for critically reading the manuscript. A pre-print version of this article has been uploaded on bioRxiv (Gorter de Vries et al., 2018b).
Supplementary Material
The Supplementary Material for this article can be found online at: https://www.frontiersin.org/articles/10.3389/fgene.2019.00242/full#supplementary-material
Data Sheet S1. Mutations and mutation rates in the IMS0408-derived isolates obtained after laboratory evolution based on whole genome sequencing analysis. (A) SNPs identified in the isolates. (B) INDELs identified in the isolates. (C) chromosomal recombinations identified in the isolates. (D) whole chromosome aneuploidy identified in the isolates. (E) loss of mitochondrial DNA identified in the isolates. (F) mutation rates in the isolates.
References
Alves, S. L., Herberts, R. A., Hollatz, C., Trichez, D., Miletti, L. C., De Araujo, P. S., et al. (2008). Molecular analysis of maltotriose active transport and fermentation by Saccharomyces cerevisiae reveals a determinant role for the AGT1 permease. Appl. Environ. Microbiol. 74, 1494–1501. doi: 10.1128/AEM.02570-07
Andreasen, A. A., and Stier, T. (1953). Anaerobic nutrition of Saccharomyces cerevisiae. I. ergosterol requirement for growth in a defined medium. J. Cell. Comp. Physiol. 41, 23–36.
Atsushi, F., Yoshiko, K., Satoru, K., Yoshio, M., Shinichi, M., and Harumi, K. (1989). Domains of the SFL1 protein of yeasts are homologous to Myc oncoproteins or yeast heat-shock transcription factor. Gene 85, 321–328. doi: 10.1016/0378-1119(89)90424-1
Bachmann, H., Pronk, J. T., Kleerebezem, M., and Teusink, B. (2015). Evolutionary engineering to enhance starter culture performance in food fermentations. Curr. Opin. Biotechnol. 32, 1–7. doi: 10.1016/j.copbio.2014.09.003
Bachmann, H., Starrenburg, M. J., Molenaar, D., Kleerebezem, M., and Van Hylckama Vlieg, J. E. (2012). Microbial domestication signatures of Lactococcus lactis can be reproduced by experimental evolution. Genome Res. 22, 115–124. doi: 10.1101/gr.121285.111
Baker, E., Wang, B., Bellora, N., Peris, D., Hulfachor, A. B., Koshalek, J. A., et al. (2015). The genome sequence of Saccharomyces eubayanus and the domestication of lager-brewing yeasts. Mol. Biol. Evol. 32, 2818–2831. doi: 10.1093/molbev/msv168
Bankevich, A., Nurk, S., Antipov, D., Gurevich, A. A., Dvorkin, M., Kulikov, A. S., et al. (2012). SPAdes: a new genome assembly algorithm and its applications to single-cell sequencing. J. Comput. Biol. 19, 455–477. doi: 10.1089/cmb.2012.0021
Bellon, J. R., Eglinton, J. M., Siebert, T. E., Pollnitz, A. P., Rose, L., De Barros Lopes, M., et al. (2011). Newly generated interspecific wine yeast hybrids introduce flavour and aroma diversity to wines. Appl. Microbiol. Biotechnol. 91, 603–612. doi: 10.1007/s00253-011-3294-3
Botstein, D., Chervitz, S. A., and Cherry, M. (1997). Yeast as a model organism. Science 277, 1259–1260. doi: 10.1126/science.277.5330.1259
Brickwedde, A., Brouwers, N., Van Den Broek, M., Gallego Murillo, J. S., Fraiture, J. L., Pronk, J. T., et al. (2018). Structural, physiological and regulatory analysis of maltose transporter genes in Saccharomyces eubayanus CBS 12357T. Front. Microbiol. 9:1786. doi: 10.3389/fmicb.2018.01786
Brickwedde, A., Van Den Broek, M., Geertman, J.-M. A., Magalhães, F., Kuijpers, N. G. A., Gibson, B., et al. (2017). Evolutionary engineering in chemostat cultures for improved maltotriose fermentation kinetics in Saccharomyces pastorianus lager brewing yeast. Front. Microbiol. 8:1690. doi: 10.3389/fmicb.2017.01690
Briggs, D. E., Brookes, P., Stevens, R., and Boulton, C. (2004). Brewing: Science and Practice. Cambridge: Elsevier.
Brouwers, N., Gorter de Vries, A. R., Van Den Broek, M., Weening, S. M., Schuurman, T. D. E., Kuijpers, N. G. A., et al. (2018). In vivo recombination of Saccharomyces eubayanus maltose-transporter genes yields a chimeric transporter that enables maltotriose fermentation. PLoS Genet. biRxiv [preprint]. doi: 10.1101/428839
Chambers, S. R., Hunter, N., Louis, E. J., and Borts, R. H. (1996). The mismatch repair system reduces meiotic homeologous recombination and stimulates recombination-dependent chromosome loss. Mol. Cell Biol. 16, 6110–6120. doi: 10.1128/MCB.16.11.6110
Chen, K., Wallis, J. W., Mclellan, M. D., Larson, D. E., Kalicki, J. M., Pohl, C. S., et al. (2009). BreakDancer: an algorithm for high-resolution mapping of genomic structural variation. Nat. Methods 6:677. doi: 10.1038/nmeth.1363
Coloretti, F., Zambonelli, C., and Tini, V. (2006). Characterization of flocculent Saccharomyces interspecific hybrids for the production of sparkling wines. Food Microbiol. 23, 672–676. doi: 10.1016/j.fm.2005.11.002
Delneri, D., Colson, I., Grammenoudi, S., Roberts, I. N., Louis, E. J., and Oliver, S. G. (2003). Engineering evolution to study speciation in yeasts. Nature 422:68. doi: 10.1038/nature01418
Dietvorst, J., Londesborough, J., and Steensma, H. (2005). Maltotriose utilization in lager yeast strains: MTT1 encodes a maltotriose transporter. Yeast 22, 775–788. doi: 10.1002/yea.1279
Dunham, M. J., Badrane, H., Ferea, T., Adams, J., Brown, P. O., Rosenzweig, F., et al. (2002). Characteristic genome rearrangements in experimental evolution of Saccharomyces cerevisiae. Proc. Natl. Acad. Sci. U.S.A. 99, 16144–16149. doi: 10.1073/pnas.242624799
Dunn, B., Paulish, T., Stanbery, A., Piotrowski, J., Koniges, G., Kroll, E., et al. (2013). Recurrent rearrangement during adaptive evolution in an interspecific yeast hybrid suggests a model for rapid introgression. PLoS Genet. 9:e1003366. doi: 10.1371/journal.pgen.1003366
Dunn, B., and Sherlock, G. (2008). Reconstruction of the genome origins and evolution of the hybrid lager yeast Saccharomyces pastorianus. Genome Res. 18, 1610–1623. doi: 10.1101/gr.076075.108
Engler, C., Kandzia, R., and Marillonnet, S. (2008). A one pot, one step, precision cloning method with high throughput capability. PLoS ONE 3:e3647. doi: 10.1371/journal.pone.0003647
Ferreira, I., Pinho, O., Vieira, E., and Tavarela, J. (2010). Brewer's Saccharomyces yeast biomass: characteristics and potential applications. Trends Food Sci. Technol. 21, 77–84. doi: 10.1016/j.tifs.2009.10.008
Fontdevila, A. (2005). Hybrid genome evolution by transposition. Cytogenet. Genome Res. 110, 49–55. doi: 10.1159/000084937
Gibbons, J. G., and Rinker, D. C. (2015). The genomics of microbial domestication in the fermented food environment. Curr. Opin. Genet. Dev. 35, 1–8. doi: 10.1016/j.gde.2015.07.003
Gibbons, J. G., Salichos, L., Slot, J. C., Rinker, D. C., Mcgary, K. L., King, J. G., et al. (2012). The evolutionary imprint of domestication on genome variation and function of the filamentous fungus Aspergillus oryzae. Curr. Biol. 22, 1403–1409. doi: 10.1016/j.cub.2012.05.033
Gibson, B., and Liti, G. (2015). Saccharomyces pastorianus: genomic insights inspiring innovation for industry. Yeast 32, 17–27. doi: 10.1002/yea.3033
Gibson, B., Prescott, K., and Smart, K. (2008). Petite mutation in aged and oxidatively stressed ale and lager brewing yeast. Lett. Appl. Microbiol. 46, 636–642. doi: 10.1111/j.1472-765X.2008.02360.x
Gibson, B. R., Lawrence, S. J., Leclaire, J. P., Powell, C. D., and Smart, K. A. (2007). Yeast responses to stresses associated with industrial brewery handling. FEMS Microbiol. Lett. 31, 535–569. doi: 10.1111/j.1574-6976.2007.00076.x
Gibson, B. R., Storgårds, E., Krogerus, K., and Vidgren, V. (2013). Comparative physiology and fermentation performance of Saaz and Frohberg lager yeast strains and the parental species Saccharomyces eubayanus. Yeast 30, 255–266. doi: 10.1002/yea.2960
González, S. S., Barrio, E., Gafner, J., and Querol, A. (2006). Natural hybrids from Saccharomyces cerevisiae, Saccharomyces bayanus and Saccharomyces kudriavzevii in wine fermentations. FEMS Yeast Res. 6, 1221–1234. doi: 10.1111/j.1567-1364.2006.00126.x
González-Ramos, D., Gorter de Vries, A. R., Grijseels, S. S., Berkum, M. C., Swinnen, S., Broek, M., et al. (2016). A new laboratory evolution approach to select for constitutive acetic acid tolerance in Saccharomyces cerevisiae and identification of causal mutations. Biotechnol. Biofuels 9:173. doi: 10.1186/s13068-016-0583-1
Gorter de Vries, A.R., Couwenberg, L.G.F., Van Den Broek, M., De La Torre Cortes, P., Ter Horst, J., Pronk, J.T., and Daran, J.-M.G. (2018a). Allele-specific genome editing using CRISPR-Cas9 is associated with loss of heterozygosity in diploid yeast. Nucleic Acids Res. 47, 1362–1372.
Gorter de Vries, A. R., De Groot, P. A., Van Den Broek, M., and Daran, J. M. G. (2017a). CRISPR-Cas9 mediated gene deletions in lager yeast Saccharomyces pastorianus. Microb. Cell Fact. 16:222. doi: 10.1186/s12934-017-0835-1
Gorter de Vries, A. R., Pronk, J. T., and Daran, J. M. G. (2017b). Industrial relevance of chromosomal copy number variation in Saccharomyces yeasts. Appl. Environ. Microbiol. 83:e03206–03216. doi: 10.1128/AEM.03206-16
Gorter de Vries, A. R., Voskamp, M. A., Van Aalst, A. C. A., Kristensen, L. H., Jansen, L., Van Den Broek, M., et al. (2018b). Laboratory evolution of a Saccharomyces cerevisiae x S. eubayanus hybrid under simulated lager-brewing conditions: genetic diversity and phenotypic convergence. BioRxiv. doi: 10.1101/476929.
Haase, S. B., and Reed, S. I. (2002). Improved flow cytometric analysis of the budding yeast cell cycle. Cell Cycle 1, 117–121. doi: 10.4161/cc.1.2.114
Hebly, M., Brickwedde, A., Bolat, I., Driessen, M. R. M., De Hulster, E. A., Pronk, J. T., et al. (2015). S. cerevisiae × S. eubayanus interspecific hybrid, the best of both worlds and beyond. FEMS Yeast Res. 15:fov005. doi: 10.1093/femsyr/fov005
Hewitt, S. K., Donaldson, I. J., Lovell, S. C., and Delneri, D. (2014). Sequencing and characterisation of rearrangements in three S. pastorianus strains reveals the presence of chimeric genes and gives evidence of breakpoint reuse. PLoS ONE 9:e92203. doi: 10.1371/journal.pone.0092203
Hittinger, C. T. (2013). Saccharomyces diversity and evolution: a budding model genus. Trends Genet. 29, 309–317. doi: 10.1016/j.tig.2013.01.002
Hope, E. A., Amorosi, C. J., Miller, A. W., Dang, K., Heil, C. S., and Dunham, M. J. (2017). Experimental evolution reveals favored adaptive routes to cell aggregation in yeast. Genetics 206, 1153–1167. doi: 10.1534/genetics.116.198895
Ibeas, J. I., and Jimenez, J. (1997). Mitochondrial DNA loss caused by ethanol in Saccharomyces flor yeasts. Appl. Environ. Microbiol. 63, 7–12.
Jansen, M. L., Daran-Lapujade, P., De Winde, J. H., Piper, M. D., and Pronk, J. T. (2004). Prolonged maltose-limited cultivation of Saccharomyces cerevisiae selects for cells with improved maltose affinity and hypersensitivity. Appl. Environ. Microbiol. 70, 1956–1963. doi: 10.1128/AEM.70.4.1956-1963.2004
Kim, S. R., Skerker, J. M., Kong, I. I., Kim, H., Maurer, M. J., Zhang, G.-C., et al. (2017). Metabolic engineering of a haploid strain derived from a triploid industrial yeast for producing cellulosic ethanol. Metabolic Eng. 40, 176–185. doi: 10.1016/j.ymben.2017.02.006
Krogerus, K., Magalhães, F., Vidgren, V., and Gibson, B. (2017). Novel brewing yeast hybrids: creation and application. Appl. Microbiol. Biotechnol. 101, 65–78. doi: 10.1007/s00253-016-8007-5
Lancaster, S. M., Payen, C., Heil, C. S., and Dunham, M. J. (2018). Fitness benefits of loss of heterozygosity in Saccharomyces hybrids. BioRxiv. biRxiv [preprint]. doi: 10.1101/452748
Lang, G. I., and Murray, A. W. (2008). Estimating the per-base-pair mutation rate in the yeast Saccharomyces cerevisiae. Genetics 178, 67–82. doi: 10.1534/genetics.107.071506
Li, H., and Durbin, R. (2010). Fast and accurate long-read alignment with burrows–wheeler transform. Bioinformatics 26, 589–595. doi: 10.1093/bioinformatics/btp698
Li, H., Handsaker, B., Wysoker, A., Fennell, T., Ruan, J., Homer, N., et al. (2009). The sequence alignment/map format and SAMtools. Bioinformatics 25, 2078–2079. doi: 10.1093/bioinformatics/btp352
Libkind, D., Hittinger, C. T., Valério, E., Gonçalves, C., Dover, J., Johnston, M., et al. (2011). Microbe domestication and the identification of the wild genetic stock of lager-brewing yeast. Proc. Natl. Acad. Sci. U.S.A. 108, 14539–14544. doi: 10.1073/pnas.1105430108
Liti, G., Barton, D. B., and Louis, E. J. (2006). Sequence diversity, reproductive isolation and species concepts in Saccharomyces. Genetics 174, 839–850. doi: 10.1534/genetics.106.062166
Lopandic, K., Pfliegler, W. P., Tiefenbrunner, W., Gangl, H., Sipiczki, M., and Sterflinger, K. (2016). Genotypic and phenotypic evolution of yeast interspecies hybrids during high-sugar fermentation. Appl. Microbiol. Biotechnol. 100, 6331–6343. doi: 10.1007/s00253-016-7481-0
Lynch, M, Sung, W, Morris, K, et al. (2008) A genome-wide view of the spectrum of spontaneous mutations in yeast. Proc. Natl. Acad. Sci. U.S.A. 105 9272–9277.
Magwene, P. M., Kayikçi, Ö., Granek, J. A., Reininga, J. M., Scholl, Z., and Murray, D. (2011). Outcrossing, mitotic recombination, and life-history trade-offs shape genome evolution in Saccharomyces cerevisiae. Proc. Natl. Acad. Sci. U.S.A. 108, 1987–1992. doi: 10.1073/pnas.1012544108
Marsit, S., and Dequin, S. (2015). Diversity and adaptive evolution of Saccharomyces wine yeast: a review. FEMS Yeast Res. 15:fov067. doi: 10.1093/femsyr/fov067
Meussdoerffer, F. G. (2009). “A comprehensive history of beer brewing,” in Handbook of Brewing: Processes, Technology, Markets (Eßlinger: Wiley-VCH), 1–42.
Monerawela, C., and Bond, U. (2017). Recombination sites on hybrid chromosomes in Saccharomyces pastorianus share common sequence motifs and define a complex evolutionary relationship between group I and II lager yeasts. FEMS Yeast Res. 17:fox047. doi: 10.1093/femsyr/fox047
Monerawela, C., and Bond, U. (2018). The hybrid genomes of Saccharomyces pastorianus: a current perspective. Yeast 35, 39–50. doi: 10.1002/yea.3250
Naseeb, S., James, S. A., Alsammar, H., Michaels, C. J., Gini, B., Nueno-Palop, C., et al. (2017). Saccharomyces jurei sp nov., isolation and genetic identification of a novel yeast species from Quercus robur. Int. J. Syst. Evol. Microbiol. 67, 2046–2052. doi: 10.1099/ijsem.0.002013
Naumov, G. I., Nguyen, H.-V., Naumova, E. S., Michel, A., Aigle, M., and Gaillardin, C. (2001). Genetic identification of Saccharomyces bayanus var. uvarum, a cider-fermenting yeast. Int. J. Food Microbiol. 65, 163–171. doi: 10.1016/S0168-1605(00)00515-8
Newlon, C., Lipchitz, L., Collins, I., Deshpande, A., Devenish, R., Green, R., et al. (1991). Analysis of a circular derivative of Saccharomyces cerevisiae chromosome III: a physical map and identification and location of ARS elements. Genetics 129, 343–357.
Nijkamp, J. F., Van Den Broek, M., Datema, E., De Kok, S., Bosman, L., Luttik, M. A., et al. (2012). De novo sequencing, assembly and analysis of the genome of the laboratory strain Saccharomyces cerevisiae CEN. PK113–7D, a model for modern industrial biotechnology. Microb. Cell Fact. 11:36. doi: 10.1186/1475-2859-11-36
Nikulin, J., Krogerus, K., and Gibson, B. (2017). Alternative Saccharomyces interspecies hybrid combinations and their potential for low-temperature wort fermentation. Yeast 35, 113–127. doi: 10.1002/yea.3246
Okuno, M., Kajitani, R., Ryusui, R., Morimoto, H., Kodama, Y., and Itoh, T. (2016). Next-generation sequencing analysis of lager brewing yeast strains reveals the evolutionary history of interspecies hybridization. DNA Res. 23, 67–80. doi: 10.1093/dnares/dsv037
Oud, B., Guadalupe-Medina, V., Nijkamp, J. F., De Ridder, D., Pronk, J. T., Van Maris, A. J., et al. (2013). Genome duplication and mutations in ACE2 cause multicellular, fast-sedimenting phenotypes in evolved Saccharomyces cerevisiae. Proc. Natl. Acad. Sci. U.S.A. 110:E4223-E4231. doi: 10.1073/pnas.1305949110
Pérez Través, L., Lopes, C. A., Barrio Esparducer, E., and Querol Simón, A. (2014). Stabilization process in Saccharomyces intra and interspecific hybrids in fermentative conditions. Int. Microbiol. 17, 213–224. doi: 10.2436/20.1501.01.224
Peris, D., Moriarty, R. V., Alexander, W. G., Baker, E., Sylvester, K., Sardi, M., et al. (2017). Hybridization and adaptive evolution of diverse Saccharomyces species for cellulosic biofuel production. Biotechnol. Biofuels 10:78. doi: 10.1186/s13068-017-0763-7
Piatkowska, E. M., Naseeb, S., Knight, D., and Delneri, D. (2013). Chimeric protein complexes in hybrid species generate novel phenotypes. PLoS Genet. 9:e1003836. doi: 10.1371/journal.pgen.1003836
Postma, E., Verduyn, C., Kuiper, A., Scheffers, W. A., and Van Dijken, J. P. (1990). Substrate-accelerated death of Saccharomyces cerevisiae CBS 8066 under maltose stress. Yeast 6, 149–158. doi: 10.1002/yea.320060209
Querol, A., and Bond, U. (2009). The complex and dynamic genomes of industrial yeasts. FEMS Microbiol. Lett. 293, 1–10. doi: 10.1111/j.1574-6968.2008.01480.x
Robinson, J. T., Thorvaldsdóttir, H., Winckler, W., Guttman, M., Lander, E. S., Getz, G., et al. (2011). Integrative genomics viewer. Nat. Biotechnol. 29:24. doi: 10.1038/nbt.1754
Salazar, A. N., Gorter de Vries, A. R., Van Den Broek, M., Wijsman, M., De La Torre Cortés, P., Brickwedde, A., et al. (2017). Nanopore sequencing enables near-complete de novo assembly of Saccharomyces cerevisiae reference strain CEN. PK113–7D. FEMS Yeast Res. 17. doi: 10.1093/femsyr/fox074
Salema-Oom, M., Pinto, V. V., Gonçalves, P., and Spencer-Martins, I. (2005). Maltotriose utilization by industrial Saccharomyces strains: characterization of a new member of the α-glucoside transporter family. Appl. Environ. Microbiol. 71, 5044–5049. doi: 10.1128/AEM.71.9.5044-5049.2005
Santaguida, S., and Amon, A. (2015). Short-and long-term effects of chromosome mis-segregation and aneuploidy. Nat. Rev. Mol. Cell. Biol. 16:473. doi: 10.1038/nrm4025
Sheltzer, J. M., Blank, H. M., Pfau, S. J., Tange, Y., George, B. M., Humpton, T. J., et al. (2011). Aneuploidy drives genomic instability in yeast. Science 333, 1026–1030. doi: 10.1126/science.1206412
Sipiczki, M. (2008). Interspecies hybridization and recombination in Saccharomyces wine yeasts. FEMS Yeast Res. 8, 996–1007. doi: 10.1111/j.1567-1364.2008.00369.x
Smukowski Heil, C. S., Desevo, C. G., Pai, D. A., Tucker, C. M., Hoang, M. L., and Dunham, M. J. (2017). Loss of heterozygosity drives adaptation in hybrid yeast. Mol. Biol. Evol. 34, 1596–1612. doi: 10.1093/molbev/msx098
Smukowski Heil, C. S., Large, C. R., Patterson, K., and Dunham, M. J. (2018). Temperature preference biases parental genome retention during hybrid evolution. BioRxiv. biRxiv [preprint]. doi: 10.1101/429803
Solis-Escalante, D., Kuijpers, N. G. A., Nadine, B., Bolat, I., Bosman, L., Pronk, J. T., et al. (2013). amdSYM, a new dominant recyclable marker cassette for Saccharomyces cerevisiae. FEMS Yeast Res. 13, 126–139. doi: 10.1111/1567-1364.12024
Steensels, J., Snoek, T., Meersman, E., Nicolino, M. P., Voordeckers, K., and Verstrepen, K. J. (2014). Improving industrial yeast strains: exploiting natural and artificial diversity. FEMS Microbiol. Rev. 38, 947–995. doi: 10.1111/1574-6976.12073
Storchova, Z. (2014). Ploidy changes and genome stability in yeast. Yeast 31, 421–430. doi: 10.1002/yea.3037
Tanaka, K., Lin, B. K., Wood, D. R., and Tamanoi, F. (1991). IRA2, an upstream negative regulator of RAS in yeast, is a RAS GTPase-activating protein. Proc. Natl. Acad. Sci. U.S.A. 88, 468–472. doi: 10.1073/pnas.88.2.468
Tanaka, K., Nakafuku, M., Satoh, T., Marshall, M. S., Gibbs, J. B., Matsumoto, K., et al. (1990). S. cerevisiae genes IRA1 and IRA2 encode proteins that may be functionally equivalent to mammalian ras GTPase activating protein. Cell 60, 803–807. doi: 10.1016/0092-8674(90)90094-U
Taylor, D. R., Zeyl, C., and Cooke, E. (2002). Conflicting levels of selection in the accumulation of mitochondrial defects in Saccharomyces cerevisiae. Proc. Natl. Acad. Sci. U.S.A. 99, 3690–3694. doi: 10.1073/pnas.072660299
Thomas, B. J., and Rothstein, R. (1989). Elevated recombination rates in transcriptionally active DNA. Cell 56, 619–630. doi: 10.1016/0092-8674(89)90584-9
Torres, E. M., Dephoure, N., Panneerselvam, A., Tucker, C. M., Whittaker, C. A., Gygi, S. P., et al. (2010). Identification of aneuploidy-tolerating mutations. Cell 143, 71–83. doi: 10.1016/j.cell.2010.08.038
Torres, E. M., Sokolsky, T., Tucker, C. M., Chan, L. Y., Boselli, M., Dunham, M. J., et al. (2007). Effects of aneuploidy on cellular physiology and cell division in haploid yeast. Science 317, 916–924. doi: 10.1126/science.1142210
Van Den Broek, M., Bolat, I., Nijkamp, J. F., Ramos, E., Luttik, M. a. H., et al. (2015). Chromosomal copy number variation in Saccharomyces pastorianus is evidence for extensive genome dynamics in industrial lager brewing strains. Appl. Environ. Microbiol. 81, 6253–6267. doi: 10.1128/AEM.01263-15
Verduyn, C., Postma, E., Scheffers, W. A., and Van Dijken, J. P. (1992). Effect of benzoic acid on metabolic fluxes in yeasts: a continuous-culture study on the regulation of respiration and alcoholic fermentation. Yeast 8, 501–517. doi: 10.1002/yea.320080703
Verhoeven, M. D., De Valk, S. C., Daran, J.-M. G., Van Maris, A. J., and Pronk, J. T. (2018). Fermentation of glucose-xylose-arabinose mixtures by a synthetic consortium of single-sugar-fermenting Saccharomyces cerevisiae strains. FEMS Yeast Res. 18:foy075. doi: 10.1093/femsyr/foy075
Vidgren, V. (2010). Maltose and Maltotriose Transport Into ale and Lager Brewer's Yeast Strains. Helsinki: VTT publications.
Vidgren, V., Huuskonen, A., Virtanen, H., Ruohonen, L., and Londesborough, J. (2009). Improved fermentation performance of a lager yeast after repair of its AGT1 maltose and maltotriose transporter genes. Appl. Environ. Microbiol. 75, 2333–2345. doi: 10.1128/AEM.01558-08
Voordeckers, K., Kominek, J., Das, A., Espinosa-Cantú, A., De Maeyer, D., Arslan, A., et al. (2015). Adaptation to high ethanol reveals complex evolutionary pathways. PLoS Genet. 11:e1005635. doi: 10.1371/journal.pgen.1005635
Wach, A., Brachat, A., Pöhlmann, R., and Philippsen, P. (1994). New heterologous modules for classical or PCR-based gene disruptions in Saccharomyces cerevisiae. Yeast 10, 1793–1808. doi: 10.1002/yea.320101310
Walker, B. J., Abeel, T., Shea, T., Priest, M., Abouelliel, A., Sakthikumar, S., et al. (2014). Pilon: an integrated tool for comprehensive microbial variant detection and genome assembly improvement. PLoS ONE 9:e112963. doi: 10.1371/journal.pone.0112963
Walther, A., Hesselbart, A., and Wendland, J. (2014). Genome sequence of Saccharomyces carlsbergensis, the world's first pure culture lager yeast. G3 (Bethesda) 4, 783–793. doi: 10.1534/g3.113.010090
Weiss, C. V., Roop, J. I., Hackley, R. K., Chuong, J. N., Grigoriev, I. V., Arkin, A. P., et al. (2018). Genetic dissection of interspecific differences in yeast thermotolerance. Nat. Genet. 50, 1501–1504. doi: 10.1038/s41588-018-0243-4
Wisselink, H. W., Toirkens, M. J., Wu, Q., Pronk, J. T., and Van Maris, A. J. A. (2009). Novel evolutionary engineering approach for accelerated utilization of glucose, xylose, and arabinose mixtures by engineered Saccharomyces cerevisiae strains. Appl. Environ. Microbiol. 75, 907–914. doi: 10.1128/AEM.02268-08
Keywords: Saccharomyces pastorianus, loss of heterozygosity, laboratory evolution, domestication, maltotriose utilization, flocculation
Citation: Gorter de Vries AR, Voskamp MA, van Aalst ACA, Kristensen LH, Jansen L, van den Broek M, Salazar AN, Brouwers N, Abeel T, Pronk JT and Daran J-MG (2019) Laboratory Evolution of a Saccharomyces cerevisiae × S. eubayanus Hybrid Under Simulated Lager-Brewing Conditions. Front. Genet. 10:242. doi: 10.3389/fgene.2019.00242
Received: 27 November 2018; Accepted: 04 March 2019;
Published: 29 March 2019.
Edited by:
Jean Marie François, UMR5504 Laboratoire d'Ingénierie des Systèmes Biologiques et des Procédés (LISBP), FranceReviewed by:
Soo Rin Kim, Kyungpook National University, South KoreaJose Sampaio, Universidade nova de Lisboa, Portugal
Copyright © 2019 Gorter de Vries, Voskamp, van Aalst, Kristensen, Jansen, van den Broek, Salazar, Brouwers, Abeel, Pronk and Daran. This is an open-access article distributed under the terms of the Creative Commons Attribution License (CC BY). The use, distribution or reproduction in other forums is permitted, provided the original author(s) and the copyright owner(s) are credited and that the original publication in this journal is cited, in accordance with accepted academic practice. No use, distribution or reproduction is permitted which does not comply with these terms.
*Correspondence: Jean-Marc G. Daran, ai5nLmRhcmFuQHR1ZGVsZnQubmw=